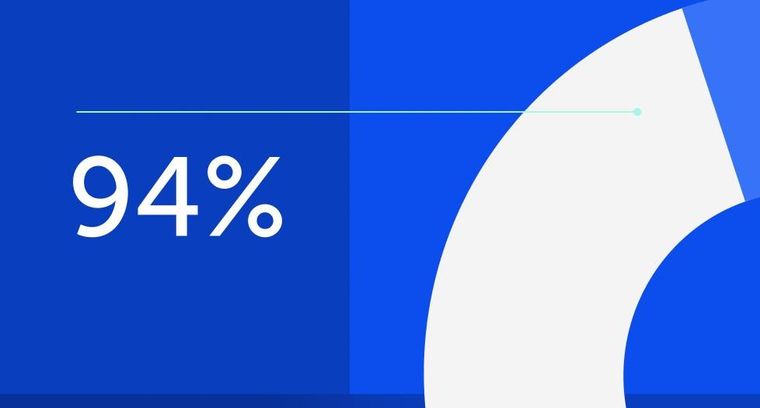
94% of researchers rate our articles as excellent or good
Learn more about the work of our research integrity team to safeguard the quality of each article we publish.
Find out more
REVIEW article
Front. Microbiol., 24 November 2022
Sec. Microbiotechnology
Volume 13 - 2022 | https://doi.org/10.3389/fmicb.2022.1059777
Pichia pastoris (syn. Komagataella spp.) has attracted extensive attention as an efficient platform for recombinant protein (RP) production. For obtaining a higher protein titer, many researchers have put lots of effort into different areas and made some progress. Here, we summarized the most recent advances of the last 5 years to get a better understanding of its future direction of development. The appearance of innovative genetic tools and methodologies like the CRISPR/Cas9 gene-editing system eases the manipulation of gene expression systems and greatly improves the efficiency of exploring gene functions. The integration of novel pathways in microorganisms has raised more ideas of metabolic engineering for enhancing RP production. In addition, some new opportunities for the manufacture of proteins have been created by the application of novel mathematical models coupled with high-throughput screening to have a better overview of bottlenecks in the biosynthetic process.
During the last three decades, Pichia pastoris (Komagataella phaffii) was a common cell factory producing recombinant proteins (RP), which shows larger physiological advantages compared with other commonly used host cells (Zhu et al., 2019; Karbalaei et al., 2020). P. pastoris is a methylotrophic yeast (Zahrl et al., 2017), usually grown in dynamic culture systems, in which the cell culture environment presents a constant change and a lot of variable factors affect the productivity of products. It has some specific physiological properties, namely, the ability to grow rapidly at high cell densities (>150 g dry cell weight/liter) in simple media and secrete proteins at high yields under bioreactor conditions (Jahic et al., 2007; Parashar and Satyanarayana, 2016). In general, the advantages of protein production using P. pastoris system include higher folding efficiency, high cell density fermentation, strong expression system, genetic stability, and a mature secretion system of secreting proteins to the external environment (by Kex2 as signal peptidase) (Schwarzhans et al., 2017; Karbalaei et al., 2020). The phase of protein initiation was separated with the generation of a large number of cells (Tolner et al., 2006). Actually, P. pastoris enjoyed a more frequent application for the heterologous protein production relative to Saccharomyces cerevisiae according to one literature survey (Bill, 2014). Driven by the AOX1 promoter, the titer of RP production by P. pastoris is higher than 10 g/L, equivalent to 30% of total cell proteins (Duan et al., 2018). Despite the operation easiness and a well-determined process of the P. pastoris expression system, it is necessary to optimize the process for achieving the largest RP production.
Historically, P. pastoris was first isolated from the exudates of a chestnut tree in France and Guilliermond named it as Zygosaccharomyces pastoris (Zahrl et al., 2017). Then, it was classified by Yamada et al. to a novel genus, Komagataellaor Pichia (Naumov et al., 2018). More than 40 years ago, It was commercially applied for single cell protein (SCP) production by virtue of the high cell density fermentation process as animal feed additive with the utilization of methanol (Cregg et al., 1985; Cereghino et al., 2002). In the 1980s, P. pastoris was first developed as a heterologous protein expression system by using powerful and well-regulated AOX1 promoter (Cregg et al., 1985). One of the first large-scale industrial production processes was established in the 1990s, capable of producing enzyme hydroxynitrile lyase by >20 g of RP/liter of culture volume (Hasslacher et al., 1997). Unlike the Y11430 strain (wild type), GS115 is a common P. pastoris strain for protein expression, particularly in industry and medicine (Julien, 2006), which has 2 encoding genes of alcohol oxidase (AOX) enzymes, namely, AOX1 and AOX2. This yeast initially attracted attention because of its native character of utilizing methanol as the sole carbon source, which can be achieved by certain metabolic pathways that involved many special enzymes (Gellissen, 2000). By now, over 5,000 proteins have achieved successful cloning and expression with P. pastoris system (Liu et al., 2022). Nevertheless, P. pastoris cannot produce or secrete all proteins to such high titers. Under normal conditions, the protein production is obviously lower, especially with the expression of complex proteins which are hetero-oligomers, attached to membrane or easily suffer proteolytic degradation (Ahmad et al., 2014).
Since it was first discovered, various approaches have been applied to reshape P. pastoris based on their needs. Although P. pastoris has served as a standard platform for production of RPs for many decades, some limitations still exist that prevent it from being a trustable “cell factory” with high productivity and adaptability. Few powerful transcription tools are currently available in P. pastoris (Yang and Zhang, 2018), meanwhile, common used promoters of P. pastoris such as PAOX1 respond to methanol, which has the drawbacks of toxic, flammable, and explosive (Gasser et al., 2015; Vogl et al., 2016). The narrow regulation mode of methanol-inducible promoters severely limits its application scope. Efforts also have been devoted to rewiring this expression host and developing new genetic tools. The beginning to use of random mutagenesis and screening procedures (Liu et al., 1992), is followed by the establishment of selectable markers (Cereghino et al., 2001) and more complex genetic engineering tools such as the Cre-lox recombinase system (Li D. et al., 2017) CRISPR/Cas9 (Gao et al., 2022a) were established over the years. The study summarized the recent advances regarding system and synthetic biology which includes metabolic engineering in the understanding of the phenotypic characteristics exhibited by recombinant Pichia and genome editing techniques like the CRISPR-Cas system. Mathematical modeling and application of high-throughput screening were also introduced here for better control of the biosynthesis pathway.
Although DNA repair mechanisms in S. cerevisiae have been deeply understood, there are still many challenges in the exploration of the alternative yeast P. pastoris (Ahmad et al., 2014). Compared to S. cerevisiae, knock-out cassettes or knock-in expression fragment present a more strenuous and low-efficient targeted integration with homologous arms in P. pastoris (Fischer and Glieder, 2019). One reason is that P. pastoris uses non-homologous end-joining (NHEJ) as a main DNA repair mechanism when DNA double strand broke (Naatsaari et al., 2012). DNA repair by homologous recombination (HR) offers several advantages over NHEJ, including more efficient screening for positive clones and improved genomic stability (Dalvie et al., 2022). However, P. pastoris presents a low HR rate, and a majority of the introduced DNA cassettes are integrated at random sites in the genome by virtue of NHEJ (Wang et al., 2016). Hence, P. pastoris gives more favor to the NHEJ pathway than recombination events (HR) for repairing double-strand breaks (DSB) (Fischer and Glieder, 2019). Currently, this issue was addressed by a double knockout of the homogeneous genes dnl4 (coding for DNA ligase IV) of Ku 70, overall increased efficiency to a comparable level of other eukaryotic cells (Ito et al., 2018). Relative to S. cerevisiae, in which short overhangs are capable of targeting strongly specific integrations, in P. pastoris, long overhangs present a larger efficiency and foreign DNAs may undergo ectopic integration (Vogl et al., 2018a). In order to overcome this issue, HR machinery with multiplex genome integration was introduced from S. cerevisiae to P. pastoris strains. Core genes related to HR were overexpressed under strong constitutive promoters (i.e., PGAP and PTEF1) and inserted into the P. pastoris chromosome. Thus, HR efficiency was enhanced to realize effective one-, two-, and three-loci genome integration as high as 100, ∼98, and ∼81% using ∼40 bp homology arms (Gao et al., 2022b).
The appearance of novel genome editing tools eased the gene disruption and specifically improved the HR efficiency. One good example is CRISPR/Cas9. CRISPR/Cas9 is a powerful genetic editing tool, and has been developed into a mature laboratory technique capable of disrupting certain genes with high efficiency in prokaryotic and eukaryotic systems (Sander and Joung, 2014). It utilizes a nuclease, introducing a DNA double-strand break at the regions complementary to the gRNA sequence under the guidance of a short RNA (guide RNA: gRNA or single guide RNA: sgRNA). Recruiting the cellular repair mechanism helps to seal these breaks, which allows to introduce difference genome modifications, i.e., NHEJ in the case of no template and homology directed repair (HDR) with appropriate repair template (Jiang and Doudna, 2017). CRISPR/Cas9 can be reprogrammed, and different loci can be targeted by changing 20 bp of gRNA (Fischer and Glieder, 2019). In spite of its convenience, CRISPR/Cas9 was first adapted to P. pastoris in 2016 (Weninger et al., 2016). Expression of Cas9 usually relies on the use of regular genomic vectors, while nuclear localization as well as 5′–3′ trimming is needed for the single guide RNAs. The identification of this RNA polymerase-III (PolIII) mediated transcription in P. pastoris can be easily achieved by small RNA sequences, highly reducing the difficulty of multiplex cloning and expression (Dalvie et al., 2020). Later research further explored the potential of this technique (Weninger et al., 2018; Yang et al., 2020). The deletion of the Ku70 involved in NHEJ would remarkably improve the HR repair, achieving nearly 100% efficiency for site-directed of gene insertion (Weninger et al., 2018; Liu et al., 2019), but with the help of CRISPR/Cas9 system, HR efficiency could also be 100% for Mxr1 (methanol expression regulator 1) using the same sgRNA without deleting Ku70 due to the help of stable Cas9 expression through integrative expression (Yang et al., 2020). Furthermore, the HR efficiency was improved by even over 54 times in NHEJ deficient strain by the CRISPR-Cas9 system, which assisted in the incorporation of donor DNA to express heterologous gene (Cai et al., 2021). However, hard to knock out genes such as OCH1, where CRISPR/Cas9 showed only 50% mutation efficiency (Weninger et al., 2016). Normal approaches solve this problem such as increasing HR efficiency by addition of hydroxyurea during transformation to stop cell division at the S/G2 phase where the status of HR is rather more active than NHEJ (Lee et al., 2018). Compared to the Ku70 mutation strain, this method does not reduce transformation efficiency and it is more suitable for indel mutation (Ahmad et al., 2019; Gassler et al., 2019). Recently, another study revealed that Ku70 mutation negatively impacted the chromosome terminal stability that caused loss of colonies, but over-expression of RAD52 can drastically improve the efficiency of HR (Cai et al., 2021).
