- 1Laboratory of Marine Environmental Microbiology, Division of Applied Biosciences, Graduate School of Agriculture, Kyoto University, Kyoto, Japan
- 2Institute for Extra-cutting-edge Science and Technology Avant-garde Research (X-star), Japan Agency for Marine-Earth Science and Technology (JAMSTEC), Yokosuka, Japan
- 3General Affairs Department, Japan Agency for Marine-Earth Science and Technology (JAMSTEC), Yokosuka, Japan
- 4Department of Marine and Earth Sciences, Marine Works Japan Ltd., Yokosuka, Japan
- 5Section for Exploration of Life in Extreme Environments, Exploratory Research Center on Life and Living Systems (ExCELLS), National Institute of Natural Sciences, Okazaki, Japan
In deep-sea hydrothermal environments, inorganic sulfur compounds are important energy substrates for sulfur-oxidizing, -reducing, and -disproportionating microorganisms. Among these, sulfur-disproportionating bacteria have been poorly understood in terms of ecophysiology and phylogenetic diversity. Here, we isolated and characterized a novel mesophilic, strictly chemolithoautotrophic, diazotrophic sulfur-disproportionating bacterium, designated strain GF1T, from a deep-sea hydrothermal vent chimney at the Suiyo Seamount in the Izu-Bonin Arc, Japan. Strain GF1T disproportionated elemental sulfur, thiosulfate, and tetrathionate in the presence of ferrihydrite. The isolate also grew by respiratory hydrogen oxidation coupled to sulfate reduction. Phylogenetic and physiological analyses support that strain GF1T represents the type strain of a new genus and species in the family Desulfobulbaceae, for which the name Desulfolithobacter dissulfuricans gen. nov. sp. nov. is proposed. Proteomic analysis revealed that proteins related to tetrathionate reductase were specifically and abundantly produced when grown via thiosulfate disproportionation. In addition, several proteins possibly involved in thiosulfate disproportionation, including those encoded by the YTD gene cluster, were also found. The overall findings pointed to a possible diversity of sulfur-disproportionating bacteria in hydrothermal systems and provided a refined picture of microbial sulfur disproportionation.
Introduction
Deep-sea hydrothermal environments are extreme habitats characterized by dynamic physicochemical conditions formed by the mixing of reducing hydrothermal fluid and oxidizing seawater (Kelley et al., 2002), and host a diversity of microorganisms that use chemical energy obtained from inorganic redox substances (Nakamura and Takai, 2014; Dick, 2019; Zeng et al., 2021). Abundant inorganic sulfur compounds, in particular, serve as both electron donors and acceptors for various microbial populations such as sulfur-oxidizers and -reducers (Elsgaard et al., 1995; Nakagawa et al., 2004; Nakagawa and Takai, 2008; Sievert et al., 2008). In addition, the occurrence of sulfur-disproportionating bacteria has been recently recognized in deep-sea hydrothermal systems (Slobodkin et al., 2012, 2013; Slobodkina et al., 2017). Sulfur disproportionation is a microbial dissimilatory metabolism in which a single intermediately oxidized inorganic sulfur compound, i.e., elemental sulfur (S0; Equation 1), sulfite, thiosulfate (Equation 2), or tetrathionate (Equation 3), is simultaneously reduced and oxidized, resulting in the production of sulfide and sulfate (Bak and Cypionka, 1987; Bak and Pfennig, 1987). This process significantly contributes to the global biogeochemical sulfur cycle (Fossing and Jørgensen, 1990; Jørgensen, 1990).
Sulfur disproportionation was firstly discovered in Desulfovibrio sulfodismutans isolated from anoxic sludge (Bak and Cypionka, 1987; Bak and Pfennig, 1987). To date, sulfur-disproportionating bacteria have been found in limited members of the phyla Firmicutes, Thermodesulfobacteria, Nitrospirae, and Proteobacteria (the classes Deltaproteobacteria and Gammaproteobacteria in particular; Finster, 2008; Slobodkin and Slobodkina, 2019; Umezawa et al., 2021). Many, but not all, sulfur-disproportionating bacteria are also capable of sulfate reduction as an alternative energy metabolism (Slobodkin and Slobodkina, 2019). Generally, their growth rates and yields under sulfur disproportionating conditions are much lower than those under sulfate-reducing conditions. Therefore, the ability of sulfur disproportionation is not thoroughly evaluated for previously characterized sulfate-reducing prokaryotes, and thus their diversity may be significantly underestimated.
Enzymes and intermediates involved in sulfur disproportionation are only partially understood. In deltaproteobacterial sulfur-disproportionating bacteria with an ability of sulfate reduction, sulfate-adenylyltransferase (Sat), adenylyl-sulfate reductase (Apr), and dissimilatory sulfite reductase (Dsr) likely play major roles in both sulfur disproportionation and sulfate reduction (Finster, 2008; Slobodkin and Slobodkina, 2019). Exceptionally, in S0-disproportionating Desulfurella amilsii, rhodanese-like sulfurtransferases have been hypothesized to play a key role instead of Sat, Apr, or Dsr (Florentino et al., 2019). In the first step of thiosulfate disproportionation by Desulfovibrio sulfodismutans and Desulfocapsa sulfexigens, thiosulfate reductase has been suggested to function in the cleavage of thiosulfate into sulfite and sulfide (Krämer and Cypionka, 1989; Frederiksen and Finster, 2003). To get a complete picture of the mechanism of sulfur disproportionation, it is important to know how commonly these known enzymes are used in diverse sulfur-disproportionating bacteria. In addition, the identification of unknown proteins is essential to fill in the missing pieces in this process.
Here, we report the isolation and characterization of the first mesophilic, chemolithoautotrophic, diazotrophic, and sulfur-disproportionating deltaproteobacterium from a deep-sea hydrothermal vent. We also aim to identify proteins involved in thiosulfate disproportionation pathway by a comparative proteomic analysis. This study represents the first proteomic study of sulfur-disproportionating Desulfobulbaceae species.
Materials and methods
Sample collection, enrichment, purification, and phylogenetic analyses
Sample collection and subsampling were performed as described previously (Hashimoto et al., 2021). Briefly, the chimney structure was obtained at the Suiyo Seamount, at a depth of 1,383 m (Supplementary Figure 1). The sample was used to inoculate MMJFE medium, which contained 1 g NaHCO3 and 20 mM ferrihydrite [prepared as described in Kostka and Nealson (1998)] per liter of modified MJ synthetic seawater under H2/CO2 (80:20; 300 kPa; Nakagawa and Takai, 2006). After 3 days of incubation at 35°C, the medium became turbid, and the color of ferrihydrite changed to black (probably due to iron sulfide). The dilution-to-extinction technique was used to obtain a pure culture (Baross and Deming, 1995). The purity was confirmed by microscopic observation, by repeated partial sequencing of the 16S rRNA gene, and by genome sequencing. The isolate was designated as strain GF1T (=JCM 34117T = DSM 111414T) and routinely cultured with MMJFE medium at 35°C unless otherwise noted.
The extraction of genomic DNA and determination and alignment of the sequence of the PCR product (1,490 bp) were performed as described previously (Hashimoto et al., 2021). A phylogenetic tree was constructed as mentioned in (Nagata et al., 2017). Briefly, the sequence of strain GF1T was aligned with a subset of 16S rRNA gene sequences, and only unambiguously aligned nucleotide positions (1,214 bases) were used for phylogenetic analyses.
Growth observation and chemotaxonomic analysis
Cells were observed using a light microscope (BX53, Olympus, Tokyo, Japan). Electron micrographs were captured using a transmission electron microscope (Tecnai G2 20; FEI, Hillsboro, OR) as described previously (Hashimoto et al., 2021).
The growth of the novel isolate was measured by direct cell counting after staining with 4′,6-diamidino-2-phenylindole (DAPI; Porter and Feig, 1980). Effects of NaCl concentration (15–45 g L−1) and pH on growth were determined at 35°C. The pH tested were 5.7, 6.1, 6.6, 6.8, and 7.4. The pH was stable during the cultivation period under sulfate-reducing condition.
Sulfide production was monitored colorimetrically using the methylene blue method with a PACKTEST (Kyoritsu Chemical-Check Lab, Tokyo, Japan). Sulfate concentration was measured by a high-pressure liquid chromatography system (Shimadzu, Kyoto, Japan) with a Shodex IC NI-424 column (Showa denko, Tokyo, Japan), and 8 mM p-hydroxybenzoic acid, 3.2 mM Bis-Tris, and 50 mM boric acid as the mobile phase at 40°C. Tetrathionate and thiosulfate were separated using Dionex IonPac AS25 column (Thermo Fisher Scientific) at 40°C and detected at 216 nm as described previously (Bak et al., 1993). The mobile phase consisted of 2.9 g L−1 NaCl dissolved in 70% acetonitrile and 10% methanol.
Disproportionation of S0 (1%, w/v), Na2SO3 (0.1%, w/v), Na2S2O3·5H2O (0.1%, w/v), or Na2S4O6·2H2O (0.1%, w/v) was tested in the sulfate-free MMJFE medium (prepared by substituting chloride salts for sulfate salts) under N2/CO2 (80:20; 300 kPa), with or without ferrihydrite (20 mM). Sulfur disproportionation was confirmed by the formation of sulfate and sulfide. When measuring changes in metabolite concentrations over time during sulfur disproportionation, 15 ml of medium was added to a 30 ml vial and 3 ml of cell cultures at the late-exponential growth phase was added from pre-culture.
To examine alternative electron donors and acceptors for autotrophic growth, each of the potential electron donors such as S0 (1%, w/v), Na2SO3 (0.1%, w/v), Na2S2O3·5H2O (0.1%, w/v) was examined in combination with S0 (1%, w/v), Na2SO3 (0.1%, w/v), Na2S2O3·5H2O (0.1%, w/v), NaNO3 (0.1%, w/v), ferric citrate (0.1%, w/v), 20 mM ferrihydrite, 20 mM hematite, or 20 mM goethite as the electron acceptors in the sulfate-free MMJFE medium without ferrihydrite under N2/CO2 (80:20; 300 kPa). In an attempt to test the growth on hydrogen gas as an electron donor, H2/CO2 (80:20) was used as the gas phase (300 kPa).
Heterotrophic growth of strain GF1T was tested by adding each of the following substrates at 0.1% (w/v) to MMJFE medium without NaHCO3 under 100% H2 (300 kPa): glucose, fructose, galactose, xylose, ribose, mannose, rhamnose, maltose, lactose, cellobiose, sucrose, arabinose, melibiose, citrate, succinate, pyruvate, lactate, propionate, malate, butyrate, formate, acetate, fumarate, malonate, tartrate, ethanol, methanol, 2-propanol, butanol, glycerol, ethylene glycol, glycine, glutamate, taurine, and casamino acids. In addition, to assess the utilization of these organic compounds as an energy source, substrates were added to MMJFE medium under N2/CO2 (80:20; 300 kPa). Fermentative growth with these substrates was also determined using the sulfate-free MMJFE medium under N2/CO2 (80:20; 300 kPa).
The utilization of N2 as a nitrogen source was tested using MMJFE medium lacking all nitrogen sources under H2/CO2/N2 (40:10:50; 300 kPa). Nitrogen fixation under sulfur-disproportionating condition was also tested in the medium designed for sulfur disproportionation tests (see above) lacking all nitrogen sources under N2/CO2 (80:20; 300 kPa). Throughout the test, 1 ml trace element solution SL-10 (Widdel et al., 1983) was substituted for 10 ml trace mineral solution (Sako et al., 1996) in MMJFE medium (per liter) to remove all possible nitrogen sources.
To confirm nitrogen fixation by strain GF1T, 15N2 tracer assays were performed under hydrogenotrophic sulfate-reducing, and S0- or thiosulfate-disproportionating condition with 300 ml medium designed for nitrogen fixation test (see above). Immediately before inoculation, 20 ml of 15N2 (99.9 atom%, Shoko Science Co., Ltd., Kanagawa, Japan) was added to the headspace of a 1,100 ml glass bottle. Control experiments were performed by using 15N2-free medium with or without inoculation. After cultivation, cells of the late-exponential growth phase were collected by filtration through 25 mm GF/F filters (0.3 μm pore size) that were pre-combusted at 400°C for 4 h. Cells on the filter were rinsed with 0.1 M HCl and immediately stored at −80°C until analysis. The nitrogen isotope ratios were determined by isotope ratio mass spectrometer (IRMS) coupled to an elemental analyzer (Flash 2000-DELTA plus Advantage ConFlo III System; Thermo Fisher Scientific) at Shoko Science Co.
