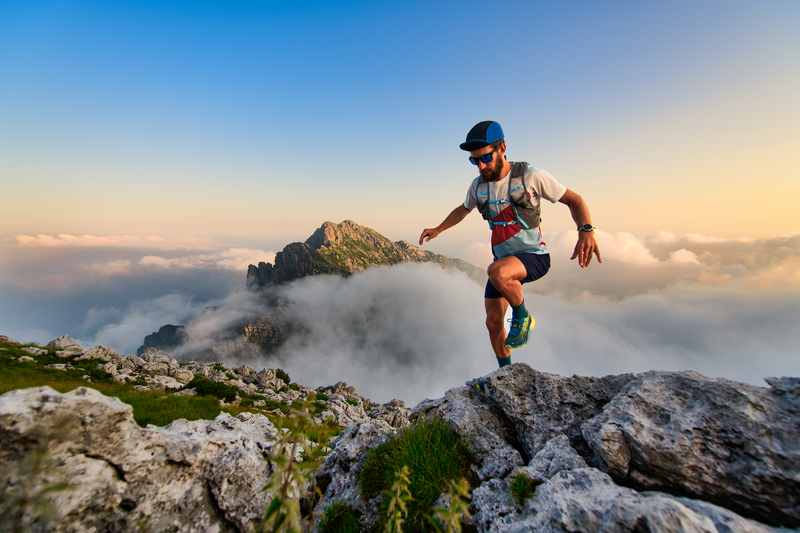
94% of researchers rate our articles as excellent or good
Learn more about the work of our research integrity team to safeguard the quality of each article we publish.
Find out more
ORIGINAL RESEARCH article
Front. Microbiol. , 04 November 2022
Sec. Antimicrobials, Resistance and Chemotherapy
Volume 13 - 2022 | https://doi.org/10.3389/fmicb.2022.1047109
Aztreonam-avibactam is a promising combination to treat carbapenem-resistant Enterobacterales including coverage for metallo-β-lactamases. Escherichia coli strains resistant to aztreonam-avibactam have emerged but resistance mechanisms remain to be elucidated. We performed a study to investigate the mechanism for aztreonam-avibactam in a carbapenem-resistant Escherichia coli clinical strain. This strain was resistant to aztreonam-avibactam (aztreonam MIC, 16 mg/L in the presence of 4 mg/L avibactam). Whole genome sequencing revealed that the strain carried metallo-β-lactamase gene blaNDM-4 and the extended-spectrum β-lactamase (ESBL) gene blaCTX-M-15 and had a YRIK four amino acid insertion in penicillin-binding protein 3 (PBP3). blaCTX-M-15 was cloned into pET-28a(+), followed by the transformation, with the gene, of E. coli strain 035125∆pCMY42 possessing the YRIK insertion in PBP3 and strain BL21 with the wildtype PBP3. blaCTX-M-14, another common ESBL gene, and blaCTX-M-199, a hybrid of blaCTX-M-14 and blaCTX-M-15 were also individually cloned into both E. coli strains for comparison. Aztreonam-avibactam resistance was only observed in the E. coli strains with the YRIK insertion in PBP3 that produced CTX-M-15 or its hybrid enzyme CTX-M-199. Checkerboard titration assays were performed to determine the synergistic effects between aztreonam-avibactam and ceftazidime or meropenem. Doubling avibactam concentration in vitro reversed aztreonam-avibactam resistance, while the combination of aztreonam-avibactam and ceftazidime or meropenem did not. In conclusion, CTX-M enzymes with activity against aztreonam, (e.g., CTX-M-15 and CTX-M-199), can confer resistance in the combination of PBP3 with YRIK insertions in metallo-β-lactamase-producing carbapenem-resistant E. coli. Doubling the concentration of avibactam may overcome such resistance.
Production of metallo-β-lactamases (MBLs), in particular NDM, is a major mechanism mediating resistance to carbapenems in Escherichia coli (Zhang R. et al., 2017; Wang et al., 2018), a member of the order Enterobacterales. MBLs can also hydrolyze cephalosporins and penicillins but not aztreonam (ATM), a monobactam (Yong et al., 2009; Nordmann et al., 2011). However, MBL-producing carbapenem-resistant Enterobacterales often produces extended-spectrum β-lactamases (ESBLs; e.g., CTX-M enzymes) and/or AmpC cephalosporinases such as CMY enzymes, both of which are serine-based enzymes, and therefore confer resistance to ATM (Wu et al., 2019). Currently, no inhibitors of MBLs have been approved for clinical use. Avibactam (AVI), a non-β-lactam β-lactamase inhibitor, is able to inhibit serine-based β-lactamases but not MBLs (Livermore et al., 2011). The combination of aztreonam-avibactam (ATM-AVI) has activity against isolates producing either serine-based β-lactamases or MBLs or both and has been recommended to treat MBL-producing carbapenem-resistant Enterobacterales (Tamma et al., 2021; Paul et al., 2022).