Besides the gene replacement and the targeted insertion, CRISPR/Cas9 can serve for creating indel mutations at targeted genomic loci by virtue of the NHEJ DNA repair mechanism under the condition of no homology target (donor DNA). HDR mediated by CRISPR/Cas9 allows DNA fragments to be effectively integrated into the genome, which is marker-free in selection, and is likely to replace, destroy or label a certain genomic locus (Singh et al., 2017). Recently, one synthetic biology toolkit based on CRISRP was established to ensure that multigene biosynthetic pathways could be integrated as well as assembled into the chromosome of P. pastoris with the selection of integration sites for the efficient and stable genome integration. Also, it characterized a panel of constitutive, methanol-inducible promoters, enabling the integration of one locus, two loci, and three loci (efficiency: ∼100, ∼93, and ∼75%, respectively) (Gao et al., 2022a). The most recent applications for CRISPR/Cas9 were shown below in Table 1.
Table 1. Genetic engineering tools for Pichia pastoris through HR,1 adapted from Cai et al. (2021).
In addition to functional studies regarding gene deletion, there are many kinds of knock-out strains that have been applied in the field of industrial biotechnology (Ito et al., 2018; Karbalaei et al., 2020; Aw et al., 2021; Guo et al., 2021). However, targeted gene knock-out of P. pastoris is still time-consuming (Ahmad et al., 2019). Typical genomic integration as well as gene replacement by HR expression cassettes for selecting markers was used extensively caused by a large number of recent patents in the field of genome editing based on CRISPR/Cas9 (Fischer and Glieder, 2019; Liao et al., 2021). Considering the shortage of selective markers, multiple genetic modifications of P. pastoris are limited (Liao et al., 2021). In the past, approaches are usually combined with zeocin-resistance marker recycling vectors that employ FRT/FLP recombinase or Cre/loxp recombination system (Li C. et al., 2017), which removes the marker sequences between two recognition sites (Kobalter et al., 2022). Recently, acetamidase (amdS) was found as a kind of effective selection marker for the integrative transformation in P. pastoris when requiring many times of gene deletion or insertion. It was allowed to easily recycle markers by counter-selection with fluoroacetamide (Piva et al., 2018). However, after the employment of the CRISPR/Cas9 system, it is also easy to achieve such recycling by CRISPR/Cas9 geneticin plasmids that contain gRNAs for targeting the Zeocin resistance gene. P. pastoris strains generated by this approach can be retransformed by Zeocin resistance markers that contain plasmids (Yang et al., 2020). Marker-free system was also possible by the use of multi loci gene integration approach mediated by CRISPR/Cas9 with efficient gRNA targets in P. pastoris. The use of high efficient sites with a 100 bp range of upstream promoter and downstream terminator enables the integration of multiple gene cassettes into genome simultaneously (Liu et al., 2019).
Plasmids can be usefully observed in bacteria, but only in a few yeast species. Generally, an episomal plasmid was not considered as an optimal choice to express the heterologous genes because of segregational instability in non-selective conditions, but it is an excellent choice regarding the expression of Cas9 (Pena et al., 2018). However, episomal plasmids for heterologous gene expression and the CRISPR/Cas9 system for genome editing have not been well developed in P. pastoris (Gu et al., 2019a). Based on previous attempts to apply the CRISPR/Cas9 system in P. pastoris, Yang et al. (2020) developed a CRISPR/Cas9 system that contained episomal sgRNA plasmid, which reached 100% genome editing efficiency in 14.7% of sgRNA. High multicopy gene editing and stable multigene editing efficiency were obtained which did not exhibit a sharp decrease due to the multi-sgRNA expression cassette. Besides HR efficiency, the vector carrying sgRNA expression cassette could be eliminated easily, benefiting the editing of other genes (Yang et al., 2020). It is necessary to identify two genetic elements, namely, the centromere and the autonomously replicating sequence (ARS), to engineer a steady episomal plasmid in a microorganism without carrying a plasmid (Piva et al., 2020; Schultz et al., 2021; Gatjen et al., 2022). Several recently identified ARS enable maintenance of episomal plasmids and efficient expression of recombinant proteins (Camattari et al., 2016; Schwarzhans et al., 2017; Nakamura et al., 2018; Gatjen et al., 2022). For instance, the episomal plasmids can be maintained in P. pastoris by a 452 bp DNA sequence (pan ARS) recovered from Kluyveromyces lactis (Camattari et al., 2016). The presence of ARS activity was found in a short fragment (111 bp) of chromosomal centromere 2 (PpARS2) (Nakamura et al., 2018). In addition, it was discovered that a piece of mitochondrial DNA (mitoARS) was able to help stabilize maintenance in the nucleosome (Schwarzhans et al., 2017). Gu et al. (2019a) thoroughly compared these ARSs, especially for creating and improving the genome editing system based on CRISPR/Cas9. Compared to a previously described method that used endogenous ARS (PARS1), CRISPR/Cas9 genome editing using panARS demonstrated an increased editing efficiency by over ten times (Weninger et al., 2016; Gu et al., 2019a). Similar findings were also discovered when establishing a Pichia surface display (PSD) system. Highest display levels were achieved with panARS-based expression vectors (Gatjen et al., 2022).
The engineering of microorganisms is now led by the reduction of manufacturing costs, mainly reached by higher titers. To efficiently enhance the protein production by P. pastoris, a fusion protein that includes the alpha mating factor secretion signal, researchers constructed human serum albumin (HSA) for promoting the protein secretion in P. pastoris (Sigar et al., 2017; Cao et al., 2018). The most recent recombinant proteins produced by P. pastoris were referred to below in Table 2.
The widely used promoters included AOX1, GAP, FLD1, ICL1, YPT1, NPS, which are usually classified into two groups: synthetic and natural promoters (Juturu and Wu, 2018). As natural promoters showed a limited capacity to tune expression levels and regulatory characteristics, many promoter engineering initiatives have been made for P. pastoris, especially for PAOX1 (Vogl, 2022). Besides PAOX1, some other methanol-inducible promoters have been characterized in P. pastoris. However, when considering the usage of methanol, it is highly inflammable and toxic. Using methanol for the high-level protein expression in the food and pharmaceutical industry is not favorable (Juturu and Wu, 2018). Some methanol free expression systems were designed for specific applications. On the other hand, synthetic promoter is becoming a trend for the improvement of protein production.
The promoter of the P. pastoris alcohol oxidase 1 gene (PAOX1) remained the most commonly used natural promoter for the RP expression vector construction in P. pastoris (Vogl and Glieder, 2013). It can be strictly inhibited by glucose or glycerol and triggered by methanol, which enables cells to grow in methanol as solely carbon source. In other words, it decouples cell growth and protein production phase. Relying on these mentioned advantages, it is of big value for the high-level protein expression and can replace the constitutive PGAP (promoter region of the glyceraldehyde-3-phosphate dehydrogenase) in certain cases (Gellissen, 2000).
The clarification of regulation mechanism promoted the development of PAOX1 engineering. Methanol expression regulator (Mxr1) essentially regulates the utilization pathway of methanol and is capable of activating a lot of genes in response to methanol (Wang et al., 2016). The overexpression of Mxr1 has the function of promoting AOX1 expression through inhibiting glycerol transporter 1 (GT1) expression (Zhan et al., 2017). Partial rewiring of PAOX1 transcriptional circuits can still maintain a basic output by overproducing a deregulated Mxr1 form in strains containing multiple PAOX1-based expression cassettes (Camara et al., 2019). Targeted editing of Mxr1 can be successfully achieved by CRISPR/Cas9 technology with plasmids containing sgRNA and the methanol expression regulator 1 homology arms (Hou et al., 2020). In other words, Mxr1 protein frame-shift mutations may reduce AOX1 protein levels as well as weaken the enzyme activity (Hou et al., 2020). In one study, the PAOX1 engineering by a mutation in the core promoter where wild type adjacent triplets were changed to cytosine or adenine triplets, a completely synthetic construction, demonstrating the strong tolerance of the core promoters to small mutations, which supported regulatory models regarding degenerate motifs, or redundant design in the future (Portela et al., 2018). Generally, modifications in PAOX1 core promoter or around upstream regulatory sequences (URS) by synthetic promoter demonstrate regulatory effects, and the engineering effort is mostly focused on 5′ untranslated regions (5′ UTR) (Vogl and Glieder, 2013). In a more recent study, the genetic modifications of PAOX1 were realized by using synthetic Adr1, Cat8, and Aca2 cis-acting DNA elements to replace specific cis-regulatory DNA elements for Mxr1, Cat8, and Aca1 binding, respectively. The combined promoter design with 3 Cat8, 3 palindromic Adr1, as well as Aca2 synthetic binding motifs can retrofit the strength of methanol to 1.97-fold that of PAOX1 (Ergün et al., 2020).
Besides PAOX1, some other methanol-inducible promoters have been characterized in P. pastoris. The expression level of the promoter of the dihydroxyacetone synthase 2 gene (PDAS2) was higher than that of PAOX1 (Vogl and Glieder, 2013), while the expression of PPEX8 and PAOX2 was much lower (Vogl, 2022). In order to identify alternative methanol inducible promoters, the transcriptional response of P. pastoris on microarray was observed under various carbon source conditions including glucose repression, derepression, and methanol-induction (Vogl et al., 2016). Fifteen different strengths of methanol-responsive promoters that participate the methanol utilization (MUT) pathway were identified. Clearly, the promoter of the CAT1 gene participating in the defenses against ROS, presented a strong methanol induction and the highest derepression level. PCAT1 induction can be achieved by using oleic acid at a similar level to methanol. Therefore, PCAT1 could be considered as an appropriate alternative derepressed, methanol-free promoter if its regulation mechanism was to be further elucidated (Vogl et al., 2016). Orthologous promoters from related yeast species were tested and even surpassed endogenous promoters in P. pastoris. Under methanol induction, the promoter of the HpMOX gene from Hansenula polymorpha (Hp) presented a similar expression levels as PAOX1 and HpFMD promoter surpassed PAOX1 by threefold (Vogl et al., 2020). These results indicated the potential of utilizing high-efficacy orthologous promoters from other eukaryotic hosts. Similar findings were revealed in the terminators, the terminator sequences from S. cerevisiae were confirmed to maintain function when transferred to P. pastoris (Vogl et al., 2020).