Cellular fatty acids, respiratory quinones, and polar lipids of strain GF1T were extracted and analyzed as described previously (Hashimoto et al., 2021). Cells of the late-exponential growth phase in MMJFE medium at 35°C were used.
Genome sequencing and analyses
The whole genome sequencing and the contamination and quality check of the genome were conducted as described in (Hashimoto et al., 2021). The functional annotation was performed with DFAST (Tanizawa et al., 2018) and RAST v2.0 (Aziz et al., 2008). Metabolic pathways were predicted using the Kyoto Encyclopedia of Genes and Genomes (KEGG) based on the list of functional genes, which were automatically annotated by DFAST and then manually curated with the BLAST algorithm (Altschul et al., 1997). The HydDB webtool was used for hydrogenase classification1 (Søndergaard et al., 2016). Protein subcellular localization was predicted using PSORTb v3.0.3 (Yu et al., 2010). Average nucleotide identity (ANI) and average amino acid identity (AAI) were calculated with OrthoANI (Lee et al., 2016) and the Kostas lab AAI calculator,2 respectively.
A maximum-likelihood phylogenomic tree was reconstructed based on a total of 145 concatenated single-copy marker genes (Supplementary Table 1) by using ezTree software with the default parameters: Jones-Taylor-Thornton (JTT) model and 1,000 bootstraps (Wu, 2018). The resulting tree was then visualized using MEGA X v10.2.6 (Kumar et al., 2018).
Shotgun proteomics sample preparation and LC–MS/MS analysis
For the shotgun proteomics, cells cultured under hydrogenotrophic sulfate-reducing and thiosulfate-disproportionating conditions were harvested at the late-exponential growth phase. Cells (about 1010 cells) were suspended in 400 μl of 100 mM triethylammonium bicarbonate (TEAB; pH 8.6) containing 2 mM phenylmethylsulfonyl fluoride (PMSF) and disrupted by sonication using a Q700 sonicator at a frequency of 40 kHz (QSonica, Newtown, CT, United States). The protein concentration of the cell-free extract was determined by a Qubit fluorometer (Thermo Fisher Scientific). The remaining procedure and LC–MS/MS data acquisitions were carried out as described previously (Kawai et al., 2022).
Proteomics analysis
Protein identification was performed with the Proteome Discoverer 2.2 software package (Thermo Fisher Scientific). The acquired spectra were searched against the list of CDSs identified in the genome of strain GF1T using the SEQUEST HT search algorithm, as described previously (Kawai et al., 2022). The results from biological triplicates were combined and filtered with a cut-off value of 1% protein false discovery rate (FDR) calculated using Protein FDR Validator node. A final dataset was generated to contain only proteins present in all three replicates. Relative protein abundances were calculated using normalized spectral abundance factors (NSAFs) method based on the number of PSMs per protein (Zybailov et al., 2006). NSAFs were then multiplied by 100 to calculate the relative protein abundance (percentage). For comparative proteomics, proteins with an average relative protein abundance of at least 0.1% in two tested conditions were mainly included in order to avoid overestimation.
Orthology analysis
Protein sequences derived from a total of 93 bacterial genomes were obtained from NCBI Datasets. Analyzed species belonged to the classes Clostridia, Nitrospira, Deltaproteobacteria, and Thermodesulfobacteria. These included 87 strains from a previous dataset used for comparative genomics of sulfur-disproportionating bacteria (Umezawa et al., 2020), five species newly added in this study Dissulfurispira thermophila T55JT (Umezawa et al., 2021), Desulfovibrio desulfuricans DSM 642T (Bak and Pfennig, 1987; Galushko and Kuever, 2019), Dissulfurimicrobium hydrothermale Sh68T (Slobodkin et al., 2016; Yvenou et al., 2022), Thermosulfurimonas marina SU872T (Frolova et al., 2018), and Thermosulfuriphilus ammonigenes ST65T (Slobodkina et al., 2017), and strain GF1T. Of the 93 species, 33 were sulfur-disproportionating bacteria. Proteins were grouped into orthologues by using OrthoFinder v2.5.4 with BLAST as the sequence search program (Emms and Kelly, 2015; Emms and Kelly, 2019). The identified ortholog groups were manually annotated based on the protein description of strain GF1T within each group. To identify thiosulfate reductase-type protein or tetrathionate reductase-type protein, proteins corresponding to thiosulfate reductase-type protein subunit A (PhsA) or tetrathionate reductase-type protein subunit A (TtrA) were manually selected based on ortholog groups and manual checking of the amino acid length. Then, other subunits were also manually determined by checking the arrangement of genes in the vicinity of phsA or ttrA in the genome. Typically, the following arrangements were assigned as genes encoding thiosulfate reductase-type protein or tetrathionate reductase-type protein, respectively: phsAB(C) or ttrBCA. The combination of ttrA with distantly encoded ttrBC was also assigned as a single tetrathionate reductase-type protein.
Results
Cell morphology and phylogeny
The cells of strain GF1T were Gram-negative and motile short rods with a single flagellum (1.0–2.1 μm in length and 0.5–1.1 μm in width; Figure 1). Spore formation was not observed throughout the microscopic observation in this study.
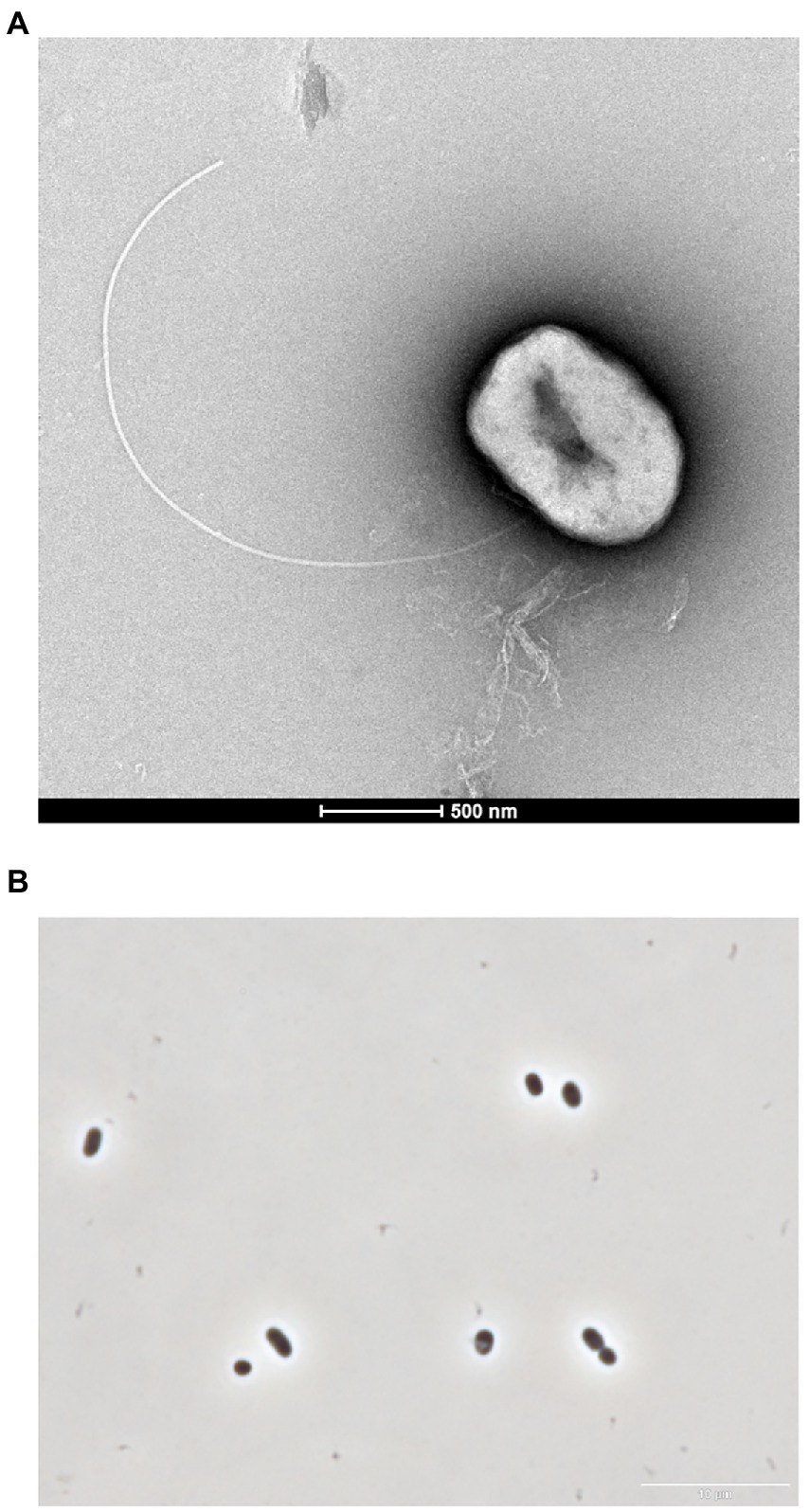
Figure 1. (A) Transmission electron micrograph showing a cell of GF1T. Scale bar represents 0.5 μm. (B) Phase contrast micrograph of strain GF1T. Scale bar represents 10 μm.
The 16S rRNA gene sequence of strain GF1T showed 98.2% similarity to that of an uncultured bacterium clone, PWB045, retrieved from the Halfdan oil field in the North Sea (Gittel et al., 2012). The closest cultured species were Desulfogranum mediterraneum 86FS1T (93.3%; Sass et al., 2002) and Desulfobulbus sp. strain KaireiS1 (92.9%; Adam et al., 2021). These similarity values are below the common index of 16S rRNA gene sequence similarity for differentiation of microorganisms at the genus level (94.5%; Yarza et al., 2014; Figure 2A).