However, ATM-AVI resistance has also emerged in E. coli (Alm et al., 2015; Russ et al., 2020) but the mechanism for resistance remains poorly understood. Several studies have demonstrated that the insertion of four amino acids (YRIN, YRIK, or YRIP) in penicillin-binding protein 3 (PBP3) leads to reduced susceptibility to ATM-AVI but does not reach the breakpoint of clinical resistance (ATM MIC ≥16 mg/L in the presence of AVI; Alm et al., 2015; Zhang Y. et al., 2017; Ma et al., 2020; Mendes et al., 2021; Ma et al., 2022). The insertion of four amino acids in PBP3 is due to a duplication of a 12-nucleotide sequence, TATCGAATTAAC for YRIN, TATCGAATTAAA for YRIK, or TATCGCATTCCT for YRIP, in the encoding gene ftsI (Alm et al., 2015; Ma et al., 2020; Wang et al., 2022) and the three types of insertion have the same impact on the susceptibility to ATM-AVI (Ma et al., 2020). When any of these four amino acid insertions are present in PBP3, the AmpC enzyme CMY-42 but not CMY-2 can confer resistance to ATM-AVI (Ma et al., 2020; Sadek et al., 2020). CMY-42 differs from CMY-2 by an amino acid substitution (S211V, Ambler position; Hentschke et al., 2011), which renders 8-fold higher MIC against ATM (Ma et al., 2020). The discrepancy between CMY-42 and CMY-2 in ATM-AVI resistance suggests that other β-lactamases with significant activity against ATM could involve in such resistance. CTX-M-14 and CTX-M-15 are the two major types of ESBLs in E. coli (Bevan et al., 2017). In this study, we report that CTX-M-15 but not CTX-M-14 can confer resistance to ATM-AVI in the presence of PBP3 with a YRIK insertion in E. coli, representing a new resistance mechanism of clinical significance.
We have previously reported three ST405 carbapenem-resistant E. coli clinical isolates, WCHEC96200, WCHEC1837, and WCHEC99540, of the same clone (Zhang et al., 2018). As the three isolates carry blaNDM-4, we tested their in vitro susceptibilities to ATM and ATM-AVI using broth microdilution according to the Clinical and Laboratory Standards Institute (CLSI; CLSI, 2022) and the breakpoints of ATM were applied for ATM-AVI as there were no available criteria for ATM-AVI. In vitro susceptibility testing throughout the study was performed in triplicate.
The −10, and −35 boxes within the promotor of blaCTX-M-14, blaCTX-M-15 and blaCTX-M-199 were predicted using BPROM.1 EachblaCTX-M gene and corresponding promoter sequence were amplified using PrimeSTAR Max DNA Polymerase (Takara; Dalian, China) and primers which contained restriction sites (sequences underlined) for cloning into. pET-28a(+; Miaolingbio; Wuhan, China). blaCTX-M-14 and its promoter sequence were amplified from a local E. coli isolate with primers NdeI-ctx14-up (5’-AATCATATGCATCAGCAAAAGGGGATGAT-3′; the restriction site is underlined in all primer sequences) and EcoRI-ctx14-dw (5’-AAAGAATTCCTGCGTTGTCGGGAAGATAC-3′). blaCTX-M-15 and its promoter sequence were amplified from isolate WCHEC96200 (Zhang et al., 2018) with primers NdeI-ctx15-up (5′-AATCATATGCGGTGGGTCATCTCTTGCTA-3′) and EcoRI-ctx15-dw (5′- AAAGAATTCCCAGGAACCACGGAGCTTAT-3′). blaCTX-M-199 and its promoter sequence were amplified with primers NheI-ctx199-up (5′-AAAGCTAGCTGAAAAGCGTGGTAATGCTG-3′) and BamHI-ctx199-dw (5′-AAAGGATCCGAGCTTATGGCCTGGTATGC-3′) from strain WCHEC025970, which we have previously reported (GenBank accession no. CP036178).