While methanol induction offers strong promotion and regulation of recombinant protein expression, it also contributes its own challenges. Due to the safety concern and strict process control for inducing methanol in large-scale fermentation, there have been some efforts in terms of the replacement of methanol as a single carbon source in P. pastoris (Wei et al., 2016). After the deletion of three transcription repressors and overexpression of one transcription factor MIT1, one methanol-free PAOX1 start-up strain was successfully constructed (Wang J. J. et al., 2017). Accordingly, a glucose-glycerol-shift induction model was built for replacing conventional glycerol/methanol induction in the wild-type strain. It is safe, economic, and environmentally friendly, but exhibits less protein expression ability. Only 77% GFP expression level was detected in glycerol relative to the wide type in methanol (Wang J. J. et al., 2017). Later, another study confirmed that any PAOX1-based strain can be converted to a methanol-free system with the simple overexpression of Mit1 or Mxr1 by derepression of PCAT1, and no addition of an alternative inducer was required, however, low feasibility and stability remain to be the main concern of this method (Vogl et al., 2018b). As one transcription regulating element in the MUT gene of P. pastoris, PDAS1, a strong methanol-inducible promoter driving dihydroxyacetone synthase expression, is also considered to be applied to RP production (Vogl et al., 2021). Constitutive expression of one single transcription factor KpTrm1 was enough to activate PDAS1 without the addition of methanol, the simplicity of PDAS1 regulation made it promising for the development of methanol-free protein expression system (Takagi et al., 2019). The protein yield remains to be a drawback in terms of these innovative methanol free system.
Despite the limitation of the selection of protein expression promoters in P. pastoris to PAOX1 or PGAP (Yang and Zhang, 2018), researchers have made efforts to find new synthetic promoters that are likely to replace conventional promoters. Engineered promoter variants (EPVs) exhibit an extremely stronger performance than natural promoters and allow to conduct “green-and-clean” production on a non-toxic carbon source in the first-choice utilization pathway of carbon source of yeast. One recent work to improve the RP expression with ethanol utilization pathway was done by Ergn et al. (2019). Transcription binding sites of alcohol dehydrogenase 2 promoter (PADH2) were engineered for designing the novel engineered promoter variants (NEPVs) (Ergn et al., 2019). The most popular method for the generation of EPVs is hybrid-promoter architectures. Using de novo synthetic sequence to replace native cis-acting DNA assists in achieving the architecture of the hybrid promoter (Ergün et al., 2020). The hybrid-architectured promoter design refers to collecting monodirectional double-promoter expression system (DPESs) with hybrid architecture composed of engineered promoter variants PADH2-Cat8-L2 and PmAOX1 and the natural promoter PGAP for enhancing and upregulating deregulated gene expressions in P. pastoris in media free of methanol (Demir and Calik, 2020). Biofunctional DPESs exhibited higher transcription and expression upregulation power relative to twin DPESs (two-copy expression systems). The most advanced technology of P. pastoris promoter engineering provides the comprehensive insights into the cis-acting DNA motifs and functions to ensure a rationally designed non-conventional promoter libraries that possess improved strength and different regulation mechanisms (Ergun and Calik, 2021). Apparently, synthetic promoter variants are becoming a trend for improvement of protein expression in the P. pastoris system (Machens et al., 2017). Lastly, since suitable promoters can mediate dynamic regulation of biosynthetic pathways and maintain cellular robustness, the appearance of the inexpensive synthetic promoters still remains to be explored for future applications (Vogl et al., 2016).
Metabolic engineering regarding P. pastoris initially featured protein glycosylation humanization (Irani et al., 2016; Pena et al., 2018). There are two main types of glycosylation, including N- and O-glycosylation. Researches show that N-glycosylation had a great effect on protein stability (Zou et al., 2013), activity (Zou et al., 2012), and specificity (Yang et al., 2015). However, the removal of N-glycosylation didn’t make great changes on the secondary structure of proteins but tertiary structure showed differences (Wang et al., 2018). Another recent study found that the degree of O-glycosylation was remarkably higher when the protein was induced by methanol compared with glucose (Radoman et al., 2021), but details of machinery required to be investigated later.
In view of the proven history of P. pastoris as an industrial cell factory for protein production, there has been an increasing interest in exploring its potential to produce primary and secondary metabolites. The RP production is a costly metabolic process, and during the process, many cellular machineries deviate from the targets of evolution regarding cell development and maintenance, precursors substances are expelled from the central carbon metabolism, and redox and energy cofactors are consumed, leading to low energy efficiency in the metabolism (Kafri et al., 2016). Thousands of biochemical reactions occur coordinately, hence cells are capable of obtaining energy as well as building blocks from the environment for maintaining their lives (Ergun et al., 2021). The metabolic constraints of recombinant protein production are shown in Figure 1.
The accuracy of metabolic flux analysis depends on the biomass composition, which is obviously different in cells grown on various carbon sources. The differences of biomass composition were found to remarkably affect the lipid flux distribution, the biosynthesis of secondary alcohol and the oxidation-reduction processes (Cankorur-Cetinkaya et al., 2017; Tomas-Gamisans et al., 2018). Considering the abundant proteinogenic amino acids, NMR can serve for evaluating how RP production affects the metabolomic profiles (Tredwell et al., 2017). On that account, correlation between these unfolded protein response (UPR) markers and specific metabolic signals was unveiled by transcript analysis of gene transcripts associated with UPR (HAC1, KAR2, PDI1) (Tredwell et al., 2017). This approach allows for the high-throughput screening of large numbers of clones in the future.
In spite of the achievements in recombinant DNA technology, the protein expression by engineered P. pastoris, faces many limitations, indicating that expression system exhibits unpredictable physiology (Prabhu et al., 2017). Above protein production restrictions can be solved via the metabolic engineering strategies through increasing the precursor supply as well as enhancing the cellular redox and energy efficiency. Protein folding and processing in the endoplasmic reticulum (ER) mainly restrict the protein production and secretion from P. pastoris. Recombinant gene overexpression is capable of destroying the inherent secretory machinery regarding P. pastoris, and accumulation of proteins folded in incorrect way occur inside the ER. For restoring the normal protein folding, an UPR pathway was triggered naturally by cells, causing expression upregulation on genes coding for chaperones as well as other folding-assisting proteins (Raschmanova et al., 2021). From another application perspective of metabolomics, metabolic biomarkers could indicate the cellular stress resulted from the UPR under when the expression was high (Tredwell et al., 2017). The effect of vacuolar protein sorting (VPS) system on protein secretion was investigated by generating VPS mutant strains. The impairment of VRS is considered as an effective means of enhancing recombinant protein secretion (Marsalek et al., 2017). The N-terminal portion of the pre-pro-α-factor is a common secretion signal, but it is capable of partially leading to aggregation, hence limiting the export from the ER (Barrero et al., 2018). A hybrid secretion signal that possessed the S. cerevisiae Ost1 signal sequence was engineered in P. Pastoris to facilitate co-translational translocation into the ER, and then the α-factor pro region (αPR). When Ost1 signal sequence was paired with the αPR, its secretion efficiency was much higher relative to the α-factor signal sequence (Barrero et al., 2018).
The manipulation of genes gives more possibilities for metabolic engineering through different carbon utilization pathways. As described before, by overexpressing only one transcription factor such as MIT1 in PAOX1-based expression strains, methanol-dependent strains could be transferred into a glucose/glycerol-regulated system (Vogl et al., 2018b). Furthermore, after adding 8 heterologous genes as well as deleting 3 native genes, it is possible to observe the transformation of the traditional P. pastoris methanol-assimilation pathway into a CO2-fixation pathway similar to the Calvin–Benson–Bassham cycle (Gassler et al., 2020), which provides us more insights in terms of innovating novel green and carbon-neutral process. Most recently, in order to regulate the target gene expression level of metabolic pathway, fast and reliable tools are applied to precisely manipulate the expression of target genes. One practical example was dCas9, which can be fused via RNA scaffolds to trans-activator domains and thus regulate the gene expression when targeted to the promoter region of a gene (Baumschabl et al., 2020).
The mathematic modeling regarding P. pastoris used for protein production mainly pays attention to 2 overall objectives: (1) predict nutrient and oxygen-related cell growth mainly for the design and control strategy of bioprocess; (2) enhance the understanding of metabolic responses under operational and nutritional conditions, thereby improving the productivity. The cell behavior was modeled to be a sub-system in a bioreactor, which may make the modeled system boundary confined to the bioreactor, cells, or both. Despite the different methods and mathematical tools used for fulfilling above objectives, a majority of them take into account the solutions of the linear equation (LE) systems or the ordinary differential equation (ODE) systems (Theron et al., 2018).
The earliest metabolic network of P. pastoris model came up with central carbon metabolism, using this model to calculate the 13C metabolic flux distributions (Maaheimo et al., 2001). The metabolic insights based on information of intracellular flux balances allow to build the metabolic model to be a useful tool before confirming the bioreactor process, for conducting partial prediction on the impact exerted by certain genetic modification (expression deletion or over expression) related parameters on the strain behavior (Kerkhoven et al., 2015; Cankorur-Cetinkaya et al., 2017). Recent years, major breakthroughs have been made in the systematic quantitative analysis of its physiology. Genome-scale metabolic models (GSMM) can be developed relying on the abundant information, hence, new approaches can serve for the engineering of host cells and bioprocess (Barrero et al., 2018). Incorporating heterologous protein production into a GSMM allows to simulate the influence of the overproduction as well as predict the improved productivity relying on the deletion or the overexpression of gene targets. Studies reported the ability of GSMM to predict the overexpression and deletion mutants that can improve the production of RP with high accuracy (Nocon et al., 2014). Taking advantages of available genomic information, thousands of metabolites and reactions are extended into the stoichiometric matrix, and GSMMs are configured (Famili and Palsson, 2003). Overall, the stoichiometric matrix imposed above constraints, and one or more flux values usually exhibited an association with the steady-state carbon source consumption rate of carbon source (Yasemi and Jolicoeur, 2021). With the help of GSMMs, the cell growth can be predicted with the supply of different nitrogen and carbon sources, the distribution of metabolic flux, as well as the way gene deletion or overexpression affects cell growth. Even the metabolic effects of some culture parameters on microbial cell factory performance like dilution rates and oxygen levels can be evaluated with the elucidation of their metabolic consequences (Torres et al., 2019).
