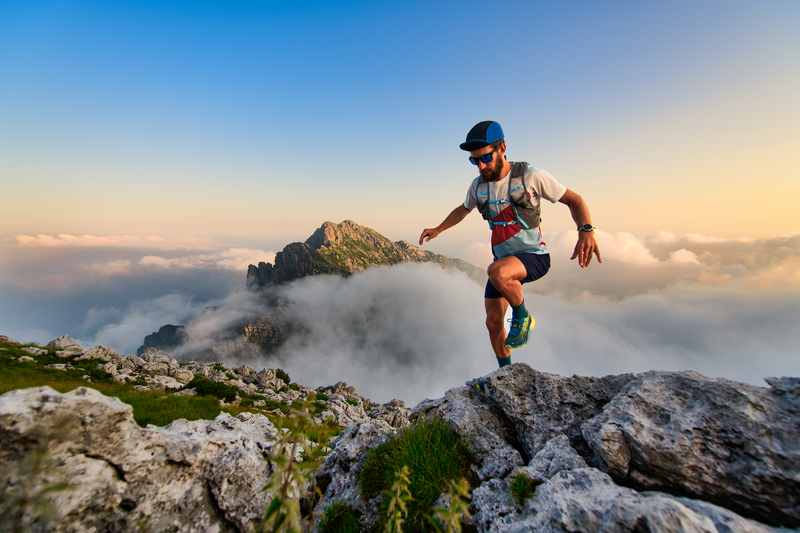
94% of researchers rate our articles as excellent or good
Learn more about the work of our research integrity team to safeguard the quality of each article we publish.
Find out more
ORIGINAL RESEARCH article
Front. Microbiol. , 08 November 2022
Sec. Microbial Symbioses
Volume 13 - 2022 | https://doi.org/10.3389/fmicb.2022.1031535
This article is part of the Research Topic Microbial Associates of Blood-Sucking Arthropods and Other Animals: Relevance to Their Physiology, Ecology and Evolution View all 12 articles
The poultry red mite Dermanyssus gallinae poses a significant threat to the health of hens and poultry production. A comprehensive understanding of D. gallinae is necessary to develop sustainable and efficacious control methods. Here we examined 144 D. gallinae collected from 18 poultry farms throughout the Japanese Archipelago for their genetic variations based on mitochondrial cytochrome c oxidase subunit I (COI) sequences, and microbiome variations based on amplicon sequencing of the 16S ribosomal RNA gene. According to COI sequencing, the Japanese samples were categorized into three haplogroups, which did not reflect the geographical distribution. Microbiome analyses found that the major bacteria associated with D. gallinae were Bartonella, Cardinium, Wolbachia, and Tsukamurella, with Bartonella being most predominant. Among 144 individual mites, all possessed one of the two major types of Bartonella (Bartonella sp. A), while 140 mites possessed the other type (Bartonella sp. B). The presence of the two strains of Bartonella was also confirmed by a single copy gene, rpoB. The presence of Bartonella in laid eggs suggested transovarial vertical transmission. Given that obligate blood-feeding arthropods generally require a supply of B vitamins from symbiotic bacteria, Bartonella may play an important role in mite survival. Rickettsiella, a major symbiont in European D. gallinae populations, and suggested to be an important symbiont by genomic data, was rarely found in Japanese populations. Cardinium detected from D. gallinae fell into a major clade found widely in arthropods, whereas Wolbachia detected in Japanese D. gallinae appear to be a new lineage, located at the base of Wolbachia phylogeny. Of the mitochondrial phylogeny, infection patterns of Cardinium and Wolbachia were strongly correlated, possibly suggesting one or both of the symbionts induce reproductive manipulations and increase spread in the host populations.
The poultry red mite Dermanyssus gallinae (Acari: Dermanyssidae), an obligate blood-feeding ectoparasite that feeds on avian blood, is globally distributed (Chauve, 1998; Sparagano et al., 2009; Wang et al., 2010), and is endemic in many commercial poultry farms, with 80%–90% of egg-laying facilities being infested (Sparagano et al., 2009; George et al., 2015). Once these mites invade a poultry house, their numbers can increase dramatically because typical conditions within poultry houses (high temperature and humidity) are ideal for D. gallinae. The densities of D. gallinae often reach up to 50,000 mites per bird. In extreme cases when densities reach 500,000 mites per bird, a hen can lose more than 3% of its blood volume every night (Kilpinen et al., 2005). Such heavy mite infestations seriously impact hen health and welfare, resulting in anemia and irritation, and can cause a 10-fold increase in hen mortality (Sigognault Flochlay et al., 2017). Predictably, D. gallinae causes a significant reduction in both egg quality and production (Kilpinen et al., 2005; Sparagano et al., 2014). In Europe, D. gallinae infestation costs the poultry industry over €231 million annually (Sigognault Flochlay et al., 2017). Furthermore, the prevalence of D. gallinae is expected to increase due to increasing acaricide resistance, climate change, and the lack of a sustainable and efficacious approach to control infestations (Chauve, 1998; Nordenfors et al., 2001). Increasing fundamental knowledge of D. gallinae can provide insights into new control methods.
Obligatory hematophagy, the practice of feeding exclusively on blood throughout all life stages, is found in a variety of arthropods. Because blood is nutritionally unbalanced, with high levels of protein, iron, and salt, but few carbohydrates, lipids, or vitamins, obligatorily hematophagous arthropods typically rely on symbiotic bacteria to obtain B vitamins (Husnik, 2018; Duron and Gottlieb, 2020). For example, tsetse flies Glossina spp. and the bed bug Cimex lectularius depend on their endosymbionts Wigglesworthia glossinidia and Wolbachia for their B vitamin supplies, respectively (Akman et al., 2002; Hosokawa et al., 2010; Michalkova et al., 2014; Nikoh et al., 2014; Moriyama et al., 2015). However, a controversy exists over vitamin-supplying symbiotic bacteria of the obligatory hematophagous D. gallinae. Initially, Rickettsia was suggested to be a symbiotic bacterium of D. gallinae in France using PCR amplification and fingerprinting methods (De Luna et al., 2009; Moro et al., 2009). According to Hubert et al. (2017), however, Bartonella-like bacteria rather than Rickettsia were considered as the mutualistic symbionts of D. gallinae because Bartonella-like bacteria was found in four of four sampling sites in Czechia, and in all stadia including eggs by amplicon sequencing. In contrast, another study showed that Rickettsiella was widespread in Europe and pseudogenized for many genes, including those involved in the amino acid synthesis pathway, but had an almost full set of genes for B vitamins biosynthesis (Price et al., 2021). In the aforementioned Czech study (Hubert et al., 2017), Rickettsiella was found in samples from only one of the four sites. Considering these discrepancies, this study investigated the genetic variations and microbiome variations of D. galline in Japanese poultry populations.