Amplicons were purified and digested with the appropriate restriction enzyme combination (NdeI and EcoRI or NheI and BamHI; Takara) and were individually ligated into similarly digested fragments of the vector pET-28a(+; Miaolingbio; Wuhan, China)using T4 ligase (Takara). The resultant plasmid constructs pET-CTXM14, pET-CTXM15, and pET-CTXM199, were individually transformed into E. coli strain BL21 (with wild-type PBP3) and strain 035125△pCMY42 (possessing a YRIK insertion in PBP3), a ST410 E. coli strain cured of the plasmid carrying blaCMY-42 (Ma et al., 2020), respectively, using the chemical method. Potential transformants were screened on LB agar plates containing 50 mg/L kanamycin. The presence of blaCTX-M-14, blaCTX-M-15, and blaCTX-M-199 in the corresponding transformants, BL21::CTX-M-14, BL21::CTX-M-15, BL21::CTX-M-199, 035125△pCMY42::CTX-M-14, 035125△pCMY42::CTX-M-15, and 035125△pCMY42::CTX-M-199 were verified by PCR using Primers T7 (5′-TAATACGACTCACTATAGGG-3′) and T7ter (5′-TGCTAGTTATTGCTCAGCGG-3′) and subsequent Sanger sequencing. Minimum inhibitory concentrations (MICs) of ATM and ATM-AVI against the transformants were determined by broth microdilution (CLSI, 2022).
Amino acid sequences of the three CTX-M enzymes were aligned using Clustal Omega2 with default settings.
Checkerboard titration assays were performed as described previously (Eucast, 2000) for the blaNDM-4-carrying isolate WCHEC96200 (Zhang et al., 2018) to determine the synergistic effects between ATM-AVI and ceftazidime (CAZ) or meropenem (MEM) in 96-well microtiter plates. Antimicrobial agents were diluted by Cation-adjusted Mueller Hinton Broth (Haibo; Qingdao, China) to obtain eight two-fold serial concentration gradients from the concentrations equal to the MICs of each agent or 1,024 mg/L if MIC was >1,024 mg/L. The fractional inhibitory concentration index (FICI) was calculated by the following: (MIC of ATM-AVI in combination with [CAZ or MEM])/(MIC of ATM-AVI) + (MIC of [CAZ or MEM] in combination with ATM-AVI)/(MIC of CAZ or MEM; Eucast, 2000). The calculated FICI was defined as synergism (≤ 0.5), indifference (> 0.5 to ≤4.0) and antagonism (> 4.0), respectively as described previously (Eucast, 2000).
MICs of ATM against strain WCHEC96200 were also determined in the presence of 8 mg/L AVI (rather than 4 mg/L in CLSI recommendations) using broth microdilution (CLSI, 2022).
The three ST405 carbapenem-resistant E. coli clinical isolates that carried blaNDM-4 (Zhang et al., 2018) were resistant to ATM (MIC >1,024 mg/L) and ATM-AVI (MIC 16/4 mg/L). Like other NDM variants, NDM-4 has no significant activity against ATM (Nordmann et al., 2012) and therefore its presence cannot explain the resistance to ATM and ATM-AVI. Draft genome sequences of the three isolates (GenBank accession no. NGUU00000000, NGUV00000000 and NGUW00000000; Zhang et al., 2018) were examined to identify potential mechanisms associated with ATM-AVI resistance. All three isolates had a four amino acid (YRIK) insertion in PBP3 and carried blaCTX-M-15, which is able to hydrolyze ATM (Zhang et al., 2018), but can be inhibited by AVI (Livermore et al., 2011). Since blaCTX-M-15 alone cannot explain the ATM-AVI resistant phenotype in the isolates, we hypothesized that the presence of PBP3 with a YRIK insertion is playing a contributing role. As such, cloning experiments were performed to examine this hypothesis.
blaCTX-M-15 was individually cloned in E. coli strain BL21 (with wild-type PBP3) and strain 035125△pCMY42 (with a YRIK insertion in PBP3), to examine whether blaCTX-M-15 is able to mediate ATM-AVI resistance in either PBP3 background. BL21::CTX-M-15 was resistant to ATM but not to ATM-AVI, while 035125△pCMY42::CTX-M-15 was resistant to both (Table 1). We noted that The European Committee on Antimicrobial Susceptibility Testing (EUCAST)3 uses different breakpoints for aztreonam (MIC, ≤1 mg/L for ‘susceptible’ and > 4 mg/L for ‘resistant’) from CLSI (≤4 mg/L and ≥ 16 mg/L, respectively). Even using EUCAST breakpoints, 035125△pCMY42::CTX-M-14 was still not resistant to aztreonam-avibactam (4 mg/L). The above findings suggest that the combination of PBP3 with a YRIK amino acid insertion and blaCTX-M-15 can mediate resistance to ATM-AVI, while blaCTX-M-15 alone cannot.
blaCTX-M-14 is another major type of blaCTX-M in the world (Bevan et al., 2017) and therefore we sought to examine whether blaCTX-M-14 could mediate resistance to ATM-AVI in the presence of PBP3 with a YRIK insertion like blaCTX-M-15. As expected, the obtained transformant BL21::CTX-M-14 was resistant to ATM (MIC, 64 mg/L) but not to ATM-AVI (MIC, 0.015/4 mg/L). However, 035125△pCMY42::CTX-M-14 was also susceptible to ATM-AVI (MIC, 4/4 mg/L), although the susceptibility was reduced by four-fold (from 1/4 to 4/4 mg/L; Table 1). This suggests that the combination of PBP3 amino acid insertion and blaCTX-M-14 is able to confer reduced susceptibility to ATM-AVI, but the level does not reach the breakpoint to define clinical resistance. In other words, the combination of PBP3 amino acid insertion and blaCTX-M-14 is still inadequate to confer resistance to ATM-AVI.