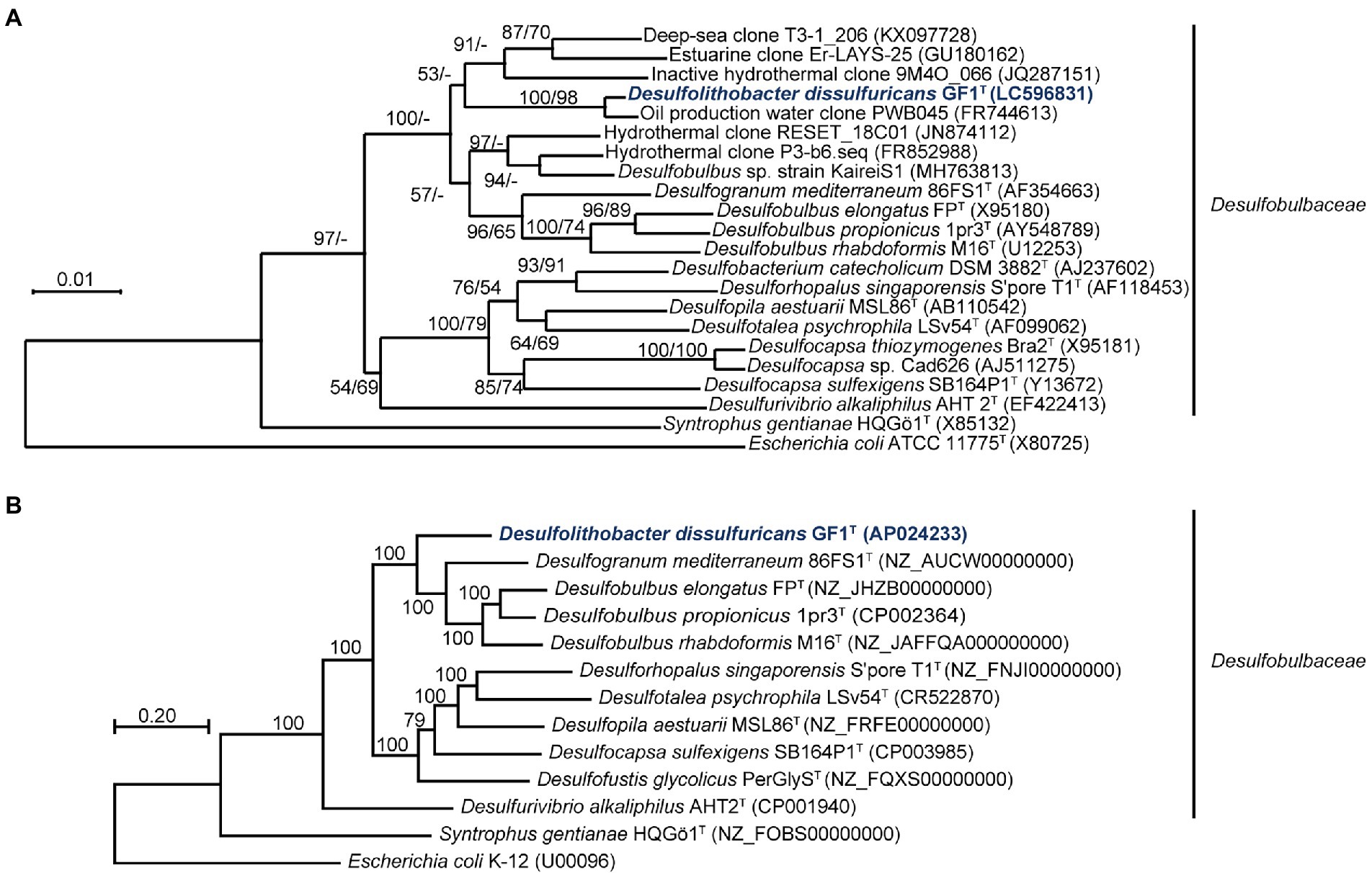
Figure 2. (A) Phylogenetic tree of representative members and environmental clones within the family Desulfobulbaceae, inferred from 16S rRNA gene sequences by the neighbor-joining method using 1,214 homologous sequence positions for each organism. Numbers at nodes are bootstrap values (percentages) based on 1,000 replicates (shown in the order neighbor-joining/maximum-likelihood). GenBank/EMBL/DDBJ accession numbers are given in parentheses. Bar, 0.01 changes per nucleotide position. (B) Maximum-likelihood phylogenetic tree of representative members within the family Desulfobulbaceae, based on concatenated 145 single-copy marker genes. Numbers at each node are bootstrap values (percentages) based on 1,000 replicates. GenBank/EMBL/DDBJ accession numbers are given in parentheses. Bar, 0.20 changes per nucleotide position.
Growth and chemotaxonomic characteristics
Strain GF1T grew in the range of temperatures (25°C–50°C), NaCl concentrations (20–40 g L−1), and pH (6.1–6.8). The optimum growth occurred at 35°C, pH 6.6, and 3.0% (w/v) NaCl (Supplementary Figure 2). The generation time and maximum cell density under the optimal conditions were about 7.6 h and 2.2 × 107 cells ml−1, respectively. During growth, the decrease in sulfate and the production of sulfide were observed (data not shown), confirming that strain GF1T was a chemolithoautotrophic sulfate reducer.
Strain GF1T did not grow on any of the tested organic compounds as a carbon source. However, the isolate was able to use formate or casamino acids as the sole energy source in the presence of CO2. Slow growth was observed when malate was provided as an electron donor (Table 1). No fermentative growth occurred with any tested substrates.
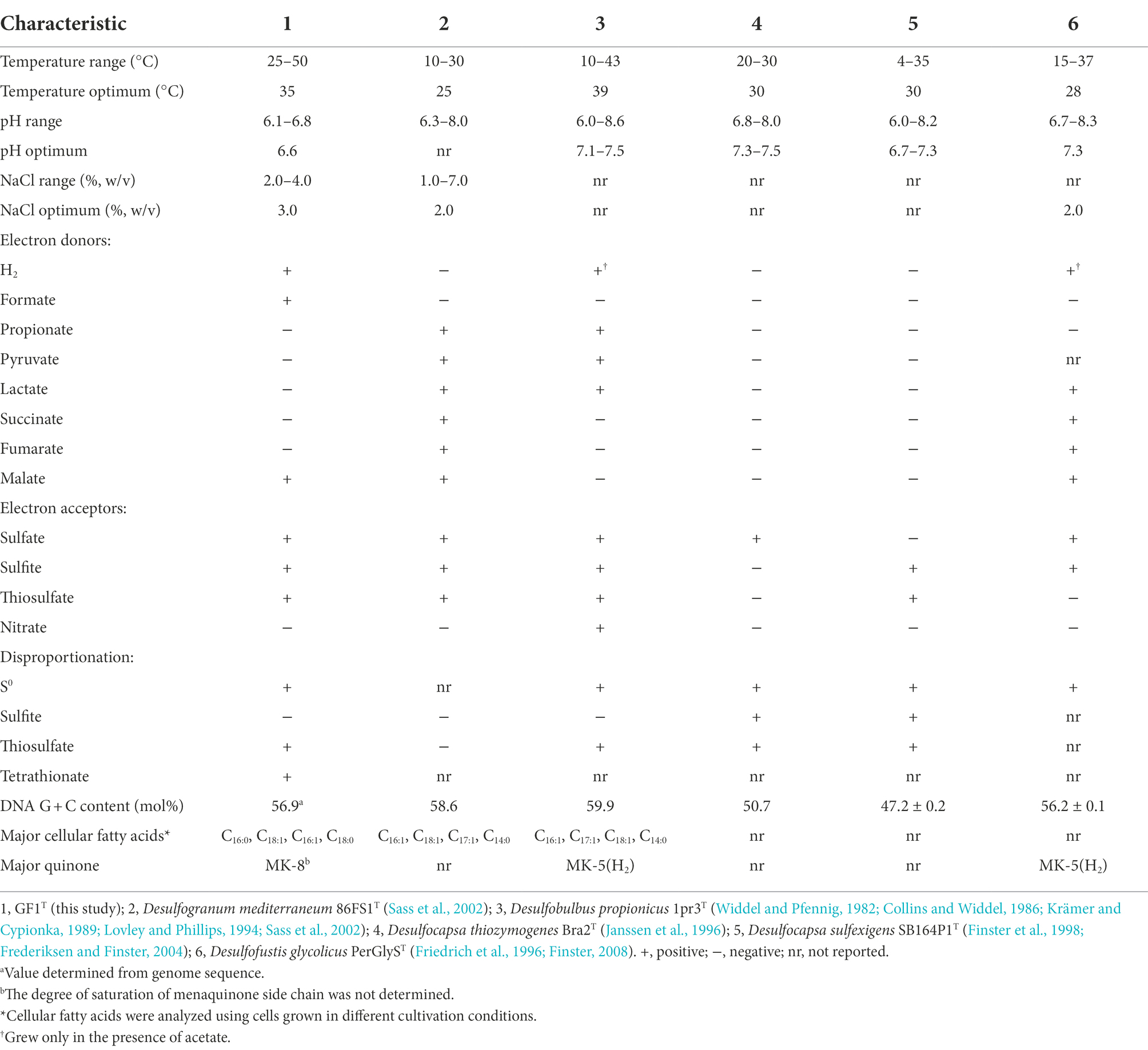
Table 1. Comparison of physiological characteristics of strain GF1T with related genera of Desulfobulbaceae.
The isolate was found to utilize H2 as an electron donor and sulfate, sulfite, and thiosulfate as electron acceptors (Table 1). In addition, strain GF1T was able to grow via disproportionation of S0 (38 h doubling time), thiosulfate (20 h doubling time), and tetrathionate (17 h doubling time) only in the presence of ferrihydrite as a sulfide scavenger. The final cell yield under sulfur disproportionation was almost the same as that under sulfate-reducing condition (about 107 cells ml−1). Although elemental sulfur could not be quantified, a decrease of thiosulfate/tetrathionate and a concomitant increase of sulfate and cell density was observed (Figure 3). During thiosulfate disproportionation, a trace amount of tetrathionate was produced and then consumed as the cell density increased (Figure 3A). In the abiotic control, no such change in tetrathionate concentration was detected. Similarly, slight thiosulfate production and subsequent consumption were observed during tetrathionate disproportionation (Figure 3C). Tetrathionate/thiosulfate present at hour 0 of incubation under thiosulfate/tetrathionate-disproportionating conditions were probably due to carryover from the pre-culture. Thiosulfate may also have been formed by the abiotic decomposition of tetrathionate in the presence of sulfide (Rowe et al., 2015). Sulfite did not serve as a substrate for disproportionation.
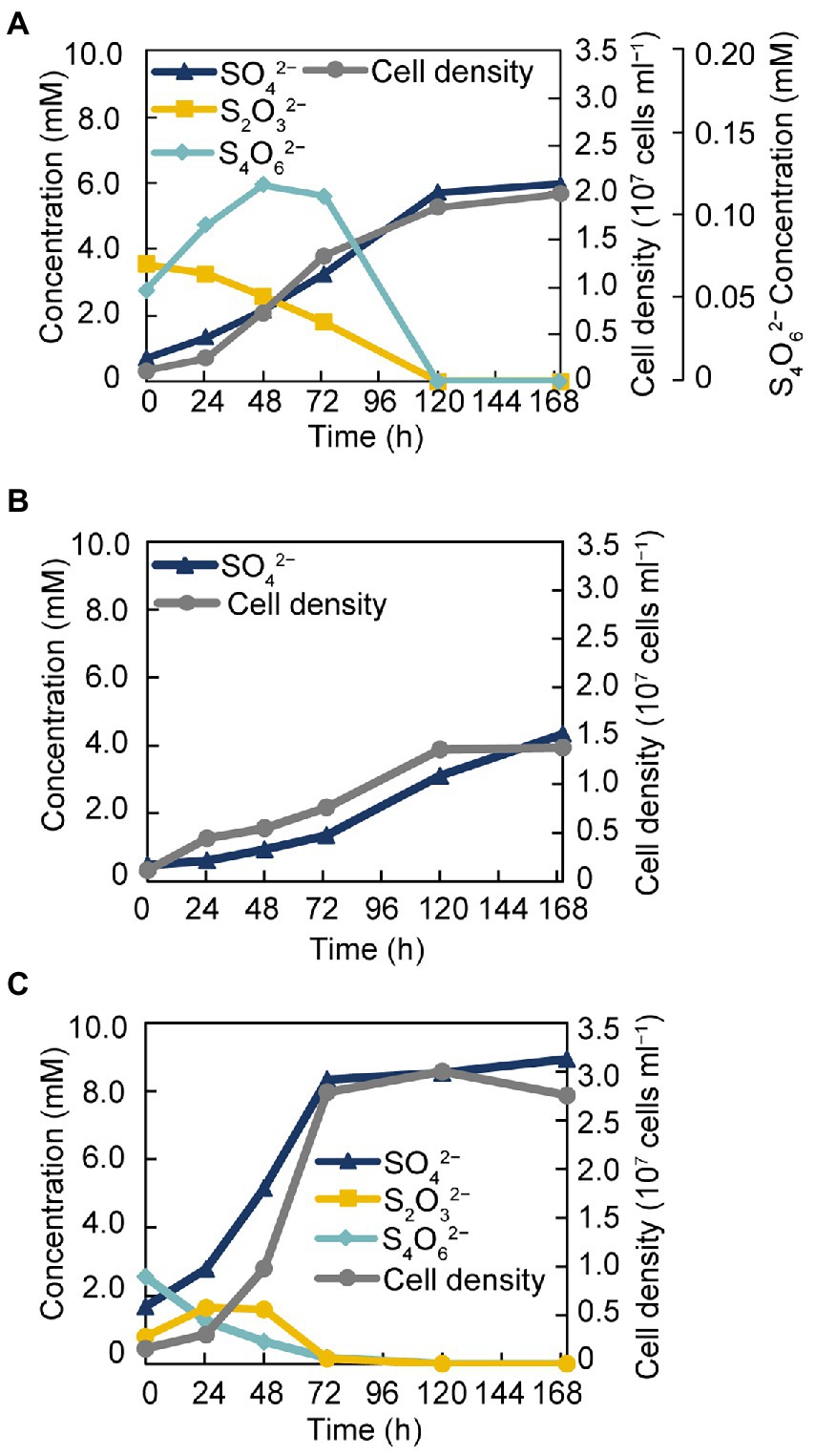
Figure 3. Growth of strain GF1T via disproportionation of thiosulfate (A), S0 (B), and tetrathionate (C).