In total, 144 individual D. gallinae mites collected from 18 poultry farms in 16 Japanese prefectures were brought to the laboratory alive and preserved in 99.5% ethanol at 4°C until DNA extraction was performed (Figure 1; Supplementary Table 1).
Figure 1. (Upper) Ventral view of adult D. gallinae. (Lower) A map of the Japanese archipelago. Prefectures where the mites were sampled are depicted in yellow. Each number represents a poultry farm. The exact location of each poultry farm is concealed.
After being crushed using an EOG-sterilized BioMasher II (Nippi, Inc., Tokyo, Japan), DNA was extracted from the whole body of each adult D. gallinae using a DNeasy Blood and Tissue Kit (Qiagen, N.V., Venlo, Netherlands) with 50 μl of EB buffer. The DNA samples were stored at −30°C until used. PCR was conducted for mitochondrial cytochrome c oxidase subunit I (COI) using KOD FX Neo (Toyobo Co. Ltd., Osaka, Japan), and the primers FCOIDG and RCOIDG designed for COI of D. gallinae (Øines and Brännström, 2011). For 16S ribosomal RNA sequencing of symbiont bacteria, PCR was conducted using Ex Taq HS (Takara Bio Inc., Kusatsu, Japan) and two sets of universal primers, 10F-1507R or 10FF-1515R. The PCR fragment was cloned using pGEM-T Easy Vector (Promega, Madison, WI, USA), Mighty mix for DNA ligation (Takara), and Escherichia coli DH5α competent cells (Takara). Plasmids were extracted using a QIAprep Spin miniprep kit (Qiagen), the purified plasmids were subjected to sequencing reactions using a BigDye Terminator v3.1 Cycle Sequencing Kit (Thermo Fisher Scientific, Waltham, MA, USA) and the sequencing primers M13F or M13RV in the flanking regions of the vector along with the bacterial universal primer 16SA2. The primer sequences are shown in Supplementary Table 2.
The molecular phylogenetic analyses were conducted utilizing maximum-likelihood estimation methods using MEGA: Molecular Evolutionary Genetics Analysis, ver. 5.2 software (Tamura et al., 2011) with 1,000 bootstrap replications. The optimum model was selected through model tests to describe each phylogenetic tree. To analyze the genetic diversity of COI sequences, a network diagram of COI haplotypes was drawn using TCS 1.21 software (Templeton et al., 1992).
To analyze the D. gallinae microbiome, hypervariable V3/V4 regions of the 16S rRNA gene were sequenced. The libraries created by using 2-step tailed PCR (Supplementary Table 2) were checked using a Synergy H1 (BioTek, Winooski, VT, USA) and a QuantiFluor dsDNA System (Promega), and the qualities were verified using a Fragment Analyzer (Agilent, Santa Clara, CA, USA) and a dsDNA 915 Reagent Kit (Agilent). These libraries were sequenced using a Miseq sequencer (Illumina, Inc., San Diego, CA USA), and the raw data were deposited in the GenBank sequence database (Accession No. DRR376882–DRR377025).
The raw amplicon sequences were individually demultiplexed and converted to FASTQ files, which were then analyzed using the Quantitative Insights Into Microbial Ecology (QIIME2) v.2020.06 pipelines (Bolyen et al., 2019). Denoising and quality control, quality filtering, correction of errors in marginal sequences, removal of chimeric sequences, removal of singletons, joining of paired-end reads, and dereplication were conducted using the DADA2 plugin with the following option: -p-trim-left-f 10 -p-trim-left-r 10 -p-trunc-len-f 270 -p-trunc-len-r 270 (Callahan et al., 2016). The DADA2 algorithm was also used to cluster representative amplicon sequence variants and to provide count frequencies for each sample. Taxonomy classification was assigned to the representative amplicon sequence variants based on the Silva v.128 99% operational taxonomic units (OTU) reference sequences1, and the GreenGenes V.13_8 99% OTU reference sequences2 databases at 97% OTU level, trained using a Naïve Bayes classifier (classify-sklearn) and the q2-feature-classifier QIIME2 plugin (McDonald et al., 2012; Quast et al., 2013; Bokulich et al., 2018). The resulting relative abundance table of annotated amplicon sequence variants was exported and used to generate taxonomy bar plots in order to visualize the relative abundance of the microbiome using QIIME2 VIEW3.
Two distinct 16S rRNA gene sequences of Bartonella (Bartonella sp. A and Bartonella sp. B) may represent either the existence of distinct bacteria or the intragenomic operon copies of a single bacterium. To distinguish these possibilities, we performed PCR, cloning, and sequencing for the single copy gene rpoB from an individual (18_4) whose microbiome was occupied mostly with Bartonella sp. A and Bartonella sp. B with similar abundance. A primer set, Univ_rpoB_F_deg and Univ_rpoB_R_deg (Supplementary Table 2; Ogier et al., 2019), was used for PCR and the cloning and sequencing were performed as described above.
PCR was conducted using KOD FX Neo or Ex Taq HS. The primers Rick-F and Rick-R were the same as those used in a previous study (Price et al., 2021). The amplicon was verified using 1.5% (w/v) agarose/TAE gel.
DNA was extracted from a pool of approximately 20 eggs freshly laid by D. gallinae females. The eggs were washed with 0.1% (w/v) benzalkonium chloride followed by two 5 min washes in 70% (v/v) ethanol, and were then subjected to DNA extraction and PCR using 27F-short and 1507R primers (Price et al., 2021). Cloning, plasmid extraction, and sequencing were performed as stated previously.