Previously, we have found blaCTX-M-199 in an E. coli clinical strain (WCHEC025970, GenBank accession no. CP036178). CTX-M-199 is a hybrid β-lactamase comprising the N and C termini of CTX-M-15 and the middle part of CTX-M-14 (Cai et al., 2017; Figure 1). MICs of ATM and ATM-AVI against transformants containing blaCTX-M-199 were identical to those containing blaCTX-M-15 (Table 1). Of note, the promoter sequences of blaCTX-M-14, blaCTX-M-15, and blaCTX-M-199 are identical (Supplementary Figure). The identical ATM and ATM-AVI MICs suggest that the key amino acid residues involved with resistance to ATM are likely located in the N and C termini of CTX-M enzymes.
Figure 1. Alignment of CTX-M-14, CTX-M-15 and CTX-M-199. CTX-M-199 is a hybrid comprising the N- and C-termini of CTX-M-15 (highlighted in yellow) and the middle part of CTX-M-14. D in CTX-M-14 and G in CTX-M-15 and CTX-M-199 at the Amber position 240 are shown in framed letters. Position numbers shown in the figure are according to the start codon.
To explore potential approaches to overcome ATM-AVI conferred by PBP3 amino acid insertion and CTX-M-15 in carbapenem-resistant E. coli strains, we attempted two approaches. First, we performed checkerboard titration assays that assessed the activity of ATM-AVI in combination with CAZ [as synergy among these has been reported before (Wenzler et al., 2017)] or MEM [as it is not hydrolyzed by CTX-M-15 and susceptibility is not impaired by PBP3 amino acid insertion (Alm et al., 2015)] on the blaNDM-4-carrying strain WCHEC96200. No synergistic effects between ATM-AVI and CAZ or MEM with >1 or 1.5 fractional inhibitory concentration index (FICI; Table 2). Second, we increased the concentration of AVI from 4 to 8 mg/L and found that in the presence of 8 mg/L AVI, the MIC of ATM for WCHEC96200 reduced from 16 to 4 mg/L, which is in the susceptible range. This suggests that doubling the concentration of AVI was able to overcome ATM-AVI resistance seen in E. coli producing CTX-M-15 and with the YRIK insertion in PBP3.
We demonstrated that in E. coli possessing PBP3 with a YRIK insertion that CTX-M-15 (and the hybrid CTX-M-199) could confer resistance to ATM-AVI but not CTX-M-14. Kinetic studies have found that CTX-M-15 (Poirel et al., 2002; Faheem et al., 2013) has strong hydrolytic activity to ATM and is able to confer high-level resistance to this agent, while CTX-M-14 has much weaker activity against ATM (Dutour et al., 2002; Ma et al., 2002; Ishii et al., 2007). The kinetic analysis of CTX-M-199 against ATM has not been reported (Cai et al., 2017; Chen et al., 2021) but we found that E. coli transformants containing blaCTX-M-199 was resistant to ATM (MIC, 128 mg/L), suggesting that CTX-M-199 is able to efficiently hydrolyze this agent. Therefore, in the presence of PBP3 with an amino acid insertion, ESBLs able to hydrolyze ATM efficiently can confer resistance to ATM-AVI. Previous studies have found that Ambler position 240 of class A β-lactamases was critical for hydrolyzing CAZ and ATM (Cantu et al., 1996; Bonnet et al., 2000). In this position, CTX-M-14 has a D, while CTX-M-15 and CTX-M-199 has a G (Figure 1) and it has been well documented that the D240G amino acid substitution of CTX-M enzymes enhances hydrolysis of CAZ (Bonnet et al., 2003; Cartelle et al., 2004; Celenza et al., 2008; Novais et al., 2008). ATM and CAZ share an identical bulky oxyimino R1 side-chain connected the β-lactam ring (Mitchell et al., 2015). The D240G substitution has also been found to enhance activity against ATM by increasing the affinity (Bonnet et al., 2001; Poirel et al., 2002; Cartelle et al., 2004). In addition, CTX-M-15 appears to have a higher kcat (1.5 S−1; Poirel et al., 2002) compared to CTX-M-14 (kcat, <0.01 S−1; Ma et al., 2002). This suggests that CTX-M-15 is likely to hydrolyze ATM more rapidly than CTX-M-14, contributing to the stronger activity of the former enzyme against ATM.
