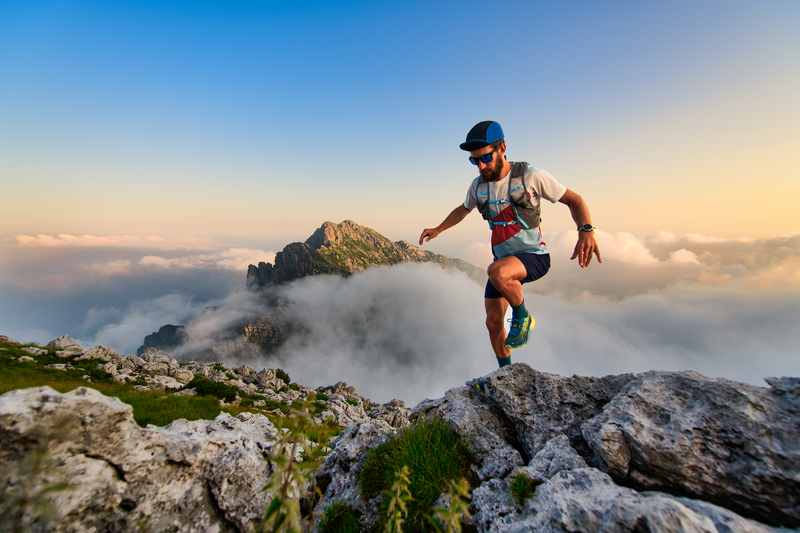
95% of researchers rate our articles as excellent or good
Learn more about the work of our research integrity team to safeguard the quality of each article we publish.
Find out more
ORIGINAL RESEARCH article
Front. Microbiol. , 11 November 2022
Sec. Microbe and Virus Interactions with Plants
Volume 13 - 2022 | https://doi.org/10.3389/fmicb.2022.1045796
This article is part of the Research Topic Fungal Wheat Diseases: Etiology, Breeding, and Integrated Management, Volume II View all 13 articles
With the increase of temperature in the winter wheat-growing regions in China, the high-temperature-resistant Blumeria graminis f. sp. tritici (Bgt) isolates developed in the fields. To clarify the key infection stages and the roles of heat shock protein (HSP) genes of high-temperature-resistant Bgt isolates defending high temperature, 3 high-temperature-resistant and 3 sensitive Bgt isolates were selected from 55 isolates after determination of temperature sensitivity. And then they were used to investigate the infection stages and the expression levels of HSP genes, including Bgthsp60, Bgthsp70, Bgthsp90, and Bgthsp104, at 18°C and 25°C. The formation frequency of abnormal appressoria and inhibition rate of haustoria formation of high-temperature-resistant isolates at 25°C were lower than those of high-temperature-sensitive isolates, while major axis of microcolonies of high-temperature-resistant isolates was higher than those of high-temperature-sensitive isolates at 25°C. The results indicated that haustoria formation and hyphal expansion were the key infection stages of defense against heat stress in high-temperature-resistant isolates. Further analyses of HSP genes found the expression levels of Bgthsp60 and Bgthsp70c were upregulated at 24 and 72 h post-inoculation in high-temperature-resistant isolates, while no significant difference was observed for Bgthsp90 and Bgthsp104 genes. Taken together, the basis of high-temperature-resistant Bgt isolates is associated with induced expression of Bgthsp60 and Bgthsp70c response to heat stress in haustoria formation and hyphal expansion stages.
Temperature is an important abiotic factor influencing several plant pathogens as various features, such as geographical range, growth rates in population, infection stages, and ability to spread, ultimately affects the prevalence of the disease (Coakley et al., 1999; Velásquez et al., 2018). For example, severity and frequency of wheat yellow rust caused by Puccinia striiformis f. sp. tritici (Pst) increased in association with increased winter temperatures and lower spring temperatures in the USA (Coakley, 1979). Prevalence of Pst was decreased with increased average annual temperatures from 1950 to 1995 (Luck et al., 2011). Increased severity of wheat spot blotch caused by Cochliobolus sativus was associated with increased average night-time temperatures in South Asia (Sharma et al., 2007). Needle blight of pine caused by Dothistroma septosporum was moving north with increasing temperature and precipitation in Canada (Woods et al., 2005). To survive temperature increasing, organisms, including plant pathogens, have developed the ability to adapt changing environment by constantly adjusting their phenotype on biological, ecological, and evolutionary processes (Knies et al., 2006; Knies and Kingsolver, 2010). For instance, high temperature affected conidia germination of Pst, P. recondita f. sp. tritici, Beauveria bassiana, and Leveillula taurica (de Vallavieille-Pope et al., 1995; Guzman-Plazola et al., 2003; Devi et al., 2005), appressoria formation in Colletotrichum gloeosporioides (Estrada et al., 2000), germ tube elongation of L. taurica (Guzman-Plazola et al., 2003), and haustoria formation in Oidium heveae, Podosphaera xanthii, and Golovinomyces orontii (Trecate et al., 2019; Cao et al., 2021). In molecular level, heat shock proteins (HSPs) resisted the effects of heat stress by protecting proteins from aggregation and degradation in many fungi (Tiwari et al., 2015). HSP genes in fungi played important roles when subjected to heat stress, such as HSP60 in Aspergillus fumigatus and A. terreus (Raggam et al., 2011), HSP70 in Trichoderma harzianum (Montero-Barrientos et al., 2008), HSP90 in Fusarium graminearum (Bui et al., 2016), and HSP104 in Saccharomyces cerevisiae (Parsell et al., 1994).