Whole-mount fluorescent in situ hybridization (FISH) targeting bacterial 16S rRNA was performed using the mites collected from Aomori Prefecture following Koga et al. (2009). For the detection of bacterial 16S rRNA, we used the probe EUB338 (5′-GCT GCC TCC CGT AGG AGT-3′) (Amann et al., 1990) labeled with Alexa Fluor 555 at the 5′ terminus. The samples were incubated in hybridization buffer containing 50 nM probe. After being washed in PBST, host nuclear DNA was stained with 4.5 μM 4′,6-diamidino-2-phenylindole (DAPI; Thermo Fisher Scientific). Then, the samples were washed with PBST again, mounted in SlowFade Diamond Antifademountant (Thermo Fisher Scientific), and observed under a laser scanning confocal microscope (LSM 700; Carl Zeiss, Germany).
In order to test whether the two bacteria, Cardinium and Wolbachia, share evolutionary trajectories in maternal lineages, the log marginal likelihood estimated on the COI tree with an assumed independent model was compared with that estimated with an assumed dependent model using BayesTraits V4.0.0.4 The log Bayes Factor (LBF), twice the difference between the two log marginal likelihoods, was used to interpret whether presence of the two bacteria were correlated (Gilks et al., 1995). LBF < 2 is considered weak evidence, 2 < LBF < 5 is considered positive evidence, 5 < LBF < 10 is considered strong evidence, and LBF >10 is considered very strong evidence.
By sequencing the mitochondrial COI region of 144 D. gallinae mites collected from 18 poultry farms (Figure 1), 31 haplotypes were categorized into three haplogroups AJ1, BJ1, and BJ2 according to previous studies (Øines and Brännström, 2011; Chu et al., 2015; Figure 2; Supplementary Figure 1; Supplementary Table 3). These haplogroups, however, do not necessarily reflect phylogenetically supported clades. The primary haplogroup BJ1 was closely related to several European populations (Norwegian, Swedish, Belgian) and a Korean population, whereas BJ2 was related to a Czech population (Figure 2; Supplementary Figure 2; Supplementary Table 4). These results may represent inter-country transport of contaminated material or infested birds as previously suggested (Øines and Brännström, 2011; Chu et al., 2015). AJ1 has only been found in the Japanese population, although it is relatively similar to the French population. AJ2 was found in Japan approximately 10 years ago (Chu et al., 2015), but was not found in the present study. Because multiple haplogroups were sometimes seen on a single poultry farm (Supplementary Table 3), invasions of D. gallinae are not likely to be very rare. In each poultry farm, chicken breeds are selected depending on their purpose (whether for eggs or meat), but mitochondrial haplogroups of D. gallinae were not associated with chicken breeds (purposes) (Supplementary Table 1). All 144 sample mites examined in the present study were identified as members of the well-supported clade of D. gallinae. Note that, as written below, D. gallinae possesses Wolbachia and Cardinium, which may confound the inference of an arthropod’s evolutionary history from mtDNA data because of maternal inheritance and linkage disequilibrium with mitochondria. Finally, the northern fowl mite, Ornithonyssus sylviarum, which resembles D. gallinae in size and appearance (Nakamae et al., 1997; Di Palma et al., 2012), is also known as a serious pest in poultry farms, but O. sylviarum was not found in the present samples.
Figure 2. Mitochondrial DNA diversity of Japanese D. gallinae examined in this study among global samples of D. gallinae. Left: A TCS haplotype network based on COI sequences (314 bp; 122 OTU) of D. gallinae. Right: A maximum-likelihood phylogenetic tree (model T92 + G) based on COI sequences (469 bp; 75 OTU) of D. gallinae together with three other species of Dermanyssus and two species of Ornithonyssus. Based on the 469-bp sequences, 144 individual mites examined in this study fell into 31 haplotypes (hap 1–hap 31). Haplogroups (A–D) are shaded by respective colors. For the network, existing haplotypes are represented by large circles, and missing haplotypes are represented by small circles which are connected by branches representing 1-bp substitutions. Based on the 314-bp sequences, the 31 haplotypes were assigned into 21 haplotypes (indicated by red circles in the network). For the phylogenetic tree, the three most representative haplotypes (hap 1, hap 2, and hap 3) are indicated with black circles and their bootstrap probability is shown at each node. Nomenclature of haplotypes used in the previous studies (Øines and Brännström, 2011; Chu et al., 2015) are given following the country names. Haplotypes of Japanese samples are shown in red (this study) and green (Chu et al., 2015). Numbers in square brackets indicate accession numbers of NCBI. See Supplementary Table 3 for sample information.
For the collected 144 sample individuals, microbiomes were analyzed through amplicon sequencing of the hypervariable V3/V4 region of 16S rRNA; 8,939,852 reads were reduced into 819 OTU (Supplementary Table 5). The 11 major OTU were Bartonella sp. B, Cardinium, Bartonella sp. A, Wolbachia, Tsukamurella, Micrococcus, Rickettsiella, Staphylococcus, Enterobacter, Rhodopseudomonas, and Psychrobacter in order of relative frequency (Figure 3; Supplementary Table 6). By assuming that an individual mite has the bacterium when the bacterium represented more than 1% of the tags analyzed, we showed that among the 144 individual mites, all had Bartonella sp. A (100%), 140 mites had Bartonella sp. B (97.2%), 76 mites had Cardinium (52.8%), 52 mites had Wolbachia (36.1%), 23 mites had Tsukamurella (16.0%), 17 mites had Micrococcus (11.8%), and 2 mites had Rickettsiella (1.4%). Box plots of the 18 poultry farms were drawn according to the Shannon diversity index (Supplementary Figure 3; Supplementary Table 7).
Figure 3. Results of amplicon sequencing of the hypervariable V3/V4 region using 144 individual D. gallinae mites from 18 poultry farms (eight individual mites from each poultry farm). For each individual, an mtDNA haplogroup (either A or B) is shown by a colored ellipse at the right (green for haplogroup A; purple for haplogroup B). Assigned bacterial taxa are color-coded as shown in the box on the right. Numbers on the left represent geographic localities shown in Figure 1.