It is known that the inhibition of β-lactamases by AVI is reversible by recyclization (Ehmann et al., 2012; Lahiri et al., 2014) and usually does not result in hydrolysis (Lahiri et al., 2014). This suggests that some free forms of CTX-M enzymes are likely to exist in the presence of AVI and are therefore available to attack ATM. As described previously, the four-amino-acid insertion is located in the tight turn between the β2b–β2c sheets adjacent to the β-lactam binding pocket pf PBP3, resulting in reduced affinity to PBP3-binding β-lactams such as ATM (Alm et al., 2015). The reduced affinity of PBP3 increases the amount of ATM exposed to CTX-M enzymes, which may need higher concentrations of AVI to protect the increased amount of ATM from the enzymatic hydrolysis. This could explain that doubling the concentration of AVI is able to reverse ATM-AVI resistance conferred by CTX-M-15 and CTX-M-199.
We are aware of limitations of our study. First, we used strain 035125△pCMY42 for studying the impact of CTX-M in the presence of a four-amino-acid insertion in PBP3 and strain BL21 for studying that of CTX-M in the absence of such an insertion. The two strains have a different genetic background, which introduces confounding factors for the cloning experiments. Nonetheless, we compared 035125△pCMY42 with and without blaCTX-M and then compared BL21 with and without blaCTX-M. The two pairs of comparisons support the presence of joint effects of CTX-M enzymes and the PBP3 alteration on the susceptibility to ATM-AVI. Second, we did not perform experiments to investigate the interaction among CTX-M enzymes, AVI, and PBP3 with or without the four-amino-acid insertion. Therefore, the exact mechanisms that CTX-M-15 and CTX-M-199 confer resistance to ATM-AVI while CTX-M-14 leads to reduced susceptibility in E. coli possessing a four-amino-acid insertion in PBP3 are still not clear. Nonetheless, the observation that production of CTX-M-15, a very common ESBL, could confer resistance to ATM-AVI in E. coli with PBP3 insertion, which is increasingly reported (Mendes et al., 2021; Wang et al., 2022) is clinically relevant. Third, we did not perform in vivo studies to verify that the ATM-AVI resistance could be reversed by increasing the concentration of AVI. The clinical implications for this in vitro observation remain to be investigated.
In conclusion, CTX-M enzymes with significant activity against ATM (e.g., CTX-M-15 and CTX-M-199) can confer resistance in MBL-producing carbapenem-resistant E. coli possessing the four-amino-acid insertion PBP3, further compromising therapeutic options. Doubling the concentration of AVI may overcome such resistance. Further studies of ATM-AVI resistance mechanisms in MBL-producing Enterobacterales are warranted to inform clinical practice.
The datasets presented in this study can be found in online repositories. The names of the repository/repositories and accession number(s) can be found at: https://www.ncbi.nlm.nih.gov/, NGUU00000000; https://www.ncbi.nlm.nih.gov/, NGUV00000000; https://www.ncbi.nlm.nih.gov/, NGUW00000000.
ZZ conceived and designed the study. KM performed the experiments. KM and ZZ analyzed the data and wrote the manuscript. All authors contributed to the article and approved the submitted version.
The relevant works of the authors were supported by the National Natural Science Foundation of China (82172309 and 81861138055) and by grants from the West China Hospital of Sichuan University (1.3.5 project for disciplines of excellence, grant number ZYYC08006 and ZYGD22001).
We are grateful for Björn Espedido, Sydney, NSW, Australia for his proofreading and helpful suggestions.
The authors declare that the research was conducted in the absence of any commercial or financial relationships that could be construed as a potential conflict of interest.
All claims expressed in this article are solely those of the authors and do not necessarily represent those of their affiliated organizations, or those of the publisher, the editors and the reviewers. Any product that may be evaluated in this article, or claim that may be made by its manufacturer, is not guaranteed or endorsed by the publisher.