Unlike the changes caused by biomass composition in the metabolic network, it was observed in a consensus genome-scale model that the variances of biomass composition didn’t make great differences to gene essentiality (Cankorur-Cetinkaya et al., 2017). The accuracy of mathematic models depends on the identifications of different reactions, meanwhile emphasizing the rankings of the altered candidate targets. The consistency and standardization of models decide whether they are appropriate for being reused (Cankorur-Cetinkaya et al., 2017). After the use of iterations, the dynamic block of the aforementioned states can be identified. The substrate uptake kinetics were determined by a kinetic block and the flux distributions were determined by a metabolic block (Sanchez et al., 2014). However, genome-scale modeling has the disadvantage of lots of simulations which was very complex in terms of the iterative method (Saitua et al., 2017). Oppositely, algorithmic optimization could serve for optimizing the protein productivity relying on the Dynamic flux balance analysis model (dFBA) (Sanchez et al., 2014). This model includes bilevel optimization, penalization schemes, and direct dynamic optimization, supplying the dynamic simulation of the extracellular bioreactor environment and intracellular fluxes in P. pastoris at the same time, and thus provide practical simplification and optimization (Emenike et al., 2018). The study investigated the RP erythropoietin, finding that the use of a small metabolic network avoided the iterative approach of GSMM (only 47 intracellular reactions relative to 1,361 reactions in the GSMM) (Emenike et al., 2018). Besides, considering the certain bioprocess restrictions as well as the strain features, the detailed relationship of specific growth rate with volumetric productivity can be predicted ahead of the practical process (Saitua et al., 2017). A larger amount of annotated genes, reactions, metabolites, as well as reaction locations can help improve the metabolic coverage and prediction accuracy of a P. pastoris GSMM, and assist in carefully adding the biosynthesis pathways regarding cofactors and vitamins for a better improvement (Ye et al., 2017).
In terms of bioreactor modeling, the elements required for construction include matrix, biomass, total protein, other medium components, and off-gas constituents. The probability distribution predicted by the model quantifying parameter distributions can be quantifiably consistent with the inter-run variability observed in the experimental data. Unlike GSMM, model prediction requires experimental data under different operating conditions. The improvement of chemical defined medium can be realized by the minimization of the number of components required while meeting cellular requirements (Hong et al., 2021).
As we still lack much knowledge about inner reactions and cellular responses. The accuracy of mathematic modeling cannot be ensured. The low efficiency of various modifications requires for rapid and functional perturbations. High throughput screening is an advanced and rapid laboratory technique that has been used in many microorganisms before (Sjostrom et al., 2014; Kenzom et al., 2015; Clare et al., 2019). The identification of desired results combined with the appliance of reverse engineering can enable the attainment of strains carrying specific mutations associated with the desired phenotype (Wang G. K. et al., 2017). High-throughput screening method can be simplified by carrying out the growth phase and induction phase together by inoculation of P. pastoris clones directly into methanol-containing medium (Kenzom et al., 2015). In addition, high-throughput screening help understand the impact of genetic engineering such as ectopic integration. It is possible to neglect the impact exerted by the integration locus in the promise of comparing enough transformed strain number (Vogl et al., 2018a). With the generation of 168 synthetic bidirectional promoters (BDPs) in P. pastoris and the leverage of naturally occurring BDPs as a parts repository, different expression profiles and ratios were fast screened for optimizing the gene co-expression. This library strategy depends on the highly conserved BDP architecture of histones and can be extended to other eukaryotes (Vogl et al., 2021).
Flow cytometry is considered as a powerful, high throughout technology that provides rapid muti-parametric analysis of single cells in solution (McKinnon, 2018). It was primarily used for measuring the fluorescence intensity produced by fluorescent-labeled antibodies which served for detecting proteins or ligands that bind to various molecules related to cells. Specially, it was used for quantifying the physiological state regarding P. pastoris in heterologous protein production process in cultures with high cell density (Zepeda et al., 2018). Thousands of individual cells were measured simultaneously by the use of fluorescent probes and several parameters (Cossarizza et al., 2019). By using flow cytometry, not only were sedimentation and possible agglomerations of biased cells minimized, but a false-positive detection of loosely agglomerated cells could also be minimized due to the in-flow velocity of the cell suspension and the force exerted on the cells (Pekarsky et al., 2018). As an at-line, single-cell and non-invasive method, flow cytometry could be applied for evaluating the unfolded protein response (UPR) and cell viability. The observed upregulation of UPR didn’t show any positive correlation with impaired viability (Raschmanova et al., 2019). Nowadays, with the development of deep convolutional neural network, a system for image-guided cell sorting and classification with the high throughput of flow cytometer was able to be established. The characteristics could be automatically extracted after being processed directly on the one-dimensional time-series waveforms (Gu et al., 2019b).
The establishment of high cell density fed-batch biomanufacturing faces difficulties in the screening phase and the earlier bioprocess development phase (mainly using shake flasks and microplates) affected by the costly and time-consuming process together with the weak experimental complexity (Totaro et al., 2021). Fortunately, microfluidics with high throughput, strong reproducibility, big parallelization, high operability, and low cost, serve as a powerful platform for analyzing SCPs (Chen et al., 2019). The high-throughput (100,000 strains/hour) P. pastoris screening system that combines the single-cell droplet microfluidic control is capable of screening a library of million strains within 10 h by consuming a small amount of (100 μL) fluorescent reagent. Compared with traditional microplate screening, the reagent cost was reduced by millions of times (Lu et al., 2019). With atmospheric and room temperature plasma (ARTP) mutagenesis and droplet-based microfluidic high-throughput screening, it is possible to explore the potential of protein production by employing iterative rounds of genome-wide diversity generation (Kenzom et al., 2015; Ma et al., 2019; Yuan et al., 2022). For example, compared to the beginning strain, the best mutant strain showed a twofold increase in cellulase activity in one research (Yuan et al., 2022). One high-throughput platform was established, which could process any protein with a His6-tag. To selectively preserve any protein of interest, vast genetic libraries of strains were encased in biocompatible gel beads using droplet microfluidics. Then, flow cytometry discovered strains with higher production titers after the fluorescent labeling of bead-retained products (Napiorkowska et al., 2021). Overall, the application of high throughput screening enabled massively parallel characterization of microorganisms and accelerated the costly and time-consuming process of strain engineering.
The development of some modern and powerful genome editing techniques engineered the expression system in a very efficient way and highly improved the HR efficiency. It is allowed to select a proper strain that accords with expectations from modeling or one that serves as a high-promise candidate relative to others. Breakthrough of these technologies makes the characterization of host strains much easier than before. The integration of promoter engineering promoted the expression level to be more controllable. Due to the safety concern raised by methanol, some novel promoters have been created for developing a methanol-independent expression system. Theoretically, it is allowed to improve the RP production in P. pastoris in methanol-free media through (a) the activation of existing pathway (s), or the generation of new regulatory circuits relying on engineered AOX1/2 promoters, or (b) the design of synthetic new pathways with different transcription factors.
More deeply understanding the metabolic responses can assist in directing the attempts for strain customization via the genetic engineering for pathway modifications. Before constructing the production process, the investigation of the metabolic engineering of the organism can help to understand the response of cells to the envisioned process. In particular, a genome-scale model can manage all the required information for the improvement of protein secretion in the design of cell factories. As the complete genome sequences are available and systems biology makes advances, genetic manipulation more frequently serves for modifying the certain cellular biochemical reactions to enhance the protein production. Despite this, the newest experimental data and more annotations are needed for a continuous upgrade and validation on the predictive accuracy exhibited by the well-recognized model previously. With the help of mathematical modeling, it would be easier to have a comprehensive understanding of the whole process and optimize key factors which influence the final yield and target productivity of proteins. There is no doubt that P. pastoris has better performance over Escherichia coli, S. cerevisiae, baculovirus/insect cells, SFV or adenovirus/mammalian host cells specific to soluble, secretable protein production. Relative to other prokaryotic and eukaryotic expression system, it boosts the advantages of (Prielhofer et al., 2013; Gupta and Shukla, 2017):
• Grow easily and fast to a high cell density in the defined medium;
• High level of productivity in an almost protein free medium;
• Easy to conduct genetic manipulation of yeast expression vectors with good characteristics;
• Easy of diverse post-translational modifications;
• Allow to purify secreted proteins from growth medium with no need to collect the yeast cells themselves;
• Free of endotoxins and virus.
Although P. pastoris was thought to be a good platform strain for RP expression, this type of system features proteolytic degradation and product truncation, which results in lower yields and less biological activity (Sinha et al., 2005). Fundamental knowledge of P. pastoris is relatively limited compared to that for S. cerevisiae, and there are fewer available molecular tools used for P. pastoris like terminators, promoters, or knockout strains (Dikicioglu et al., 2014). The expression level for most proteins still remains to be improved for the requirement of industrial use. Although RPs from yeast are likely to be less useful in biopharmaceutical applications due to the emergence of hyper-mannosylation, it was reported that the glycoengineered P. pastoris strains could produce RPs with highly uniformed human N-linked glycans (Potgieter et al., 2009). However, It is expected that the capacity of protein production by P. pastoris can be highly improved later and show more advantages over other yeasts.
YP: data collection, investigation, writing – original draft, and review and editing. JY: review and editing (supporting) and proofreading. JW and LY: supervision. HF: main supervision, revising, reviewing, and proofreading. All authors contributed to the article and approved the submitted version.
The financial supports from the National Natural Science Foundation of China (NSFC) (No. 22007079) and the Start-up Fund for Talent Introduction from ZJU-Hangzhou Global Scientific and Technological Innovation Center (K02013020) were acknowledged.
The authors declare that the research was conducted in the absence of any commercial or financial relationships that could be construed as a potential conflict of interest.
All claims expressed in this article are solely those of the authors and do not necessarily represent those of their affiliated organizations, or those of the publisher, the editors and the reviewers. Any product that may be evaluated in this article, or claim that may be made by its manufacturer, is not guaranteed or endorsed by the publisher.