While Rickettsia was previously suggested to be a symbiotic bacterium of D. gallinae (De Luna et al., 2009; Moro et al., 2009), the reads of Rickettsiales were not found in the present study, except for Wolbachia and a very few reads of plant mitochondria. Additionally, Spiroplasma, which was previously detected in a French D. gallinae study (Moro et al., 2009), was not found in the present study. A few reads found in some samples were Erysipelothrix and Pasteurella, which are suspected hen and human pathogens which have been detected in D. gallinae (Chirico et al., 2003; Moro et al., 2009; Eriksson et al., 2010; Moro et al., 2011; Hubert et al., 2017). However, the Erysipelothrix sequences obtained in this study had only 82–87% similarity with known hen-pathogenic Erysipelothrix rhusiopathiae sequences (Takahashi et al., 1994; Accession No.: NR_040837), whereas the Pasteurella sequences obtained in this study had 93%–94% similarity with known human opportunistic pathogen Pasteurella multocida sequences (Kuhnert et al., 2000; Accession No.: NR_041809). Determining whether these Erysipelothrix and Pasteurella in D. gallinae could be pathogenic for hens and/or humans requires further investigation.
The cloning and sequencing of nearly full sequences of 16S rRNA from one sample (8_1) from Tochigi Prefecture identified two strains of Bartonella, with each corresponding to either Bartonella sp. A or Bartonella sp. B. These sequences were closely related but differed in 66 bp among 1,437 bp, and formed a cluster with the Bartonella sp. sequences obtained from D. gallinae in a previous study (Hubert et al., 2017; Figure 4A). Although not well-supported by bootstrap value, this cluster was distinct from other Bartonella and Rhizobiales bacteria. It has been reported that 16S rRNA is not a suitable marker for Bartonella (La Scola et al., 2003) because of the existence of multiple copies in its genome (Viezens and Arvand, 2008; Banerjee et al., 2020). To distinguish whether Bartonella sp. A and Bartonella sp. B described by 16S rRNA amplicon sequencing are actually from a single strain, we sequenced a single copy gene rpoB (385 bp). An individual 18_4, whose microbiome was mostly occupied by Bartonella sp. A and Bartonella sp. B with a nearly equal ratio, was subjected to PCR, cloning, and sequencing (Supplementary Figure 4). All the obtained sequences (17 out of 17) were Bartonella-like, which consist of two types of sequences differing in 76 bp. This result strongly suggests that Bartonella sp. A and Bartonella sp. B are distinct strains of D. gallinae. Furthermore, these sequences of rpoB were a new lineage that differed from the known Bartonella and Rhizobiales (Figure 4B). It remains unknown, however, which of the rpoB sequences corresponds to Bartonella sp. A or Bartonella sp. B.
Figure 4. Phylogenetic relationship of the 16S rRNA sequences (A) and rpoB (B) of Bartonella and related species. (A) A maximum-likelihood phylogeny (model K2 + G + I) inferred from 1,175 aligned nucleotide sites are shown with bootstrap probability at each node. (B) A maximum-likelihood phylogeny (model K2 + G) inferred from 385 aligned nucleotide site. The sequences in bold with black circle are those obtained in this study. OTU of Bartonella from D. gallinae are shaded with light green. Numbers in square brackets indicate NCBI accession numbers.
A nearly full sequence of Rickettsiella 16S rRNA was obtained from a sample from Hokkaido (2_1) which had a relatively high abundance of Rickettsiella in the amplicon sequencing (Figure 3). This sequence was identical to the Rickettsiella sequence obtained from UK samples, and differed by 1 bp from Czech samples (Figure 5). These D. gallinae Rickettsiella sequences were related to Rickettsiella which infect the relict tick Haemaphysalis concinna and the seabird tick Ixodes uriae. The clade composed of the mite/tick Rickettsiella sequences also include Rickettsiella of the pea aphid Acyrthosiphon pisum and is distinct from the other clade composed of Rickettsiella from insects and isopods.
Figure 5. Phylogenetic relationship of the 16S rRNA sequences of Rickettsiella and related species. A maximum-likelihood phylogeny (model HKY + G + I) inferred from 1,018 aligned nucleotide sites is shown with bootstrap probability at each node. The sequences in bold with black circle are those obtained in this study. OTU of Rickettsiella from D. gallinae are shaded in pink. Numbers in square brackets indicate NCBI accession numbers.
We hypothesize that Bartonella sp. A is necessary for the survival or reproduction of D. gallinae because all 144 D. gallinae individuals examined had Bartonella sp. A. Although, Bartonella sp. B might be derived from the blood meal because the 16S rRNA sequence of Bartonella sp. B, which was possessed by 140 out of 144 D. gallinae individuals (97.2%), matched the sequence of Bartonella obtained from sampled avian blood (Accession No. MN320519, MN320520, MN320527). Cloning and sequencing of the bacterial 16S rRNA sequences using surface-sterilized D. gallinae eggs from Ibaraki Prefecture (derived from a different farm from those used for amplicon sequencing) identified both Bartonella sp. A and B (Figure 6), which is consistent with a previous study which detected Bartonella on eggs using amplicon sequencing (Hubert et al., 2017). These results may suggest that both Bartonella sp. A and B are vertically transmitted transovarially via egg cytoplasm. Then we performed whole-mount FISH targeting bacterial 16S rRNA to examine the localization of symbiotic bacteria using a D. gallinae adult and egg collected from Aomori Prefecture. As is shown by microbiome data (population No. 3 in Figure 3), this population has only Bartonella sp. A and Bartonella sp. B as major bacteria. The FISH preparation showed that, in the adult female, reddish fluorescence is observed throughout the cell cytoplasm except for the nucleus (Supplementary Figure 5), which is typical for intracellular symbionts. The bacteria appear to be present throughout the mite body rather than localized in a distinct bacteriocyte. FISH preparation was also made on eggs, but no clear bacterial image was observed. This may be due to the chorion and/or yolk proteins that inhibit the transmission of fluorescent signals. Note that these results were obtained with the universal 16S rRNA probe for bacteria, so further analysis with Bartonella-specific probes would be needed.
Figure 6. Relative abundance of bacteria associated with D. gallinae eggs derived from Ibaraki Prefecture based on 16S rRNA sequences obtained by cloning and sequencing of universal PCR products. The three primary bacterial taxa are colored as shown on the right. Two clones (Staphylococcus and Clostridium) are indicated with yellow. The number of clones (among 26 clones) is shown in parenthesis.