Wheat powdery mildew is a regionally epidemic disease, caused by the parasitic fungus Blumeria graminis f. sp. tritici (Bgt), which over-summer in cool, high-altitude wheat-growing areas in China. As the mean monthly atmospheric temperature of the major wheat-growing areas in China had increased from 1970 to 2012 by a mean 0.329°C per decade (Tang et al., 2017), the minimum altitude for over-summering of Bgt decreased during the disease survey (Li et al., 2013), suggesting that high-temperature-resistant isolates exist in the fields. Wan’s data proved this hypothesis, temperature sensitivity distribution of Bgt isolates in the fields in China was abnormal (Wan et al., 2010). However, the key infection stages of defense against heat stress in high-temperature-resistant Bgt isolates and the role of HSP genes in key infection stages remain unknown.
In this study, conidia germination, appressoria formation, haustoria formation, and hyphal expansion of Bgt isolates with different temperature sensitivity at 18°C and 25°C were investigated. And then expression levels of HSP genes were analyzed in these infection stages, including Bgthsp60, Bgthsp70, Bgthsp90, and Bgthsp104. The purposes of the present study were to clarify the key infection stages of Bgt isolates affected by high temperature and roles of HSP genes in response to heat stress. These results will help us to understanding the molecular basis of high thermal resistance mechanism in Bgt.
Bgt-infected wheat leaves were collected from fields in Beijing, Shaanxi, Henan, and Yunnan province/city in China. After isolation and purification as described previously (Xu et al., 2014), 55 isolates were used in this study (Table 1). These isolates were reproduced on seedlings of highly susceptible wheat cultivar ‘Jingshuang16’, which has no effective genes against Chinese Bgt isolates. Briefly, seeds were sown in a Φ 5 cm glass tube covered with 5 layers of gauze to prevent accidental contamination. Conidia of Bgt isolates were used to inoculate on seedlings at one-leaf stage and incubated in a growth chamber (Panasonic, Ehime-ken, Japan, temperature fluctuation range: ±0.5°C) with a 16-h-light/8-h-dark cycle at 18°C.
Temperature sensitivity was tested using seedling sensitivity assays. Briefly, conidia of Bgt isolates were used to inoculate on wheat seedlings and incubated in growth chamber at 18°C, 22°C, 24°C, 26°C, and 28°C, respectively, with 18°C as the reference incubation temperature. For each temperature, 15 wheat seedlings (one leaf seedling stage) were inoculated. Disease severity (percentage of diseased area to total leaf area) was recorded at 10 days post inoculation (dpi). Disease inhibition rate (DIR) was calculated by (1−average disease severity in treatment temperature/average disease severity at 18°C) × 100%. The linear regression equation was constructed with culture temperature (X) as dependent variables and DIR (Y) as independent variables as follows:
ET50 represented the temperature at which the DIR (Y) reached 50%.
To screen the high-temperature-sensitive and resistant Bgt isolates, ET50 and DIR at 26°C were used as two indictors. First, Bgt isolates with the ET50 less than 24°C and more than 25°C were classified as candidate high-temperature-sensitive and candidate high-temperature-resistant isolates, respectively. Then, the candidate isolates with high DIR at 26°C were considered high-temperature-sensitive isolates, and those with low DIR at 26°C were considered high-temperature-resistant isolates.
The temperature ranging from 15°C to 22°C is considered the optimum temperature for development of Bgt (Last, 1963), while Bgt is rarely grown at 26°C on each leaf segment. Therefore, 25°C, a sublethal high temperature was used as the heat stress treatment, and 18°C was used as the reference temperature in histological observation and HSPs expression analyses. For histological observation assay of Bgt during infection stages, one-leaf seedlings were cut into 3.5 cm-long segments. Ninety-six leaf segments were floated on a water agar amended with 60 μg/ml benzimidazole in 10 × 10 cm plates. Two plates were evenly inoculated with around 40 mg of conidia (collected and quantified to 100 μl using a 1.5 ml Eppendorf tube) of a single isolate using a settling tower. Plates were incubated at 18°C and 25°C, respectively. Conidia germination, appressoria formation (normal and abnormal appressoria formation), haustoria formation, and hyphal expansion were examined at 8, 24, 48, and 72 h post-inoculation (hpi). Glass slide was used to catch spores during inoculation to estimate the inoculation density.
To test conidia germination, about 100 Bgt conidia on each leaf segment were randomly selected and the number of conidia with primary germ tubes was counted at 8 hpi. For testing appressoria formation, the number of normal and abnormal appressoria was recorded for about 100 germinated conidia on each leaf segment at 24 hpi. In addition, the length of appressorial germ tube (AGT; 24 hpi) was measured using CellSens Dimension software. The formation frequency of haustoria was calculated by the percentage of the number of haustoria to the number of inoculated spores on each leaf segment (80 mm2 areas) at 48 hpi. Inhibition rate of haustoria formation was calculated according to the following formula:
The major axis of the microcolonies was presented by the average hyphal expansion width of 30 microcolonies on each leaf segment at 72 hpi using a microscope at 100× magnification. Disease severities of five leaf segments were measured at 10 dpi. Three independent repeats were performed for statistical analysis.
For histological observation assay of Bgt during infection stages, infected leaf segments by Bgt isolates were stained with Wheat germ agglutinin (WGA) conjugated to fluorescein isothiocyanate (Sigma-Aldrich, St. Louis, MO, USA) as described previously (Ayliffe et al., 2011). After decolorating in ethanol/acetic acid (1:1 v/v), infected leaf segments were cleared in saturated chloral hydrate until translucent, followed by soaking in 1 M KOH for 1 h and neutralized in 50 mM Tris–HCl (pH 7.5). These samples were stained with a 20 μg/ml solution of WGA, followed by rinsing with distilled water. Histological observation was conducted on fluorescence microscope Olympus BX61 (Olympus Corporation, Tokyo, Japan) with blue light excitation (450–480 nm).