Ahmad, M., Hirz, M., Pichler, H., and Schwab, H. (2014). Protein expression in Pichia pastoris: Recent achievements and perspectives for heterologous protein production. Appl. Microbiol. Biotechnol. 98, 5301–5317. doi: 10.1007/s00253-014-5732-5
Ahmad, M., Winkler, C. M., Kolmbauer, M., Pichler, H., Schwab, H., and Emmerstorfer-Augustin, A. (2019). Pichia pastoris protease-deficient and auxotrophic strains generated by a novel, user-friendly vector toolbox for gene deletion. Yeast 36, 557–570. doi: 10.1002/yea.3426
Allonso, D., Pereira, I. B., Alves, A. M. B., Kurtenbach, E., and Mohana-Borges, R. (2019). Expression of soluble, glycosylated and correctly folded dengue virus NS1 protein in Pichia pastoris. Protein Expr. Purif. 162, 9–17. doi: 10.1016/j.pep.2019.05.003
Aw, R., De Wachter, C., Laukens, B., De Rycke, R., De Bruyne, M., Bell, D., et al. (2021). Knockout of RSN1, TVP18 or CSC1-2 causes perturbation of Golgi cisternae in Pichia pastoris. Traffic 22, 48–63. doi: 10.1111/tra.12773
Barrero, J. J., Casler, J. C., Valero, F., Ferrer, P., and Glick, B. S. (2018). An improved secretion signal enhances the secretion of model proteins from Pichia pastoris. Microb. Cell Factories 17:13. doi: 10.1186/s12934-018-1009-5
Baumschabl, M., Prielhofer, R., Mattanovich, D., and Steiger, M. G. (2020). Fine-Tuning of Transcription in Pichia pastoris Using dCas9 and RNA Scaffolds. Acs Synth. Biol. 9, 3202–3209. doi: 10.1021/acssynbio.0c00214
Bill, R. M. (2014). Playing catch-up with Escherichia coli: Using yeast to increase success rates in recombinant protein production experiments. Front. Microbiol. 5:85. doi: 10.3389/fmicb.2014.00085
Cai, P., Duan, X. P., Wu, X. Y., Gao, L. H., Ye, M., and Zhou, Y. J. (2021). Recombination machinery engineering facilitates metabolic engineering of the industrial yeast Pichia pastoris. Nucleic Acids Res. 49, 7791–7805. doi: 10.1093/nar/gkab535
Cámara, E., Monforte, S., Albiol, J., and Ferrer, P. (2019). Deregulation of methanol metabolism reverts transcriptional limitations of recombinant Pichia pastoris (Komagataella spp) with multiple expression cassettes under control of the AOX1 promoter. Biotechnol. Bioeng. 116, 1710–1720. doi: 10.1002/bit.26947
Camattari, A., Goh, A., Yip, L. Y., Tan, A. H. M., Ng, S. W., Tran, A., et al. (2016). Characterization of a panARS-based episomal vector in the methylotrophic yeast Pichia pastoris for recombinant protein production and synthetic biology applications. Microb. Cell Fact. 15:139. doi: 10.1186/s12934-016-0540-5
Cankorur-Cetinkaya, A., Dikicioglu, D., and Oliver, S. G. (2017). Metabolic modeling to identify engineering targets for Komagataella phaffii: The effect of biomass composition on gene target identification. Biotechnol. Bioeng. 114, 2605–2615. doi: 10.1002/bit.26380
Cao, J. C., de la Fuente-Nunez, C., Ou, R. W., Torres, M. D., Pande, S. G., Sinskey, A. J., et al. (2018). Yeast-based synthetic biology platform for antimicrobial peptide production. Acs Synth. Biol. 7, 896–902. doi: 10.1021/acssynbio.7b00396
Cereghino, G. P. L., Cereghino, J. L., Ilgen, C., and Cregg, J. M. (2002). Production of recombinant proteins in fermenter cultures of the yeast Pichia pastoris. Curr. Opin. Biotechnol. 13, 329–332. doi: 10.1016/S0958-1669(02)00330-0
Cereghino, G. P. L., Cereghino, J. L., Sunga, A. J., Johnson, M. A., Lim, M., Gleeson, M. A. G., et al. (2001). New selectable marker/auxotrophic host strain combinations for molecular genetic manipulation of Pichia pastoris. Gene 263, 159–169. doi: 10.1016/S0378-1119(00)00576-X
Chen, P., Chen, D. J., Li, S. J., Ou, X. W., and Liu, B. F. (2019). Microfluidics towards single cell resolution protein analysis. Trac Trends Anal. Chem. 117, 2–12. doi: 10.1016/j.trac.2019.06.022
Clare, R. H., Bardelle, C., Harper, P., Hong, W. D., Borjesson, U., Johnston, K. L., et al. (2019). Industrial scale high-throughput screening delivers multiple fast acting macrofilaricides. Nat. Commun. 10:11 doi: 10.1038/s41467-018-07826-2
Cossarizza, A., Chang, H. D., Radbruch, A., Acs, A., Adam, D., Adam-Klages, S., et al. (2019). Guidelines for the use of flow cytometry and cell sorting in immunological studies (second edition). Eur. J. Immunol. 49, 1457–1973. doi: 10.1002/eji.201970107
Cregg, J. M., Barringer, K. J., Hessler, A., and Madden, Y. K. R. (1985). Pichia-pastoris as a host system for transformations. Mol. Cell. Biol. 5, 3376–3385. doi: 10.1128/mcb.5.12.3376-3385.1985
Dalvie, N. C., Leal, J., Whittaker, C. A., Yang, Y. C., Brady, J. R., Love, K. R., et al. (2020). Host-Informed Expression of CRISPR Guide RNA for Genomic Engineering in Komagataella phaffii. Acs Synth. Biol. 9, 26–35. doi: 10.1021/acssynbio.9b00372
Dalvie, N. C., Lorgeree, T., Biedermann, A. M., Love, K. R., and Love, J. C. (2022). Simplified Gene Knockout by CRISPR-Cas9-Induced Homologous Recombination. Acs Synth. Biol. 11, 497–501. doi: 10.1021/acssynbio.1c00194
De-Mei, M., Jing-Fang, Z., Xiao, L., Hong-Xia, D., Ya-Jun, G., Xiao-Fang, G., et al. (2017). Recombinant expression, purification and antimicrobial activity of a novel antimicrobial peptide PaDef in Pichia pastoris. Protein Expr. Purif. 130, 90–99. doi: 10.1016/j.pep.2016.10.003
Demir, I., and Calik, P. (2020). Hybrid-architectured double-promoter expression systems enhance and upregulate-deregulated gene expressions in Pichia pastorisin methanol-free media. Appl. Microbiol. Biotechnol. 104, 8381–8397. doi: 10.1007/s00253-020-10796-5
Dewi, K. S., Chairunnisa, S., Swasthikawati, S., Yuliawati Agustiyanti, D. F., Mustopa, A. Z., et al. (2022). Production of codon-optimized Human papillomavirus type 52 L1 virus-like particles in Pichia pastoris BG10 expression system. Prep. Biochem. Biotechnol. [Epub ahead of print]. doi: 10.1080/10826068.2022.2048262
Dikicioglu, D., Wood, V., Rutherford, K. M., McDowall, M. D., and Oliver, S. G. (2014). Improving functional annotation for industrial microbes: A case study with Pichia pastoris. Trends Biotechnol. 32, 397–400. doi: 10.1016/j.tibtech.2014.05.003
Duan, X. P., Gao, J. Q., and Zhou, Y. J. J. (2018). Advances in engineering methylotrophic yeast for biosynthesis of valuable chemicals from methanol. Chin. Chem. Lett. 29, 681–686. doi: 10.1016/j.cclet.2017.11.015
Eissazadeh, S., Moeini, H., Dezfouli, M. G., Heidary, S., Nelofer, R., and Abdullah, M. P. (2017). Production of recombinant human epidermal growth factor in Pichia pastoris. Braz. J. Microbiol. 48, 286–293. doi: 10.1016/j.bjm.2016.10.017
Emenike, V. N., Schenkendorf, R., and Krewer, U. (2018). Model-based optimization of biopharmaceutical manufacturing in Pichia pastoris based on dynamic flux balance analysis. Comput. Chem. Eng. 118, 1–13. doi: 10.1016/j.compchemeng.2018.07.013
Ergn, B. G., Gasser, B., Mattanovich, D., and Alik, P. (2019). Engineering of alcohol dehydrogenase 2 hybrid-promoter architectures in Pichia pastoris to enhance recombinant protein expression on ethanol. Biotechnol. Bioeng. 116, 2674–2686. doi: 10.1002/bit.27095
Ergun, B. G., Berrios, J., Binay, B., and Fickers, P. (2021). Recombinant protein production in Pichia pastoris: From transcriptionally redesigned strains to bioprocess optimization and metabolic modelling. FEMS Yeast Res. 21:foab057. doi: 10.1093/femsyr/foab057
Ergun, B. G., and Calik, P. (2021). “Hybrid-architectured promoter design to deregulate expression in yeast,” in Recombinant protein expression: Eukaryotic hosts, Vol. 660, eds W. B. Odell and Z. Kelman (London: Academic Press Ltd-Elsevier Science Ltd), 105–125. doi: 10.1016/bs.mie.2021.05.014
Ergün, B. G., Demir, R., ÖZdamar, T. H., Gasser, B., Mattanovich, D., and Çalık, P. (2020). Engineered deregulation of expression in yeast with designed hybrid CC romoter architectures in coordination with discovered master regulator transcription factor. Adv. Biosyst. 4:1900172. doi: 10.1002/adbi.201900172
Famili, I., and Palsson, B. O. (2003). Systemic metabolic reactions are obtained by singular value decomposition of genome-scale stoichiometric matrices. J. Theor. Biol. 224, 87–96. doi: 10.1016/S0022-5193(03)00146-2
