- 1Biotechnology Center, Universidad Católica del Norte, Antofagasta, Chile
- 2Cátedra de Biotecnología, Facultad de Farmacia y Bioquímica, Universidad de Buenos Aires, Buenos Aires, Argentina
- 3Instituto de Nanobiotecnología (NANOBIOTEC), Universidad de Buenos Aires (UBA) - Consejo Nacional de Investigaciones Científicas y Técnicas (CONICET), Buenos Aires, Argentina
- 4Departamento de Biología, Facultad de Ciencias, Universidad de Chile, Antofagasta, Chile
- 5Nucleus for the Study of Cancer at a Basic, Applied, and Clinical Level, Universidad Católica del Norte, Antofagasta, Chile
The literature has reported the isolation of arsenate-dependent growing microorganisms which lack a canonical homolog for respiratory arsenate reductase, ArrAB. We recently isolated an arsenate-dependent growing bacterium from volcanic arsenic-bearing environments in Northern Chile, Fusibacter sp. strain 3D3 (Fas) and studied the arsenic metabolism in this Gram-positive isolate. Features of Fas deduced from genome analysis and comparative analysis with other arsenate-reducing microorganisms revealed the lack of ArrAB coding genes and the occurrence of two arsC genes encoding for putative cytoplasmic arsenate reductases named ArsC-1 and ArsC-2. Interestingly, ArsC-1 and ArsC-2 belong to the thioredoxin-coupled family (because of the redox-active disulfide protein used as reductant), but they conferred differential arsenate resistance to the E. coli WC3110 ΔarsC strain. PCR experiments confirmed the absence of arrAB genes and results obtained using uncouplers revealed that Fas growth is linked to the proton gradient. In addition, Fas harbors ferredoxin-NAD+ oxidoreductase (Rnf) and electron transfer flavoprotein (etf) coding genes. These are key molecular markers of a recently discovered flavin-based electron bifurcation mechanism involved in energy conservation, mainly in anaerobic metabolisms regulated by the cellular redox state and mostly associated with cytoplasmic enzyme complexes. At least three electron-bifurcating flavoenzyme complexes were evidenced in Fas, some of them shared in conserved genomic regions by other members of the Fusibacter genus. These physiological and genomic findings permit us to hypothesize the existence of an uncharacterized arsenate-dependent growth metabolism regulated by the cellular redox state in the Fusibacter genus.
Introduction
The occurrence of an active As biogeochemical cycle in sediment samples of Salar de Ascotán, Northern Chile, has been previously confirmed by our group, by performing enrichment cultures (Lara et al., 2012). In addition, 16S rDNA sequencing analysis revealed that, despite being members of genera that had not been previously reported as As metabolizing, several isolates obtained from that salt flat are capable to metabolize As. Interestingly, one of these isolates belongs to the Fusibacter genus and we reported it as Fusibacter sp. strain 3D3 (hereafter called Fas; Serrano et al., 2017). In addition, by a preliminary analysis of the draft sequence of Fas genome we have revealed for the first time in a Fusibacter species the presence of an arsenate reductase gene (arsC) and of all the genes encoding the Rnf complex, and suggested the absence of genes encoding ArrAB proteins involved in dissimilatory arsenate reduction (Serrano et al., 2017).
ArsC is a cytoplasmic arsenate reductase reducing As(V) to As(III), which is then extruded out of the cell by the specific pumps. Three different ArsC prokaryotic families have been defined based on their protein structures, reduction mechanisms and location of the catalytic cysteine residues (Villadangos et al., 2011): (i) the glutathione (GSH)/glutaredoxin (Grx)-coupled class in Gram-negative bacteria such as Escherichia coli (Mukhopadhyay and Rosen, 2002); (ii) the thioredoxin (Trx)/thioredoxin reductase (TR)-dependent class in Gram-positive bacteria (Zegers et al., 2001); (iii) the mycothiol (MSH)/mycoredoxin (Mrx)-dependent class also in Gram-positive bacteria such as Actinobacteria spp. (Ordonez et al., 2009). Kinetic data on arsenate reduction have shown higher catalytic efficiency in Trx-linked arsenate reductases than in Grx-linked ones. In some cases, the efflux of As(III) can also be coupled to the electrochemical proton gradient, where chemical energy in the form of ATP is used to pump As(III) with the help of the ATPase ArsA (Rosen and Liu, 2009).
The number and type (Trx or Grx clade) of arsC genes present in the genomes of prokaryotic organisms is related to As resistance levels (Li and Krumholz, 2007; Achour-Rokbani et al., 2010; Cuebas et al., 2011; Villadangos et al., 2011). The Trx reducing system has been reported to be the most efficient system exploited by arsenate reductases (Villadangos et al., 2011). Besides, arsenate reductases with the same structural fold but depending on two different thiol-disulfide relay mechanisms (Trx and GSH) have also been observed in a single bacterial species, Corynebacterium glutamicum ATCC 13032 (Villadangos et al., 2011). In that case, a different role has been proposed for both enzymes, representatives of different families of prokaryotic ArsC: the Trx-dependent one would reduce arsenate to regulate the gene expression of the other, that is involved in the As resistance (Villadangos et al., 2011). A predominance of Trx-linked ArsC have been found in low G + C Gram-positive bacteria (Messens and Silver, 2006), which is the predominant bacterial group that we found in the volcanic As-impacted environments of Northern Chile (Lara et al., 2012; Escudero et al., 2013).
The absence of homologs of the respiratory arsenate reductase genes, arrAB, has been reported for different isolated microorganisms. In Desulfomicrobium strain Ben-RB, neither ArrA nor ArsC were evidenced by PCR, and a cytochrome with increased activity in cultures with As(V) was proposed as the responsible for arsenate reduction (Macy et al., 2000). In Pyrobaculum aerophilum, a tetrathionate reductase (ttrA), highly expressed by culturing in presence of As(V), was hypothesized as a novel type of respiratory arsenate reductase (Cozen et al., 2009). In Citrobacter TSA, the authors reported that only arsC mRNA was strongly expressed when it was cultured with As(V), and hypothesized the occurrence of a linked electron flow to the cytoplasmic ArsC protein (Blum et al., 2018).
Interestingly, similar gaps in the energy metabolism of anaerobes (Bertsch et al., 2013; Buckel and Thauer, 2013) were closed by the characterization of Flavin-Based Electron Bifurcation (FBEB). This type of energy conservation is based on two main components: (i) the electron-bifurcating (electron transfer) flavoprotein complexes (Etf-complexes), where ferredoxins and flavodoxins act as low-potential terminal acceptors, and (ii) the electron transport phosphorylation (ETP) with protons (ferredoxin-proton reductase, Ech) or NAD+ (ferredoxin-NAD+ reductase, Rnf) as electron acceptors, where ferredoxins and flavodoxins re-oxidation drive electrochemical H+ and Na+ pumps (Schuchmann and Müller, 2016). Up to 12 Etf multienzyme complexes have been reported (Buckel and Thauer, 2013; Peters et al., 2018; Poudel et al., 2018; Liang et al., 2019). Almost all are located in the cytoplasm and are involved in the electron bifurcation (referred to as electron confurcation when operating in reverse) mechanism and associated to energy conservation. Furthermore, the studies on the distribution of the identified FBEB enzymes have shown that they are predominantly present among members of the Firmicutes and contribute to diverse metabolic pathways (Poudel et al., 2018; Liang et al., 2019). A key role in balancing the ratio of oxidized to reduced NAD(H) and ferredoxin (Fd) pools has been proposed for the FBEB mechanism. The presence of FBEB enzymes has also been identified in arsenate reducers (e.g., Alkaliphilus oremlandii OhILAs) but its activity was not clarified under As reduction conditions (Poudel et al., 2018).
Then, as we have previously reported that Fas possesses key molecular elements for As respiration (Serrano et al., 2017), the aim of this work was to assess the dependence of Fas growth on As(V) reduction and to elucidate the role of the cytoplasmic ArsC and the Rnf complex in this energy metabolism by culturing, revisiting its genome, and genetic approaches. In that way, we expect to contribute to the understanding of the means to achieve arsenate respiration in ArrAB-independent microorganisms, abundant in As bearing volcanic environments.
Materials and methods
Bacterial strains
Fusibacter sp. strain 3D3 was isolated at the Centro de Biotecnología, Universidad Católica del Norte, Antofagasta, Chile, from samples collected in the hypersaline sediments of the Salar de Ascotán in Northern Chile and deposited in the American Type Culture Collection as Fusibacter ascotence ATCC BAA-2418 (hereinafter referred to as Fas). The necessary tests and deposits to describe the isolate as a new species are running, and “Fusibacter ascotence” is the proposed name. The Fas draft genome assembly (Serrano et al., 2017) is available on NCBI (RefSeq GCF_001748365.1, GenBank GCA_001748365.1). The E. coli WC3110 ΔarsC strain was generously given by Dr. Barry P. Rosen.
Culture characterization
All growth experiments were performed in duplicate in serum bottles containing 20 ml liquid Newman-modified minimal medium with lactate (10 mM), sulfate (20 mM), arsenate (2 mM), yeast extract (0.1%), NaCl (10 g L−1), and cysteine (1 mM), pH 7, inoculated with 1×106 cells mL−1 from a fresh culture and incubated at 30°C in an anaerobic chamber under N2:CO2:H2 gas atmosphere (80:15:5 v/v) for 5 to 10 days in the dark, unless otherwise stated. An abiotic control was carried out in sterile medium without inoculum. Cell growth was monitored by microscope cell counting using a Neubauer improved chamber (0.01 mm x 0.0025 mm2, Marienfeld). To test for growth in the presence of oxygen, aerobic cultures were performed in shaking flasks incubated at 100 rpm in a rotatory shaker. The range of temperature for growth was tested between 15°C and 37°C, and the range of pH between 4 and 9. To assess the fermentative metabolism, Fas was grown with alternative substrates: lactate, acetate, citrate, glucose, galactose, glycine, or tryptone (10 mM). Sodium thiosulfate (10 mM), sodium sulfate (0 to10 mM), elemental sulfur (1%), yeast extract (0.2%) or cysteine (1 mM) were added to culture media to determine its ability to obtain energy from sulfate reduction and to use different sulfur sources. To test for arsenate resistance and the optimal concentration of As for energy metabolism, a range of concentrations between 0 and 16 mM was assayed. To find out if the growth of Fas on As(V) as electron acceptor was linked to oxidative phosphorylation and formation of proton or sodium gradients, growth was also evaluated with the addition of the protonophore 3,3′,4′,5-tetrachlorosalicylanilide (TCS, 20 μM) or the sodium-specific ionophore N,N,N′,N′-tetracyclohexyl-1,2-phenylenedioxydiacetamide (ETH2120, 20 μM; Tremblay et al., 2012; Wang et al., 2016).
Analytical methods
To evaluate the arsenate and sulfate reducing activity, arsenic concentrations in the culture medium from bacterial cultures were measured after filtering through 0.02 μm pore size using Hydride Generation Atomic Absorption Spectroscopy (HG-AAS) and the As speciation, As(III) and As(V), was analyzed using a Chromatography PSA 10.055 Millennium Excalibur. Lactate, acetate, and sulfate were quantified by ion chromatography (Dionex ™ 3,200, Thermo Scientific) with an IonPac™ AS11-HC (4×250) analytical column with AG11-HC pre-column, injected with 10 μl of filtered bacterial culture supernatant, using a run time of 15 min at 30°C and a flow of 1.5 ml min−1. To calculate the concentrations according to the peak area, linear standard curves were performed in the range of 0–100 mg L−1 (R2˃0.99).