Diazotrophic growth was observed under both sulfate-reducing and sulfur-disproportionating conditions. N2 fixation was demonstrated by assimilation of 15N2 into cellular components (Supplementary Table 2).
The major cellular fatty acids of strain GF1T were C16:0 (36.3%), C18:1 (26.5%), C16:1 (24.7%), and C18:0 (6.0%; Table 1). The respiratory quinone of strain GF1T was menaquinone-8 (MK-8). Strain GF1T possessed phosphatidylethanolamine (PE), phosphatidylglycerol (PG), and an unidentified polar lipid, as Desulfogranum japonicum Pro1T did (Supplementary Figure 3).
Genome characteristics
The genome of strain GF1T was assembled into one circular chromosomal contig of 3,596,428 bases and contained 3,242 coding sequences (CDSs). The G + C content was 56.9%, and the mean depth of coverage was 292×. No genome contamination was confirmed by ContEst16S. The topology of the phylogenomic tree (Figure 2B) was generally consistent with that of the 16S rRNA gene-based phylogenetic tree (Figure 2A). The ANI and AAI between strain GF1T and related genera of Desulfobulbaceae supported that the isolate could be differentiated at the genus level within the family Desulfobulbaceae, based on the previously proposed threshold (Goris et al., 2007; Richter and Rosselló-Móra, 2009; Konstantinidis et al., 2017; Supplementary Figure 4).
A complete gene set for dissimilatory sulfate reduction was found in the genome, including sulfate adenylyltransferase (sat), adenylylsulfate reductase subunit α/β (aprAB), dissimilatory sulfite reductase subunit α/β (dsrAB), dsrC, dsrD, and dsrMKJOP complex (Supplementary Table 3). Genes corresponding to APS reductase-associated electron transfer complex (qmoABC) were also identified. In addition, genome analysis revealed the presence of genes potentially involved in sulfur disproportionation, including molybdopterin oxidoreductases, which may act as a subunit of thiosulfate, polysulfide, or tetrathionate reductase.
In addition to Sat and Apr, sulfite oxidoreductase has also been proposed as a possible enzyme involved in the oxidative route of sulfur disproportionation (Finster, 2008), but no gene encoding sulfite oxidoreductase was identified in the genome of strain GF1T. Genes associated with the oxidation of S0 and thiosulfate, such as sulfur-oxidizing (sox) system, sulfide: quinone oxidoreductase (sqr), or sulfur oxygenase reductase (sor), were missing in the genome, as previously reported in several sulfur-disproportionating bacteria (Slobodkin and Slobodkina, 2019).
The genes of the Wood-Ljungdahl pathway for CO2 fixation were identified (Supplementary Table 3). For molecular hydrogen oxidization, the isolate harbored genes encoding membrane-anchored periplasmic [NiFe] hydrogenase (HynABC), cytoplasmic [NiFe] methyl-viologen-reducing hydrogenase, and hydrogenase accessory proteins. Genes from the TCA cycle were present in the genome of strain GF1T, which may be responsible for the metabolism of C4-dicarboxylates including malate. Further, all genes for C4-dicarboxylate transporters (Dct), which function in the uptake of C4 compounds, were detected in the genome (GF1_10880 to GF1_10900). Genes involved in amino acid transport were encoded in the genome. The genome also contained genes needed for nitrogen fixation, which was congruent with its ability to grow diazotrophically (Supplementary Table 3). Additionally, genes encoding flagella components were identified in the genome.
Comparative proteomic analysis
To identify proteins that were specifically and/or abundantly produced when strain GF1T grew via sulfur disproportionation, a comparative proteomic analysis was performed using cells grown under two different conditions: thiosulfate-disproportionating condition (hereinafter referred to as TD) and hydrogenotrophic sulfate-reducing condition (SR). We identified a total of 1,172 proteins (Figure 4A). Thereof, 36 and 476 were exclusively found in TD and SR, respectively. Of the 36 proteins exclusively found in TD, the top 10 most abundant proteins were listed in Table 2. The most abundant TD-specific protein exhibited a high level of amino acid sequence similarity (70.6%) to the molybdopterin-binding subunit A of tetrathionate reductase of Dissulfuribacter thermophilus (WP_067617696), that is a sulfur disproportionator originated from a deep-sea hydrothermal vent (Slobodkin et al., 2013). The other subunits of tetrathionate reductase-type protein, the electron transfer subunit B (TtrB) and the membrane-anchor subunit C (TtrC), were also identified only in TD (Table 2). In addition, a hypothetical protein encoded by a gene adjacent to ttrBCA in the GF1T genome (GF1_03960) and another molybdopterin oxidoreductase (GF1_03980) were uniquely found in TD (Supplementary Table 4), suggesting its potential involvement in thiosulfate disproportionation. Other proteins exclusively found in TD contained subunits of NADH-quinone oxidoreductase (Nuo; GF1_30510, GF1_30520, GF1_31140, GF1_31220; Supplementary Table 4). In addition, a rhodanese-like domain-containing protein (Rhd; GF1_13980) was detected only in TD, which was in accordance with the proteome data from Desulfurella amilsii grown under S0-disproportionating condition (Florentino et al., 2019).
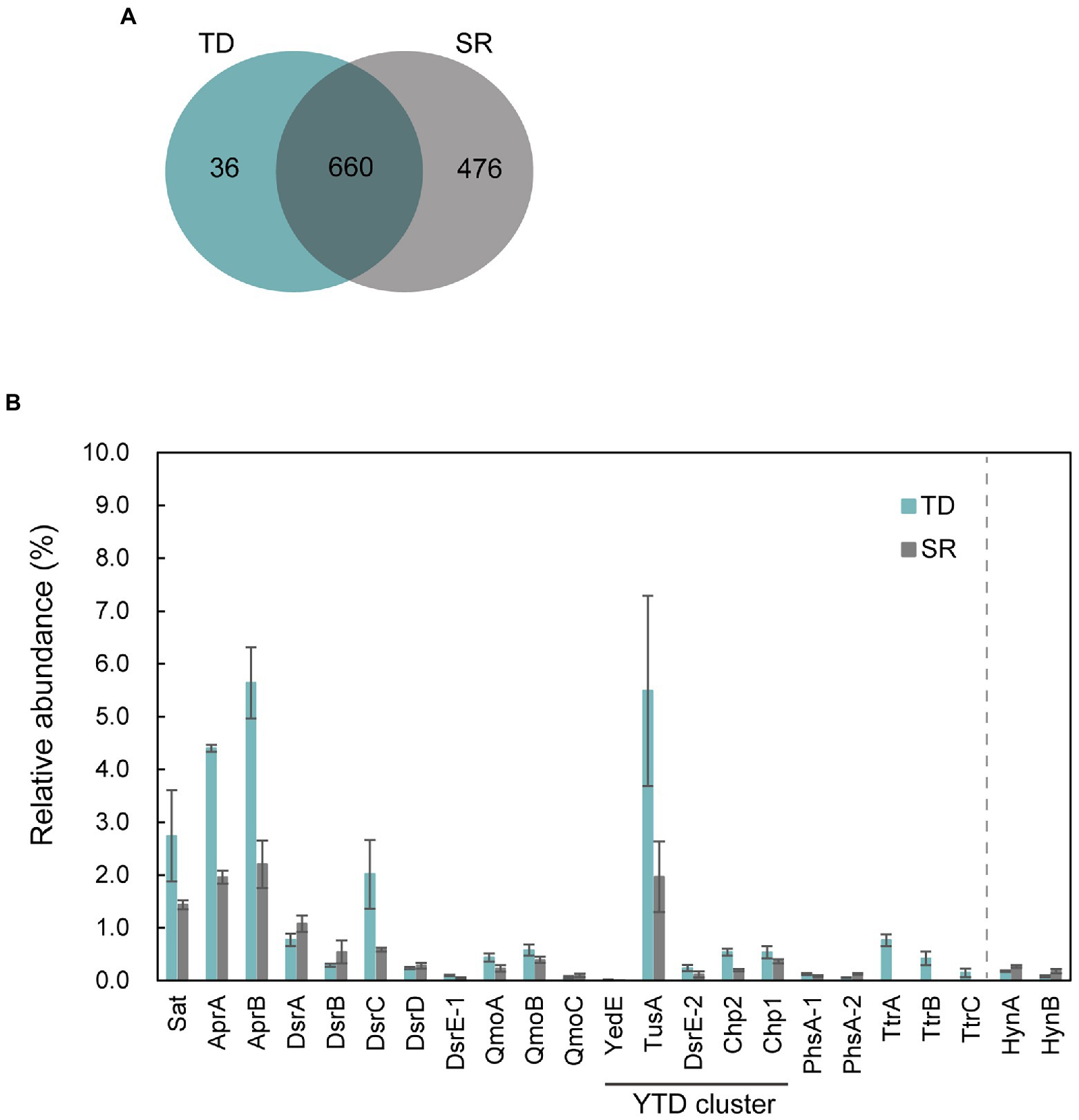
Figure 4. Proteomic profiles of strain GF1T under thiosulfate disproportionation (TD) and sulfate reduction (SR). (A) Venn diagram of shared/unique proteins identified in the proteome of TD (Blue) and SR (Gray). (B) Relative abundance (%) of proteins potentially involved in sulfur metabolism found in the proteome of strain GF1T under TD (Blue) and SR (Gray). Relative abundance is based on the average NSAF values of the biological triplicates. Error bars represent standard error of the biological triplicates. HynA/B is shown for comparison. The locus tag for each protein is listed in Supplementary Table 9.
The top 20 most abundant proteins in TD and SR are shown in Supplementary Table 5, and all proteins found in TD and SR are listed in Supplementary Tables 6, 7, respectively. Among 660 proteins detected in both TD and SR, relatively abundant proteins in TD included proteins possibly involved in sulfur metabolism (Figure 4B, Supplementary Tables 8, 9); DsrC, the substrate for DsrAB in dissimilatory sulfate reduction pathway (Santos et al., 2015), was found to be 3.4-fold more abundantly produced in TD than in SR (GF1_24490). Sat and AprAB, which have been suggested to function in the oxidative route of sulfur disproportionation, were even more abundantly produced in TD (more than 1.5-fold change). APS reductase-associated electron transfer complex (QmoABC) was also abundantly produced in TD, except for transmembrane subunit QmoC. In contrast, DsrAB and its allosteric activator DsrD, which work in sulfate reduction and potentially in the reductive branch of sulfur disproportionation, were slightly less produced in TD than in SR. DsrE/F like family protein (hereafter DsrE-1; GF1_04100) was present in both conditions, although the relative protein content was <0.1%.
In addition, it was noteworthy that all five proteins (referred to as YedE, TusA, DsrE-2, Chp2, and Chp1) encoded by the YTD gene cluster (GF1_30690 to GF1_30730) were more abundant in TD (Figure 4B), although the relative protein content of YedE (GF1_30690) was <0.1% (0.01% in TD and 0.007% in SR). The YTD cluster is reported to potentially have an important role in microbial sulfur disproportionation, but its function has not been determined in detail (Umezawa et al., 2020).
Moreover, two proteins corresponding to thiosulfate reductase-type protein subunit A (PhsA), molybdopterin-containing oxidoreductase, were detected in both TD and SR. PhsA-1 (GF1_30490) was slightly more abundant in TD and PhsA-2 (GF1_10120) was more abundant in SR (Figure 4B). Another subunit of thiosulfate reductase-type protein, PhsB, was also found in both TD and SR (PhsB-1, GF1_30500 and PhsB-2, GF1_10130; Supplementary Tables 6, 7). Further, a full set of enzymes required for CO2 fixation by the Wood-Ljungdhal pathway were detected in both TD and SR.