Faridi, S., and Satyanarayana, T. (2018). Thermo-alkali-stable alpha-carbonic anhydrase of Bacillus halodurans: Heterologous expression in Pichia pastoris and applicability in carbon sequestration. Environ. Sci. Pollut. Res. 25, 6838–6849. doi: 10.1007/s11356-017-0820-6
Fischer, J. E., and Glieder, A. (2019). Current advances in engineering tools for Pichia pastoris. Curr. Opin. Biotechnol. 59, 175–181. doi: 10.1016/j.copbio.2019.06.002
Gao, J. C., Xu, J. H., Zuo, Y. M., Ye, C. F., Jiang, L. J., Feng, L. J., et al. (2022a). Synthetic biology toolkit for marker-less integration of multigene pathways into Pichia pastoris via CRISPR/Cas9. Acs Synth. Biol. 11, 623–633. doi: 10.1021/acssynbio.1c00307
Gao, J. C., Ye, C. F., Cheng, J. T., Jiang, L. H., Yuan, X. H., and Lian, J. Z. (2022b). Enhancing homologous recombination efficiency in Pichia pastoris for multiplex genome integration using short homology arms. Acs Synth. Biol. 11, 547–553. doi: 10.1021/acssynbio.1c00366
Gasser, B., Steiger, M. G., and Mattanovich, D. (2015). Methanol regulated yeast promoters: Production vehicles and toolbox for synthetic biology. Microb. Cell Fact. 14:3. doi: 10.1186/s12934-015-0387-1
Gassler, T., Heistinger, L., Mattanovich, D., Gasser, B., and Prielhofer, R. (2019). “CRISPR/Cas9-mediated homology-directed genome editing in Pichia pastoris,” in Recombinant protein production in yeast, eds B. Gasser and D. Mattanovich (Totowa, NJ: Humana Press Inc), 211–225. doi: 10.1007/978-1-4939-9024-5_9
Gassler, T., Sauer, M., Gasser, B., Egermeier, M., Troyer, C., Causon, T., et al. (2020). The industrial yeast Pichia pastoris is converted from a heterotroph into an autotroph capable of growth on CO2. Nat. Biotechnol. 38, 210–216. doi: 10.1038/s41587-019-0363-0
Gatjen, D., Tomszak, F., Dettmann, J. C., Droste, M., Nolle, V., and Wieczorek, M. (2022). Design of a novel switchable antibody display system in Pichia pastoris. Appl. Microbiol. Biotechnol. 106, 6209–6224. doi: 10.1007/s00253-022-12108-5
Gellissen, G. (2000). Heterologous protein production in methylotrophic yeasts. Appl. Microbiol. Biotechnol. 54, 741–750. doi: 10.1007/s002530000464
Gu, Y., Gao, J. C., Cao, M. F., Dong, C., Lian, J. Z., Huang, L., et al. (2019a). Construction of a series of episomal plasmids and their application in the development of an efficient CRISPR/Cas9 system in Pichia pastoris. World J. Microbiol. Biotechnol. 35:79. doi: 10.1007/s11274-019-2654-5
Gu, Y., Zhang, A. C., Han, Y. Y., Li, J., Chen, C., and Lo, Y. H. (2019b). Machine learning based real-time image-guided cell sorting and classification. Cytometry A 95A, 499–509. doi: 10.1002/cyto.a.23764
Guo, F., Dai, Z. X., Peng, W. F., Zhang, S. J., Zhou, J., Ma, J. F., et al. (2021). Metabolic engineering ofPichia pastorisfor malic acid production from methanol. Biotechnol. Bioeng. 118, 357–371. doi: 10.1002/bit.27575
Gupta, S. K., and Shukla, P. (2017). Sophisticated cloning, fermentation, and purification technologies for an enhanced therapeutic protein production: A review. Front. Pharmacol. 8:17. doi: 10.3389/fphar.2017.00419
Hasslacher, M., Schall, M., Hayn, M., Bona, R., Rumbold, K., Lückl, J., et al. (1997). High-level intracellular expression of hydroxynitrile lyase from the tropical rubber TreeHevea brasiliensisin. Microb. Hosts 11, 61–71. doi: 10.1006/prep.1997.0765
Hong, M. S., Velez-Suberbie, M. L., Maloney, A. J., Biedermann, A., Love, K. R., Love, J. C., et al. (2021). Macroscopic modeling of bioreactors for recombinant protein producing Pichia pastoris in defined medium. Biotechnol. Bioeng. 118, 1199–1212. doi: 10.1002/bit.27643
Hou, C. L., Yang, Y. K., Xing, Y., Zhan, C. J., Liu, G. Q., Liu, X. X., et al. (2020). Targeted editing of transcriptional activator MXR1 on the Pichia pastoris genome using CRISPR/Cas9 technology. Yeast 37, 305–312. doi: 10.1002/yea.3462
Irani, Z. A., Kerkhoven, E. J., Shojaosadati, S. A., and Nielsen, J. (2016). Genome-scale metabolic model of Pichia pastoris with native and humanized glycosylation of recombinant proteins. Biotechnol. Bioeng. 113, 961–969. doi: 10.1002/bit.25863
Ito, Y., Watanabe, T., Aikawa, S., Nishi, T., Nishiyama, T., Nakamura, Y., et al. (2018). Deletion of DNA ligase IV homolog confers higher gene targeting efficiency on homologous recombination in Komagataella phaffii. FEMS Yeast Res. 18:foy074. doi: 10.1093/femsyr/foy074
Jahic, M., Veide, A., Charoenrat, T., Teeri, T., and Enfors, S. O. (2007). Process technology for production and recovery of heterologous proteins with pichia pastoris (vol 22, pg 1472, 2006). Biotechnol. Progr. 23, 516–516. doi: 10.1021/bp078000r
Jiang, F., and Doudna, J. A. (2017). CRISPR–Cas9 Structures and Mechanisms. Annu. Rev. Biophys. 46, 505–529. doi: 10.1146/annurev-biophys-062215-010822
Julien, C. (2006). Production of humanlike recombinant proteins in Pichia pastoris. From expression vectors to fermentation strategy. Bio. Int. 4, 22–31.
Juturu, V., and Wu, J. C. (2018). Heterologous protein expression in Pichia pastoris: Latest research progress and applications. Chembiochem 19, 7–21. doi: 10.1002/cbic.201700460
Kafri, M., Metzl-Raz, E., Jona, G., and Barkai, N. (2016). The cost of protein production. Cell Rep. 14, 22–31. doi: 10.1016/j.celrep.2015.12.015
Karbalaei, M., Rezaee, S. A., and Farsiani, H. (2020). Pichia pastoris: A highly successful expression system for optimal synthesis of heterologous proteins. J. Cell. Physiol. 235, 5867–5881. doi: 10.1002/jcp.29583
Kenzom, T., Srivastava, P., and Mishra, S. (2015). Simplified high-throughput screening of AOX1-expressed laccase enzyme in Pichia pastoris. Anal. Biochem. 489, 59–61. doi: 10.1016/j.ab.2015.08.016
Kerkhoven, E. J., Lahtvee, P. J., and Nielsen, J. (2015). Applications of computational modeling in metabolic engineering of yeast. FEMS Yeast Res. 15, 1–13. doi: 10.1111/1567-1364.12199
Kobalter, S., Radkohl, A., Schwab, H., Emmerstorfer-Augustin, A., and Pichler, H. (2022). Plasmid-Based Gene Knockout Strategy with Subsequent Marker Recycling in Pichia pastoris. Methods Mol. Biol. 2513, 135–151. doi: 10.1007/978-1-0716-2399-2_9
Lee, H., Han, C., Lee, H. W., Park, G., Jeon, W., Ahn, J., et al. (2018). Development of a promising microbial platform for the production of dicarboxylic acids from biorenewable resources. Biotechnol. Biofuels 11:310. doi: 10.1186/s13068-018-1310-x
Lee, J. Y., Chen, H., Liu, A. L., Alba, B. M., and Lim, A. C. (2017). Auto-induction of Pichia pastoris AOX1 promoter for membrane protein expression. Protein Expr. Purif. 137, 7–12. doi: 10.1016/j.pep.2017.06.006
Li, C., Lin, Y., Zheng, X. Y., Yuan, Q. Y., Pang, N., Liao, X. H., et al. (2017). Recycling of a selectable marker with a self-excisable plasmid in Pichia pastoris. Scientific Reports 7, 10. doi: 10.1038/s41598-017-11494-5
Li, D., Zhang, B., Li, S. T., Zhou, J., Cao, H., Huang, Y., et al. (2017). A novel vector for construction of markerless multicopy overexpression transformants in Pichia pastoris. Front. Microbiol. 8:1698. doi: 10.3389/fmicb.2017.01698
Lia, H. M., Ali, Z., Liu, X. L., Jiang, L., Tang, Y. J., and Dai, J. G. (2019). Expression of recombinant tachyplesin I in Pichia pastoris. Protein Expr. Purif. 157, 50–56. doi: 10.1016/j.pep.2019.01.012
Liao, X. H., Li, L., Jameel, A., Xing, X. H., and Zhang, C. (2021). A versatile toolbox for CRISPR-based genome engineering in Pichia pastoris. Appl. Microbiol. Biotechnol. 105, 9211–9218. doi: 10.1007/s00253-021-11688-y
Liu, H., Tan, X., Veenhuis, M., and Cregg, J. M. (1992). An efficient screen for peroxisome-deficient mutants of Pichia-pastoris. Mol. Biol. Cell 3, A182–A182. doi: 10.1128/jb.174.15.4943-4951.1992
Liu, Q., Shi, X. N., Song, L. L., Liu, H. F., Zhou, X. S., Wang, Q. Y., et al. (2019). CRISPR-Cas9-mediated genomic multiloci integration in Pichia pastoris. Microb. Cell Fact. 18:144. doi: 10.1186/s12934-019-1194-x
Liu, Q., Song, L. L., Peng, Q. Q., Zhu, Q. Y., Shi, X. N., Xu, M. Q., et al. (2022). A programmable high-expression yeast platform responsive to user-defined signals. Sci. Adv. 8:eabl5166. doi: 10.1126/sciadv.abl5166
Lu, T., Tu, R., Yuan, H., Liu, H., and Wang, Q. (2019). Development and application of a droplet-based microfluidic high-throughput screening of Pichia pastoris. Chin. J. Biotechnol. 35, 1317–1325.