Amplicon sequence analysis detected Rickettsiella from only 2 out of 144 D. gallinae individuals. To test whether Rickettsiella was failed to be detected in many samples due to primer mismatches or other factors specific to amplicon sequencing, diagnostic PCR was performed for Rickettsiella utilizing primers used in a previous study (Price et al., 2021). Among 16 samples from Hokkaido Prefecture (populations No. 1 and 2), Rickettsiella was detected from only one individual (2_1), which is consistent with the results of the amplicon sequence analysis (Supplementary Figure 6); we therefore conclude that Rickettsiella infection is very rare in Japanese D. gallinae. This contrasts with European populations of D. gallinae, which have been reported to be highly infected with Rickettsiella (De Luna et al., 2009; Moro et al., 2009; Price et al., 2021). In Czechia, however, Rickettsiella was detected from only one of four sample sites studied (Hubert et al., 2017). Although no mitochondrial haplotypes were examined in relation to symbiotic bacteria in the European studies, we cannot rule out the possibility that haplogroups A and B have Bartonella as an essential symbiont and haplogroup C has Rickettsiella as an essential symbiont. This hypothesis may be worth testing in future research.
To summarize, Japanese D. gallinae are predominantly infested with Bartonella, which likely plays an important role in the survival of these mites. Bartonella bacteria generally have a hemotropic lifestyle and is found in mammalian hosts and blood-sucking parasitic arthropods, such as lice and fleas (Kosoy et al., 2012; Gutiérrez et al., 2014; Theonest et al., 2019). We speculate that Bartonella contributes to the synthesis of B vitamins, although this has not yet been demonstrated in any hosts.
As noted in the introduction, Rickettsiella is considered to be an important symbiont of D. gallinae because it has a nearly complete set of B vitamin synthetic pathways (Price et al., 2021). However, the biotin (vitamin B7) synthesis pathway has been shown to be incomplete due to the loss of bioH (Price et al., 2021). In the present study, two Rickettsiella-possessing individuals were found that had both Bartonella sp. A and B. Considering the contrasting frequencies of Bartonella and Rickettsiella, it is likely that Bartonella is an important symbiont for D. gallinae, whereas Rickettsiella has an auxiliary role or pathogenic effect, at least in Japanese red mite populations.
The nearly full sequences of 16S rRNA of Cardinium and Wolbachia were obtained from one sample (8_1) from Tochigi Prefecture. Phylogenetically, the Cardinium derived from D. gallinae fell into the group A of Cardinium, the most common group found among various arthropods (Nakamura et al., 2009) (Figure 7A). In contrast, the Wolbachia obtained from D. gallinae was idiosyncratic, and was located basally of all previously published Wolbachia, but formed a well-supported clade with other Wolbachia (Figure 7B).
Figure 7. Phylogenetic relationships of the 16S rRNA sequences of Cardinium (A) and Wolbachia (B). (A) Maximum-likelihood tree (the model is K2 + G + I based on 1,175 bp) of Cardinium, which can be separated into three groups (A, B, and C). (B) Maximum-likelihood tree (the model is HKY + G + I based on 1,226 bp) of Wolbachia. Supergroups of Wolbachia are indicated in round brackets. A bootstrap probability is given at each node. The sequences in bold with black circle are those obtained in this study. Numbers in square brackets indicate NCBI accession numbers.
Both Cardinium and Wolbachia are known as maternally inherited symbionts which manipulate host reproduction in various manners (such as cytoplasmic incompatibility, feminization, male killing, and induction of parthenogenesis) to enhance their own transmission (Werren et al., 2008; Hurst and Frost, 2015). In spider mites, it has been reported that Wolbachia and Cardinium cause cytoplasmic incompatibility (Breeuwer, 1997; Gotoh et al., 2007), and that Cardinium causes feminization (Weeks et al., 2001; Chigira and Miura, 2005). Because of the linkage disequilibrium between maternally inherited bacteria and host mitochondrial DNA, the spread of maternally inherited symbionts can result in indirect selection on mitochondrial DNA (Hurst and Jiggins, 2005). This study compared the infection frequencies of Cardinium and Wolbachia in respective mtDNA haplotypes (Supplementary Figure 7). While haplogroup BJ1 had a low Cardinum and/or Wolbachia infection rate (infection rate of either bacterium is 25.4% (18/71)), haplogroup AJ1 and haplogroup BJ2 had very high infection rates of 79.1% (34/43) and 96.7% (29/30), respectively. These conspicuously biased infection rates may indicate that Cardinum and/or Wolbachia manipulate host reproduction. The LBF that inferred dependency between Cardinium and Wolbachia was 26.539, which is very strong evidence for correlation (Gilks et al., 1995). We hypothesize that Wolbachia, Cardinium, or both may induce reproductive manipulations in D. gallinae and its symbionts, and that the associated cytoplasms (i.e., mitochondrial haplotypes) spread in the host populations.
We investigated the microbiome of the red poultry mite D. gallinae in Japanese populations. Using Illumina Miseq amplicon sequencing, and full 16S rRNA sequencing using universal primers, we identified the following properties of the D. gallinae microbiome. First, all individual mites had Bartonella sp. A, and most individuals had Bartonella sp. B, which are both closely related to the Bartonella harbored by D. gallinae in Czechia. These Bartonella species, which are probably vertically transmitted through eggs, may play important roles in D. gallinae survival. Second, unlike many European populations, Rickettsiella was rarely found and is unlikely to play an important role in the survival of D. gallinae, at least in Japanese populations. Third, Cardinium and Wolbachia were found at relatively high frequencies, and while the Cardinium identified belonged to a lineage commonly found in insects and other arthropods, the Wolbachia belonged to a novel lineage that is located basally to all other Wolbachia found so far. It is possible that these Cardinium and Wolbachia strongly impact the mitochondrial genome dynamics of D. gallinae. It should also be noted that regarding all the detected bacteria, no direct evidence for their roles within D. gallinae was identified because D. gallinae could not be successfully reared despite multiple attempts following Bruneau et al. (2001). Inoculation and removal of each bacterium to fulfill Koch’s postulate, as well as genome sequencing of each bacterium will be necessary to evaluate the impact of the bacteria on D. gallinae.
The data presented in the study are deposited in NCBI, accession numbers LC710609-LC710644, LC731006-LC731007, and DRR376882–DRR377025.
YN, TS, KW, HE, and DK conceived and designed the experiments. YN organized all the samples and performed experiments. TS analyzed the raw reads of Illumina Miseq. YN wrote the draft manuscript and DK revised the manuscript with the inputs from all authors. All authors contributed to the article and approved the submitted version.