The SR-specific proteins contained proteins encoded by a hydrogenase gene cluster, including membrane-anchored periplasmic [NiFe] hydrogenase, cytoplasmic [NiFe] methyl-viologen-reducing hydrogenase/heterodisulfide reductase (mvh/hdr), and hydrogenase accessory proteins (GF1_18040, GF1_18060, GF1_18080, GF1_18140 to GF1_18220, GF1_18260 to GF1_18290; Supplementary Table 10). For membrane-anchored periplasmic [NiFe] hydrogenase (HynABC), only HynC (GF1_18080) was specifically present in SR, and HynAB (GF1_18090, GF1_18100) was more abundantly produced in SR than in TD (Figure 4B). The SR-specific most abundant protein (hypothetical protein encoded by GF1_18120) was also encoded by a gene within this hydrogenase cluster (Supplementary Figure 5), but its functional assignment was unclear. SulP family inorganic anion transporter (GF1_18110), encoded by a gene adjacent to GF1_18120, was also uniquely produced in SR.
Orthologous proteins in diverse bacteria
Orthologs of the subunits of tetrathionate reductase-type protein in strain GF1T were present in Desulfitobacterium hafniense, 12 other Deltaproteobacteria, and four Thermodesulfobacteria members (Supplementary Tables 11, 12). Of these, 11 species had complete TtrBCA. Desulfoluna spongiiphila, Desulfosarcina cetonica, Desulfobulbus propionicus, and Caldimicrobium thiodismutans had TtrAB cluster lacking TtrC. Desulfuromusa kysingii and Dissulfuribacter thermophilus had complete TtrBCA as well as TtrAB lacking TtrC. Of all these species, six deltaproteobacterial species and four thermodesulfobacterial species were reported to be sulfur-disproportionating bacteria. All of them were capable of thiosulfate disproportionation, except Desulfurella amilsii, which has not been tested for thiosulfate disproportionation. Of these sulfur-disproportionating species with orthologs of tetrathionate reductase-type protein, Dissulfuribacter thermophilus, Dissulfurimicrobium hydrothermale, and four thermodesulfobacterial species originated from thermal ecosystems. Further, all these six species had orthologous proteins encoded by the YTD gene cluster (Supplementary Table 13). Thermosulfurimonas dismutans and Thermosulfurimonas marina were the only thiosulfate-disproportionating species with an ortholog of tetrathionate reductase-type protein but lacking thiosulfate reductase-type protein. No other species with orthologs of tetrathionate reductase-type protein has reports on the ability of sulfur disproportionation, except Desulfatirhabdium butyrativorans, which is unable to disproportionate thiosulfate and sulfite (Balk et al., 2008). Ten thiosulfate-disproportionating species contained neither an ortholog of thiosulfate reductase-type protein nor an ortholog of tetrathionate reductase-type protein.
Orthologs of thiosulfate reductase-type protein in strain GF1T were found in three Clostridia, four Nitrospira, 30 other Deltaproteobacteria, and nine Thermodesulfobacteria members (Supplementary Tables 11, 14). Among these species, 15 were identified as sulfur-disproportionating bacteria, eight were unable to disproportionate inorganic sulfur compounds, and 23 species have no reports on sulfur disproportionation. Sulfur-disproportionating species with orthologs of thiosulfate reductase-type protein included Desulfotomaculum nigrificans, 12 other deltaproteobacterial species, and two thermodesulfobacterial species. All these species except Desulforhopalus singaporensis, Desulfovibrio cuneatus, and Paucidesulfovibrio longus were able to disproportionate thiosulfate. Desulfotomaculum nigrificans, Desulfobacter curvatus, Desulfocapsa sulfexigens, Desulfonatronospira thiodismutans, and Desulfovibrio aminophilus were thiosulfate-disproportionating species with an ortholog of thiosulfate reductase-type protein but lacking tetrathionate reductase-type protein.
Discussion
In this study, we isolated and characterized strain GF1T, which is the first mesophilic sulfur disproportionator from a deep-sea hydrothermal vent. Strain GF1T differs from other sulfur-disproportionating bacteria from deep-sea hydrothermal systems in that it is a facultative sulfate reducer (Slobodkin et al., 2012, 2013; Slobodkina et al., 2017). In addition, strain GF1T is unique in that it can utilize N2 as the sole nitrogen source under sulfur-disproportionating conditions and it is in line with the identification of nifDK genes in the genome. Although N2 fixation ability has not been well investigated in sulfur-disproportionating bacteria, nif genes have been found in the genomes of sulfur disproportionators, i.e., Desulfocapsa sulfexigens, Thermosulfurimonas dismutans, and Dissulfurimicrobium hydrothermale (Finster et al., 2013; Mardanov et al., 2016; Yvenou et al., 2022). Phylogenetic analysis showed that the isolate is closely related to the genus Desulfogranum but within a previously uncultivated cluster of Desulfobulbaceae members (Figure 2). Major cellular fatty acids of strain GF1T are similar to that of the members of the genus Desulfogranum (Galushko and Kuever, 2020), whereas the strain exhibits a much higher percentage of C16:0. Further, the respiratory quinone of the isolate (MK-8) is different from the major quinone of the closely related genus Desulfogranum (MK-5(H2); Table 1; Galushko and Kuever, 2020). Based on the molecular, physiological, and chemotaxonomic analyses, we propose that strain GF1T represents the type strain of a novel species in a new genus, Desulfolithobacter dissulfuricans gen. nov., sp. nov.
Comparative proteomic study revealed that proteins related to the subunits of molybdopterin-containing tetrathionate reductase-type protein (GF1_03930 to GF1_03950) were specifically and abundantly produced (Table 2, Figure 4B) when strain GF1T was grown via thiosulfate disproportionation. Tetrathionate reductase is an enzyme that is responsible for the reduction of tetrathionate to thiosulfate (Hensel et al., 1999), but our result indicated its possible involvement in thiosulfate disproportionation by strain GF1T. Given that thiosulfate is simultaneously reduced and oxidized during disproportionation, it is possible that identified tetrathionate reductase-type protein could play a major role in either oxidation or reduction step (Figure 5). However, it should be noted that it is thermodynamically unfavorable to oxidize thiosulfate to tetrathionate (Em = +180 mV) using the MK-8 (Em = −70 mV) identified in our strain.
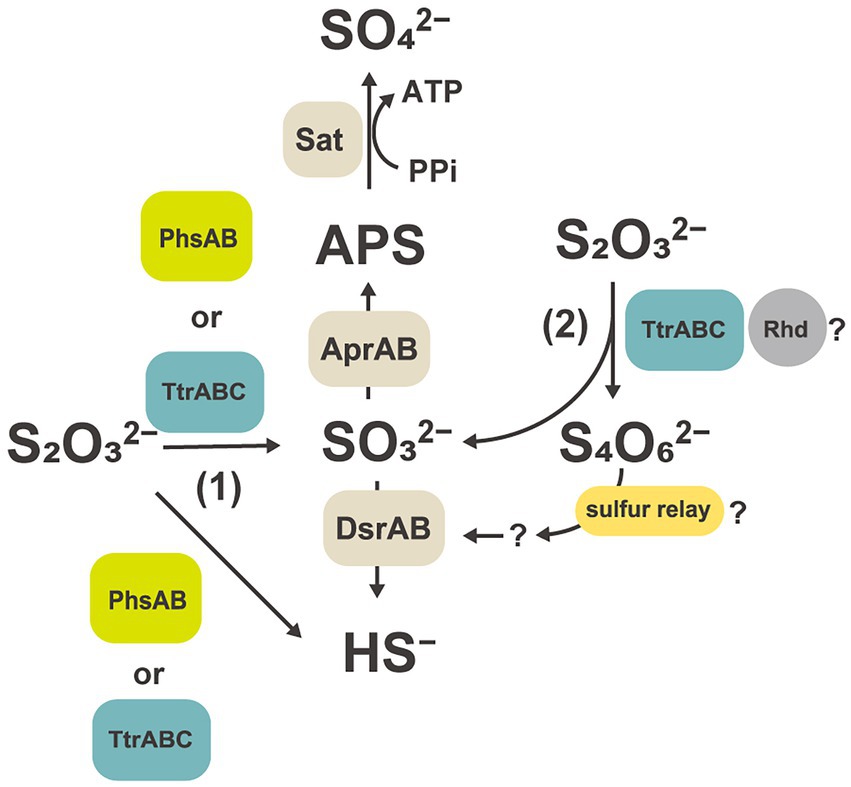
Figure 5. Hypothetical thiosulfate disproportionation pathways in strain GF1T based on a comparative proteomic analysis. Sat, sulfate-adenylyltransferase; Apr, adenylyl-sulfate reductase; APS, adenosine 5′-phosphosulfate; Dsr, dissimilatory sulfite reductase subunit; Phs, thiosulfate reductase-type protein; Ttr, tetrathionate reductase-type protein; Rhd, rhodanese-like domain-containing protein.
Tetrathionate reductase-type protein has been suggested to be functionally diverse; for example, sequences showing a BCA order of genes but not clustering with tetrathionate reductase-type protein were identified and whose metabolic function is still unclear (Duval et al., 2008). Further, tetrathionate reductase-type sequence which may function as dissimilatory As(V) reductases, lacking tetrathionate reductase activity, was reported in (Muramatsu et al., 2020). Therefore, further biochemical and enzymological characterization would be needed to elucidate the function of tetrathionate reductase-type protein identified in this study.
Another unexpected result of the proteomic analysis was that the protein content of thiosulfate reductase-type protein was relatively low under thiosulfate disproportionation condition (0.12%, PhsA-1; 0.06%, PhsA-2; Figure 4B). This observation may imply that thiosulfate reductase, which is believed to function in the first step of thiosulfate disproportionation pathway (Krämer and Cypionka, 1989; Frederiksen and Finster, 2003), may not be a major player in thiosulfate disproportionation in our isolate. In addition, Phs identified in this study lacked subunit C. To date, no functional analysis of PhsAB has been performed, and C subunit is considered to be essential for the enzyme to interact with its co-substrate, quinone. However, a recent study proposed that PhsAB in a deltaproteobacterial Desulfurella amilsii can mediate the cleavage of thiosulfate into sulfite and sulfide (Florentino et al., 2019).
Moreover, in our proteomic study, all proteins encoded by the YTD gene cluster were more abundantly detected in thiosulfate-disproportionating condition (Figure 4B). This result supported the idea that the YTD gene cluster can be involved in sulfur disproportionation (Umezawa et al., 2020). Although its functional assignment is unclear, TusA and DsrE in the YTD gene cluster are known to be involved in sulfur transfer to the Dsr system in sulfur-oxidizing bacteria, using their conserved cysteine residues as sulfane sulfur binding sites (Stockdreher et al., 2012, 2014; Venceslau et al., 2014; Tanabe et al., 2019; Dahl, 2020). Taking into account that TusA (GF1_30700) and DsrE-2 (GF1_30710) encoded by the YTD gene cluster in strain GF1T harbored conserved cysteine residues, these proteins can work as sulfur carrier proteins in strain GF1T. In addition, a recent study on DsrE/TusA homolog proteins from an acidothermophilic sulfur-oxidizing archaeon Metallosphaera cuprina has biochemically proven that tetrathionate reacts with DsrE/TusA homolog proteins and its thiosulfonate is irreversibly transferred (Liu et al., 2014). Thus, tetrathionate produced by tetrathionate reductase-type protein may be consumed by the reaction with TusA and DsrE in thiosulfate disproportionation in strain GF1T. In this case, sulfite derived from released thiosulfonate may eventually be passed to the Dsr system by sulfur relay, finally converted to sulfide according to the previously proposed mechanism (Santos et al., 2015; Figure 6). Furthermore, as recently proposed for Desulfurivibrio alkaliphilus (Thorup et al., 2017), it is possible that enzymes already known to be involved in sulfur metabolism have an unexpected function. Although further detailed biochemical or molecular genetic experiments are necessary to justify our hypothetical model reconstructed from the proteomic results, the model may represent a novel reaction pathway underlying thiosulfate disproportionation in strain GF1T.