The Supplementary material for this article can be found online at: https://www.frontiersin.org/articles/10.3389/fmicb.2022.1047109/full#supplementary-material
1. ^http://www.softberry.com/berry.phtml?topic=bprom&group=programs&subgroup=gfindb
Alm, R. A., Johnstone, M. R., and Lahiri, S. D. (2015). Characterization of Escherichia coli NDM isolates with decreased susceptibility to aztreonam/avibactam: role of a novel insertion in PBP3. J. Antimicrob. Chemother. 70, 1420–1428. doi: 10.1093/jac/dku568
Bevan, E. R., Jones, A. M., and Hawkey, P. M. (2017). Global epidemiology of CTX-M β-lactamases: temporal and geographical shifts in genotype. J. Antimicrob. Chemother. 72, 2145–2155. doi: 10.1093/jac/dkx146
Bonnet, R., Dutour, C., Sampaio, J. L., Chanal, C., Sirot, D., Labia, R., et al. (2001). Novel cefotaximase (CTX-M-16) with increased catalytic efficiency due to substitution Asp-240-->Gly. Antimicrob. Agents Chemother. 45, 2269–2275. doi: 10.1128/AAC.45.8.2269-2275.2001
Bonnet, R., Recule, C., Baraduc, R., Chanal, C., Sirot, D., De Champs, C., et al. (2003). Effect of D240G substitution in a novel ESBL CTX-M-27. J. Antimicrob. Chemother. 52, 29–35. doi: 10.1093/jac/dkg256
Bonnet, R., Sampaio, J. L., Chanal, C., Sirot, D., De Champs, C., Viallard, J. L., et al. (2000). A novel class a extended-spectrum β-lactamase (BES-1) in Serratia marcescens isolated in Brazil. Antimicrob. Agents Chemother. 44, 3061–3068. doi: 10.1128/AAC.44.11.3061-3068.2000
Cai, J., Cheng, Q., Shen, Y., Gu, D., Fang, Y., Chan, E. W., et al. (2017). Genetic and functional characterization of blaCTX-M-199, a novel tazobactam and sulbactam resistance-encoding gene located in a conjugative mcr-1-bearing IncI2 plasmid. Antimicrob. Agents Chemother. 61, e00562–e00517. doi: 10.1128/AAC.00562-17
Cantu, C. 3rd, Huang, W., and Palzkill, T. (1996). Selection and characterization of amino acid substitutions at residues 237-240 of TEM-1 β-lactamase with altered substrate specificity for aztreonam and ceftazidime. J. Biol. Chem. 271, 22538–22545. doi: 10.1074/jbc.271.37.22538
Cartelle, M., Del Mar Tomas, M., Molina, F., Moure, R., Villanueva, R., and Bou, G. (2004). High-level resistance to ceftazidime conferred by a novel enzyme, CTX-M-32, derived from CTX-M-1 through a single Asp240-Gly substitution. Antimicrob. Agents Chemother. 48, 2308–2313. doi: 10.1128/AAC.48.6.2308-2313.2004
Celenza, G., Luzi, C., Aschi, M., Segatore, B., Setacci, D., Pellegrini, C., et al. (2008). Natural D240G Toho-1 mutant conferring resistance to ceftazidime: biochemical characterization of CTX-M-43. J. Antimicrob. Chemother. 62, 991–997. doi: 10.1093/jac/dkn339
Chen, J., Chen, S., Jiang, Y., Zhang, R., and Cai, J. (2021). Fecal carriage and genetic characterization of CTX-M-1/9/1-producing Escherichia coli from healthy humans in Hangzhou, China. Front. Microbiol. 12:616687. doi: 10.3389/fmicb.2021.616687
CLSI (2022). Methods for dilution antimicrobial susceptibility tests for bacteria that grow aerobically, 11th Edn. Wayne, PA, USA: Clinical and Laboratory Standards Institute.
Dutour, C., Bonnet, R., Marchandin, H., Boyer, M., Chanal, C., Sirot, D., et al. (2002). CTX-M-1, CTX-M-3, and CTX-M-14 β-lactamases from Enterobacteriaceae isolated in France. Antimicrob. Agents Chemother. 46, 534–537. doi: 10.1128/AAC.46.2.534-537.2002
Ehmann, D. E., Jahic, H., Ross, P. L., Gu, R. F., Hu, J., Kern, G., et al. (2012). Avibactam is a covalent, reversible, non-β-lactam β-lactamase inhibitor. Proc. Natl. Acad. Sci. U. S. A. 109, 11663–11668. doi: 10.1073/pnas.1205073109
Eucast (2000). EUCAST definitive document e.Def 1.2, may 2000: terminology relating to methods for the determination of susceptibility of bacteria to antimicrobial agents. Clin. Microbiol. Infect. 6, 503–508. doi: 10.1046/j.1469-0691.2000.00149.x
Faheem, M., Rehman, M. T., Danishuddin, M., and Khan, A. U. (2013). Biochemical characterization of CTX-M-15 from Enterobacter cloacae and designing a novel non-β-lactam-β-lactamase inhibitor. PLoS One 8:e56926. doi: 10.1371/journal.pone.0056926