Trx and TR enzymatic assays
To evaluate the involvement of the Trx system in the early (30 min) and late (8 h) response to As(V) exposure, the Trx and TR activities were measured at 30°C using 50 μg of whole cell extracts as described previously (Norambuena et al., 2012), a control test without exposure to As(V) and one more without cell extract were included in the experiment. Total Trx activity was determined by the insulin precipitation assay (Holmgren, 1979). The standard assay mixture contained 0.1 M potassium phosphate (pH 7.0), 1 mM EDTA, and 0.13 mM bovine insulin in the absence or in presence of the cellular extract, the reaction was started upon the addition of 1 mM DTT and the increase of the absorbance at 650 nm produced by the reduction of the insulin alpha-chain was monitored. TR was assayed for reductive activity toward 5,5-dithio-bis-(2-nitrobenzoic acid; DTNB) with NADPH to form 5-thio-2-nitrobenzoic acid (TNB), producing a strong yellow color that was measured at 412 nm (Arner et al., 1999).
DNA purification
Bacterial DNA was extracted and purified using the High Pure PCR Template Preparation kit (Roche, cat. n° 11,796,828,001) according to the manufacturer’s protocol. PCR products were purified using the QIAquick PCR Purification kit (QIAGEN, cat. n° 28,104), and digested DNA products were extracted from the agarose gel using the QIAquick Gel Extraction kit (QIAGEN, cat. n° 28,704), according to the manufacturer’s instructions.
PCR conditions
The presence of an arrA gene in the Fas genome was assessed by a PCR assay performed using the universal arrAf and arrAr primers to target an arrA internal ∼160–200 bp DNA fragment, as previously described (Malasarn et al., 2004). Genomic DNA from Shewanella sp. strain ANA-3 was used as a positive control. To clone the arsC-1Fas and arsC-2Fas genes, specific primers were designed based on both nucleotide sequences obtained from Fas genome and modified to include XhoI and HindIII restriction sites (Supplementary Table S1). The PCR assays were performed with the Phusion High-Fidelity DNA Polymerase (Thermo Scientific, F-350S) according to the manufacturer’s instructions. The following PCR conditions were used: initial denaturation at 95°C for 30 s, followed by 30 cycles of 98°C for 10 s, 56.1°C (arsC-1Fas) or 60.0°C (arsC-2Fas) for 25 s, and 56.1°C (arsC-1Fas) or 60.0°C (arsC-2Fas) for 1 min, and a final elongation at 56.1°C or 60°C for 3 min. Then, PCR products were visualized by electrophoresis and purified from the 1.5% agarose gel. Screening for recombinant colonies was made by PCR as previously described (Green and Sambrook, 2019) using the PCR conditions described above and the GoTag® kit (Promega, M3001).
Cloning, heterologous expression of arsC genes, and evaluation of As(V) resistance
To confirm if Fas ArsC confers resistance to As(V), the arsC-1Fas and arsC-2Fas genes were amplified as described above. The purified PCR products were subjected to A-tailing with Taq DNA polymerase and to ligation into a T-vector (pGEM®-T Easy Vector, Promega, cat. n° A1360). The ligation product was used to transform E. coli JM109 (Competent Cells, Promega, cat. n° L2005) and recombinant clones were checked by sequencing. Plasmids with the arsC-1Fas and arsC-2Fas correct sequences were purified by miniprep (Wizard® Plus SV Minipreps, Promega, cat. n° A1360) and double digested with XhoI and HindIII (Thermo Scientific™, cat. n° ER0691 and ER0501, respectively) to release the inserts, which were subsequently purified and ligated upstream the His-tag into the expression vector pTrcHis2 (Invitrogen™, cat. n° V36520). The recombinant vectors were transformed into the E. coli WC3110 ΔarsC strain. Positive clones were cultured in LB medium with ampicillin 50 μg/ml for 12 h at 37°C. The expression of both arsC genes was induced with IPTG 1 mM and the conferred ability to growth in the presence of 0, 0.5, 1, 1.5, 2.5 and 5 mM arsenate was monitored by OD600 and compared to a clone of E. coli WC3110 transformed with the pTrc-lacZ used as control. The specific growth rate (μ) was calculated with the equation: μ = (lnX-lnX0)/(t-t0), where X and X0 represent the cell concentration or OD600, and t and t0 the time. The doubling time (td) was determined using the equation: td = ln2/μ.
Bioinformatic analysis
The genome of Fas was sequenced on an Illumina MiSeq platform at MR DNA (Molecular Research LP, Shallowater, TX, United States) as described in Serrano et al., 2017. Sequencing the library obtained with Nextera DNA Sample Preparation Kit, Illumina, an estimate of 20,000 (2 × 300-bp paired-end) reads with >50-fold coverage was retrieved. A draft genome of ~5.1 Mbp was assembled de novo using Newbler v2.0.01.14 and contained 57 contigs with 4,780 genes identified using RAST (Brettin et al., 2015). The genomes of Fusibacter sp. strain 3D3 (BDHH00000000.1), F. ferrireducens strain Q10-2T (JADKNH000000000.1), F. paucivorans strain SEBR 4211T (JAHBCL000000000.1), F. tunisiensis strain BELH1T (JAFBDT000000000.1), Fusibacter sp. strain A1 (JABKBY000000000.1) were obtained from the NCBI database and also annotated on the RAST platform using default settings.
The genome sequence of Fas was screened to search for genes encoding components and regulators of arsenic redox and transport system, as well as those associated with thiol redox systems and energy metabolisms (Serrano et al., 2017). Curation of genes of interest was performed by reciprocal analysis against each other and the sequences available in the public databases to establish similarities and differences regarding gene identity, structure and function, gene context and control signals (Altschul and Lipman, 1990; Altschul et al., 1997; Sigrist et al., 2002; Tatusov et al., 2003; Punta et al., 2012). The sequence collections available on the NCBI (Benson et al., 2009) and the Comprehensive Microbial Resource of the J. Craig Venter Institute (Rockville, MD, United States; Davidsen et al., 2010) websites facilitated comparative genomic studies with other organisms of interest whose genome sequencing had already been completed.
For the classification of the Fas ArsC into families, depending on different thiol-disulfide relay mechanisms, its sequences were compared with known and putative arsenate reductases dependent of Grx or Trx proteins from E. coli (P0AB96), Staphylococcus aureus (P0A006), Fusibacter sp. 3D3 (WP_069871881, ArsC-1 and WP_069871901, ArsC-2) and Citrobacter sp. TSA-1 (PAX80297; PAX80313 and PAX80519). The protein subcellular localization was predicted with PSORTb v3.0.2.1 To determine the presence of transmembrane regions, DeepTMHMM2 was used.
For the verification of the EtfB (electron transfer flavoprotein subunit beta) domain conservation across Fusibacter, previously known proteins from Geobacter metallireducens GS-15, Thermotoga maritima MSB8, Clostridium ljungdahlii PETC, Rhodopseudomonas palustris BisA53, Acetobacterium woodii WB1, Clostridium kluyveri DSM 555, Acidaminococcus fermentans VR4, and Megasphaera elsdenii T81 were used (Garcia Costas et al., 2017). The protein sequences were obtained from the NCBI database, aligned by MUSCLE using the default parameters (Edgar, 2004) and visualized by CLC Genomic Workbench 8.5.1 (Qiagen). Subsequently, using BLAST on the RAST platform, EtfB-type proteins were searched in Fusibacter genomes, and the results were verified by BLASTp in the NCBI database. A similar procedure was applied to the products of the remaining genes.
The 16S similarity was determined with BLAST Global Alignment using the sequences from Fusibacter sp. strain 3D3 (GCA_001748365), F. paucivorans (NR_024886), F. tunisiensis (GCA_016908355), F. bizertensis (KJ420408), and F. fontis (LM999901).
Results
Microbial growth
Fas grew optimally at temperatures ranging from 20 to 37°C, with the lower doubling time (td) observed at 30°C (Figure 1A) and under neutral (6–8) pH conditions (Figure 1B). Fas grew in synthetic medium containing up to 50 g L−1 of NaCl, and the optimum was 10 g L−1 (Serrano et al., 2017). No growth differences were detected at increasing SO4−2 concentrations (Figure 1C). The highest specific growth rate (μ) was observed at 2–8 mM of As(V) and pH 7 in anaerobiosis, but it grew in the range of 0.5 to up to 16 mM of As(V; Figure 1D). The lowest concentration of As(V) that completely prevented growth (MIC) of Fas was 24 mM. Liquid cultures reached total bacterial numbers between 3.1×108 and 6.5×108 cells mL−1 at the stationary phase (Figure 2).
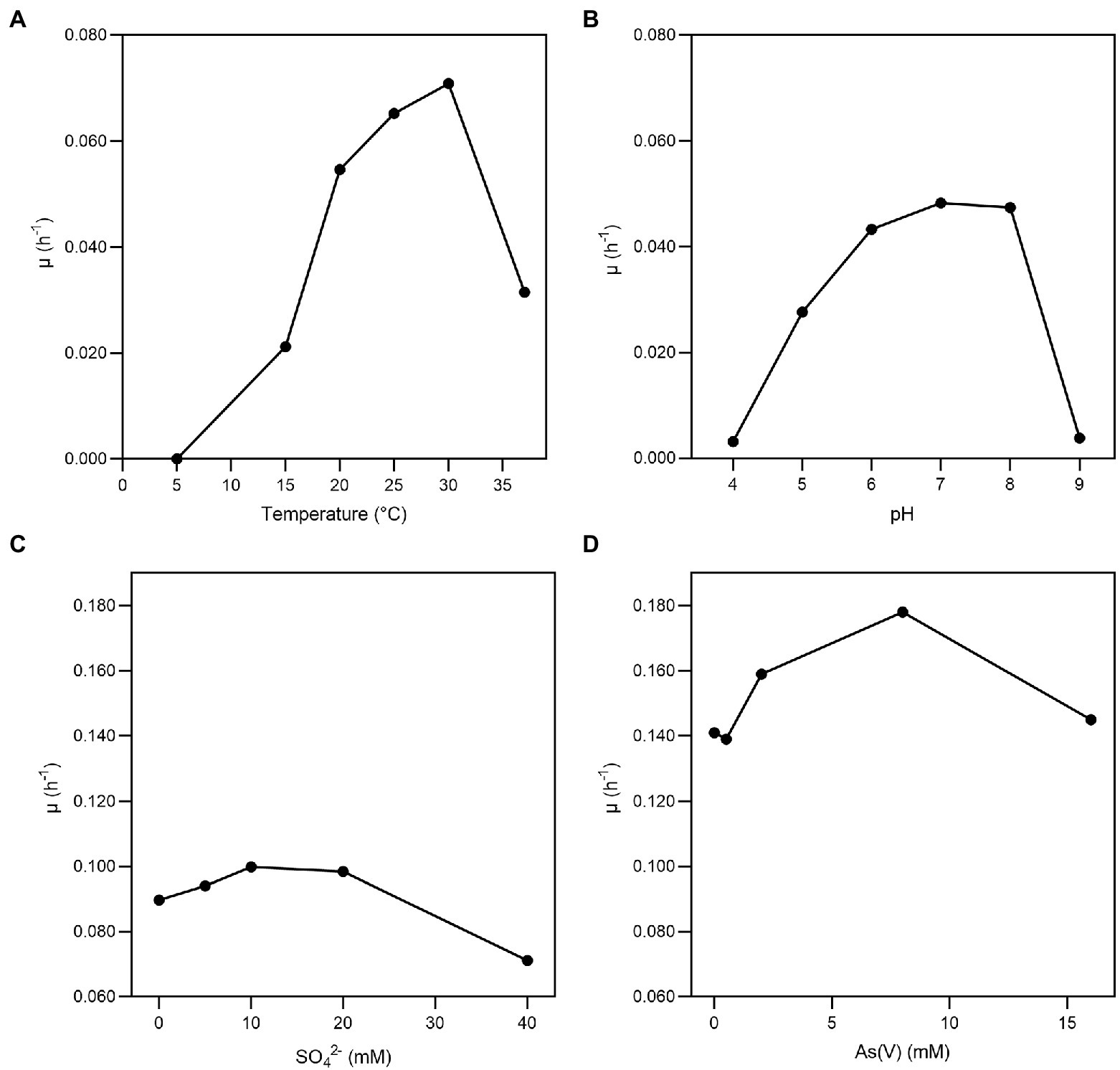
Figure 1. Growth of Fas in arsenate-containing medium. Specific growth rate (μ) of Fas growth on increasing temperatures (A), pH (B), concentrations of SO42− (C), and As(V) (D). Optimum temperature (30°C), pH (7), and concentrations of As (2 mM) and sulfate (20 mM) were used in the experiments except when different values of the corresponding variables were analyzed.