To identify HSP genes of Bgt, amino acid sequences of HSP60, HSP70, HSP90, and HSP104 of Saccharomyces cerevisiae and Aspergillus nidulans were employed as queries to search the Bgt genome under the NCBI accession GCA_900519115.1 (Wicker et al., 2013). All the sequences were submitted to Conserved Domains Database (CDD) to confirm the conserved domains of HSP family in Bgt.1 WoLF PSORT was used to predict protein subcellular localization.2
To analyze gene expressions of Bgthsp60, Bgthsp70 (Bgthsp70a, Bgthsp70b, and Bgthsp70c), Bgthsp90, and Bgthsp104 (Bgthsp104a and Bgthsp104b), the detached leaf segments were inoculated with conidia and incubated at 18°C and 25°C, respectively, and then collected and snap frozen in liquid nitrogen at 0, 24, 48, and 72 hpi. Total RNA was extracted with Trizol reagent (Invitrogen, Camarillo, CA, United States). The first-strand cDNA was synthesized with FastKing One-Step RT-PCR Kit (TIANGEN, Beijing, China). Real-time PCR amplifications were conducted with TranStart Top Green qPCR SuperMix (TRANSGEN BIOTECH, Beijing, China) and performed on ABI 7500 real-time PCR system (Applied Biosystems Inc., Foster City, CA, United States). Relative expressions were calculated using the 2-△△CT method (Livak and Schmittgen, 2001) with β-tubulin as reference gene and 0 hpi of the one independent repeats of 13–10–3-2-2 as reference sample. The primers used for quantitative PCR were listed in Table 2. Three independent repeats were performed for statistical analysis.
Two-way analysis of variance (ANOVA) with SAS software version 9.4 (SAS Institute Inc., Cary, NC, USA) was used to assess the difference in conidia germination frequencies, formation frequencies of appressoria and abnormal appressoria, haustoria formation frequencies, lengths of AGT, major axis of the microcolonies, disease severity, and genes expression levels between two culture temperatures or high-temperature-sensitive and resistant isolates. One-way ANOVA was used to test the effect of temperature on inhibition rate of haustoria formation.
Temperature sensitivity tests showed that ET50 of the 55 Bgt isolates ranged from 22.20°C to 25.77°C, and mean ET50 was 24.57 ± 0.71°C (Table 3). Depending on the discrimination system, 11 isolates with ET50 less than 24°C were classified as candidates of high-temperature-sensitive isolates, and 18 isolates with ET50 greater than 25°C were classified as candidates of high-temperature-resistant isolates. The DIR at 26°C of those candidate high-temperature-sensitive isolates ranged from 51.54% to 98.78%, while those candidate high-temperature-resistant isolates ranged from 20.91% to 82.64% (Figure 1). Three isolates with more than 85% DIR at 26°C were selected as high-temperature-sensitive isolates, and three isolates with less than 60% DIR at 26°C were selected as high-temperature-resistant isolates (Table 3). And then, the six isolates were used in histological observation.
Figure 1. ET50 and disease inhibition rate (DIR) at 26°C of 11 high-temperature-sensitive and 18 high-temperature-resistant candidate isolates of Blumeria graminis f. sp. tritici. Line chart depicted the ET50, and bar chart depicted the DIR at 26°C.
For both high-temperature-sensitive and resistant isolates incubated at 18°C, conidia germination started at 30 min post inoculation, but most of the conidia completed germination at 8 hpi, and the average germination frequency reached about 76.46% (Supplementary Table S1). About 85.22% germinated conidia had formed appressoria at 24 hpi (Figure 2A; Supplementary Table S1), after that about 12.06% appressoria were deformed (Supplementary Table S1). The abnormal appressoria were usually multi-lobed or absence of AGT hooking leading to elongation of the AGT (Figures 2B,C). Totally, around 8.46% appressoria developed to form primary haustoria (Figure 2D; Supplementary Table S1) and developed into mature haustoria with a finger-like structure at 48 hpi (Figure 2E). Then, the secondary hyphae developed from the appressoria and expanded to form microcolonies at 72 hpi (Figure 2F).
Figure 2. Histological observation on key infection stages of B. graminis f. sp. tritici isolates at 18°C. (A) Appressorium, (B,C) Abnormal appressoria, (D) Primary haustoria, (E) Finger-like matured haustoria, (F) Microcolony. AP = appressorium; AAP = abnormal appressorium; PH = primary haustorium; H = haustorium; MC = microcolony. A-E bars=60µm, F bar=130µm.
There were no significant differences in conidia germination frequencies and appressoria formation frequencies among high-temperature-sensitive and resistant Bgt isolates at 25°C (Figures 3A,B). However, the formation frequencies of abnormal appressoria of high-temperature-resistant isolates were significantly lower than those of two high-temperature-sensitive isolates at 25°C (Figure 3C). In addition, there was no significant effect of high temperature on the length of AGT (Figure 3D). Furthermore, for all the high-temperature-sensitive and resistant Bgt isolates, about 11.30% conidia developed haustoria at 18°C, while only around 5.62% conidia developed haustoria at 25°C (Figure 3E). And the inhibition rates of haustoria formation at 25°C in high-temperature-resistant isolates (except 13–1–4-1-1-1) were significantly reduced compared with the high-temperature-sensitive isolates (Figure 3F). Moreover, major axis of microcolonies of high-temperature-resistant isolates at 25°C were higher in comparison with high-temperature-sensitive isolates (Figure 3G), which suggests that the hyphal extension of high-temperature-resistant isolates is much more easily at high temperature. Notably, disease severities of all isolates were significantly decreased at 25°C in comparison with those at 18°C; furthermore, disease severities of high-temperature-sensitive isolates were decreased more than those of high-temperature-resistant isolates at 25°C (Figure 3H).