Ma, C. X., Tan, Z. L., Lin, Y., Han, S. Y., Xing, X. H., and Zhang, C. (2019). Gel microdroplet-based high-throughput screening for directed evolution of xylanase-producing Pichia pastoris. J. Biosci. Bioeng. 128, 662–668. doi: 10.1016/j.jbiosc.2019.05.008
Maaheimo, H., Fiaux, J., Cakar, Z. P., Bailey, J. E., Sauer, U., and Szyperski, T. (2001). Central carbon metabolism of Saccharomyces cerevisiae explored by biosynthetic fractional13C labeling of common amino acids. Eur. J. Biochem. 268, 2464–2479. doi: 10.1046/j.1432-1327.2001.02126.x
Machens, F., Balazadeh, S., Mueller-Roeber, B., and Messerschmidt, K. (2017). Synthetic promoters and transcription factors for heterologous protein expression in Saccharomyces cerevisiae. Front. Bioeng. Biotechnol. 5:63. doi: 10.3389/fbioe.2017.00063
Marsalek, L., Gruber, C., Altmann, F., Aleschko, M., Mattanovich, D., Gasser, B., et al. (2017). Disruption of genes involved in CORVET complex leads to enhanced secretion of heterologous carboxylesterase only in protease deficient Pichia pastoris. Biotechnol. J. 12:1600584. doi: 10.1002/biot.201600584
McKinnon, K. M. (2018). Flow cytometry: An overview. Curr. Protoc. Immunol. 120, 5.1.1–5.1.11. doi: 10.1002/cpim.40
Meng, D. M., Li, W. J., Shi, L. Y., Lv, Y. J., Sun, X. Q., Hu, J. C., et al. (2019). Expression, purification and characterization of a recombinant antimicrobial peptide Hispidalin in Pichia pastoris. Protein Expr. Purif. 160, 19–27. doi: 10.1016/j.pep.2019.03.007
Naatsaari, L., Mistlberger, B., Ruth, C., Hajek, T., Hartner, F. S., and Glieder, A. (2012). Deletion of the Pichia pastoris KU70 homologue facilitates platform strain generation for gene expression and synthetic biology. PLoS One 7:e39720. doi: 10.1371/journal.pone.0039720
Nakamura, Y., Nishi, T., Noguchi, R., Ito, Y., Watanabe, T., Nishiyama, T., et al. (2018). A stable, autonomously replicating plasmid vector containing Pichia pastoris Centromeric DNA. Appl. Environ. Microbiol. 84:e02882-17. doi: 10.1128/AEM.02882-17
Napiorkowska, M., Pestalozzi, L., Panke, S., Held, M., and Schmitt, S. (2021). High-throughput optimization of recombinant protein production in microfluidic gel beads. Small 17:e2005523. doi: 10.1002/smll.202005523
Naumov, G. I., Naumova, E. S., and Boundy-Mills, K. L. (2018). Description of Komagataella mondaviorum sp. nov., a new sibling species of Komagataella (Pichia) pastoris. Antonie Van Leeuwenhoek 111, 1197–1207. doi: 10.1007/s10482-018-1028-6
Nocon, J., Steiger, M. G., Pfeffer, M., Sohn, S. B., Kim, T. Y., Maurer, M., et al. (2014). Model based engineering of Pichia pastoris central metabolism enhances recombinant protein production. Metab. Eng. 24, 129–138. doi: 10.1016/j.ymben.2014.05.011
Parashar, D., and Satyanarayana, T. (2016). Enhancing the production of recombinant acidic α-amylase and phytase in Pichia pastoris under dual promoters [constitutive (GAP) and inducible (AOX)] in mixed fed batch high cell density cultivation. Process Biochem. 51, 1315–1322. doi: 10.1016/j.procbio.2016.07.027
Pekarsky, A., Veiter, L., Rajamanickam, V., Herwig, C., Grunwald-Gruber, C., Altmann, F., et al. (2018). Production of a recombinant peroxidase in different glyco-engineered Pichia pastoris strains: A morphological and physiological comparison. Microb. Cell Fact. 17:183. doi: 10.1186/s12934-018-1032-6
Pena, D. A., Gasser, B., Zanghellini, J., Steiger, M. G., and Mattanovich, D. (2018). Metabolic engineering of Pichia pastoris. Metab. Eng. 50, 2–15. doi: 10.1016/j.ymben.2018.04.017
Piva, L. C., De Marco, J. L., de Moraes, L. M. P., Reis, V. C. B., and Torres, F. A. G. (2018). Acetamidase as a dominant recyclable marker for Komagataella phaffii strain engineering. Appl. Microbiol. Biotechnol. 102, 2753–2761. doi: 10.1007/s00253-018-8824-9
Piva, L. C., De Marco, J. L., de Moraes, L. M. P., Reis, V. C. B., and Torres, F. A. G. (2020). Construction and characterization of centromeric plasmids forKomagataella phaffiiusing a color-based plasmid stability assay. PLoS One 15:e0235532. doi: 10.1371/journal.pone.0235532
Portela, R. M. C., Vogl, T., Ebner, K., Oliveira, R., and Glieder, A. (2018). Pichia pastoris Alcohol Oxidase 1 (AOX1) core promoter engineering by high resolution systematic mutagenesis. Biotechnol. J. 13:e1700340. doi: 10.1002/biot.201700340
Potgieter, T. I., Cukan, M., Drummond, J. E., Houston-Cummings, N. R., Jiang, Y. W., Li, F., et al. (2009). Production of monoclonal antibodies by glycoengineered Pichia pastoris. J. Biotechnol. 139, 318–325. doi: 10.1016/j.jbiotec.2008.12.015
Prabhu, A. A., Boro, B., Bharali, B., Chakraborty, S., and Dasu, V. V. (2017). Gene and process level modulation to overcome the bottlenecks of re-combinant proteins expression in Pichia pastoris. Curr. Pharm. Biotechnol. 18, 1200–1223. doi: 10.2174/1389201019666180329112827
Prielhofer, R., Maurer, M., Klein, J., Wenger, J., Kiziak, C., Gasser, B., et al. (2013). Induction without methanol: Novel regulated promoters enable high-level expression in Pichia pastoris. Microb. Cell Fact. 12:5. doi: 10.1186/1475-2859-12-5
Qian, S., Fusheng, C., Fang, G., Yongkang, L., Siyi, G., and Zhengqiang, J. (2018). A novel aspartic protease from Rhizomucor miehei expressed in Pichia pastoris and its application on meat tenderization and preparation of turtle peptides. Food Chem. 245, 570–577. doi: 10.1016/j.foodchem.2017.10.113
Radoman, B., Grunwald-Gruber, C., Schmelzer, B., Zavec, D., Gasser, B., Altmann, F., et al. (2021). The degree and length of O-glycosylation of recombinant proteins produced in Pichia pastoris depends on the nature of the protein and the process type. Biotechnol. J. 16:e2000266. doi: 10.1002/biot.202000266
Raschmanova, H., Weninger, A., Knejzlik, Z., Melzoch, K., and Kovar, K. (2021). Engineering of the unfolded protein response pathway in Pichia pastoris: Enhancing production of secreted recombinant proteins. Appl. Microbiol. Biotechnol. 105, 4397–4414. doi: 10.1007/s00253-021-11336-5
Raschmanova, H., Zamora, I., Borcinova, M., Meier, P., Weninger, A., Machler, D., et al. (2019). Single-cell approach to monitor the unfolded protein response during biotechnological processes with Pichia pastoris. Front. Microbiol. 10:335. doi: 10.3389/fmicb.2019.00335
Saitua, F., Torres, P., Perez-Correav, J. R., and Agosin, E. (2017). Dynamic genome-scale metabolic modeling of the yeast Pichia pastoris. BMC Syst. Biol. 11:27. doi: 10.1186/s12918-017-0408-2
Sanchez, B. J., Perez-Correa, J. R., and Agosin, E. (2014). Construction of robust dynamic genome-scale metabolic model structures of Saccharomyces cerevisiae through iterative re-parameterization. Metab. Eng. 25, 159–173. doi: 10.1016/j.ymben.2014.07.004
Sander, J. D., and Joung, J. K. (2014). CRIRISPR-Cas systems for editing, regulating and targeting genomes. Nat. Biotechnol. 32, 347–355. doi: 10.1038/nbt.2842
Schultz, J. C., Cao, M. F., Mejia, A., and Zhao, H. M. (2021). CUT&RUN identifies centromeric DNA regions of Rhodotorula toruloides IFO0880. FEMS Yeast Res. 21:foab066. doi: 10.1093/femsyr/foab066
Schwarzhans, J. P., Luttermann, T., Geier, M., Kalinowski, J., and Friehs, K. (2017). Towards systems metabolic engineering in Pichia pastoris. Biotechnol. Adv. 35, 681–710. doi: 10.1016/j.biotechadv.2017.07.009
Shen, W. L., Yang, N., Teng, D., Hao, Y., Ma, X. X., Mao, R. Y., et al. (2021). Design and high expression of non-glycosylated lysostaphins in Pichia pastoris and their pharmacodynamic study. Front. Microbiol. 12:637662. doi: 10.3389/fmicb.2021.637662
Sigar, M., Maity, N., and Mishra, S. (2017). Enhancing granulocyte colony-stimulating factor expression in Pichia pastoris through fusion with human serum albumin. Prep. Biochem. Biotechnol. 47, 364–370. doi: 10.1080/10826068.2016.1252922
Singh, V., Braddick, D., and Dhar, P. K. (2017). Exploring the potential of genome editing CRISPR-Cas9 technology. Gene 599, 1–18. doi: 10.1016/j.gene.2016.11.008
Sinha, J., Plantz, B. A., Inan, M., and Meagher, M. M. (2005). Causes of proteolytic degradation of secreted recombinant proteins produced in methylotrophic yeast Pichia pastoris: Case study with recombinant ovine interferon-tau. Biotechnol. Bioeng. 89, 102–112. doi: 10.1002/bit.20318
Sjostrom, S. L., Bai, Y. P., Huang, M. T., Liu, Z. H., Nielsen, J., Joensson, H. N., et al. (2014). High-throughput screening for industrial enzyme production hosts by droplet microfluidics. Lab Chip 14, 806–813. doi: 10.1039/C3LC51202A
Takagi, S., Tsutsumi, N., Terui, Y., Kong, X. Y., Yurimoto, H., and Sakai, Y. (2019). Engineering the expression system for Komagataella phaffii (Pichia pastoris): An attempt to develop a methanol-free expression system. FEMS Yeast Res. 19:foz059. doi: 10.1093/femsyr/foz059
Theron, C. W., Berrios, J., Delvigne, F., and Fickers, P. (2018). Integrating metabolic modeling and population heterogeneity analysis into optimizing recombinant protein production by Komagataella (Pichia) pastoris. Appl. Microbiol. Biotechnol. 102, 63–80. doi: 10.1007/s00253-017-8612-y
Tolner, B., Smith, L., Begent, R., and Chester, K. A. (2006). Production of recombinant protein in Pichia pastoris by fermentation. Nat. Protoc. < < cps:it > refvol < /cps:it > > 1, 1006–1021. doi: 10.1038/nprot.2006.126