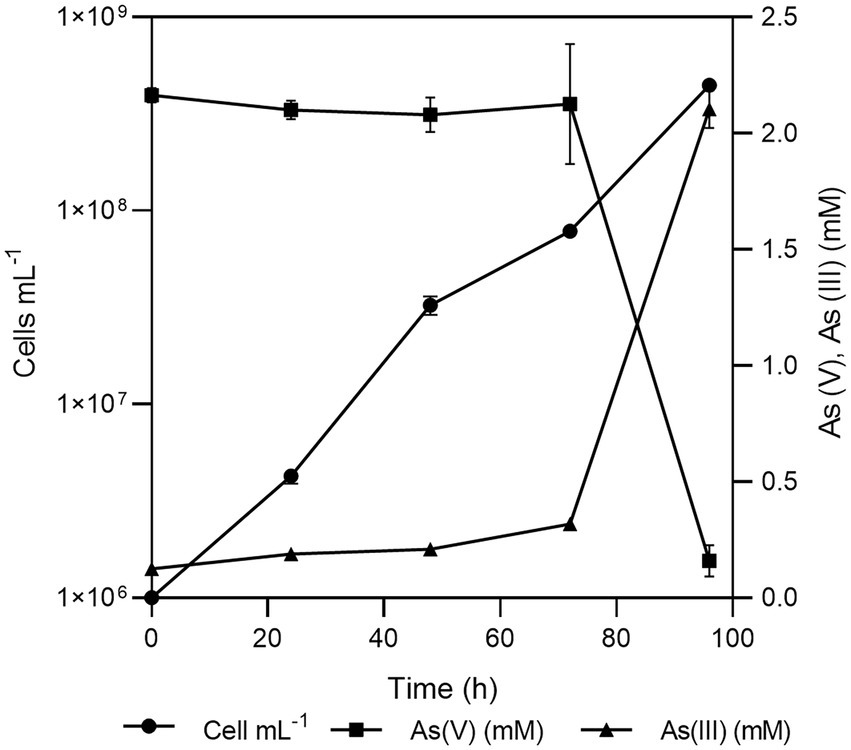
Figure 2. Growth of Fas in Newman-modified minimal medium with lactate (10 mM), sulfate (20 mM), arsenate (2 mM), yeast extract (0.1%), NaCl (10 g L-1), and cysteine (1 mM). Error bars represent the standard error of the mean of triplicate cultures. Sterile control experiments were also performed but the results were not shown for clarity.
No significant changes were noticed in the doubling time in minimal medium plus arsenate (2 mM) by the addition of sulfate (0 to 20 mM; Figure 1C; Serrano et al., 2017). Fas grew with a doubling time of 0.35 h in minimal medium plus arsenate (2 mM) and sulfate (20 mM) as electron acceptors, which increased to 4 h when not supplemented with arsenate (Figure 1D).
Features of Fusibacter sp. strain 3D3 metabolism
Substrates and products
The strain was not able to grow in aerobiosis but grew in anaerobiosis on lactate in the presence of sulfate and arsenate as electron acceptors. Defined as a heterotrophic strain, Fas could use lactate, glucose and tryptone and required yeast extract to grow (Table 1). Growth without the addition of electron acceptors was successful up to the second subculture (data not shown) as it was in the previously reported culture medium for Fusibacter (Ravot et al., 1999). Besides, the highest As(V) to As(III) reduction ratio was evidenced between 72 and 96 h (Supplementary Figure S1).
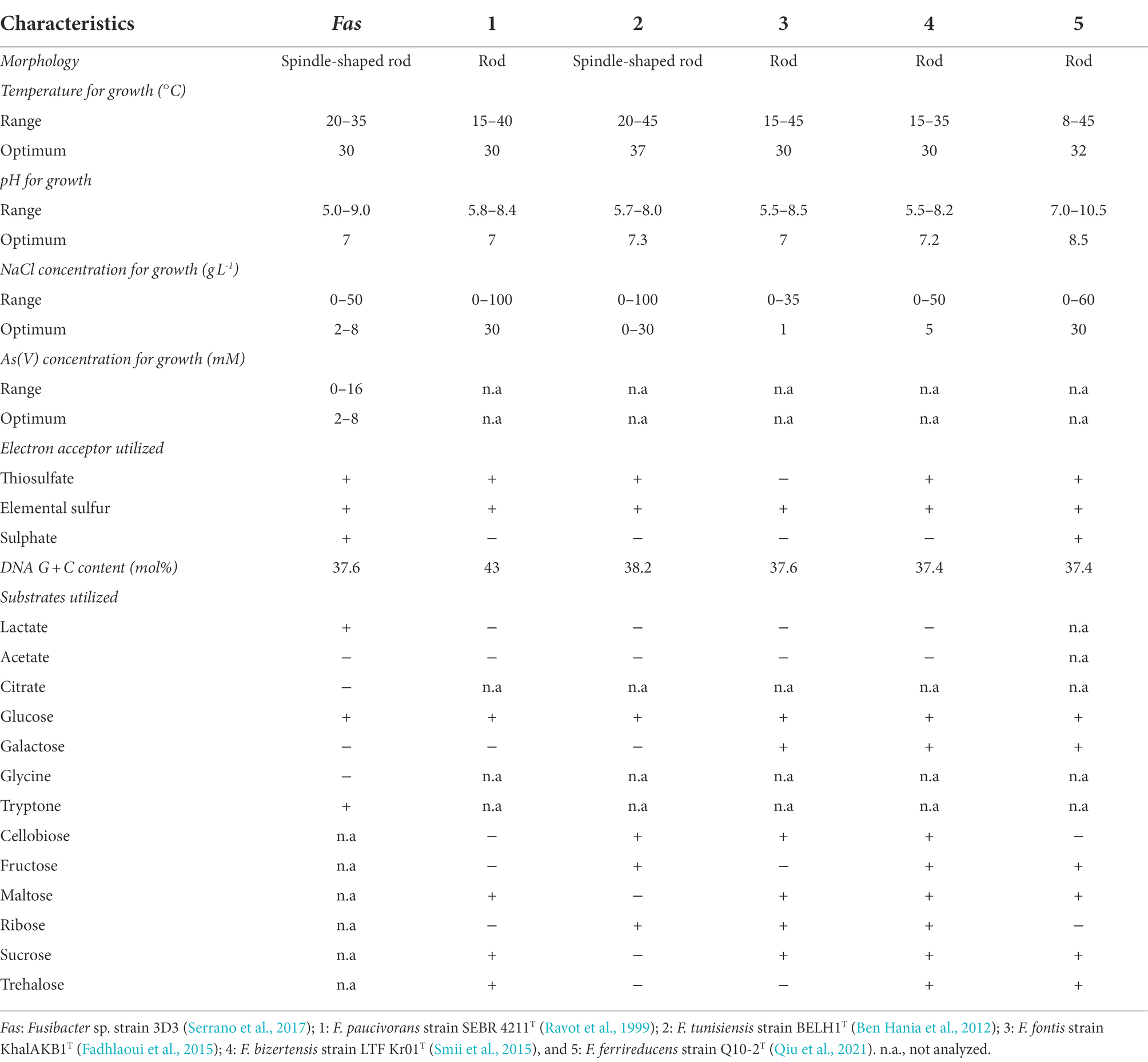
Table 1. Comparison of the characteristics of Fusibacter sp. strain 3D3 and other Fusibacter type strains from literature, with sequenced genomes.
Fas can be differentiated from F. paucivorans, F. tunisiensis, F. fontis, F. bizertensis and F. ferrireducens by its use of lactate as substrate, of sulfate as electron acceptor, the NaCl concentration for growth, its genomic DNA G + C content (Table 1) and its phylogeny (Qiu et al., 2021). Concerning the resistance to arsenic, to our knowledge it was not reported for other isolated members of the genus.
Lactate was consumed (12.3 mM, with 0.08 mM of acetate, and 3 mM of butyrate formed) while arsenate (1.85 mM) and sulfate (2.3 mM) were reduced. The amount of arsenite formed could not be determined quantitatively, as it tended to precipitate as yellow arsenic sulfide. On the other hand, sulfate reduction by Fas was demonstrated by sulfide and arsenic sulfide mineral production. Interestingly, neither sulfate nor thiosulfate reduction was involved in energy conservation as it has been reported for other members of the Fusibacter genus (Ravot et al., 1999; Ben Hania et al., 2012).
The ability of Fas to use lactate as electron donor when reducing arsenate suggests that arsenate respiration supports its growth. However, the arsenate reduced/lactate oxidized molar ratio observed was 0.32 ± 0.044 and the acetate produced/lactate consumed ratio was 0.23 ± 0.05, when the theoretical values predicted for isolated arsenate reduction reactions when lactate is transformed to acetate by respiring microorganisms are 2 and 1, respectively (Macy et al., 2000). In addition, the concomitant reduction of sulfate and the production of an arsenic sulfide precipitate does not allow an accurate quantification of arsenite and sulfide during Fas growth (Serrano et al., 2017).
Assessment of specific sulfur species source for growth
The lack of differences in the sodium sulfate dose curve led us to study the role of sulfur sources in arsenate reduction. Fas cultures with sodium sulfate, sodium thiosulfate and elemental sulfur were performed and combined with organic sulfur such as yeast extract and cysteine, both supplements required in Newman’s medium (Supplementary Figure S1). Culture without any source of inorganic sulfur was also carried out. All cultures were performed with 2 mM As(V).
The behavior of Fas cultures amended with sodium sulfate and sodium thiosulfate did not show significant differences, reaching the highest level of arsenate reduction with Yeast/Cys complete medium. Furthermore, the intake of cysteine (empty square) as unique source of organic sulfur appeared to rise up to 50% of the total arsenate reduction in all conditions at 96 h, and it was especially evident when inorganic sulfur was absent. Cultures supplemented only with yeast extract (filled triangle) induced lower arsenate reduction ratio than cysteine in all experiments. Growth (cell number) and sulfide production were also measured (Supplementary Figure S1).