Figure 3. Differences in infection stages of high-temperature-sensitive and resistant isolates of B. graminis f. sp. tritici at 18°C and 25°C. (A) Germination frequencies of conidia; (B) Formation frequencies of appressoria; (C) Formation frequencies of abnormal appressoria; (D) Lengths of appressorial germ tube (AGT); (E) Formation frequencies of haustoria; (F) Inhibition rate of haustoria formation; (G) Major axis of microcolonies; (H) Disease severity. The inhibition rate of haustoria formation was analyzed by one-way analysis of variance (ANOVA), while other data were analyzed using two-way ANOVA with Duncan’s multiple range test. The error bar showed standard error of three independent repeats. The different letters above the error bars indicated significant differences (p ≤ 0.05).
Totally, 1 hsp60 gene, 3 homologous hsp70 genes (Bgthsp70a, Bgthsp70b, and Bgthsp70c), 1 hsp90 gene, and 2 homologous hsp104 genes (Bgthsp104a and Bgthsp104b) were identified in the Bgt genome. The conserved domains of HSPs were confirmed using CDD database. There is a GroEL domain in Bgthsp60 gene, a DnaK domain in 3 homologous Bgthsp70 genes, a HSP83 domain in Bgthsp90 gene, and a ClpB domain in both 2 homologous Bgthsp104 genes. Subcellular localization prediction by WoLF PSORT showed that HSP60 was localized in mitochondria, and HSP70a, HSP70b, HSP90, HSP104a, and HSP104b were localized in cytoplasm, while HSP70c was localized in endoplasmic reticulum (ER; Table 4).
Two high-temperature-sensitive isolates, 13–14–7-2-2 and 13–11–4-2-2-1, and two high-temperature-resistant isolates, 13–10–3-2-2 and 13–14–8-2-2, were used to analyze the HSP genes expression levels at 24, 48, and 72 hpi at 18°C and 25°C. At 24 hpi, the expression levels of Bgthsp60, Bgthsp70a, Bgthsp70c, and Bgthsp104a in high-temperature-resistant Bgt isolates were increased at 25°C, while the expression levels of Bgthsp70a, Bgthsp70c, and Bgthsp104a were decreased in high-temperature-sensitive isolates at 25°C in comparison with those at 18°C (Figure 4A). At 48 hpi, expression levels of Bgthsp60 in all Bgt isolates were increased at 25°C in comparison with those at 18°C (Figure 4B). At 72 hpi, expression levels of Bgthsp60, Bgthsp70c, Bgthsp90, Bgthsp104a, and Bgthsp104b in all Bgt isolates were increased at 25°C in comparison with those at 18°C (Figure 4C).
Figure 4. Expression levels of Bgthsp60, Bgthsp70, Bgthsp90 and Bgthsp104 under different temperature conditions at (A) 24 h post-inoculation (hpi), (B) 48 hpi, (C) 72 hpi. β-tubulin was used as reference gene and 0 hpi of the one independent repeat of 13–10–3-2-2 as reference sample. The error bar showed standard error of three independent repeats. Data were analyzed by two-way ANOVA with Duncan’s multiple range test. The different letters above the error bars indicated significant differences (p ≤ 0.05).
At 25°C, the expression levels of Bgthsp60 were upregulation in high-temperature-resistant isolates at 48 and 72 hpi, while no difference or down-regulation in high-temperature-sensitive isolates was observed (Figure 5A). At 25°C, the expression levels of Bgthsp70c in high-temperature-resistant isolates were greatly increased at 24 and 72 hpi and higher than those of high-temperature-sensitive isolates at 24 hpi (Figure 5B).
Figure 5. Time course of gene expression level of Bgthsp60 (A) and Bgthsp70c (B) in high-temperature-sensitive and resistant isolates at 25°C. Infected leaf segments by B. graminis f. sp. tritici isolates were collected at 0, 24, 48, and 72 h post-inoculation (hpi). β-tubulin was used as reference gene and 0 hpi of the one independent repeat of 13–10–3-2-2 as reference sample. The error bar showed standard error of three independent repeats.
To survive climatic warming, plant pathogens evolved to adapt changing temperature by adjusting the biology, ecology, and evolutionary processes. Although the life cycle of Pst will be limited by increasing temperatures, new isolates of Pst better adapted to high temperatures than the isolates collected before 2000 that dominated the pathogen population in south central USA (Milus et al., 2006, 2009). High-temperature-resistant Bgt isolates had existed in the fields in 2008 and accounted for 17.7% (20/113; Wan et al., 2010). Furthermore, the parasitic fitness of high-temperature-resistant isolates was higher than that of high-temperature-sensitive isolates under higher temperatures (Wan et al., 2012). In this research, three high-temperature-resistant and sensitive Bgt isolates were selected in 55 Bgt isolates to clarify the high parasitic fitness of high-temperature-resistant isolates at cytological and molecular levels under higher temperatures.