The authors declare that this study received funding from SC Environmental Science Co., Ltd. The funder was not involved in the study design, collection, analysis, interpretation of data, the writing of this article, or the decision to submit it for publication.
We thank Takayo Nakakura, Masae Takashima, and Maria Murakami for their technical assistance, and Takuhiko Yokoyama for graphical illustration.
HE was employed by the company SC Environmental Science Co., Ltd.
The remaining authors declare that the research was conducted in the absence of any commercial or financial relationships that could be construed as a potential conflict of interest.
All claims expressed in this article are solely those of the authors and do not necessarily represent those of their affiliated organizations, or those of the publisher, the editors and the reviewers. Any product that may be evaluated in this article, or claim that may be made by its manufacturer, is not guaranteed or endorsed by the publisher.
The Supplementary material for this article can be found online at: https://www.frontiersin.org/articles/10.3389/fmicb.2022.1031535/full#supplementary-material
1. ^https://data.qiime2.org/2022.2/common/silva-138-99-515-806-nb-classifier.qza
2. ^https://data.qiime2.org/2022.2/common/gg-13-8-99-515-806-nb-classifier.qza
4. ^http://www.evolution.reading.ac.uk/BayesTraitsV4.0.0/BayesTraitsV4.0.0.html
Akman, L., Yamashita, A., Watanabe, H., Oshima, K., Shiba, T., Hattori, M., et al. (2002). Genome sequence of the endocellular obligate symbiont of tsetse flies, Wigglesworthia glossinidia. Nat. Genet. 32, 402–407. doi: 10.1038/ng986
Amann, R. I., Krumholz, L., and Stahl, D. A. (1990). Fluorescent-oligonucleotide probing of whole cells for determinative, phylogenetic, and environmental studies in microbiology. J. Bacteriol. 172, 762–770. doi: 10.1128/jb.172.2.762-770.1990
Banerjee, R., Shine, O., Rajachandran, V., Krishnadas, G., Minnick, M. F., Paul, S., et al. (2020). Gene duplication and deletion, not horizontal transfer, drove intra-species mosaicism of Bartonella henselae. Genomics 112, 467–471. doi: 10.1016/j.ygeno.2019.03.009
Bokulich, N. A., Kaehler, B. D., Rideout, J. R., Dillon, M., Bolyen, E., Knight, R., et al. (2018). Optimizing taxonomic classification of marker-gene amplicon sequences with QIIME 2’s q2-feature-classifier plugin. Microbiome 6:90. doi: 10.1186/s40168-018-0470-z
Bolyen, E., Rideout, J. R., Dillon, M. R., Bokulich, N. A., Abnet, C. C., Al-Ghalith, G. A., et al. (2019). Reproducible, interactive, scalable and extensible microbiome data science using QIIME 2. Nat. Biotechnol. 37, 852–857. doi: 10.1038/s41587-019-0209-9
Breeuwer, J. A. J. (1997). Wolbachia and cytoplasmic incompatibility in the spider mites Tetranychus urticae and T. turkestani. Heredity 79, 41–47. doi: 10.1038/hdy.1997.121
Bruneau, A., Dernburg, A., Chauve, C., and Zenner, L. (2001). First in vitro cycle of the chicken mite, Dermanyssus gallinae (DeGeer 1778), utilizing an artificial feeding device. Parasitology 123, 583–589. doi: 10.1017/S0031182001008836
Callahan, B. J., McMurdie, P. J., Rosen, M. J., Han, A. W., Johnson, A. J. A., and Holmes, S. P. (2016). DADA2: high-resolution sample inference from Illumina amplicon data. Nat. Methods 13, 581–583. doi: 10.1038/nmeth.3869
Chauve, C. (1998). The poultry red mite Dermanyssus gallinae (De Geer, 1778): current situation and future prospects for control. Vet. Parasitol. 79, 239–245. doi: 10.1016/S0304-4017(98)00167-8
Chigira, A., and Miura, K. (2005). Detection of ‘Candidatus Cardinium’ bacteria from the haploid host Brevipalpus Californicus (Acari: Tenuipalpidae) and effect on the host. Exp. Appl. Acarol. 37, 107–116. doi: 10.1007/s10493-005-0592-4
Chirico, J., Eriksson, H., Fossum, O., and Jansson, D. (2003). The poultry red mite, Dermanyssus gallinae, a potential vector of Erysipelothrix rhusiopathiae causing erysipelas in hens. Med. Vet. Entomol. 17, 232–234. doi: 10.1046/j.1365-2915.2003.00428.x
Chu, T. T. H., Murano, T., Uno, Y., Usui, T., and Yamaguchi, T. (2015). Molecular epidemiological characterization of poultry red mite, Dermanyssus gallinae, in Japan. J. Vet. Med. Sci. 77, 1397–1403. doi: 10.1292/jvms.15-0203
De Luna, C. J., Moro, C. V., Guy, J. H., Zenner, L., and Sparagano, O. A. E. (2009). Endosymbiotic bacteria living inside the poultry red mite (Dermanyssus gallinae). Exp. Appl. Acarol. 48, 105–113. doi: 10.1007/s10493-008-9230-2
Di Palma, A., Giangaspero, A., Cafiero, M. A., and Germinara, G. S. (2012). A gallery of the key characters to ease identification of Dermanyssus gallinae (Acari: Gamasida: Dermanyssidae) and allow differentiation from Ornithonyssus sylviarum (Acari: Gamasida: Macronyssidae). Parasit. Vectors 5:104. doi: 10.1186/1756-3305-5-104
Duron, O., and Gottlieb, Y. (2020). Convergence of nutritional symbioses in obligate blood feeders. Trends Parasitol. 36, 816–825. doi: 10.1016/j.pt.2020.07.007
Eriksson, H., Brännström, S., Skarin, H., and Chirico, J. (2010). Characterization of Erysipelothrix rhusiopathiae isolates from laying hens and poultry red mites (Dermanyssus gallinae) from an outbreak of erysipelas. Avian Pathol. 39, 505–509. doi: 10.1080/03079457.2010.518313
George, D. R., Finn, R. D., Graham, K. M., Mul, M. F., Maurer, V., Moro, C. V., et al. (2015). Should the poultry red mite Dermanyssus gallinae be of wider concern for veterinary and medical science? Parasit. Vectors 8:178. doi: 10.1186/s13071-015-0768-7