Assessment of the role of proton/sodium gradient
The addition of 20 μM of sodium ion ionophore ETH2120 did not have a significant influence on the growth of the strain with lactate as the electron donor whether sulfate-arsenate (Figure 3A squares) or only arsenate (Figure 3B squares) were present as electron acceptors. On the other hand, 20 μM of the protonophore TCS completely inhibited the growth on lactate-sulfate-arsenate (Figure 3A triangles) and lactate-arsenate (Figure 3B triangles). Growth experiments showed that lactate-sulfate-arsenate and lactate-arsenate were insensitive to the Na+ ionophore ETH2120 but were highly sensitive to the protonophore TCS.
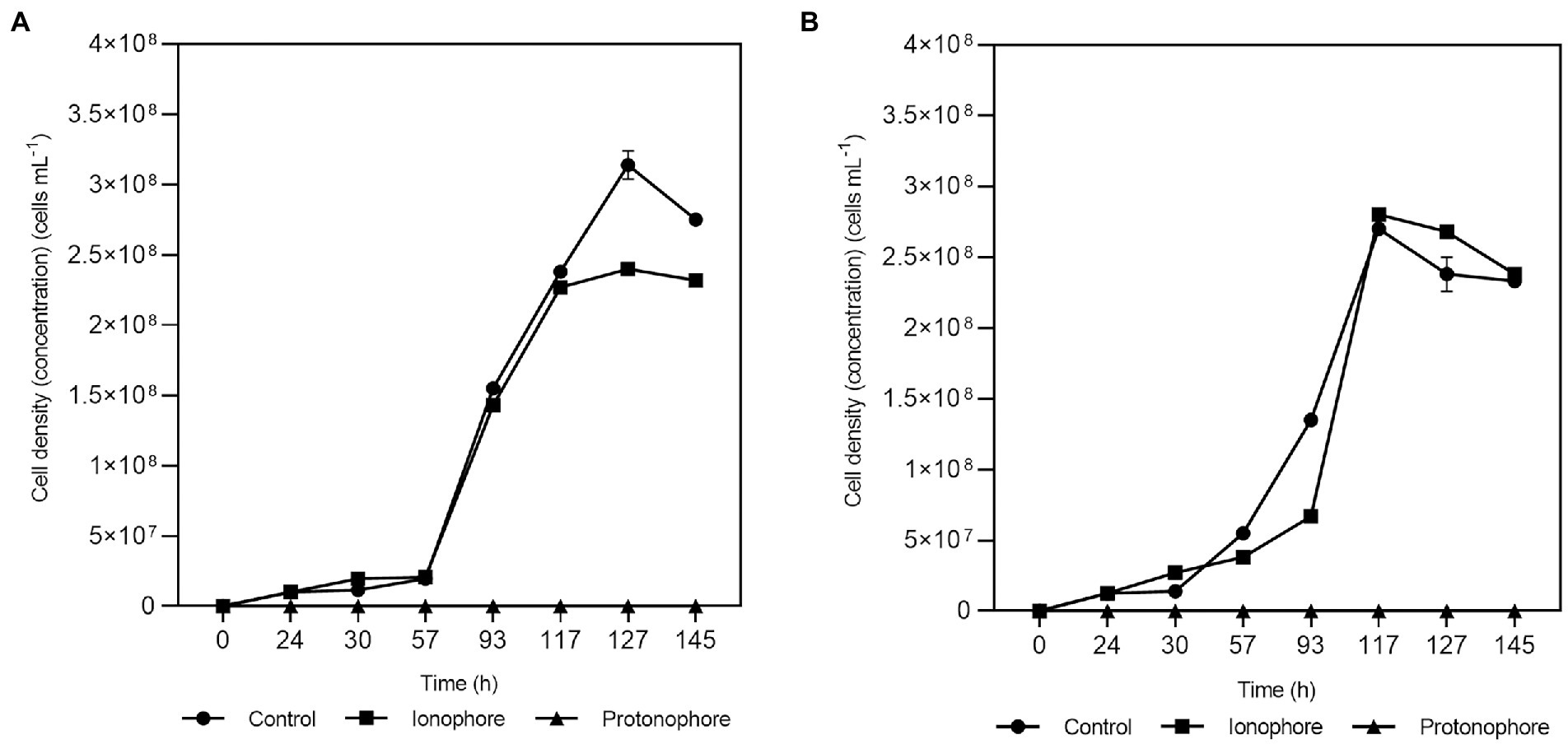
Figure 3. Effects of ETH2120 and TCS on Fas growth. Growth curves with lactate-sulfate-arsenate (A) and lactate-arsenate (B), with addition of 20 μM ETH2120 as ionophore (■), 20 μM TCS as protonophore (▲) or no addition (●). Error bars show standard deviation of duplicates.
In the protonophore test, the resting cells were also decreasing and demonstrated to be highly sensitive to TCS suggesting that Fas needed the proton gradient for energy generation. Moreover, the inability of the strain to grow in the presence of TCS is consistent with the role of that gradient in the generation of a proton motive force.
Assessment of the Trx system in the response to arsenate exposure
To gain insight into the thiol redox system involved in arsenate reduction and considering that the reductase ArsC of Fas was inferred by homology to be from the Trx/TR-dependent class, Trx (Figure 4A) and TR (Figure 4B) activity analysis were performed with cellular extracts. In addition, we compared the Trx activity with representative of Gram-positive (B. subtilis) and Gram-negative (E. coli) bacteria. The activity of Trx and TR increased after the exposure to arsenate (Figure 4). The Trx enzymatic activity was more than 7-fold higher than that determined in E. coli ATTC 4468 and B. subtilis HB 7038 without As(V; data not shown). A significant increase (p < 0.01) of Trx at 30 min (1.5-fold) and 8 h (2.3-fold) of As(V) exposure, and TR activities (2 and 4-fold at the same times) were observed. The results evidenced that Trx and TR were induced after the As(V) exposure.
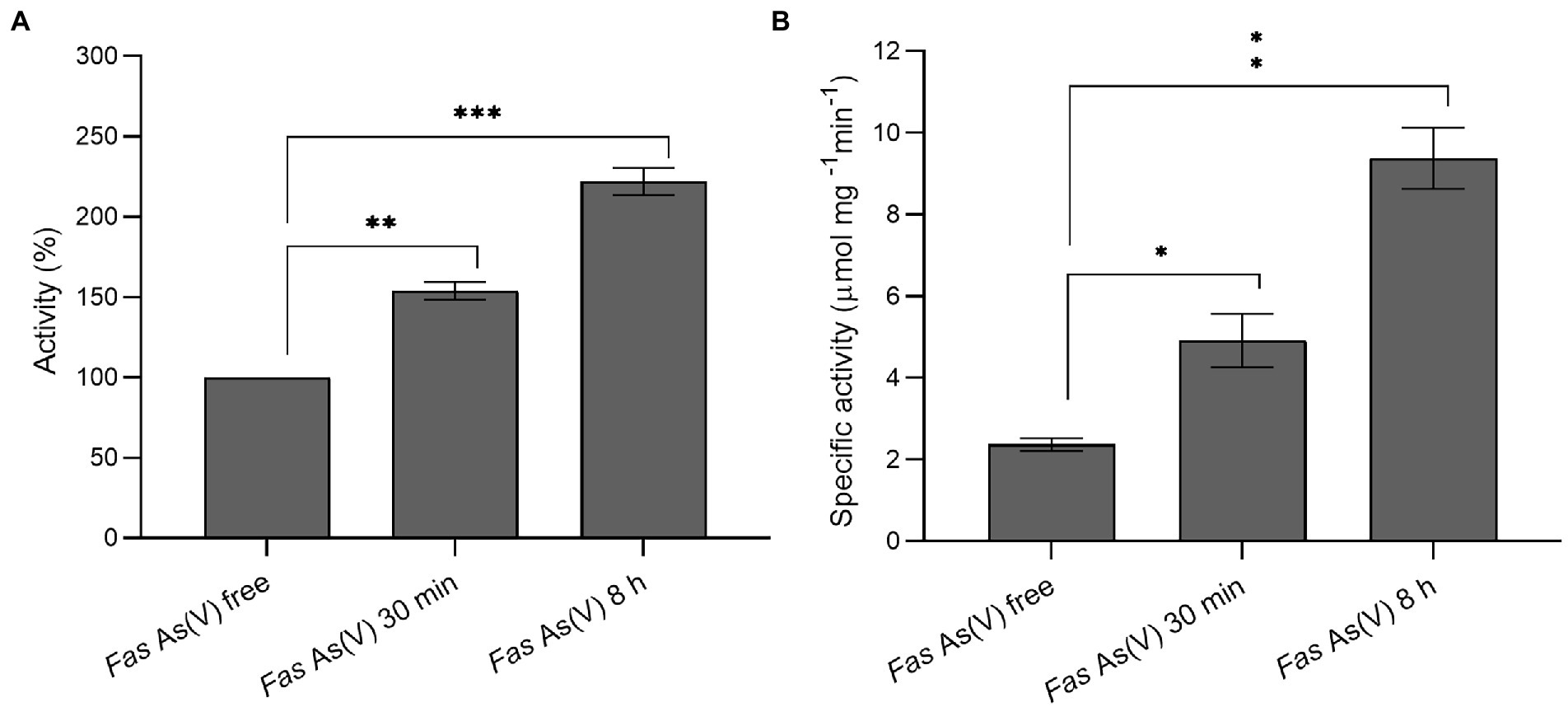
Figure 4. Measurement of thioredoxin and thioredoxin reductase activities. (A) Thioredoxin activity of Fas cells exposed to As(V) compared to the activity of cells grown without As(V). (B) Specific thioredoxin reductase activity of Fas before and after As(V) exposure. *p < 0.05; **p < 0.01; ***p < 0.001.
Genomic features
Genes involved in arsenate reduction and energy metabolisms
As noted in Tables 2, 3 and Supplementary Table S2, Fas draft genome sequence has genes coding for proteins involved in electron bifurcation and in arsenic, phosphate, and sulfur metabolisms. Arsenic detoxification genes (arsACMR; acr3) are clearly present in Fas genome (Serrano et al., 2017). Fas contains genes coding for two putative cytoplasmic arsenate reductases with only 32% of identity in their aminoacid sequences, both clustering with genes coding for the thioredoxin-coupled family (Supplementary Figure S2). We performed an in silico analysis of the arsC-1Fas and ArsC-2Fas sequences, together with other previously known/characterized ArsC proteins, to determine their possible cellular localization. The results of the analysis indicate that they are soluble proteins possibly located in the cytoplasm (Supplementary Table S2).The revisited genomic context of arsC-2 (arsD > arsR-2 > pno > acr3 > arsC-2; Serrano et al., 2017) includes genes encoding for an arsenical resistant operon repressor (ArsD), a transcriptional regulator (ArsR), a 4Fe-4S ferredoxin (Pno, pyridine nucleotide-disulfide oxidoreductase NADH dehydrogenase), an arsenite efflux permease (Acr3), and the arsenate reductase (ArsC-2; Slyemi and Bonnefoy, 2012; Table 2). ATPase encoding gene that provide energy for arsenite efflux (arsA), included in the canonical ars operon of other Clostridiales, was also found in Fas even in another genomic context. Otherwise, the genomic context of arsC-1Fas revealed the presence of genes coding for ferredoxin and a redox-active disulfide protein (thioredoxin). Besides, two TR encoding genes were also identified in the Fas genome by the BLAST analysis (Table 3; Supplementary Table S3).