Temperature is an important abiotic factor having a critical influence, positive, or negative, on infection stages of plant pathogens, such as conidia germination, appressoria formation, germ tube elongation, and haustoria formation in Pst, Puccinia recondita f. sp. tritici, Colletotrichum acutatum, Leveillula taurica, Oidium neolycopersici, O. heveae, Podosphaera xanthii, and Golovinomyces orontii (Guzman-Plazola et al., 2003; Leandro et al., 2003; Mieslerová and Lebeda, 2010; Trecate et al., 2019; Cao et al., 2021). This research showed that conidia germination of two types isolates was not affected at 25°C, which indicates that 25°C was not unfavorable temperature for conidia germination of Bgt. This is consistent with previous reports that temperatures between 15°C and 25°C were favorable for conidia germination of B. graminis f. sp. hordei on plain agar substrate (Yarwood et al., 1954). In addition, the optimum temperatures of appressorial formation of C. acutatum ranged from 17.6°C to 26.5°C, while this formation was inhibited until 30°C (Leandro et al., 2003). In this research, there were no significant differences in the formation frequencies of appressoria in two types isolates between 18°C and 25°C. This indicates that 25°C is favorable temperature as 18°C for appressorial formation of Bgt. These results also suggest that 25°C, a sublethal high temperature, has little effect on the precede penetration of Bgt.
Although the formation frequency of abnormal appressoria was increased at 25°C in comparison with at 18°C for high-temperature-sensitive and resistant isolates, the abnormal appressoria formation of high-temperature-resistant isolates was lower than that of high-temperature-sensitive isolates at 25°C, indicating that the high-temperature-resistant isolates were better adapted to high temperature. Previous research showed that the abnormal appressoria were caused by the failure of appressoria penetrating host cells (Nonomura et al., 2010), and finally resulted in the failure of haustoria formation. Although high temperature has little effect on the quantity of appressoria formation, it significantly increased abnormal morphology of appressoria, such as absence of AGT hooking and multi-lobed appressoria, followed by dramatically decreased haustoria formation frequency. A previous report also found that temperature significantly affected haustoria formation of O. heveae (Cao et al., 2021). In addition, the inhibition rate of haustoria formation in high-temperature-resistant Bgt isolates was lower than the high-temperature-sensitive isolates, suggesting that the haustoria formation of high-temperature-sensitive isolates was more severely inhibited by 25°C than high-temperature-resistant isolates. Moreover, the hyphal extension of high-temperature-resistant isolates was much more easily at 25°C than that of high-temperature-sensitive isolates. These results suggest that haustoria formation and hyphal expansion are key infection stages for high-temperature-resistant isolates defending heat stress.
In this study, 7 HSPs were identified in Bgt, of which Bgthsp60 and Bgthsp70c in high-temperature-resistant isolates played important roles at 25°C in the stage of haustoria formation and hyphal expansion.
Bgthsp60 was localized in mitochondria and significantly upregulated only in high-temperature-resistant Bgt isolates at 25°C compared with 18°C in the stage of hyphal expansion (Figure 4C). This suggests that upregulation of Bgthsp60 was associated with hyphal expansion to defend heat stress in high-temperature-resistant Bgt isolates. Similar results were also obtained in Aspergillus fumigatus and A. terreus, the expression levels were upregulation 5.9-fold and 6.7-fold at high temperature of 40°C compared with that at regular temperature of 25°C (Raggam et al., 2011). HSP60 was required for maintaining mitochondrial protein homeostasis together with the co-chaperonin Hsp10 by preventing protein aggregation, and mediating folding and refolding under heat stress (Martin et al., 1992; Caruso Bavisotto et al., 2020). In addition, heat stress usually increased the number of interaction proteins with HSP60, which were related to metabolism demanded to proliferate in B. graminis (Both et al., 2005), including amino acid and protein metabolism and carbohydrate metabolism (Guimarães et al., 2011). These results suggest that Bgthsp60 were induced by high temperature, and play a part in the process of defending high temperatures, but it remains to verify how HSP60 work in high-temperature-resistant Bgt isolates.
Bgthsp70c was identified as an ER lumenal HSP70, and upregulated in all isolates at 25°C compared with 18°C in the stage of haustoria formation and hyphal expansion. Especially, in the stage of haustoria formation, the levels of Bgthsp70c expression of high-temperature-resistant isolates were more than that of high-temperature-sensitive isolates at 25°C (Figure 5B). The result shows that Bgthsp70c of high-temperature-resistant isolates could contribute to tolerance to heat stress in the stage of haustoria formation. ER HSP70 proteins as molecular chaperones played key roles in protein transport into the ER and proper protein folding in the ER lumen, such as Kar2p and Lhs1p in fungi (Brodsky et al., 1995; Craven et al., 1996). In addition, Lhs1 was necessary for proper growth, conidiation, and pathogenicity in fungi, such as Magnaporthe oryzae, Beauveria bassiana, and Fusarium pseudograminearum (Yi et al., 2009; Chen et al., 2019; Wang et al., 2020). Particularly, Lhs1 properly processed of secreted proteins, including effectors, was requisite for successful disease development in F. pseudograminearum and M. oryzae (Yi et al., 2009; Chen et al., 2019). Binding protein (Bip) is also a member of the ER HSP70 family (Denecke et al., 1991). When plants were subjected to heat stress, BiP genes were upregulated via the unfolded protein response pathway, in Arabidopsis (Deng et al., 2011), and pepper (Capsicum annuum L.; Wang et al., 2017). The silencing of CaBiP1 decreased the tolerance of pepper to heat stress. Conversely, overexpression of CaBiP1 increased the tolerance of Arabidopsis (Wang et al., 2017). Therefore, it is supposed that overexpressed Bgthsp70c reduced the number of misfolded proteins in the stage of haustoria formation in high-temperature-resistant isolates at high temperature.