Hentschke, M., Kotsakis, S. D., Wolters, M., Heisig, P., Miriagou, V., and Aepfelbacher, M. (2011). CMY-42, a novel plasmid-mediated CMY-2 variant AmpC β-lactamase. Microb. Drug Resist. 17, 165–169. doi: 10.1089/mdr.2010.0137
Ishii, Y., Galleni, M., Ma, L., Frere, J. M., and Yamaguchi, K. (2007). Biochemical characterisation of the CTX-M-14 β-lactamase. Int. J. Antimicrob. Agents 29, 159–164. doi: 10.1016/j.ijantimicag.2006.09.005
Lahiri, S. D., Johnstone, M. R., Ross, P. L., Mclaughlin, R. E., Olivier, N. B., and Alm, R. A. (2014). Avibactam and class C β-lactamases: mechanism of inhibition, conservation of the binding pocket, and implications for resistance. Antimicrob. Agents Chemother. 58, 5704–5713. doi: 10.1128/AAC.03057-14
Livermore, D. M., Mushtaq, S., Warner, M., Zhang, J., Maharjan, S., Doumith, M., et al. (2011). Activities of NXL104 combinations with ceftazidime and aztreonam against carbapenemase-producing Enterobacteriaceae. Antimicrob. Agents Chemother. 55, 390–394. doi: 10.1128/AAC.00756-10
Ma, K., Feng, Y., Mcnally, A., and Zong, Z. (2020). Struggle to survive: the choir of target alteration, hydrolyzing enzyme, and plasmid expression as a novel aztreonam-avibactam resistance mechanism. mSystems 5, 00821–00820. doi: 10.1128/mSystems.00821-20
Ma, K., Feng, Y., and Zong, Z. (2022). Aztreonam-avibactam may not replace ceftazidime/avibactam: the case of KPC-21 carbapenemase and penicillin-binding protein 3 with four extra amino acids. Int. J. Antimicrob. Agents 60:106642. doi: 10.1016/j.ijantimicag.2022.106642
Ma, L., Ishii, Y., Chang, F. Y., Yamaguchi, K., Ho, M., and Siu, L. K. (2002). CTX-M-14, a plasmid-mediated CTX-M type extended-spectrum β-lactamase isolated from Escherichia coli. Antimicrob. Agents Chemother. 46, 1985–1988. doi: 10.1128/AAC.46.6.1985-1988.2002
Mendes, R. E., Doyle, T. B., Streit, J. M., Arhin, F. F., Sader, H. S., and Castanheira, M. (2021). Investigation of mechanisms responsible for decreased susceptibility of aztreonam/avibactam activity in clinical isolates of Enterobacterales collected in Europe, Asia and Latin America in 2019. J. Antimicrob. Chemother. 76, 2833–2838. doi: 10.1093/jac/dkab279
Mitchell, J. M., Clasman, J. R., June, C. M., Kaitany, K. C., Lafleur, J. R., Taracila, M. A., et al. (2015). Structural basis of activity against aztreonam and extended spectrum cephalosporins for two carbapenem-hydrolyzing class D β-lactamases from Acinetobacter baumannii. Biochemistry 54, 1976–1987. doi: 10.1021/bi501547k
Nordmann, P., Boulanger, A. E., and Poirel, L. (2012). NDM-4 metallo-β-lactamase with increased carbapenemase activity from Escherichia coli. Antimicrob. Agents Chemother. 56, 2184–2186. doi: 10.1128/AAC.05961-11
Nordmann, P., Poirel, L., Walsh, T. R., and Livermore, D. M. (2011). The emerging NDM carbapenemases. Trends Microbiol. 19, 588–595. doi: 10.1016/j.tim.2011.09.005
Novais, A., Canton, R., Coque, T. M., Moya, A., Baquero, F., and Galan, J. C. (2008). Mutational events in cefotaximase extended-spectrum β-lactamases of the CTX-M-1 cluster involved in ceftazidime resistance. Antimicrob. Agents Chemother. 52, 2377–2382. doi: 10.1128/AAC.01658-07
Paul, M., Carrara, E., Retamar, P., Tangden, T., Bitterman, R., Bonomo, R. A., et al. (2022). European Society of Clinical Microbiology and Infectious Diseases (ESCMID) guidelines for the treatment of infections caused by multidrug-resistant gram-negative bacilli (endorsed by European society of intensive care medicine). Clin. Microbiol. Infect. 28, 521–547. doi: 10.1016/j.cmi.2021.11.025
Poirel, L., Gniadkowski, M., and Nordmann, P. (2002). Biochemical analysis of the ceftazidime-hydrolysing extended-spectrum β-lactamase CTX-M-15 and of its structurally related β-lactamase CTX-M-3. J. Antimicrob. Chemother. 50, 1031–1034. doi: 10.1093/jac/dkf240
Russ, D., Glaser, F., Shaer Tamar, E., Yelin, I., Baym, M., Kelsic, E. D., et al. (2020). Escape mutations circumvent a tradeoff between resistance to a β-lactam and resistance to a β-lactamase inhibitor. Nat. Commun. 11:2029. doi: 10.1038/s41467-020-15666-2