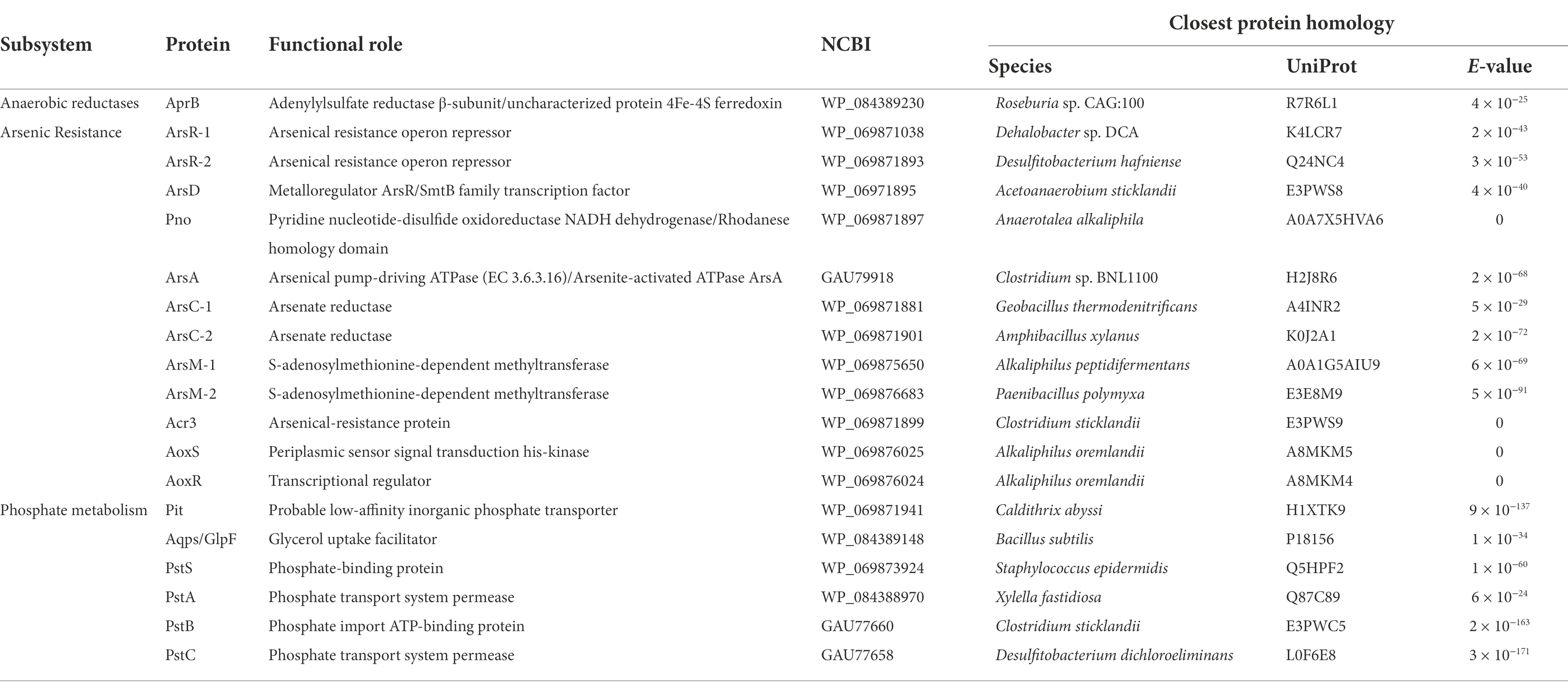
Table 2. BLAST results (UniProtKB reference proteomes+SwissProt databases) of predicted proteins related to arsenic resistance, phosphate, and sulfur metabolisms in Fas.
The dissimilatory arsenate reductase arrAB gene cluster, involved in anaerobic respiration using As(V) as electron acceptor, was not found in Fas as it has been previously reported (Serrano et al., 2017). However, several genes predicted to be involved in the synthesis of the molybdenum cofactor included in the known catalytic site of ArrA (Slyemi and Bonnefoy, 2012) and in other cytoplasmic iron–sulfur proteins that catalyze ferredoxin-dependent redox reactions (Buckel and Thauer, 2013) were identified in the genome of Fas.
The NADH-dependent reduced ferredoxin:NADP+ oxidoreductase, α and b subunits (NfnAB), evidenced by BLAST analysis against the Pyrococcus furiosus proteins (Lubner et al., 2017), is present in Fas and is conserved in the genomes of the Fusibacter genus. NfnAB is an electron bifurcating enzyme complex which couples the reduction of NADP+ with reduced ferredoxin (Fdred) and the reduction of NADP+ with NADH in a reversible reaction (Buckel and Thauer, 2018a).
Bacteria transport inorganic phosphate through the constitutive low-affinity Pit transporter (Phosphate inorganic transport), however it is prone to transport As(V), when present. The inducible high-affinity phosphate transport system Pst (Phosphate specific transport; Vera et al., 2008; Slyemi and Bonnefoy, 2012) solve this issue. Both systems are present in the Fas genome.
The transmembrane ATP synthases (F0F1-ATPase complex) which are involved in ATP synthesis by obtaining the energy of a transmembrane gradient created by the difference in protons (H+), and in ATP hydrolysis in the reverse direction reactions, are encoded in the Fas genome (Supplementary Table S4). The order is conserved in the genomes of the Fusibacter genus (subunits I, A, C, C, B, Delta, Alpha, Gamma, Beta, Epsilon).
In addition, the occurrence of the genes rnfC, D, G, E, A, B reported as encoding for the membrane-associated ferredoxin-dependent Rhodobacter nitrogen fixing (Rnf) complex was revealed by BLAST analysis in Fas genome, and in the whole genus. The Rnf complex is responsible for transmembrane Na+/H+ transport (Müller et al., 2008) and for Na+/H+ gradient harvesting (Biegel et al., 2011).
A search in Fusibacter genomes for genes involved in the fermentation process (Gutiérrez-Preciado et al., 2018) revealed the occurrence of genes coding for an aldehyde dehydrogenase and butanoate metabolism in most of them, while genes involved in lactate/pyruvate metabolism were not present neither in F. tunisiensis nor in F. paucivorans. Genes codifying for pyruvate decarboxylase, alcohol dehydrogenase (cytochrome c), and proteins involved in the citrate cycle were absent (Table 4).
Searching for etfB genes in the Fusibacter genomes allowed us to find genomic contexts that would code for proteins involved in electron bifurcation. To verify the presence of key features (motifs 1 and 2) of the electron bifurcating EtfBs, an alignment of putative Fusibacter EtfBs and previously characterized proteins was performed (Supplementary Figure S3). We found that all Fusibacter genomes code for group 2A EtfBs, Fas and F. ferrireducens also code for group 2B, while only F. paucivorans code for group 2C elements.
To better understand their possible role in Fas, we compared the genomic contexts of the etf encoding genes in Fusibacter (Figure 5). All the analyzed genomes contain the gene encoding for the electron transfer flavoprotein subunit beta followed by the alpha subunit encoding gene. We found at least one copy of the genes encoding for EtfA, EtfB, and a putative butyryl-CoA dehydrogenase (Bcd) in Fusibacter genomes. Interestingly, bcd is always located upstream the etf genes cluster.
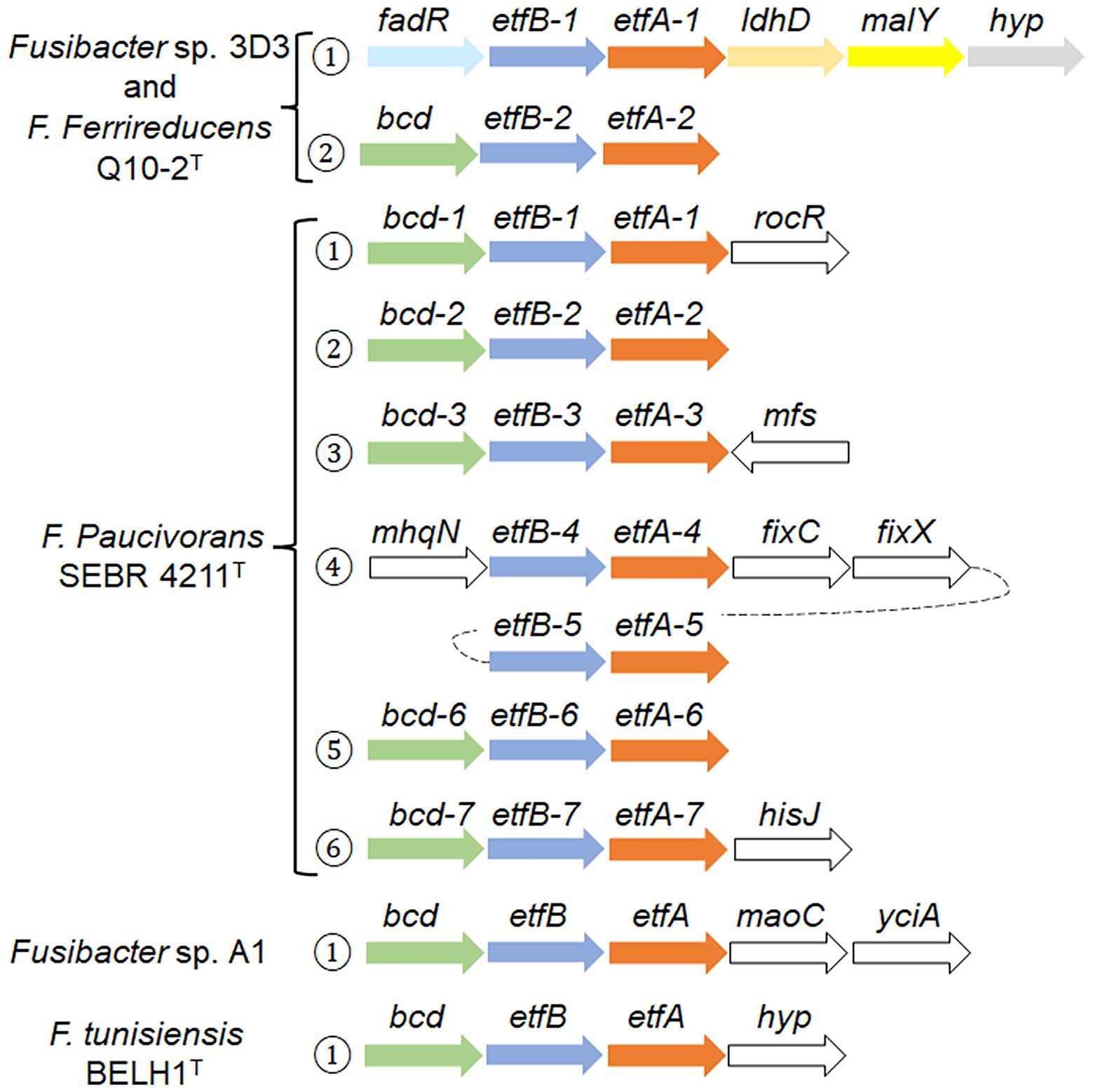
Figure 5. Genomic context of etf related genes in Fusibacter. Numbers in circles indicate the occurrence of the etf copies. The arrows represent the orientation of the gene (size is not at scale), and the same colors indicate homology, except white. The gene products are described in the text and the gene product accession numbers are listed in Supplementary Table S5.
Fas and F. ferrireducens have two identical genetic arrangement. In context 1, fadR (which codifies for a transcriptional regulator) is located upstream etfB-1 and etfA-1 genes, and downstream of both are ldh that codifies for a lactate/glycolate dehydrogenase (COG0277), malY that codifies a putative pyridoxal 5′-phosphate (PLP)-dependent C-S lyase (COG1168) and a gene that codifies for a hypothetical protein conserved in both genomes, while in context 2, only a bcd gene was identified upstream etfBA-2.