HSP90 in F. graminearum and HSP104 in Saccharomyces cerevisiae were thermotolerance factors. Deletion of hsp90 stopped growing after heat shock (48°C for 30 min), whereas the wild-type isolates showed slightly delayed growth in F. graminearum (Bui et al., 2016). The ability of S. cerevisiae to withstand high temperatures was reduced when hsp104 was knockout (Parsell et al., 1994). However, the expression levels of hsp90, hsp104a, and hsp104b showed no difference in high-temperature-resistant Bgt isolates at 25°C compared with that at 18°C, indicating that these genes could not be thermotolerance factors in Bgt.
In this research, it is confirmed that haustoria formation and hyphal expansion were key infection stages for high-temperature-resistant isolates to defend heat stress. In addition, upregulation of Bgthsp60 and Bgthsp70c is associated with heat stress in high-temperature-resistant isolates in these stages. It is supposed that Bgthsp60 and Bgthsp70c of high-temperature-resistant Bgt isolates could play a role in the process of defending high temperatures. However, the high thermal resistance mechanism of Bgt in haustoria formation and hyphae needs a deeper understanding.
The datasets presented in this study can be found in online repositories. The names of the repository/repositories and accession number(s) can be found in the article/Supplementary material.
MZ, WL, and JF contributed to conception and design of the experiments. MZ and CZ performed the experiments. MZ, AW, WL, FX, ZM, and YZ analyzed the data. MZ wrote the manuscript. All authors contributed to the article and approved the submitted version.
This research was financially supported by the National Natural Science Foundation of China (31972226).
The authors thank D. W. HU (Zhejiang University, Hangzhou, China) for his help in the histological study of Blumeria graminis f. sp. tritici and Y. LUO (University of California-Davis, California, USA) and F. P. CHEN (Fujian Agriculture and Forestry University, Fujian, China) for their helps to advice on revision of manuscript.
The authors declare that the research was conducted in the absence of any commercial or financial relationships that could be construed as a potential conflict of interest.
All claims expressed in this article are solely those of the authors and do not necessarily represent those of their affiliated organizations, or those of the publisher, the editors and the reviewers. Any product that may be evaluated in this article, or claim that may be made by its manufacturer, is not guaranteed or endorsed by the publisher.
The Supplementary material for this article can be found online at: https://www.frontiersin.org/articles/10.3389/fmicb.2022.1045796/full#supplementary-material
Ayliffe, M., Devilla, R., Mago, R., White, R., Talbot, M., Pryor, A., et al. (2011). Nonhost resistance of rice to rust pathogens. Mol. Plant-Microbe Interact. 24, 1143–1155. doi: 10.1094/MPMI-04-11-0100
Both, M., Csukai, M., Stumpf, M. P., and Spanu, P. D. (2005). Gene expression profiles of Blumeria graminis indicate dynamic changes to primary metabolism during development of an obligate biotrophic pathogen. Plant Cell 17, 2107–2122. doi: 10.1105/tpc.105.032631
Brodsky, J. L., Goeckeler, J., and Schekman, R. (1995). BiP and Sec63p are required for both co-and posttranslational protein translocation into the yeast endoplasmic reticulum. Proc. Natl. Acad. Sci. U. S. A. 92, 9643–9646. doi: 10.1073/pnas.92.21.9643
Bui, D. C., Lee, Y., Lim, J. Y., Fu, M., Kim, J. C., Choi, G. J., et al. (2016). Heat shock protein 90 is required for sexual and asexual development, virulence, and heat shock response in Fusarium graminearum. Sci. Rep. 6, 1–11. doi: 10.1038/srep28154
Cao, X. R., Xu, X. M., Che, H. Y., West, J. S., and Luo, D. Q. (2021). Effects of temperature and leaf age on conidial germination and disease development of powdery mildew on rubber tree. Plant Pathol. 70, 484–491. doi: 10.1111/ppa.13281
Caruso Bavisotto, C., Alberti, G., Vitale, A. M., Paladino, L., Campanella, C., Rappa, F., et al. (2020). Hsp60 post-translational modifications: functional and pathological consequences. Front. Mol. Biosci. 7:95. doi: 10.3389/fmolb.2020.00095
Chen, L., Geng, X., Ma, Y., Zhao, J., Chen, W., Xing, X., et al. (2019). The ER lumenal Hsp70 protein FpLhs1 is important for conidiation and plant infection in Fusarium pseudograminearum. Front. Microbiol. 10:1401. doi: 10.3389/fmicb.2019.01401
Coakley, S. M. (1979). Climate variability in the Pacific northwest and its effect on stripe rust disease of winter wheat. Clim. Chang. 2, 33–51. doi: 10.1007/bf00138225
Coakley, S. M., Scherm, H., and Chakraborty, S. (1999). Climate change and plant disease management. Annu. Rev. Phytopathol. 37, 399–426. doi: 10.1146/annurev.phyto.37.1.399
Craven, R. A., Egerton, M., and Stirling, C. J. (1996). A novel Hsp70 of the yeast ER lumen is required for the efficient translocation of a number of protein precursors. EMBO J. 15, 2640–2650. doi: 10.1002/j.1460-2075.1996.tb00624.x