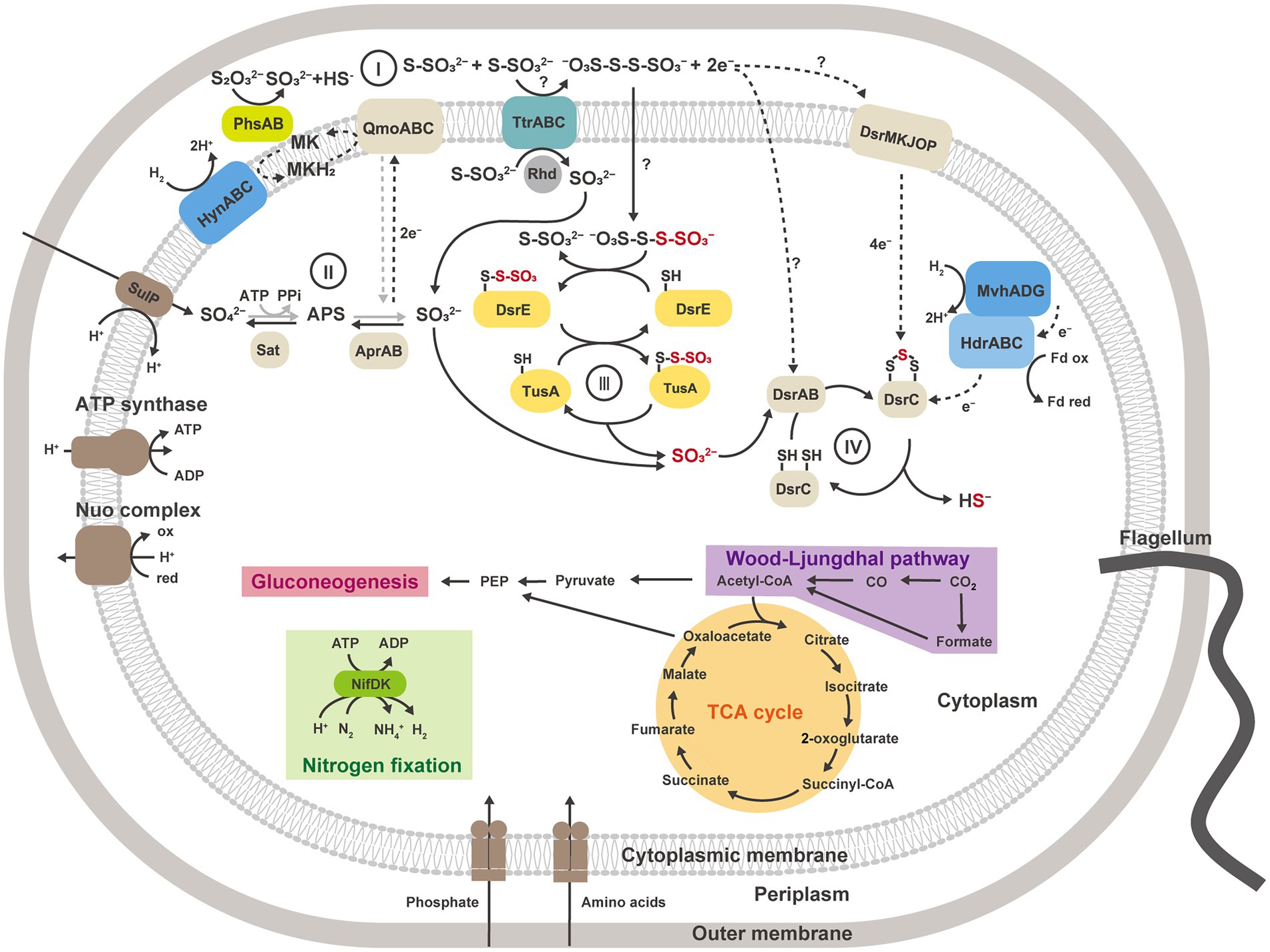
Figure 6. Metabolic reconstruction of strain GF1T and proposed model for thiosulfate disproportionation in strain GF1T, involving the enzyme reaction by tetrathionate reductase-type protein (I), oxidation of sulfite to sulfate by Apr and Sat (II), possible transfer of thiosulfonate derived from tetrathionate by potential sulfur carrier proteins and delivery of sulfite to DsrAB (III), reduction of sulfite to sulfide by DsrAB (IV). The strain grows via sulfur disproportionation and respiratory hydrogen oxidation coupled to sulfate reduction in the presence of CO2. When reactions can work in both directions, the pathway for dissimilatory sulfate reduction is shown in gray. Sat, sulfate-adenylyltransferase; Apr, adenylyl-sulfate reductase; APS, adenosine 5′-phosphosulfate; Dsr, dissimilatory sulfite reductase subunit; Phs, thiosulfate reductase-type protein; Ttr, tetrathionate reductase-type protein; Rhd, rhodanese-like domain-containing protein; Qmo, APS reductase-associated electron transfer complex.
Orthology analysis revealed that orthologous proteins of thiosulfate reductase-type protein were found in nearly half of the analyzed species (Supplementary Table 11). This may be partly because proteins orthologous to thiosulfate reductase-type protein can be possessed not only by thiosulfate-disproportionating bacteria but also by thiosulfate-reducing bacteria. Compared to thiosulfate reductase-type protein, orthologous proteins of tetrathionate reductase-type protein were less prevalent but present not only in strain GF1T but also in 10 other sulfur-disproportionating species (Supplementary Table 11). Eight of these 10 species, with the exception of Desulfobulbus propionicus and Caldimicrobium thiodismutans, had orthologs of complete TtrBCA. An ortholog of tetrathionate reductase-type protein was also found in Desulfatirhabdium butyrativorans, which was reported to be unable to disproportionate thiosulfate and sulfite, but its inability should be confirmed using sulfide scavenger (Balk et al., 2008). These results indicated that tetrathionate reductase-type protein might be commonly possessed by many sulfur-disproportionating bacteria and associated with sulfur disproportionation. In addition, we found that bacteria with both an ortholog of tetrathionate reductase-type protein and orthologous proteins encoded by the YTD gene cluster tend to be able to perform sulfur disproportionation. Moreover, interestingly, most of them were derived from thermal environments, including deep-sea hydrothermal systems. Although the connection between the habitational and genetic features is unknown, these findings suggest that tetrathionate reductase-type protein, as well as proteins encoded by the YTD gene cluster, is one of the key proteins in sulfur disproportionation, especially for species inhabiting thermal environments.
Overall, the physiological and proteomic analyses of a novel sulfur-disproportionating Desulfobulbaceae species strain GF1T provide a key insight into a unique involvement of tetrathionate reductase-type protein and proteins encoded by the YTD gene cluster in thiosulfate disproportionation. Furthermore, the identification of orthologs in this study will reinforce the hypothetical common metabolism of sulfur-disproportionating populations in thermal environments. Our findings provide a steppingstone toward elucidating the diversity of sulfur disproportionating bacteria and the genetic and metabolic evolution underlying sulfur disproportionation.
Description of Desulfolithobacter gen. nov
Desulfolithobacter (De.sul.fo.li.tho.bac’ter. L. prep. de, from; L. neut. n. sulfur, sulfur; Gr. masc. n. lithos, stone; N.L. masc. n. bacter, rod; N.L. masc. n. Desulfolithobacter, a rod-shaped lithotrophic sulfate-reducer). Motile rods that stain Gram-negative. Strictly anaerobic. Mesophilic. Chemolithoautotrophic. Able to utilize molecular hydrogen as an electron donor and sulfate as an electron acceptor. NaCl is absolutely required for growth. The G + C content of genomic DNA is about 57%. Major cellular fatty acids are C16:0, C18:1, C16:1, and C18:0. Based on the 16S rRNA gene sequence, the genus Desulfolithobacter is distantly related to the genus Desulfogranum. Members of the genus Desulfolithobacter occur in deep-sea hydrothermal fields. The type species is Desulfolithobacter dissulfuricans.
Description of Desulfolithobacter dissulfuricans sp. nov
Desulfolithobacter dissulfuricans (dis.sul. fu’ri.cans Gr. adv. Dis, in two; L. neut. n. sulfur, sulfur; N.L. part. Adj. dissulfuricans, disproportionating sulfur). Cells are motile, Gram-negative, short rods, and ~1.0–2.1 μm in length and 0.5–1.1 μm in width. Growth occurs between 25°C and 50°C, 20 and 40 g L−1 NaCl, and pH 6.1 and 6.8. Cells are strictly anaerobic. Chemolithoautotrophic growth occurs with H₂, formate, casamino acids, or malate as the electron donor and sulfate, sulfite, or thiosulfate as the electron acceptor. S0, thiosulfate, and tetrathionate are disproportionated. The major cellular fatty acids are C16:0, C18:1, C16:1, and C18:0. The major respiratory quinone is MK-8. The G + C DNA content is 56.9%. The type strain, GF1ᵀ (=JCM 34117T = DSM 111414T), was isolated from the deep-sea hydrothermal vent chimney obtained from the Suiyo Seamount in the Izu-Bonin Arc, Japan.
Data availability statement
The data presented in the study are deposited in the ProteomeXchange Consortium via the jPOST partner repository (https://jpostdb.org/), accession numbers JPST001840 and PXD036606 (Okuda et al., 2017), and in the GenBank/EMBL/DDBJ repository, accession numbers LC596831 and AP024233.
Author contributions
YH, JM, KT, and SN designed this study. YH performed all the experiments except for the transmission electron microscope (TEM) observations and LC–MS/MS analysis. AT performed the TEM observations. YH and SSh prepared the samples for LC–MS/MS analysis. SSh conducted LC–MS/MS analysis. All authors contributed to the article and approved the submitted version.
Funding
This work was partially supported by JSPS KAKENHI grant numbers JP 16H04843, 20H03322, and 19K05783.
Acknowledgments
We are very grateful to the R/V Yokosuka crew and the DSV Shinkai 6500 operation team for helping us to collect samples from the deep-sea hydrothermal environment. We are also thankful to those who supported ‘GACHINKO Cruise’ project of JAMSTEC. We thank Masayuki Miyazaki of JAMSTEC for helpful assistance with a high-pressure liquid chromatography analysis. We also thank Wataru Takahagi of JAMSTEC for help with programming support.
Conflict of interest
AT belongs to Depertment of Marine and Earth Sciences, Marine Works Japan Ltd.
The remaining authors declare that the research was conducted in the absence of any commercial or financial relationships that could be construed as a potential conflict of interest.
Publisher’s note
All claims expressed in this article are solely those of the authors and do not necessarily represent those of their affiliated organizations, or those of the publisher, the editors and the reviewers. Any product that may be evaluated in this article, or claim that may be made by its manufacturer, is not guaranteed or endorsed by the publisher.
Supplementary material
The Supplementary material for this article can be found online at: https://www.frontiersin.org/articles/10.3389/fmicb.2022.1042116/full#supplementary-material
Abbreviations
ANI, average nucleotide identity; AAI, average amino acid identity; PSMs, peptide spectrum matches; NSAFs, normalized spectral abundance factors; TD, thiosulfate-disproportionating condition; SR, sulfate-reducing condition.