Surprisingly, F. paucivorans contains seven copies of the etfB-etfA pair in six different contexts. Contexts 1, 2, 3, and 6 present the upstream arrangement with the bcd gene. In context 4, mhqN, which codifies for a nitroreductase family protein (cd02137), is found upstream etfBA-4 while fixC and fixX are downstream and code for a flavoprotein dehydrogenase (COG0644) and a ferredoxin-like protein (COG2440) respectively. The fifth copy etfBA-5 was identified downstream fixX. In addition, F. paucivorans has four orphan etfB genes, possibly belonging to the 2C2 group due to its phylogeny and because it does not have any etfA or etfB genes fused in a single open-reading frame, as it has been described in the group 2C1 (Garcia Costas et al., 2017). Independently, we also identified in F. paucivorans genes coding for a nitrogenase reductase and maturation protein (nifH), the regulatory proteins P-II (glnA and glnB) and α and β subunits of the nitrogenase (nifD and nifK). This opens the possibility that in this Fusibacter species some etf genes participate in nitrogen fixation. Indeed, no other Fusibacter possesses nitrogen fixation genes (results not shown).
Fusibacter sp. A1 and F. tunisiensis had only one specific genomic context with etf-related genes. In Fusibacter sp. A1, downstream etfA we found maoC, that codifies for an acyl dehydratase (COG2030), followed by yciA, coding for an acyl-CoA hydrolase (COG1607), both related to lipid transport and metabolism.
Detection of dissimilatory arsenate reductase arrAB genes
To corroborate the above mentioned, that the dissimilatory arsenate reductase arrAB gene cluster were not found in the draft genome sequence, an attempt was made to amplify these genes using specific primers, although an amplification product was not obtained either (Supplementary Figure S3). This suggests that a different mechanism, independent of ArrAB, is conferring the ability to obtain energy from arsenate reduction.
Heterologous expression of arsC genes
Two genes encoding arsenate reductases were identified in the Fas genome sequence and specific primers were designed for their amplification by PCR. The amplified genes (arsC-1Fas and arsC-2Fas; Supplementary Figure S4) were first cloned in the pGEM-T cloning vector, then released through enzymatic DNA digestion (Supplementary Figure S5) and ligated into the pTrcHis2A expression vector. The presence of the insert in the expression vector was checked by colony PCR (Supplementary Figure S6) or releasing the insert through DNA digestion of plasmids (Supplementary Figure S7). The activity of the gene product coded by the insert was tested by growing the recombinant E. coli WC3110 in the presence of As(V; Figure 6). Complementation of the E. coli WC3110 ΔarsC strain with the insert of both putative arsCFas genes evidenced changes in As(V). A higher resistance to As(V) was conferred by ArsC-2Fas compared to ArsC-1Fas. The control strain E. coli WC3110 overexpressing lacZ did not grow at concentrations higher than 1 mM of As(V). Growth of E. coli WC3110 strain without insert was not observed. These biological data are the first metabolic evidence needed to confirm the existence of the proposed metabolism in Fas.
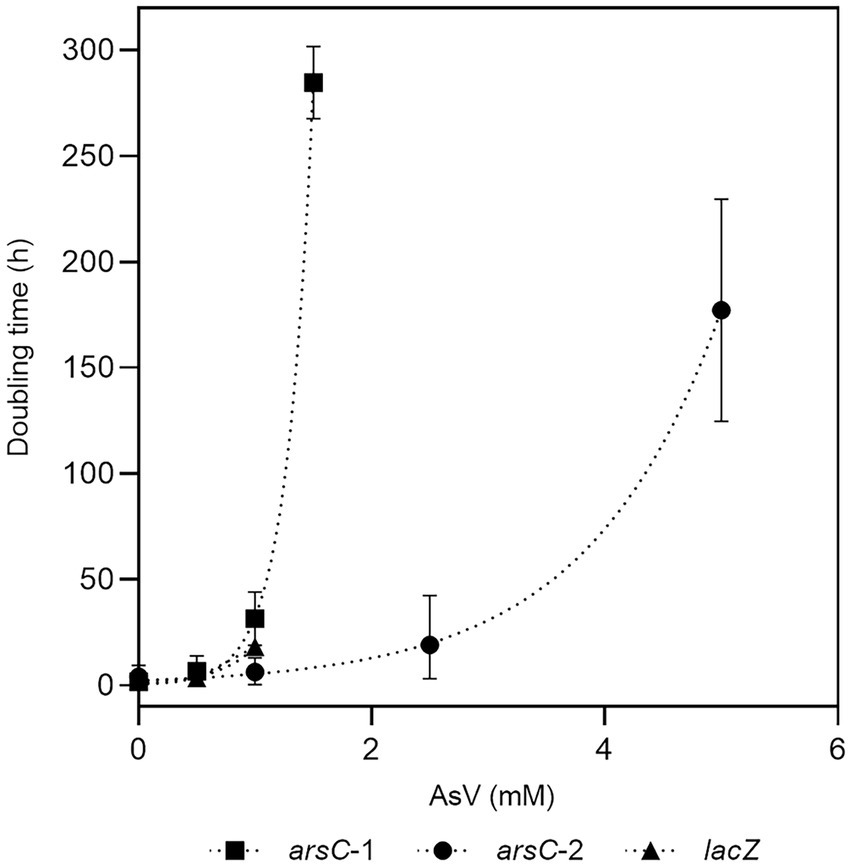
Figure 6. Evaluation of resistance to As(V) conferred by arsC-1 and arsC-2 from Fas. Growth of E. coli WC3110 ΔarsC strain complemented by arsC-1Fas SHT, arsC-2Fas SHT or lacZ genes in presence of As(V).
Discussion
Phylogenetic analysis performed with the 16S rRNA genes had formerly grouped Fas inside the Gram-positive Fusibacter genus (Serrano et al., 2017). The comparison of the 16S rRNA sequences of Fas and other Fusibacter type strains revealed an identity of 98% with F. ferrireducens, 94% with F. paucivorans, F. tunisiensis and F. fontis, and 93% with F. bizertensis. Furthermore, The in silico average nucleotide identity (ANI) with its closest relative is 80.1% (Qiu et al., 2021). This gives us an additional indication that isolated 3D3 is a member of the Fusibacter genus.
Taking together the observed growth features of Fas compared with other species of the Fusibacter genus (Table 1) and the insights into their genome sequences (Tables 2, 3; Supplementary Table S1) we can propose a rationale to justify the singularity of the energetic metabolism of Fas. It grows strictly in anaerobiosis by reducing arsenate and using lactate as electron donor and its growth is improved by increasing arsenate concentration (Figure 1), being 2 mM the optimum level. To date, arsenic metabolism was not reported for the other Fusibacter species. Despite the arsenate reducing activity, the dissimilatory arsenate reductase arrA gene was not detected neither by the Fas genome sequence analysis (Serrano et al., 2017) nor by PCR assays (Supplementary Figure S3). Furthermore, neither arrC [which encodes the membranous subunit suggested to play the role of menaquinone oxidation and is present in some arsenate reducing bacteria (van Lis et al., 2013)], nor omc (encoding an outer-surface, octaheme c-type cytochrome), nor cymA genes (encoding a membrane-bound MKH2 oxidizing protein and reported in arsenate respiring Shewanella sp. strains; Murphy and Saltikov, 2007; Kim et al., 2011) were evidenced in the Fas genome (Table 2). The heterologous expression on E. coli WC3110 ΔarsC strain has allowed us to confirm that ArsC-1Fas and ArsC-2Fas are functional and confer arsenic resistance (Figure 6). Both arsCFas genes belong to the Enterobacterial clade one (Zegers et al., 2001) and, therefore, encode a TR-dependent class of ArsC. In addition, the enzymatic analysis revealed a high Trx and TR activity in cells cultured with As, supporting the inference about the Trx dependence of the ArsC of Fas. As has been suggested before, Trx and TR provide early responses to oxidative stress in Leptospirillum ferriphilum. However, contrary to the observed in other microorganisms (Norambuena et al., 2012), a decrease in Trx activity was not observed in Fas after 8 h of exposure to As(V) probably because of the TR induction, that restores the Trx activity necessary for As(V) reduction. A 2.8-fold upregulation of Trx (Pandey et al., 2012) has been also observed in Anabaena after exposure to the oxidative stress generated by As (Pandey et al., 2012). Its ArsC enzyme has been classified, as well, inside the Trx dependent family (Pandey et al., 2013).
All the previously reported strains of the Fusibacter genus are fermentative bacteria (Ravot et al., 1999; Ben Hania et al., 2012; Fadhlaoui et al., 2015; Smii et al., 2015; Qiu et al., 2021). Interestingly, Fas can use lactate and glucose as substrates, while. F. tunisiensis, F. paucivorans, F. bizertensis, and F. ferrireducens cannot utilize lactate (Ravot et al., 1999; Ben Hania et al., 2012; Smii et al., 2015; Qiu et al., 2021). The genetic evidence agrees with the observed physiology on the culture conditions tested (Table 4). Furthermore, all the reported Fusibacter species have the ability to reduce sulfured nutriments (Ravot et al., 1999; Ben Hania et al., 2012; Fadhlaoui et al., 2015; Smii et al., 2015; Qiu et al., 2021). Sulfate reduction by Fas was demonstrated by sulfide and arsenic sulfide mineral production, and thiosulfate reduction was also positively checked. In addition, thiosulfate and sulfate were more efficient than sulfur to stimulate cell growth (Supplementary Figure S1) perhaps because of the low solubility of sulfur. Other Fusibacter species reduce thiosulfate and sulfur (but not sulfate or sulfite), and only F. ferrireducens shares with Fas the ability to reduce sulfate. Neither sulfate nor thiosulfate were involved in energy conservation in Fas as it has been reported for the other members of the Fusibacter genus (Ben Hania et al., 2012; Fadhlaoui et al., 2015). That feature could also be related to other cellular mechanisms present in microorganisms to cope with stress, such as arsenic stress, i.e., sulfur assimilation (Cleiss-Arnold et al., 2010). F. paucivorans growth experiments with sulfured nutriments revealed that the addition of thiosulfate relieved the inhibition produced by the H2 released by the glucose fermenting metabolism. In addition, a differential pattern of glucose fermentation products was observed in cultures with thiosulfate, represented by a decrease in butyrate levels together with an increase in acetate production (Ravot et al., 1999). As well as for other fermenting bacteria, those results confirm the Huber hypothesis that sulfur reduction plays a role of an electron sink reaction to prevent H2 accumulation from fermentation metabolism (Boileau et al., 2016). Therefore, the lactate/butyrate fermentation metabolism in Fusibacter should be regulated by the cellular redox state, resembling the reported for other Firmicutes (Detman et al., 2019). Interestingly, a redox-sensing transcriptional repressor gene encoding a protein whose DNA binding activity is modulated by the NADH/NAD+ ratio (Detman et al., 2019) is located downstream to the acetyl-CoA acetyltransferase encoding gene in Fas and in other Fusibacter genomes (data not shown). The acetyl-CoA acetyltransferase is in charge of the first step during the acetyl-CoA fermentation to butyrate pathway after the split in the three alternative fermentation pathways. Moreover, the results obtained from the growth experiments with and without addition of the protonophore TCS and the ionophore ETH2120 (Tremblay et al., 2012) revealed that Fas does require the formation of a proton gradient to get energy for growing on arsenate (Figure 3). In addition, the occurrence of genes encoding for the Rnf complex in Fas genome (Table 2) allows us to infer the capacity of Fas for energy conservation/utilization via proton translocating ferredoxin oxidation/reduction. Finally, the F0F1ATP synthase would couple ATP synthesis to the electrochemical gradient based on differences in the proton concentration generated.