Gilks, W. R., Richardson, S., and Spiegelhalter, D. (1995). Markov Chain Monte Carlo in Practice. Boca Raton, FL: CRC Press, doi: 10.1201/b14835
Gotoh, T., Noda, H., and Ito, S. (2007). Cardinium symbionts cause cytoplasmic incompatibility in spider mites. Heredity 98, 13–20. doi: 10.1038/sj.hdy.6800881
Gutiérrez, R., Cohen, L., Morick, D., Mumcuoglu, K. Y., Harrus, S., and Gottlieb, Y. (2014). Identification of different Bartonella species in the cattle tail louse (Haematopinus quadripertusus) and in cattle blood. Appl. Environ. Microbiol. 80, 5477–5483. doi: 10.1128/AEM.01409-14
Hosokawa, T., Koga, R., Kikuchi, Y., Meng, X.-Y., and Fukatsu, T. (2010). Wolbachia as a bacteriocyte-associated nutritional mutualist. Proc. Natl. Acad. Sci. 107, 769–774. doi: 10.1073/pnas.0911476107
Hubert, J., Erban, T., Kopecky, J., Sopko, B., Nesvorna, M., Lichovnikova, M., et al. (2017). Comparison of microbiomes between red poultry mite populations (Dermanyssus gallinae): predominance of Bartonella-like bacteria. Microb. Ecol. 74, 947–960. doi: 10.1007/s00248-017-0993-z
Hurst, G. D. D., and Frost, C. L. (2015). Reproductive parasitism: maternally inherited symbionts in a biparental world. Cold Spring Harb. Perspect. Biol. 7:a017699. doi: 10.1101/cshperspect.a017699
Hurst, G. D. D., and Jiggins, F. M. (2005). Problems with mitochondrial DNA as a marker in population, phylogeographic and phylogenetic studies: the effects of inherited symbionts. Proc. R. Soc. B Biol. Sci. 272, 1525–1534. doi: 10.1098/rspb.2005.3056
Husnik, F. (2018). Host–symbiont–pathogen interactions in blood-feeding parasites: nutrition, immune cross-talk and gene exchange. Parasitology 145, 1294–1303. doi: 10.1017/S0031182018000574
Kilpinen, O., Roepstorff, A., Permin, A., Nørgaard-Nielsen, G., Lawson, L. G., and Simonsen, H. B. (2005). Influence of Dermanyssus gallinae and Ascaridia galli infections on behaviour and health of laying hens (Gallus gallus domesticus). Br. Poult. Sci. 46, 26–34. doi: 10.1080/00071660400023839
Koga, R., Tsuchida, T., and Fukatsu, T. (2009). Quenching autofluorescence of insect tissues for in situ detection of endosymbionts. Appl. Entomol. Zool. 44, 281–291. doi: 10.1303/aez.2009.281
Kosoy, M., Hayman, D. T. S., and Chan, K.-S. (2012). Bartonella bacteria in nature: where does population variability end and a species start? Infect. Genet. Evol. 12, 894–904. doi: 10.1016/j.meegid.2012.03.005
Kuhnert, P., Boerlin, P., Emler, S., Krawinkler, M., and Frey, J. (2000). Phylogenetic analysis of Pasteurella multocida subspecies and molecular identification of feline P. multocida subsp. septica by 16S rRNA gene sequencing. Int. J. Med. Microbiol. 290, 599–604. doi: 10.1016/S1438-4221(00)80008-9
La Scola, B., Zeaiter, Z., Khamis, A., and Raoult, D. (2003). Gene-sequence-based criteria for species definition in bacteriology: the Bartonella paradigm. Trends Microbiol. 11, 318–321. doi: 10.1016/S0966-842X(03)00143-4
McDonald, D., Price, M. N., Goodrich, J., Nawrocki, E. P., DeSantis, T. Z., Probst, A., et al. (2012). An improved Greengenes taxonomy with explicit ranks for ecological and evolutionary analyses of bacteria and archaea. ISME J. 6, 610–618. doi: 10.1038/ismej.2011.139
Michalkova, V., Benoit, J. B., Weiss, B. L., Attardo, G. M., and Aksoy, S. (2014). Vitamin B6 generated by obligate symbionts is critical for maintaining proline homeostasis and fecundity in tsetse flies. Appl. Environ. Microbiol. 80, 5844–5853. doi: 10.1128/AEM.01150-14
Moriyama, M., Nikoh, N., Hosokawa, T., and Fukatsu, T. (2015). Riboflavin provisioning underlies Wolbachia’s fitness contribution to its insect host. MBio 6, e01732–e01715. doi: 10.1128/mBio.01732-15
Moro, C. V., Thioulouse, J., Chauve, C., Normand, P., and Zenner, L. (2009). Bacterial taxa associated with the hematophagous mite Dermanyssus gallinae detected by 16S rRNA PCR amplification and TTGE fingerprinting. Res. Microbiol. 160, 63–70. doi: 10.1016/j.resmic.2008.10.006
Moro, C. V., Thioulouse, J., Chauve, C., and Zenner, L. (2011). Diversity, geographic distribution, and habitat-specific variations of microbiota in natural populations of the chicken mite, Dermanyssus gallinae. J. Med. Entomol. 48, 788–796. doi: 10.1603/ME10113
Nakamae, H., Fujisaki, K., Kishi, S., Yashiro, M., Oshiro, S., and Furuta, K. (1997). The new parasitic ecology of chicken mites Dermanyssus gallinae, parasitizing and propagating on chickens even in the daytime. Jpn. Poult. Sci. 34, 110–116. doi: 10.2141/jpsa.34.110
Nakamura, Y., Kawai, S., Yukuhiro, F., Ito, S., Gotoh, T., Kisimoto, R., et al. (2009). Prevalence of Cardinium bacteria in planthoppers and spider mites and taxonomic revision of “Candidatus Cardinium hertigii” based on detection of a new Cardinium group from biting midges. Appl. Environ. Microbiol. 75, 6757–6763. doi: 10.1128/AEM.01583-09
Nikoh, N., Hosokawa, T., Moriyama, M., Oshima, K., Hattori, M., and Fukatsu, T. (2014). Evolutionary origin of insect–Wolbachia nutritional mutualism. Proc. Natl. Acad. Sci. 111, 10257–10262. doi: 10.1073/pnas.1409284111
Nordenfors, H., Höglund, J., Tauson, R., and Chirico, J. (2001). Effect of permethrin impregnated plastic strips on Dermanyssus gallinae in loose-housing systems for laying hens. Vet. Parasitol. 102, 121–131. doi: 10.1016/S0304-4017(01)00528-3