Tomas-Gamisans, M., Ferrer, P., and Albiol, J. (2018). Fine-tuning the P-pastoris iMT1026 genome-scale metabolic model for improved prediction of growth on methanol or glycerol as sole carbon sources. Microb. Biotechnol. 11, 224–237. doi: 10.1111/1751-7915.12871
Torres, P., Saa, P. A., Albiol, J., Ferrer, P., and Agosin, E. (2019). Contextualized genome-scale model unveils high-order metabolic effects of the specific growth rate and oxygenation level in recombinant Pichia pastoris. Metab. Eng. Commun. 9:e00103. doi: 10.1016/j.mec.2019.e00103
Totaro, D., Radoman, B., Schmelzer, B., Rothbauer, M., Steiger, M. G., Mayr, T., et al. (2021). Microscale perfusion-based cultivation forPichia pastorisClone screening enables accelerated and optimized recombinant protein production processes. Biotechnol. J. 16:2000215. doi: 10.1002/biot.202000215
Tredwell, G. D., Aw, R., Edwards-Jones, B., Leak, D. J., and Bundy, J. G. (2017). Rapid screening of cellular stress responses in recombinant Pichia pastoris strains using metabolite profiling. J. Ind. Microbiol. Biotechnol. 44, 413–417. doi: 10.1007/s10295-017-1904-5
Unver, Y., Yildiz, M., Kilic, D., Taskin, M., Firat, A., and Askin, H. (2018). Efficient expression of recombinant human telomerase inhibitor 1 (hPinX1) in Pichia pastoris. Prep. Biochem. Biotechnol. 48, 535–540. doi: 10.1080/10826068.2018.1466160
Vogl, T. (2022). Engineering of promoters for gene expression in Pichia pastoris. Methods Mol. Biol. 2513, 153–177. doi: 10.1007/978-1-0716-2399-2_10
Vogl, T., Fischer, J. E., Hyden, P., Wasmayer, R., Sturmberger, L., and Glieder, A. (2020). Orthologous promoters from related methylotrophic yeasts surpass expression of endogenous promoters of Pichia pastoris. AMB Express 10:38. doi: 10.1186/s13568-020-00972-1
Vogl, T., Gebbie, L., Palfreyman, R. W., and Speight, R. (2018a). Effect of plasmid design and type of integration event on recombinant protein expression in Pichia pastoris. Appl. Environ. Microbiol. 84:e02712-17. doi: 10.1128/AEM.02712-17
Vogl, T., Sturmberger, L., Fauland, P. C., Hyden, P., Fischer, J. E., Schmid, C., et al. (2018b). Methanol independent induction in Pichia pastoris by simple derepressed overexpression of single transcription factors. Biotechnol. Bioeng. 115, 1037–1050. doi: 10.1002/bit.26529
Vogl, T., and Glieder, A. (2013). Regulation of Pichia pastoris promoters and its consequences for protein production. New Biotechnol. 30, 385–404. doi: 10.1016/j.nbt.2012.11.010
Vogl, T., Kickenweiz, T., Pitzer, J., Sturmberger, L., Weninger, A., Biggs, B. W., et al. (2021). Engineered bidirectional promoters enable rapid multi-gene co-expression optimization. Nat. Commun. 12:1287. doi: 10.1038/s41467-021-21369-z
Vogl, T., Sturmberger, L., Kickenweiz, T., Wasmayer, R., Schmid, C., Hatzl, A. M., et al. (2016). A Toolbox of Diverse Promoters Related to Methanol Utilization: Functionally Verified Parts for Heterologous Pathway Expression in Pichia pastoris. Acs Synth. Biol. 5, 172–186. doi: 10.1021/acssynbio.5b00199
Wang, G. K., Huang, M. T., and Nielsen, J. (2017). Exploring the potential of Saccharomyces cerevisiae for biopharmaceutical protein production. Curr. Opin. Biotechnol. 48, 77–84. doi: 10.1016/j.copbio.2017.03.017
Wang, J. J., Wang, X. L., Shi, L., Qi, F., Zhang, P., Zhang, Y. X., et al. (2017). Methanol-independent protein expression by AOX1 promoter with trans-acting elements engineering and glucose-glycerol-shift induction in Pichia pastoris. Sci. Rep. 7:41850. doi: 10.1038/srep41850
Wang, X. L., Wang, Q., Wang, J. J., Bai, P., Shi, L., Shen, W., et al. (2016). Mit1 transcription factor mediates methanol signaling and regulates the alcohol Oxidase 1 (AOX1) Promoter in Pichia pastoris. J. Biol. Chem. 291, 6245–6261. doi: 10.1074/jbc.M115.692053
Wang, Z. Y., Guo, C., Liu, L., and Huang, H. (2018). Effects of N-glycosylation on the biochemical properties of recombinant bEK(L) expressed in Pichia pastoris. Enzyme Microb. Technol. 114, 40–47. doi: 10.1016/j.enzmictec.2018.03.004
Wei, S., Ying, X., Yiqi, L., Xiaolong, W., Mengmeng, H., Menghao, C., et al. (2016). A novel methanol-free Pichia pastoris system for recombinant protein expression. Microb. Cell Fact. 15:178. doi: 10.1186/s12934-016-0578-4
Weninger, A., Fischer, J. E., Raschmanova, H., Kniely, C., Vogl, T., and Glieder, A. (2018). Expanding the CRISPR/Cas9 toolkit for Pichia pastoris with efficient donor integration and alternative resistance markers. J. Cell. Biochem. 119, 3183–3198. doi: 10.1002/jcb.26474
Weninger, A., Hatzl, A. M., Schmid, C., Vogl, T., and Glieder, A. (2016). Combinatorial optimization of CRISPR/Cas9 expression enables precision genome engineering in the methylotrophic yeast Pichia pastoris. J. Biotechnol. 235, 139–149. doi: 10.1016/j.jbiotec.2016.03.027
Xu, H., Guo, M. Y., Gao, Y. H., Bai, X. H., and Zhou, X. W. (2017). Expression and characteristics of manganese peroxidase from Ganoderma lucidum in Pichia pastoris and its application in the degradation of four dyes and phenol. BMC Biotechnol. 17:19. doi: 10.1186/s12896-017-0338-5
Yang, M., Yu, X. W., Zheng, H. Y., Sha, C., Zhao, C. F., Qian, M. Q., et al. (2015). Role of N-linked glycosylation in the secretion and enzymatic properties of Rhizopus chinensis lipase expressed in Pichia pastoris. Microb. Cell Fact. 14, 40. doi: 10.1186/s12934-015-0225-5
Yang, Y. K., Liu, G. Q., Chen, X., Liu, M., Zhan, C. J., Liu, X. X., et al. (2020). High efficiency CRISPR/Cas9 genome editing system with an eliminable episomal sgRNA plasmid in Pichia pastoris. Enzyme Microb. Technol. 138:109556. doi: 10.1016/j.enzmictec.2020.109556
Yang, Z., and Zhang, Z. (2018). Engineering strategies for enhanced production of protein and bio-products in Pichia pastoris: A review. Biotechnol. Adv. 36, 182–195. doi: 10.1016/j.biotechadv.2017.11.002
Yasemi, M., and Jolicoeur, M. (2021). Modelling cell metabolism: A review on constraint-based steady-state and kinetic approaches. Processes 9:322. doi: 10.3390/pr9020322
Ye, R., Huang, M. Z., Lu, H. Z., Qian, J. C., Lin, W. L., Chu, J., et al. (2017). Comprehensive reconstruction and evaluation of Pichia pastoris genome-scale metabolic model that accounts for 1243 ORFs. Bioresour. Bioprocess. 4:22. doi: 10.1186/s40643-017-0152-x
Yuan, H. L., Zhou, Y., Lin, Y. P., Tu, R., Guo, Y. F., Zhang, Y. Y., et al. (2022). Microfluidic screening and genomic mutation identification for enhancing cellulase production in Pichia pastoris. Biotechnol. Biofuels Bioprod. 15:50. doi: 10.1186/s13068-022-02150-w
Zahrl, R. J., Pena, D. A., Mattanovich, D., and Gasser, B. (2017). Systems biotechnology for protein production in Pichia pastoris. Fems Yeast Res. 17:fox068. doi: 10.1093/femsyr/fox068
Zepeda, A. B., Figueroa, C. A., Pessoa, A., and Farias, J. G. (2018). Free fatty acids reduce metabolic stress and favor a stable production of heterologous proteins in Pichia pastoris. Braz. J. Microbiol. 49, 856–864. doi: 10.1016/j.bjm.2018.03.008
Zhan, C. J., Yang, Y. K., Zhang, Z. Y., Li, X., Liu, X. X., and Bai, Z. H. (2017). Transcription factor Mxr1 promotes the expression of Aox1 by repressing glycerol transporter 1 in Pichia pastoris. Fems Yeast Res. 17:fox015. doi: 10.1093/femsyr/fox015
Zhu, T. C., Sun, H. B., Wang, M. Y., and Li, Y. (2019). Pichia pastoris as a versatile cell factory for the production of industrial enzymes and chemicals: Current status and future perspectives. Biotechnol. J. 14:e1800694. doi: 10.1002/biot.201800694
Zou, S. P., Huang, S., Kaleem, I., and Li, C. (2013). N-glycosylation enhances functional and structural stability of recombinant beta-glucuronidase expressed in Pichia pastoris. J. Biotechnol. 164, 75–81. doi: 10.1016/j.jbiotec.2012.12.015
Keywords: Pichia pastoris, recombinant protein, CRISPR/Cas9, metabolic engineering, modeling
Citation: Pan Y, Yang J, Wu J, Yang L and Fang H (2022) Current advances of Pichia pastoris as cell factories for production of recombinant proteins. Front. Microbiol. 13:1059777. doi: 10.3389/fmicb.2022.1059777
Received: 02 October 2022; Accepted: 07 November 2022;
Published: 24 November 2022.
Edited by:
Nuno Pereira Mira, University of Lisbon, PortugalCopyright © 2022 Pan, Yang, Wu, Yang and Fang. This is an open-access article distributed under the terms of the Creative Commons Attribution License (CC BY). The use, distribution or reproduction in other forums is permitted, provided the original author(s) and the copyright owner(s) are credited and that the original publication in this journal is cited, in accordance with accepted academic practice. No use, distribution or reproduction is permitted which does not comply with these terms.
*Correspondence: Hao Fang, ZmFuZ2hAemp1LmVkdS5jbg==
Disclaimer: All claims expressed in this article are solely those of the authors and do not necessarily represent those of their affiliated organizations, or those of the publisher, the editors and the reviewers. Any product that may be evaluated in this article or claim that may be made by its manufacturer is not guaranteed or endorsed by the publisher.
Research integrity at Frontiers
Learn more about the work of our research integrity team to safeguard the quality of each article we publish.