In agreement with the genomic characterization of the ArsCFas inside the Trx-dependent class, the enzymatic analysis has shown an increased level of Trx and TR activities after arsenate addition (Figure 4). Besides, it is known that thioredoxin is also involved in sulfur assimilation, evidenced in the early response to arsenic and in maintaining the cellular redox state (Cleiss-Arnold et al., 2010).
Fas has all the known genomic resources for the pathway of lactate fermentation to acetate and butyrate in Firmicutes (Detman et al., 2019). Interestingly, the genomes of Fas and F. ferrireducens contain two genomic contexts that may be involved in the electron bifurcation process of the electron-transferring flavoproteins (EtfAB) type (Buckel and Thauer, 2018a). According to the model proposed for lactate and acetate transformation to butyrate (Detman et al., 2019), there must be a lactate dehydrogenase/EtfAB complex and a butyryl CoA dehydrogenase/EtfAB complex (Figure 7A). We propose that contexts 1 and 2 encode the elements for the transformation of lactate and butyryl CoA, respectively (Figure 5). Acetate production was observed in Fas and butyrate plus acetate production was confirmed in F. paucivorans (Ravot et al., 1999). Clostridium butyricum and Acetobacterium woodii were shown to transform lactate during fermentation by an enzyme complex of Ldh, EtfAB (Weghoff et al., 2015; Detman et al., 2019). Analysis of the genomes of C. butyricum, and A. woodii among others, showed conservation of the genetic context of at least the genes coding for these proteins (Weghoff et al., 2015;Poudel et al., 2018; Detman et al., 2019). Proteins related to butyryl CoA transformation involve butyryl-CoA dehydrogenase/electron transfer flavoproteins EtfA and EtfB (Li et al., 2008). This complex is also encoded in a conserved array (Poudel et al., 2018; Detman et al., 2019), like the genomic context 2 found in Fas (Figure 5). Furthermore, the occurrence of a NADH dependent reduced ferredoxin NADP+ oxidoreductase complex, the second type of FBEB complexes (Buckel and Thauer, 2018a), in Fas genome (Table 2) permits to hypothesize that energy for growth should be provided by the energetic link of cellular ferredoxin and NAD+ pools through the Rnf function to generate chemiosmotic potential when ferredoxin is higher than NADH level or, in reverse, for ferredoxin generation when NADH is higher (Westphal et al., 2018), for a more efficient metabolism in anoxic environments. In addition, Nfn could play the reported role of balancing the redox state of the pyridine nucleotide NAD(H) and NADP(H) pools and, in that way, favor the catabolic or anabolic reactions (Poudel et al., 2018). Also, the occurrence of multiple and different bifurcating (Bf) enzymes observed in Fas has been already detected in several Firmicutes genomes, and Bf-Ldh, Bf-Bcd and Nfn, that share NAD(H) and ferredoxin as common substrates, usually participate in those combinations (Lubner et al., 2017).
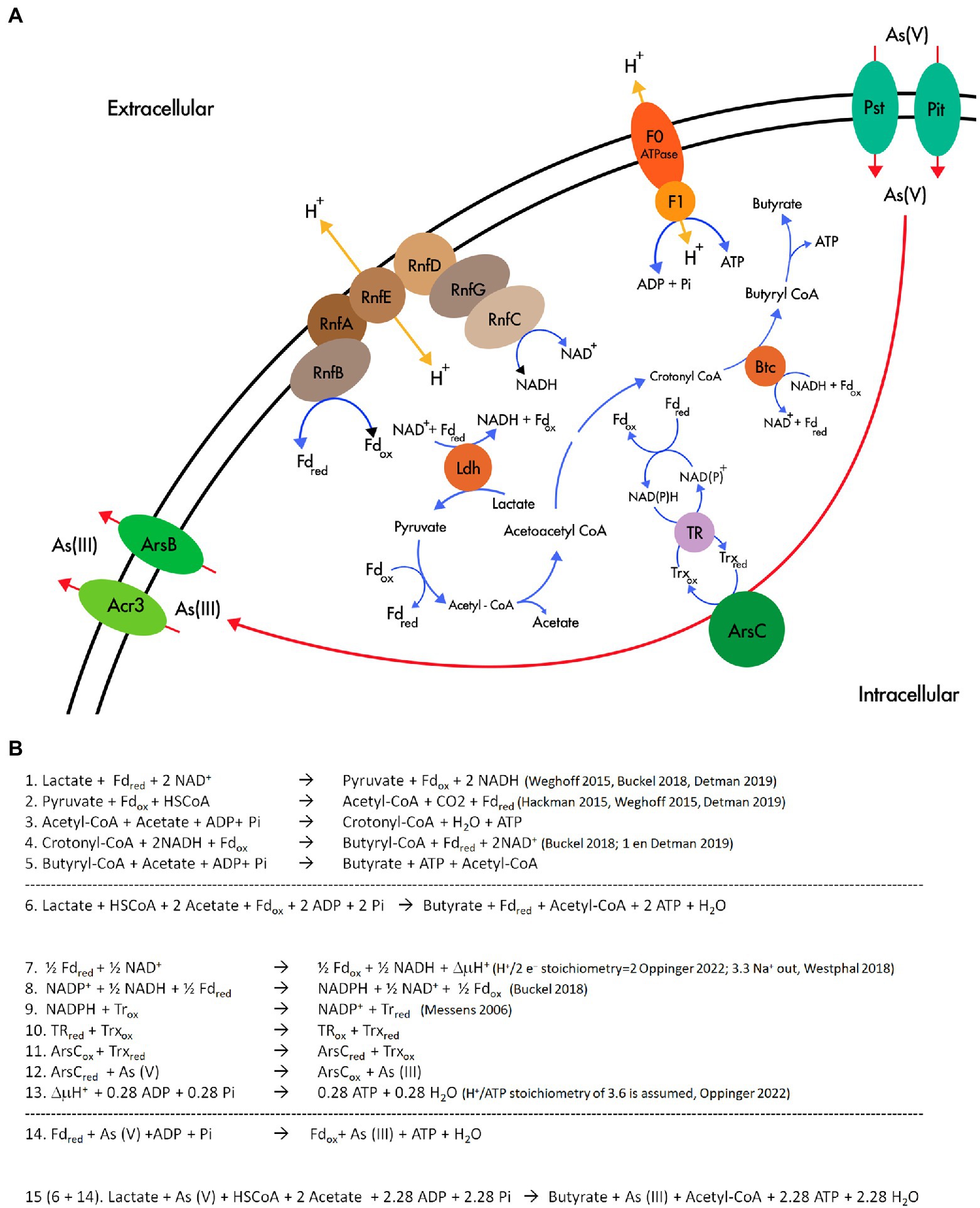
Figure 7. Flavin based electron bifurcation and its link with As(V) reduction. (A) Working model of the involvement of FBEB and the As(V) reduction in Fas. (B) Stoichiometry of the metabolism proposed in (A).
The revealed specialization for lactate fermentative metabolism present in Fas and already reported in Firmicutes (Detman et al., 2019), with the participation of FBEB and Rnf, supports the availability of NADH and Fdred and ATP generation (Figure 7A and Equations 1–5 in Figure 7B). NADH and Fdred should be the soluble electron carriers required for producing NADPH and starting the cascade of thiol reductases (NADPH→TR→Trx→ArsC; Messens and Silver, 2006) involved in As(V) reduction by ArsC Trx type of Fas (Figure 7A,B, equations 7–15). In that way, besides generating ΔμH+ coupled to ATP synthesis by ATP synthase, FBEB would conduct As(V) reduction by providing low-potential ferredoxin. The As(III) efflux pumps present in the Ars operon allow As(III) elimination and As4S4 precipitation outside the cells.
The analysis of the reported stoichiometry (Messens and Silver, 2006; Hackmann and Firkins, 2015; Weghoff et al., 2015; Westphal et al., 2018; Buckel and Thauer, 2018a) hints us that it is plausible that As(V) could play a role similar to that of CO2 in acetogenic bacteria (Buckel and Thauer, 2018b), of terminal acceptor of the electrons derived from reduced ferredoxin, the low potential electron carrier generated by electron bifurcation (Figure 7B).
This rationale allows us to formulate a hypothetical metabolism (Figure 7A) similar to the evidenced in other anaerobic microorganisms (Peters et al., 2018): Arsenate reduction provides additional energy to arsenate reducing fermenters independent of ArrAB for growing through a new mechanism that involves soluble ferredoxin electron carrier, FBEB complexes, the cytoplasmic ArsC, and the membrane-associated ion-translocating complex Rnf. As previously reported, this system could be regulated by the redox state (Detman et al., 2019). The energetic link of cellular NADH and ferredoxin should be the way in which the electrons reach As(V) in the cytoplasm, converting it in an electron sink/electron acceptor, similar to the role assigned to ferric iron in F. ferrireducens (Qiu et al., 2021).
Finally, the ecological relevance of the proposed metabolism was suggested by different approaches in two arsenic rich environments. A metagenomic analysis evidenced that the Trx-ArsC is much more diverse (assigned to Bacteroidetes, uncultured Proteobacteria, Firmicutes, Alphaproteobacteria, Deltaproteobacteria, Gammaproteobacteria, Actinobacteria, Verrucomicrobia, Spirochaetes, and Deinococcus-Thermus) than the Grx-ArsC (assigned only to Gammaproteobacteria) in the high altitude modern stromatolites at the base of the Socompa Volcano in the Argentinian Puna (Altiplano; Kurth et al., 2017). Furthermore, the predominant occurrence of genes predicted to encode Trx-ArsC instead of Grx-ArsC cytoplasmic arsenate reductase at As concentrations higher than 4 mg L−1 was evidenced by PCR amplification using three specific primer pairs for each type (Escudero et al., 2013). This work was done in a regional survey at the High Andes, the same environment where Fas was isolated from.
Data availability statement
The datasets presented in this study can be found in online repositories. The names of the repository/repositories and accession number(s) can be found in the article/ Supplementary material.
Author contributions
CD and MA conceptualized this study, designed the methodology, and conducted the research and formal data analysis. SM, NG, and SV contributed to the analysis and interpretation of the data. CD and MA wrote the first draft. All authors contributed to the review, editing, and revision of the article and approved the submitted version.
Funding
This article was funded by FONDECYT Project 1100795 from the Chilean National Commission for Science and Technology (CONICYT), FIC-R 2015/BIP 40013423-0 and the Research Support from Minera Escondida Ltda. Project 32002137.
Acknowledgments
The authors are appreciative of the technical support of Olga Encalada, Antonio Serrano, Lorena Escudero, Cinthya Tebes-Cayo, Nia Oetiker, and of María Celia Chong D. for her support in the model designing. A preprint version of this manuscript is available on the bioRxiv server, https://www.biorxiv.org/content/10.1101/2022.06.08.495031v1 (Acosta Grinok et al., 2022).
Conflict of interest
The authors declare that the research was conducted in the absence of any commercial or financial relationships that could be construed as a potential conflict of interest.
Publisher’s note
All claims expressed in this article are solely those of the authors and do not necessarily represent those of their affiliated organizations, or those of the publisher, the editors and the reviewers. Any product that may be evaluated in this article, or claim that may be made by its manufacturer, is not guaranteed or endorsed by the publisher.