Footnotes
References
Adam, N., Han, Y., Laufer-Meiser, K., Bährle, R., Schwarz-Schampera, U., Schippers, A., et al. (2021). Deltaproteobacterium strain KaireiS1, a mesophilic, hydrogen-oxidizing and sulfate-reducing bacterium from an inactive deep-sea hydrothermal chimney. Front. Microbiol. 12:686276. doi: 10.3389/fmicb.2021.686276
Altschul, S. F., Madden, T. L., Schäffer, A. A., Zhang, J., Zhang, Z., Miller, W., et al. (1997). Gapped BLAST and PSI-BLAST: a new generation of protein database search programs. Nucleic Acids Res. 25, 3389–3402. doi: 10.1093/nar/25.17.3389
Aziz, R. K., Bartels, D., Best, A. A., DeJongh, M., Disz, T., Edwards, R. A., et al. (2008). The RAST server: rapid annotations using subsystems technology. BMC Genomics 9:75. doi: 10.1186/1471-2164-9-75
Bak, F., and Cypionka, H. (1987). A novel type of energy metabolism involving fermentation of inorganic Sulphur compounds. Nature 326, 891–892. doi: 10.1038/326891a0
Bak, F., and Pfennig, N. (1987). Chemolithotrophic growth of Desulfovibrio sulfodismutans sp. nov. by disproportionation of inorganic sulfur compounds. Arch. Microbiol. 147, 184–189. doi: 10.1007/BF00415282
Bak, F., Schuhmann, A., and Jansen, K.-H. (1993). Determination of tetrathionate and thiosulfate in natural samples and microbial cultures by a new, fast and sensitive ion chromatographic technique. FEMS Microbiol. Ecol. 12, 257–264. doi: 10.1111/j.1574-6941.1993.tb00038.x
Balk, M., Altinbaş, M., Rijpstra, W. I. C., Damsté, J. S. S., and Stams, A. J. M. (2008). Desulfatirhabdium butyrativorans gen. nov., sp. nov., a butyrate-oxidizing, sulfate-reducing bacterium isolated from an anaerobic bioreactor. Int. J. Syst. Evol. Microbiol. 58, 110–115. doi: 10.1099/ijs.0.65396-0
Baross, J. A., and Deming, J. W. (1995). “Growth at high temperatures: isolation and taxonomy, physiology, and ecology,” in The Microbiology of Deep-Sea Hydrothermal Vents. ed. D. M. Karl (Boca Raton: CRC Press), 169–217.
Collins, M. D., and Widdel, F. (1986). Respiratory Quinones of Sulphate-reducing and Sulphur-reducing bacteria: a systematic investigation. Syst. Appl. Microbiol. 8, 8–18. doi: 10.1016/S0723-2020(86)80141-2
Dahl, C. (2020). “Chapter 3 a biochemical view on the biological sulfur cycle,” in Environmental Technologies to Treat Sulphur Pollution: Principles and Engineering. ed. P. N. L. Lens (London, United Kingdom: IWA Publishing), 55–96.
Dick, G. J. (2019). The microbiomes of deep-sea hydrothermal vents: distributed globally, shaped locally. Nat. Rev. Microbiol. 17, 271–283. doi: 10.1038/s41579-019-0160-2
Duval, S., Ducluzeau, A. L., Nitschke, W., and Schoepp-Cothenet, B. (2008). Enzyme phylogenies as markers for the oxidation state of the environment: the case of respiratory arsenate reductase and related enzymes. BMC Evol. Biol. 8:206. doi: 10.1186/1471-2148-8-206
Elsgaard, L., Guezennec, J., Benbouzid-Rollet, N., and Prieur, D. (1995). Mesophilic sulfate-reducing bacteria from three deep-sea hydrothermal vent sites. Oceanol. Acta 18, 95–104.
Emms, D. M., and Kelly, S. (2015). OrthoFinder: solving fundamental biases in whole genome comparisons dramatically improves orthogroup inference accuracy. Genome Biol. 16:157. doi: 10.1186/s13059-015-0721-2
Emms, D. M., and Kelly, S. (2019). OrthoFinder: phylogenetic orthology inference for comparative genomics. Genome Biol. 20:238. doi: 10.1186/s13059-019-1832-y
Finster, K. (2008). Microbiological disproportionation of inorganic sulfur compounds. J. Sulfur Chem. 29, 281–292. doi: 10.1080/17415990802105770
Finster, K. W., Kjeldsen, K. U., Kube, M., Reinhardt, R., Mussmann, M., Amann, R., et al. (2013). Complete genome sequence of Desulfocapsa sulfexigens, a marine deltaproteobacterium specialized in disproportionating inorganic sulfur compounds. Stand. Genomic Sci. 8, 58–68. doi: 10.4056/sigs.3777412
Finster, K., Liesack, W., and Thamdrup, B. (1998). Elemental sulfur and thiosulfate disproportionation by Desulfocapsa sulfoexigens sp. nov., a new anaerobic bacterium isolated from marine surface sediment. Appl. Environ. Microbiol. 64, 119–125. doi: 10.1128/AEM.64.1.119-125.1998
Florentino, A. P., Pereira, I. A. C., Boeren, S., van den Born, M., Stams, A. J. M., and Sánchez-Andrea, I. (2019). Insight into the sulfur metabolism of Desulfurella amilsii by differential proteomics. Environ. Microbiol. 21, 209–225. doi: 10.1111/1462-2920.14442
Fossing, H., and Jørgensen, B. B. (1990). Oxidation and reduction of radiolabeled inorganic sulfur compounds in an estuarine sediment, Kysing Fjord, Denmark. Geochim. Cosmochim. Acta 54, 2731–2742. doi: 10.1016/0016-7037(90)90008-9
Frederiksen, T.-M., and Finster, K. (2003). Sulfite-oxido-reductase is involved in the oxidation of sulfite in Desulfocapsa sulfoexigens during disproportionation of thiosulfate and elemental sulfur. Biodegradation 14, 189–198. doi: 10.1023/A:1024255830925
Frederiksen, T. M., and Finster, K. (2004). The transformation of inorganic sulfur compounds and the assimilation of organic and inorganic carbon by the sulfur disproportionating bacterium Desulfocapsa sulfoexigens. Antonie Van Leeuwenhoek 85, 141–149. doi: 10.1023/B:ANTO.0000020153.82679.f4
Friedrich, M., Springer, N., Ludwig, W., and Schink, B. (1996). Phylogenetic positions of Desulfofustis glycolicus gen. nov., sp. nov., and Syntrophobotulus glycolicus gen. nov., sp. nov., two new strict anaerobes growing with glycolic acid. Int. J. Syst. Bacteriol. 46, 1065–1069. doi: 10.1099/00207713-46-4-1065
Frolova, A. A., Slobodkina, G. B., Baslerov, R. V., Novikov, A. A., Bonch-Osmolovskaya, E. A., and Slobodkin, A. I. (2018). Thermosulfurimonas marina sp. nov., an autotrophic sulfur-disproportionating and nitrate-reducing bacterium isolated from a shallow-sea hydrothermal vent. Microbiology 87, 502–507. doi: 10.1134/S0026261718040082
Galushko, A., and Kuever, J. (2019). “Desulfovibrio” in Bergey’s Manual of Systematics of Archaea and Bacteria. eds. M. E. Trujillo, S. Dedysh, P. DeVos, B. Hedlund, P. Kämpfer, and F. A. Rainey, et al. (Hoboken, New Jersey: John Wiley & Sons, Ltd).
Galushko, A., and Kuever, J. (2020). “Desulfogranum gen. nov” in Bergey’s Manual of Systematics of Archaea and Bacteria. eds. M. E. Trujillo, S. Dedysh, P. DeVos, B. Hedlund, P. Kämpfer, and F. A. Rainey, et al. (Hoboken, New Jersey: John Wiley & Sons, Ltd).
Gittel, A., Kofoed, M. V. W., Sørensen, K. B., Ingvorsen, K., and Schramm, A. (2012). Succession of Deferribacteres and Epsilonproteobacteria through a nitrate-treated high-temperature oil production facility. Syst. Appl. Microbiol. 35, 165–174. doi: 10.1016/j.syapm.2012.01.003
Goris, J., Konstantinidis, K. T., Klappenbach, J. A., Coenye, T., Vandamme, P., and Tiedje, J. M. (2007). DNA-DNA hybridization values and their relationship to whole-genome sequence similarities. Int. J. Syst. Evol. Microbiol. 57, 81–91. doi: 10.1099/ijs.0.64483-0
Hashimoto, Y., Tame, A., Sawayama, S., Miyazaki, J., Takai, K., and Nakagawa, S. (2021). Desulfomarina profundi gen. nov., sp. nov., a novel mesophilic, hydrogen-oxidizing, sulphate-reducing chemolithoautotroph isolated from a deep-sea hydrothermal vent chimney. Int. J. Syst. Evol. Microbiol. 71:005083. doi: 10.1099/ijsem.0.005083
Hensel, M., Hinsley, A. P., Nikolaus, T., Sawers, G., and Berks, B. C. (1999). The genetic basis of tetrathionate respiration in salmonella typhimurium. Mol. Microbiol. 32, 275–287. doi: 10.1046/j.1365-2958.1999.01345.x
Janssen, P. H., Schuhmann, A., Bak, F., and Liesack, W. (1996). Disproportionation of inorganic sulfur compounds by the sulfate-reducing bacterium Desulfocapsa thiozymogenes gen. nov., sp. nov. Arch. Microbiol. 166, 184–192. doi: 10.1007/s002030050374
Jørgensen, B. B. (1990). A thiosulfate shunt in the sulfur cycle of marine sediments. Science 249, 152–154. doi: 10.1126/science.249.4965.152
Kawai, S., Shimamura, S., Shimane, Y., and Tsukatani, Y. (2022). Proteomic time-course analysis of the filamentous anoxygenic phototrophic bacterium, Chloroflexus aurantiacus, during the transition from respiration to phototrophy. Microorganisms 10:1288. doi: 10.3390/microorganisms10071288
Kelley, D. S., Baross, J. A., and Delaney, J. R. (2002). Volcanoes, fluids, and life at mid-ocean ridge spreading centers. Annu. Rev. Earth Planet. Sci. 30, 385–491. doi: 10.1146/annurev.earth.30.091201.141331
Konstantinidis, K. T., Rosselló-Móra, R., and Amann, R. (2017). Uncultivated microbes in need of their own taxonomy. ISME J. 11, 2399–2406. doi: 10.1038/ismej.2017.113
Kostka, J., and Nealson, K. (1998). “Isolation, cultivation and characterization of iron-and manganese-reducing bacteria,” in Techniques in Microbial Ecology. eds. R. S. Burlage, R. Atlas, D. Stahl, G. Geesey, and G. Sayler (Oxford, New York: Oxford University Press), 58–78.