de Vallavieille-Pope, C., Huber, L., Leconte, M., and Goyeau, H. (1995). Comparative effects of temperature and interrupted wet periods on germination, penetration, and infection of Puccinia recondita f. sp. tritici and P. striiformis on wheat seedlings. Phytopathology 85, 409–415. doi: 10.1094/phyto-85-409
Denecke, J., Goldman, M., Demolder, J., Seurinck, J., and Botterman, J. (1991). The tobacco luminal binding protein is encoded by a multigene family. Plant Cell 3, 1025–1035. doi: 10.1105/tpc.3.9.1025
Deng, Y., Humbert, S., Liu, J. X., Srivastava, R., Rothstein, S. J., and Howell, S. H. (2011). Heat induces the splicing by IRE1 of a mRNA encoding a transcription factor involved in the unfolded protein response in Arabidopsis. Proc. Natl. Acad. Sci. U. S. A. 108, 7247–7252. doi: 10.1073/pnas.1102117108
Devi, K. U., Sridevi, V., Mohan, C. M., and Padmavathi, J. (2005). Effect of high temperature and water stress on in vitro germination and growth in isolates of the entomopathogenic fungus Beauveria bassiana (Bals.) Vuillemin. J. Invertebr. Pathol. 88, 181–189. doi: 10.1016/j.jip.2005.02.001
Estrada, A., Dodd, J. C., and Jeffries, P. (2000). Effect of humidity and temperature on conidial germination and appressorium development of two Philippine isolates of the mango anthracnose pathogen Colletotrichum gloeosporioides. Plant Pathol. 49, 608–618. doi: 10.1046/j.1365-3059.2000.00492.x
Guimarães, A. J., Nakayasu, E. S., Sobreira, T. J., Cordero, R. J., Nimrichter, L., Almeida, I. C., et al. (2011). Histoplasma capsulatum heat-shock 60 orchestrates the adaptation of the fungus to temperature stress. PLoS One 6:e14660. doi: 10.1371/journal.pone.0014660
Guzman-Plazola, R. A., Davis, R. M., and Marois, J. J. (2003). Effects of relative humidity and high temperature on spore germination and development of tomato powdery mildew (Leveillula taurica). Crop Prot. 22, 1157–1168. doi: 10.1016/s0261-2194(03)00157-1
Knies, J. L., Izem, R., Supler, K. L., Kingsolver, J. G., and Burch, C. L. (2006). The genetic basis of thermal reaction norm evolution in lab and natural phage populations. PLoS Biol. 4:e201. doi: 10.1371/journal.pbio.0040201
Knies, J. L., and Kingsolver, J. G. (2010). Erroneous Arrhenius: modified Arrhenius model best explains the temperature dependence of ectotherm fitness. Am. Nat. 176, 227–233. doi: 10.1086/653662
Last, F. (1963). Effect of temperature on cereal powdery mildews. Plant Pathol. 12, 132–133. doi: 10.1111/j.1365-3059.1963.tb00232.x
Leandro, L., Gleason, M., Nutter, F. Jr., Wegulo, S., and Dixon, P. (2003). Influence of temperature and wetness duration on conidia and appressoria of Colletotrichum acutatum on symptomless strawberry leaves. Phytopathology 93, 513–520. doi: 10.1094/phyto.2003.93.4.513
Li, B., Cao, X., Chen, L., Zhou, Y., Duan, X., Luo, Y., et al. (2013). Application of geographic information systems to identify the oversummering regions of Blumeria graminis f. sp. tritici in China. Plant Dis. 97, 1168–1174. doi: 10.1094/pdis-10-12-0957-re
Livak, K. J., and Schmittgen, T. D. (2001). Analysis of relative gene expression data using real-time quantitative PCR and the 2− ΔΔCT method. Methods 25, 402–408. doi: 10.1006/meth.2001.1262
Luck, J., Spackman, M., Freeman, A., Tre Bicki, P., Griffiths, W., Finlay, K., et al. (2011). Climate change and diseases of food crops. Plant Pathol. 60, 113–121. doi: 10.1111/j.1365-3059.2010.02414.x
Martin, J., Horwich, A. L., and Hartl, F. U. (1992). Prevention of protein denaturation under heat stress by the chaperonin Hsp60. Science 258, 995–998. doi: 10.1126/science.1359644
Mieslerová, B., and Lebeda, A. (2010). Influence of temperature and light conditions on germination, growth and conidiation of Oidium neolycopersici. J. Phytopathol. 158, 616–627. doi: 10.1111/j.1439-0434.2009.01663.x
Milus, E. A., Kristensen, K., and Hovmøller, M. S. (2009). Evidence for increased aggressiveness in a recent widespread strain of Puccinia striiformis f. sp. tritici causing stripe rust of wheat. Phytopathology 99, 89–94. doi: 10.1094/phyto-99-1-0089
Milus, E., Seyran, E., and McNew, R. (2006). Aggressiveness of Puccinia striiformis f. sp. tritici isolates in the south-Central United States. Plant Dis. 90, 847–852. doi: 10.1094/pd-90-0847
Montero-Barrientos, M., Hermosa, R., Nicolás, C., Cardoza, R. E., Gutiérrez, S., and Monte, E. (2008). Overexpression of a Trichoderma HSP70 gene increases fungal resistance to heat and other abiotic stresses. Fungal Genet. Biol. 45, 1506–1513. doi: 10.1016/j.fgb.2008.09.003
Nonomura, T., Nishitomi, A., Matsuda, Y., Soma, C., Xu, L., Kakutani, K., et al. (2010). Polymorphic change of appressoria by the tomato powdery mildew Oidium neolycopersici on host tomato leaves reflects multiple unsuccessful penetration attempts. Fungal Biol. 114, 917–928. doi: 10.1016/j.funbio.2010.08.008