Sadek, M., Juhas, M., Poirel, L., and Nordmann, P. (2020). Genetic features leading to reduced susceptibility to aztreonam-avibactam among metallo-β-lactamase-producing Escherichia coli isolates. Antimicrob. Agents Chemother. 64, 01659–01620. doi: 10.1128/AAC.01659-20
Tamma, P. D., Aitken, S. L., Bonomo, R. A., Mathers, A. J., Van Duin, D., and Clancy, C. J. (2021). Infectious Diseases Society of America guidance on the treatment of extended-spectrum β-lactamase producing Enterobacterales (ESBL-E), carbapenem-resistant Enterobacterales (CRE), and Pseudomonas aeruginosa with difficult-to-treat resistance (DTR-P. aeruginosa). Clin. Infect. Dis. 72, e169–e183. doi: 10.1093/cid/ciaa1478
Wang, Q., Jin, L., Sun, S., Yin, Y., Wang, R., Chen, F., et al. (2022). Occurrence of high levels of Cefiderocol resistance in Carbapenem-resistant Escherichia coli before its approval in China: a Report from China CRE-network. Microbiol. Spectr. 10:e0267021. doi: 10.1128/spectrum.02670-21
Wang, Q., Wang, X., Wang, J., Ouyang, P., Jin, C., Wang, R., et al. (2018). Phenotypic and genotypic characterization of carbapenem-resistant Enterobacteriaceae: data from a longitudinal large-scale CRE study in China (2012-2016). Clin. Infect. Dis. 67, S196–S205. doi: 10.1093/cid/ciy660
Wenzler, E., Deraedt, M. F., Harrington, A. T., and Danizger, L. H. (2017). Synergistic activity of ceftazidime-avibactam and aztreonam against serine and metallo-β-lactamase-producing gram-negative pathogens. Diagn. Microbiol. Infect. Dis. 88, 352–354. doi: 10.1016/j.diagmicrobio.2017.05.009
Wu, W., Feng, Y., Tang, G., Qiao, F., Mcnally, A., and Zong, Z. (2019). NDM metallo-β-lactamases and their bacterial producers in health care settings. Clin. Microbiol. Rev. 32, e00115–e00118. doi: 10.1128/CMR.00115-18
Yong, D., Toleman, M. A., Giske, C. G., Cho, H. S., Sundman, K., Lee, K., et al. (2009). Characterization of a new metallo-β-lactamase gene, blaNDM-1, and a novel erythromycin esterase gene carried on a unique genetic structure in Klebsiella pneumoniae sequence type 14 from India. Antimicrob. Agents Chemother. 53, 5046–5054. doi: 10.1128/AAC.00774-09
Zhang, X., Feng, Y., Zhou, W., Mcnally, A., and Zong, Z. (2018). Cryptic transmission of ST405 Escherichia coli carrying Bla NDM-4 in hospital. Sci. Rep. 8:390. doi: 10.1038/s41598-017-18910-w
Zhang, Y., Kashikar, A., Brown, C. A., Denys, G., and Bush, K. (2017). Unusual Escherichia coli PBP 3 insertion sequence identified from a collection of carbapenem-resistant Enterobacteriaceae tested in vitro with a combination of ceftazidime-, ceftaroline-, or aztreonam-avibactam. Antimicrob. Agents Chemother. 61, 00389–00317. doi: 10.1128/AAC.00389-17
Keywords: resistance, avibactam, aztreonam, CTX-M, Escherichia coli
Citation: Ma K and Zong Z (2022) Resistance to aztreonam-avibactam due to CTX-M-15 in the presence of penicillin-binding protein 3 with extra amino acids in Escherichia coli. Front. Microbiol. 13:1047109. doi: 10.3389/fmicb.2022.1047109
Received: 17 September 2022; Accepted: 18 October 2022;
Published: 04 November 2022.
Edited by:
Xian-Zhi Li, Health Canada, CanadaReviewed by:
Qi Wang, Peking University People's Hospital, ChinaCopyright © 2022 Ma and Zong. This is an open-access article distributed under the terms of the Creative Commons Attribution License (CC BY). The use, distribution or reproduction in other forums is permitted, provided the original author(s) and the copyright owner(s) are credited and that the original publication in this journal is cited, in accordance with accepted academic practice. No use, distribution or reproduction is permitted which does not comply with these terms.
*Correspondence: Zhiyong Zong, em9uZ3poaXlAc2N1LmVkdS5jbg==
Disclaimer: All claims expressed in this article are solely those of the authors and do not necessarily represent those of their affiliated organizations, or those of the publisher, the editors and the reviewers. Any product that may be evaluated in this article or claim that may be made by its manufacturer is not guaranteed or endorsed by the publisher.
Research integrity at Frontiers
Learn more about the work of our research integrity team to safeguard the quality of each article we publish.