Ogier, J.-C., Pagès, S., Galan, M., Barret, M., and Gaudriault, S. (2019). rpoB, a promising marker for analyzing the diversity of bacterial communities by amplicon sequencing. BMC Microbiol. 19:171. doi: 10.1186/s12866-019-1546-z
Øines, Ø., and Brännström, S. (2011). Molecular investigations of cytochrome c oxidase subunit I (COI) and the internal transcribed spacer (ITS) in the poultry red mite, Dermanyssus gallinae, in northern Europe and implications for ITS transmission between laying poultry farms. Med. Vet. Entomol. 25, 402–412. doi: 10.1111/j.1365-2915.2011.00958.x
Price, D. R. G., Bartley, K., Blake, D. P., Karp-Tatham, E., Nunn, F., Burgess, S. T. G., et al. (2021). A Rickettsiella endosymbiont is a potential source of essential B-vitamins for the poultry red mite, Dermanyssus gallinae. Front. Microbiol. 12:695346. doi: 10.3389/fmicb.2021.695346
Quast, C., Pruesse, E., Yilmaz, P., Gerken, J., Schweer, T., Yarza, P., et al. (2013). The SILVA ribosomal RNA gene database project: improved data processing and web-based tools. Nucleic Acids Res. 41, D590–D596. doi: 10.1093/nar/gks1219
Sigognault Flochlay, A., Thomas, E., and Sparagano, O. (2017). Poultry red mite (Dermanyssus gallinae) infestation: a broad impact parasitological disease that still remains a significant challenge for the egg-laying industry in Europe. Parasit. Vectors 10:357. doi: 10.1186/s13071-017-2292-4
Sparagano, O. A. E., George, D. R., Harrington, D. W. J., and Giangaspero, A. (2014). Significance and control of the poultry red mite, Dermanyssus gallinae. Annu. Rev. Entomol. 59, 447–466. doi: 10.1146/annurev-ento-011613-162101
Sparagano, O., Pavlićević, A., Murano, T., Camarda, A., Sahibi, H., Kilpinen, O., et al. (2009). “Prevalence and key figures for the poultry red mite Dermanyssus gallinae infections in poultry farm systems” in Control of Poultry Mites (Dermanyssus). ed. O. A. E. Sparagano (Springer Netherlands: Dordrecht, Netherlands), 3–10. doi: 10.1007/978-90-481-2731-3_2
Takahashi, T., Takagi, M., Yamaoka, R., Ohishi, K., Norimatsu, M., Tamura, Y., et al. (1994). Comparison of the pathogenicity for chickens of Erysipelothrix rhusiopathiae and Erysipelothrix tonsillarum. Avian Pathol. 23, 237–245. doi: 10.1080/03079459408418992
Tamura, K., Peterson, D., Peterson, N., Stecher, G., Nei, M., and Kumar, S. (2011). MEGA5: molecular evolutionary genetics analysis using maximum likelihood, evolutionary distance, and maximum parsimony methods. Mol. Biol. Evol. 28, 2731–2739. doi: 10.1093/molbev/msr121
Templeton, A. R., Crandall, K. A., and Sing, C. F. (1992). A cladistic analysis of phenotypic associations with haplotypes inferred from restriction endonuclease mapping and DNA sequence data. III. Cladogram estimation. Genetics 132, 619–633. doi: 10.1093/genetics/132.2.619
Theonest, N. O., Carter, R. W., Amani, N., Doherty, S. L., Hugho, E., Keyyu, J. D., et al. (2019). Molecular detection and genetic characterization of Bartonella species from rodents and their associated ectoparasites from northern Tanzania. PLoS One 14:e0223667. doi: 10.1371/journal.pone.0223667
Viezens, J., and Arvand, M. (2008). Simultaneous presence of two different copies of the 16S rRNA gene in Bartonella henselae. Microbiology 154, 2881–2886. doi: 10.1099/mic.0.2008/018630-0
Wang, F. F., Wang, M., Xu, F. R., Liang, D. M., and Pan, B. L. (2010). Survey of prevalence and control of ectoparasites in caged poultry in China. Vet. Rec. 167, 934–937. doi: 10.1136/vr.c6212
Weeks, A. R., Marec, F., and Breeuwer, J. A. J. (2001). A mite species that consists entirely of haploid females. Science 292, 2479–2482. doi: 10.1126/science.1060411
Keywords: symbiont bacteria, Bartonella, Rickettsiella, Cardinium, Wolbachia
Citation: Nishide Y, Sugimoto TN, Watanabe K, Egami H and Kageyama D (2022) Genetic variations and microbiome of the poultry red mite Dermanyssus gallinae . Front. Microbiol. 13:1031535. doi: 10.3389/fmicb.2022.1031535
Received: 30 August 2022; Accepted: 11 October 2022;
Published: 08 November 2022.
Edited by:
Yuval Gottlieb, Hebrew University of Jerusalem, IsraelReviewed by:
Daniel R. G. Price, Moredun Research Institute, United KingdomCopyright © 2022 Nishide, Sugimoto, Watanabe, Egami and Kageyama. This is an open-access article distributed under the terms of the Creative Commons Attribution License (CC BY). The use, distribution or reproduction in other forums is permitted, provided the original author(s) and the copyright owner(s) are credited and that the original publication in this journal is cited, in accordance with accepted academic practice. No use, distribution or reproduction is permitted which does not comply with these terms.
*Correspondence: Yudai Nishide, bmlzaGl5dTBAYWZmcmMuZ28uanA=; Daisuke Kageyama, a2FneW1hZEBnby5qcA==
Disclaimer: All claims expressed in this article are solely those of the authors and do not necessarily represent those of their affiliated organizations, or those of the publisher, the editors and the reviewers. Any product that may be evaluated in this article or claim that may be made by its manufacturer is not guaranteed or endorsed by the publisher.
Research integrity at Frontiers
Learn more about the work of our research integrity team to safeguard the quality of each article we publish.