Parsell, D. A., Kowal, A. S., Singer, M. A., and Lindquist, S. (1994). Protein disaggregation mediated by heat-shock protein Hspl04. Nature 372, 475–478. doi: 10.1038/372475a0
Raggam, R. B., Salzer, H. J., Marth, E., Heiling, B., Paulitsch, A. H., and Buzina, W. (2011). Molecular detection and characterisation of fungal heat shock protein 60. Mycoses 54, e394–e399. doi: 10.1111/j.1439-0507.2010.01933.x
Sharma, R., Duveiller, E., and Ortiz-Ferrara, G. (2007). Progress and challenge towards reducing wheat spot blotch threat in the eastern Gangetic Plains of South Asia: is climate change already taking its toll? Field Crop Res. 103, 109–118. doi: 10.1016/j.fcr.2007.05.004
Tang, X., Cao, X., Xu, X., Jiang, Y., Luo, Y., Ma, Z., et al. (2017). Effects of climate change on epidemics of powdery mildew in winter wheat in China. Plant Dis. 101, 1753–1760. doi: 10.1094/pdis-02-17-0168-re
Tiwari, S., Thakur, R., and Shankar, J. (2015). Role of heat-shock proteins in cellular function and in the biology of fungi. Biotechnol. Res. Int. 2015:132635, 1–11. doi: 10.1155/2015/132635
Trecate, L., Sedláková, B., Mieslerová, B., Manstretta, V., Rossi, V., and Lebeda, A. (2019). Effect of temperature on infection and development of powdery mildew on cucumber. Plant Pathol. 68, 1165–1178. doi: 10.1111/ppa.13038
Velásquez, A. C., Castroverde, C. D. M., and He, S. Y. (2018). Plant–pathogen warfare under changing climate conditions. Curr. Biol. 28, R619–R634. doi: 10.1016/j.cub.2018.03.054
Wan, Q., Ding, K., Duan, X., and Zhou, Y. (2010). Sensitivity of population of Blumeria graminis f. sp. tritici isolates to temperature in 2008. Acta Pharmacol. Sin. 40, 106–109. doi: 10.13926j.cnki.apps.2010.01.008
Wan, Q., Ding, K., Zhou, Y., Duan, X., and Zou, Y. (2012). Parasitic fitness of Blumeria graminis f. sp. tritici isolates with different sensitivity to temperature. Acta Pharmacol. Sin. 42, 186–194. doi: 10.13926/j.cnki.apps.2012.02.011
Wang, J., Chen, J., Hu, Y., Ying, S., and Feng, M. (2020). Roles of six Hsp70 genes in virulence, cell wall integrity, antioxidant activity and multiple stress tolerance of Beauveria bassiana. Fungal Genet. Biol. 144:103437. doi: 10.1016/j.fgb.2020.103437
Wang, H., Niu, H., Zhai, Y., and Lu, M. (2017). Characterization of BiP genes from pepper (Capsicum annuum L.) and the role of CaBiP1 in response to endoplasmic reticulum and multiple abiotic stresses. Front. Plant Sci. 8:1122. doi: 10.3389/fpls.2017.01122
Wicker, T., Oberhaensli, S., Parlange, F., Buchmann, J. P., Shatalina, M., Roffler, S., et al. (2013). The wheat powdery mildew genome shows the unique evolution of an obligate biotroph. Nat. Genet. 45, 1092–1096. doi: 10.1038/ng.2704
Woods, A., Coates, K. D., and Hamann, A. (2005). Is an unprecedented Dothistroma needle blight epidemic related to climate change? Bioscience 55, 761–769. doi: 10.1641/0006-3568(2005)055[0761:iaudnb]2.0.co;2
Xu, Z., Duan, X. Y., Zhou, Y. L., Guo, Q. Y., Yao, Q., and Cao, S. Q. (2014). Population genetic analysis of Blumeria graminis f. sp. tritici in Qinghai Province, China. J Integr. Agric. 13, 1952–1961. doi: 10.1016/s2095-3119(13)60591-2
Yarwood, C., Sidky, S., Cohen, M., and Santilli, V. (1954). Temperature relations of powdery mildews. Hilgardia 22, 603–622. doi: 10.3733/hilg.v22n17p603
Keywords: Blumeria graminis f. sp. tritici, high-temperature-resistant isolate, infection stage, histological observation, expression level, heat shock protein
Citation: Zhang M, Wang A, Zhang C, Xu F, Liu W, Fan J, Ma Z and Zhou Y (2022) Key infection stages defending heat stress in high-temperature-resistant Blumeria graminis f. sp. tritici isolates. Front. Microbiol. 13:1045796. doi: 10.3389/fmicb.2022.1045796
Received: 16 September 2022; Accepted: 25 October 2022;
Published: 11 November 2022.
Edited by:
Paul Christiaan Struik, Wageningen University and Research, NetherlandsReviewed by:
Antonin Dreiseitl, Agricultural Research Institute Kromeriz, CzechiaCopyright © 2022 Zhang, Wang, Zhang, Xu, Liu, Fan, Ma and Zhou. This is an open-access article distributed under the terms of the Creative Commons Attribution License (CC BY). The use, distribution or reproduction in other forums is permitted, provided the original author(s) and the copyright owner(s) are credited and that the original publication in this journal is cited, in accordance with accepted academic practice. No use, distribution or reproduction is permitted which does not comply with these terms.
*Correspondence: Wei Liu, d2xpdXNkYXVAMTYzLmNvbQ==; Jieru Fan, ZmFuamllcnVAY2Fhcy5jbg==
Disclaimer: All claims expressed in this article are solely those of the authors and do not necessarily represent those of their affiliated organizations, or those of the publisher, the editors and the reviewers. Any product that may be evaluated in this article or claim that may be made by its manufacturer is not guaranteed or endorsed by the publisher.
Research integrity at Frontiers
Learn more about the work of our research integrity team to safeguard the quality of each article we publish.