Krämer, M., and Cypionka, H. (1989). Sulfate formation via ATP sulfurylase in thiosulfate-and sulfite-disproportionating bacteria. Arch. Microbiol. 151, 232–237. doi: 10.1007/BF00413135
Kumar, S., Stecher, G., Li, M., Knyaz, C., and Tamura, K. (2018). MEGA X: molecular evolutionary genetics analysis across computing platforms. Mol. Biol. Evol. 35, 1547–1549. doi: 10.1093/molbev/msy096
Lee, I., Kim, Y. O., Park, S. C., and Chun, J. (2016). OrthoANI: an improved algorithm and software for calculating average nucleotide identity. Int. J. Syst. Evol. Microbiol. 66, 1100–1103. doi: 10.1099/ijsem.0.000760
Liu, L. J., Stockdreher, Y., Koch, T., Sun, S. T., Fan, Z., Josten, M., et al. (2014). Thiosulfate transfer mediated by DsrE/TusA homologs from acidothermophilic sulfur-oxidizing archaeon Metallosphaera cuprina. J. Biol. Chem. 289, 26949–26959. doi: 10.1074/jbc.M114.591669
Lovley, D. R., and Phillips, E. J. P. (1994). Novel processes for anaerobic sulfate production from elemental sulfur by sulfate-reducing bacteria. Appl. Environ. Microbiol. 60, 2394–2399. doi: 10.1128/aem.60.7.2394-2399.1994
Mardanov, A. V., Beletsky, A. V., Kadnikov, V. V., Slobodkin, A. I., and Ravin, N. V. (2016). Genome analysis of Thermosulfurimonas dismutans, the first thermophilic sulfur-disproportionating bacterium of the phylum Thermodesulfobacteria. Front. Microbiol. 7:950. doi: 10.3389/fmicb.2016.00950
Muramatsu, F., Tonomura, M., Yamada, M., Kasahara, Y., Yamamura, S., Iino, T., et al. (2020). Possible involvement of a tetrathionate reductase homolog in dissimilatory arsenate reduction by Anaeromyxobacter sp. strain PSR-1. Appl. Environ. Microbiol. 86, e00829–e00820. doi: 10.1128/AEM.00829-20
Nagata, R., Takaki, Y., Tame, A., Nunoura, T., Muto, H., Mino, S., et al. (2017). Lebetimonas natsushimae sp. nov., a novel strictly anaerobic, moderately thermophilic chemoautotroph isolated from a deep-sea hydrothermal vent polychaete nest in the mid-Okinawa trough. Syst. Appl. Microbiol. 40, 352–356. doi: 10.1016/j.syapm.2017.06.002
Nakagawa, T., Nakagawa, S., Inagaki, F., Takai, K., and Horikoshi, K. (2004). Phylogenetic diversity of sulfate-reducing prokaryotes in active deep-sea hydrothermal vent chimney structures. FEMS Microbiol. Lett. 232, 145–152. doi: 10.1016/S0378-1097(04)00044-8
Nakagawa, S., and Takai, K. (2006). 3 the isolation of thermophiles from deep-sea hydrothermal environments. Methods Microbiol. 35, 55–91. doi: 10.1016/S0580-9517(08)70006-0
Nakagawa, S., and Takai, K. (2008). Deep-sea vent chemoautotrophs: diversity, biochemistry and ecological significance. FEMS Microbiol. Ecol. 65, 1–14. doi: 10.1111/j.1574-6941.2008.00502.x
Nakamura, K., and Takai, K. (2014). Theoretical constraints of physical and chemical properties of hydrothermal fluids on variations in chemolithotrophic microbial communities in seafloor hydrothermal systems. Prog Earth Planet Sci 1:5. doi: 10.1186/2197-4284-1-5
Okuda, S., Watanabe, Y., Moriya, Y., Kawano, S., Yamamoto, T., Matsumoto, M., et al. (2017). jPOSTrepo: an international standard data repository for proteomes. Nucleic Acids Res. 45, D1107–D1111. doi: 10.1093/nar/gkw1080
Porter, K. G., and Feig, Y. S. (1980). The use of DAPI for identifying and counting aquatic microflora. Limnol. Oceanogr. 25, 943–948. doi: 10.4319/lo.1980.25.5.0943
Richter, M., and Rosselló-Móra, R. (2009). Shifting the genomic gold standard for the prokaryotic species definition. Proc. Natl. Acad. Sci. U. S. A. 106, 19126–19131. doi: 10.1073/pnas.0906412106
Rowe, A. R., Chellamuthu, P., Lam, B., Okamoto, A., and Nealson, K. H. (2015). Marine sediments microbes capable of electrode oxidation as a surrogate for lithotrophic insoluble substrate metabolism. Front. Microbiol. 5:784. doi: 10.3389/fmicb.2014.00784
Sako, Y., Takai, K., Ishida, Y., Uchida, A., and Katayama, Y. (1996). Rhodothermus obamensis sp. nov., a modern lineage of extremely thermophilic marine bacteria. Int. J. Syst. Bacteriol. 46, 1099–1104. doi: 10.1099/00207713-46-4-1099
Santos, A. A., Venceslau, S. S., Grein, F., Leavitt, W. D., Dahl, C., Johnston, D. T., et al. (2015). A protein trisulfide couples dissimilatory sulfate reduction to energy conservation. Science 350, 1541–1545. doi: 10.1126/science.aad3558
Sass, A., Rütters, H., Cypionka, H., and Sass, H. (2002). Desulfobulbus mediterraneus sp. nov., a sulfate-reducing bacterium growing on mono-and disaccharides. Arch. Microbiol. 177, 468–474. doi: 10.1007/s00203-002-0415-5
Sievert, S. M., Hügler, M., Taylor, C. D., and Wirsen, C. O. (2008). “Sulfur oxidation at deep-sea hydrothermal vents,” in Microbial Sulfur Metabolism. eds. C. Dahl and C. G. Friedrich (Berlin, Heidelberg: Springer), 238–258.
Slobodkin, A. I., Reysenbach, A. L., Slobodkina, G. B., Baslerov, R. V., Kostrikina, N. A., Wagner, I. D., et al. (2012). Thermosulfurimonas dismutans gen. nov., sp. nov., an extremely thermophilic sulfur-disproportionating bacterium from a deep-sea hydrothermal vent. Int. J. Syst. Evol. Microbiol. 62, 2565–2571. doi: 10.1099/ijs.0.034397-0
Slobodkin, A. I., Reysenbach, A. L., Slobodkina, G. B., Kolganova, T. V., Kostrikina, N. A., and Bonch-Osmolovskaya, E. A. (2013). Dissulfuribacter thermophilus gen. nov., sp. nov., a thermophilic, autotrophic, sulfur-disproportionating, deeply branching deltaproteobacterium from a deep-sea hydrothermal vent. Int. J. Syst. Evol. Microbiol. 63, 1967–1971. doi: 10.1099/ijs.0.046938-0
Slobodkin, A. I., and Slobodkina, G. B. (2019). Diversity of sulfur-disproportionating microorganisms. Microbiology 88, 509–522. doi: 10.1134/S0026261719050138
Slobodkin, A. I., Slobodkina, G. B., Panteleeva, A. N., Chernyh, N. A., Novikov, A. A., and Bonch-Osmolovskaya, E. A. (2016). Dissulfurimicrobium hydrothermale gen. nov., sp. nov., a thermophilic, autotrophic, sulfur-disproportionating deltaproteobacterium isolated from a hydrothermal pond. Int. J. Syst. Evol. Microbiol. 66, 1022–1026. doi: 10.1099/ijsem.0.000828
Slobodkina, G. B., Reysenbach, A. L., Kolganova, T. V., Novikov, A. A., Bonch-Osmolovskaya, E. A., and Slobodkin, A. I. (2017). Thermosulfuriphilus ammonigenes gen. nov., sp. nov., a thermophilic, chemolithoautotrophic bacterium capable of respiratory ammonification of nitrate with elemental sulfur. Int. J. Syst. Evol. Microbiol. 67, 3474–3479. doi: 10.1099/ijsem.0.002142
Søndergaard, D., Pedersen, C. N. S., and Greening, C. (2016). HydDB: a web tool for hydrogenase classification and analysis. Sci. Rep. 6:34212. doi: 10.1038/srep34212
Stockdreher, Y., Sturm, M., Josten, M., Sahl, H. G., Dobler, N., Zigann, R., et al. (2014). New proteins involved in sulfur trafficking in the cytoplasm of Allochromatium vinosum. J. Biol. Chem. 289, 12390–12403. doi: 10.1074/jbc.M113.536425
Stockdreher, Y., Venceslau, S. S., Josten, M., Sahl, H. G., Pereira, I. A. C., and Dahl, C. (2012). Cytoplasmic sulfurtransferases in the purple sulfur bacterium Allochromatium vinosum: evidence for sulfur transfer from DsrEFH to DsrC. PLoS One 7:e40785. doi: 10.1371/journal.pone.0040785
Tanabe, T. S., Leimkühler, S., and Dahl, C. (2019). “Chapter seven – the functional diversity of the prokaryotic sulfur carrier protein TusA,” in Advances in Microbial Physiology. ed. R. K. Poole (London, United Kingdom: Academic Press), 233–277.
Tanizawa, Y., Fujisawa, T., and Nakamura, Y. (2018). DFAST: a flexible prokaryotic genome annotation pipeline for faster genome publication. Bioinformatics 34, 1037–1039. doi: 10.1093/bioinformatics/btx713
Thorup, C., Schramm, A., Findlay, A. J., Finster, K. W., and Schreiber, L. (2017). Disguised as a sulfate reducer: growth of the deltaproteobacterium Desulfurivibrio alkaliphilus by sulfide oxidation with nitrate. MBio 8, e00671–e00617. doi: 10.1128/mBio.00671-17
Umezawa, K., Kojima, H., Kato, Y., and Fukui, M. (2020). Disproportionation of inorganic sulfur compounds by a novel autotrophic bacterium belonging to Nitrospirota. Syst. Appl. Microbiol. 43:126110. doi: 10.1016/j.syapm.2020.126110
Umezawa, K., Kojima, H., Kato, Y., and Fukui, M. (2021). Dissulfurispira thermophila gen. nov., sp. nov., a thermophilic chemolithoautotroph growing by sulfur disproportionation, and proposal of novel taxa in the phylum Nitrospirota to reclassify the genus Thermodesulfovibrio. Syst. Appl. Microbiol. 44:126184. doi: 10.1016/j.syapm.2021.126184
Venceslau, S. S., Stockdreher, Y., Dahl, C., and Pereira, I. A. C. (2014). The “bacterial heterodisulfide,” DsrC is a key protein in dissimilatory sulfur metabolism. Biochim. Biophys. Acta Bioenerg. 1837, 1148–1164. doi: 10.1016/j.bbabio.2014.03.007
Widdel, F., Kohring, G.-W., and Mayer, F. (1983). Studies on dissimilatory sulfate-reducing bacteria that decompose fatty acids III. Characterization of the filamentous gliding Desulfonema limicola gen. nov. sp. nov., and Desulfonema magnum sp. nov. Arch. Microbiol. 134, 286–294. doi: 10.1007/bf00407804
Widdel, F., and Pfennig, N. (1982). Studies on dissimilatory sulfate-reducing bacteria that decompose fatty acids II. Incomplete oxidation of propionate by Desulfobulbus propionicus gen. nov., sp. nov. Arch. Microbiol. 131, 360–365. doi: 10.1007/BF00411187
Wu, Y.-W. (2018). ezTree: an automated pipeline for identifying phylogenetic marker genes and inferring evolutionary relationships among uncultivated prokaryotic draft genomes. BMC Genomics 19:921. doi: 10.1186/s12864-017-4327-9
Yarza, P., Yilmaz, P., Pruesse, E., Glöckner, F. O., Ludwig, W., Schleifer, K. H., et al. (2014). Uniting the classification of cultured and uncultured bacteria and archaea using 16S rRNA gene sequences. Nat. Rev. Microbiol. 12, 635–645. doi: 10.1038/nrmicro3330
Yu, N. Y., Wagner, J. R., Laird, M. R., Melli, G., Rey, S., Lo, R., et al. (2010). PSORTb 3.0: improved protein subcellular localization prediction with refined localization subcategories and predictive capabilities for all prokaryotes. Bioinformatics 26, 1608–1615. doi: 10.1093/bioinformatics/btq249
Yvenou, S., Allioux, M., Slobodkin, A., Slobodkina, G., Jebbar, M., and Alain, K. (2022). Genetic potential of Dissulfurimicrobium hydrothermale, an obligate sulfur-disproportionating thermophilic microorganism. Microorganisms 10:60. doi: 10.3390/microorganisms10010060
Zeng, X., Alain, K., and Shao, Z. (2021). Microorganisms from deep-sea hydrothermal vents. Mar. Life Sci. Technol. 3, 204–230. doi: 10.1007/s42995-020-00086-4
Keywords: sulfur disproportionation, Deltaproteobacteria, Desulfobulbaceae, deep-sea hydrothermal vent, comparative proteomic analysis, chemolithoautotroph
Citation: Hashimoto Y, Shimamura S, Tame A, Sawayama S, Miyazaki J, Takai K and Nakagawa S (2022) Physiological and comparative proteomic characterization of Desulfolithobacter dissulfuricans gen. nov., sp. nov., a novel mesophilic, sulfur-disproportionating chemolithoautotroph from a deep-sea hydrothermal vent. Front. Microbiol. 13:1042116. doi: 10.3389/fmicb.2022.1042116
Edited by:
Inês A. Cardoso Pereira, Universidade Nova de Lisboa, PortugalReviewed by:
Kai Waldemar Finster, Aarhus University, DenmarkMichael Hügler, Water Technology Center (TZW), Technologiezentrum Wasser Germany
Barbara Schoepp-Cothenet, Centre National de la Recherche Scientifique, France
Copyright © 2022 Hashimoto, Shimamura, Tame, Sawayama, Miyazaki, Takai and Nakagawa. This is an open-access article distributed under the terms of the Creative Commons Attribution License (CC BY). The use, distribution or reproduction in other forums is permitted, provided the original author(s) and the copyright owner(s) are credited and that the original publication in this journal is cited, in accordance with accepted academic practice. No use, distribution or reproduction is permitted which does not comply with these terms.
*Correspondence: Satoshi Nakagawa, bmFrYWdhd2Euc2F0b3NoaS43dUBreW90by11LmFjLmpw