Supplementary material
The Supplementary material for this article can be found online at: https://www.frontiersin.org/articles/10.3389/fmicb.2022.1029886/full#supplementary-material
Footnotes
References
Achour-Rokbani, A., Cordi, A., Poupin, P., Bauda, P., and Billard, P. (2010). Characterization of the ars gene cluster from extremely arsenic-resistant microbacterium sp. strain A33. Appl. Environ. Microbiol. 76, 948–955. doi: 10.1128/AEM.01738-09
Acosta Grinok, M., Vázquez, S., Guiliani, N., Marín, S., and Demergasso, C. (2022). Looking for the mechanism of arsenate respiration in an arsenate-dependent growing culture of Fusibacter sp. strain 3D3, independent of ArrAB. bioRxiv. doi: 10.1101/2022.06.08.495031
Altschul, S. F., and Lipman, D. J. (1990). Protein database searches for multiple alignments. Proc. Natl. Acad. Sci. U. S. A. 87, 5509–5513. doi: 10.1073/pnas.87.14.5509
Altschul, S. F., Madden, T. L., Schaffer, A. A., Zhang, J. H., Zhang, Z., Miller, W., et al. (1997). Gapped BLAST and PSI-BLAST: a new generation of protein database search programs. Nucleic Acids Res. 25, 3389–3402. doi: 10.1093/nar/25.17.3389
Arner, E. S., Zhong, L., and Holmgren, A. (1999). Preparation and assay of mammalian thioredoxin and thioredoxin reductase. Methods Enzymol. 300, 226–239. doi: 10.1016/S0076-6879(99)00129-9
Ben Hania, W., Fraj, B., Postec, A., Fadhlaoui, K., Hamdi, M., Ollivier, B., et al. (2012). Fusibacter tunisiensis sp. nov., isolated from an anaerobic reactor used to treat olive-mill wastewater. Int. J. Syst. Evol. Microbiol. 62, 1365–1368. doi: 10.1099/ijs.0.034603-0
Benson, D. A., Karsch-Mizrachi, I., Lipman, D. J., Ostell, J., and Sayers, E. W. (2009). GenBank. Nucleic Acids Res. 37, D26–D31. doi: 10.1093/nar/gkn723
Bertsch, J. P. A., Buckel, W., and Müller, V. (2013). An electron-bifurcating caffeyl-CoA reductase. J. Biol. Chem. 288, 11304–11311. doi: 10.1074/jbc.M112.444919
Biegel, E., Schmidt, S., Gonzalez, J. M., and Mueller, V. (2011). Biochemistry, evolution and physiological function of the Rnf complex, a novel ion-motive electron transport complex in prokaryotes. Cell. Mol. Life Sci. 68, 613–634. doi: 10.1007/s00018-010-0555-8
Blum, J. S., Hernandez-Maldonado, J., Redford, K., Sing, C., Bennett, S. C., Saltikov, C. W., et al. (2018). Arsenate-dependent growth is independent of an ArrA mechanism of arsenate respiration in the termite hindgut isolate Citrobacter sp. strain TSA-1. Can. J. Microbiol. 64, 619–627. doi: 10.1139/cjm-2017-0523
Boileau, C., Auria, R., Davidson, S., Casalot, L., Christen, P., Liebgott, P. P., et al. (2016). Hydrogen production by the hyperthermophilic bacterium Thermotoga maritima part I: effects of sulfured nutriments, with thiosulfate as model, on hydrogen production and growth. Biotechnol. Biofuels 9:269. doi: 10.1186/s13068-016-0678-8
Brettin, T., Davis, J. J., Disz, T., Edwards, R. A., Gerdes, S., Olsen, G. J., et al. (2015). RASTtk: a modular and extensible implementation of the RAST algorithm for building custom annotation pipelines and annotating batches of genomes. Sci. Rep. 5:8365. doi: 10.1038/srep08365
Buckel, W., and Thauer, R. K. (2013). Energy conservation via electron bifurcating ferredoxin reduction and proton/Na+ translocating ferredoxin oxidation. BBA-Bioenergetics 1827, 94–113. doi: 10.1016/j.bbabio.2012.07.002
Buckel, W., and Thauer, R. K. (2018a). Flavin-based electron bifurcation, a new mechanism of biological energy coupling. Chem. Rev. 118, 3862–3886. doi: 10.1021/acs.chemrev.7b00707
Buckel, W., and Thauer, R. K. (2018b). Flavin-based electron bifurcation, ferredoxin, flavodoxin, and anaerobic respiration with protons (Ech) or NAD(+) (Rnf) as electron acceptors: a historical review. Front. Microbiol. 9:401. doi: 10.3389/fmicb.2018.00401
Cleiss-Arnold, J., Koechler, S., Proux, C., Fardeau, M. L., Dillies, M. A., Coppee, J. Y., et al. (2010). Temporal transcriptomic response during arsenic stress in Herminiimonas arsenicoxydans. BMC Genomics 11. doi: 10.1186/1471-2164-11-709
Cozen, A. E., Weirauch, M. T., Pollard, K. S., Bernick, D. L., Stuart, J. M., and Lowe, T. M. (2009). Transcriptional map of respiratory versatility in the hyperthermophilic crenarchaeon Pyrobaculum aerophilum. J. Bacteriol. 191, 782–794. doi: 10.1128/jb.00965-08
Cuebas, M., Villafane, A., McBride, M., Yee, N., and Bini, E. (2011). Arsenate reduction and expression of multiple chromosomal ars operons in Geobacillus kaustophilus A1. Microbiology 157, 2004–2011. doi: 10.1099/mic.0.048678-0
Davidsen, T., Beck, E., Ganapathy, A., Montgomery, R., Zafar, N., Yang, Q., et al. (2010). The comprehensive microbial resource. Nucleic Acids Res. 38, D340–D345. doi: 10.1093/nar/gkp912
Detman, A., Mielecki, D., Chojnacka, A., Salamon, A., Blaszczyk, M. K., and Sikora, A. (2019). Cell factories converting lactate and acetate to butyrate: clostridium butyricum and microbial communities from dark fermentation bioreactors. Microb. Cell Factories 18:36. doi: 10.1186/s12934-019-1085-1
Edgar, R. C. (2004). MUSCLE: multiple sequence alignment with high accuracy and high throughput. Nucleic Acids Res. 32, 1792–1797. doi: 10.1093/nar/gkh340
Escudero, L. V., Casamayor, E. O., Chong, G., Pedros-Alio, C., and Demergasso, C. (2013). Distribution of microbial arsenic reduction, oxidation and extrusion genes along a wide range of environmental arsenic concentrations. PLoS One 8:e78890. doi: 10.1371/journal.pone.0078890
Fadhlaoui, K., Ben Hania, W., Postec, A., Fauque, G., Hamdi, M., Ollivier, B., et al. (2015). Fusibacter fontis sp. nov., a sulfur-reducing, anaerobic bacterium isolated from a mesothermic Tunisian spring. Int. J. Syst. Evol. Microbiol. 65, 3501–3506. doi: 10.1099/ijsem.0.000445
Garcia Costas, A. M., Poudel, S., Miller, A. F., Schut, G. J., Ledbetter, R. N., Fixen, K. R., et al. (2017). Defining electron bifurcation in the electron-transferring flavoprotein family. J. Bacteriol. 199. doi: 10.1128/JB.00440-17
Green, M. R., and Sambrook, J. (2019). Screening bacterial colonies using X-gal and IPTG: alpha-complementation. Cold Spring Harb. Protoc. 2019:pdb.prot101329. doi: 10.1101/pdb.prot101329
Gutiérrez-Preciado, A., Saghaï, A., Moreira, D., Zivanovic, Y., Deschamps, P., and López-García, P. (2018). Functional shifts in microbial mats recapitulate early earth metabolic transitions. Nature Ecol. Evol. 2, 1700–1708. doi: 10.1038/s41559-018-0683-3
Hackmann, T. J., and Firkins, J. L. (2015). Electron transport phosphorylation in rumen butyrivibrios: unprecedented ATP yield for glucose fermentation to butyrate. Front. Microbiol. 6:622. doi: 10.3389/fmicb.2015.00622
Holmgren, A. (1979). Thioredoxin catalyzes the reduction of insulin disulfides by dithiothreitol and dihydrolipoamide. J. Biol. Chem. 254, 9627–9632. doi: 10.1016/S0021-9258(19)83562-7
Kim, D. H., Jiang, S., Lee, J. H., Cho, Y. J., Chun, J., Choi, S. H., et al. (2011). Draft genome sequence of Shewanella sp. strain HN-41, which produces arsenic-sulfide nanotubes. J. Bacteriol. 193, 5039–5040. doi: 10.1128/jb.05578-11
Kurth, D., Amadio, A., Ordoñez, O., Albarracín, V., Gärtner, W., and Farías, M. (2017). Arsenic metabolism in high altitude modern stromatolites revealed by metagenomic analysis. Sci. Rep. 7:1024. doi: 10.1038/s41598-017-00896-0
Lara, J., Escudero Gonzalez, L., Ferrero, M., Chong Diaz, G., Pedros-Alio, C., and Demergasso, C. (2012). Enrichment of arsenic transforming and resistant heterotrophic bacteria from sediments of two salt lakes in northern Chile. Extremophiles 16, 523–538. doi: 10.1007/s00792-012-0452-1
Li, F., Hinderberger, J., Seedorf, H., Zhang, J., Buckel, W., and Thauer, R. K. (2008). Coupled ferredoxin and crotonyl coenzyme a (CoA) reduction with NADH catalyzed by the butyryl-CoA dehydrogenase/Etf complex from clostridium kluyveri. J. Bacteriol. 190, 843–850. doi: 10.1128/JB.01417-07
Li, X., and Krumholz, L. R. (2007). Regulation of arsenate resistance in Desulfovibrio desulfuricans G20 by an arsRBCC operon and an arsC gene. J. Bacteriol. 189, 3705–3711. doi: 10.1128/JB.01913-06
Liang, J. Y., Huang, H. Y., and Wang, S. N. (2019). Distribution, evolution, catalytic mechanism, and physiological functions of the flavin-based electron-bifurcating NADH-dependent reduced ferredoxin: NADP(+) oxidoreductase. Front. Microbiol. 10:373. doi: 10.3389/fmicb.2019.00373
Lubner, C. E., Jennings, D. P., Mulder, D. W., Schut, G. J., Zadvornyy, O. A., Hoben, J. P., et al. (2017). Mechanistic insights into energy conservation by flavin-based electron bifurcation. Nat. Chem. Biol. 13:655. doi: 10.1038/nchembio.2348
Macy, J. M., Santini, J. M., Pauling, B. V., O'Neill, A. H., and Sly, L. I. (2000). Two new arsenate/sulfate-reducing bacteria: mechanisms of arsenate reduction. Arch. Microbiol. 173, 49–57. doi: 10.1007/s002030050007
Malasarn, D., Saltikov, C. W., Campbell, K. M., Santini, J. M., Hering, J. G., and Newman, D. K. (2004). arrA is a reliable marker for as(V) respiration. Science 306:455. doi: 10.1126/science.1102374
Messens, J., and Silver, S. (2006). Arsenate reduction: thiol cascade chemistry with convergent evolution. J. Mol. Biol. 362, 1–17. doi: 10.1016/j.jmb.2006.07.002
Mukhopadhyay, R., and Rosen, B. P. (2002). Arsenate reductases in prokaryotes and eukaryotes. Environ. Health Perspect. 110, 745–748. doi: 10.1289/ehp.02110s5745
Müller, V., Imkamp, F., Biegel, E., Schmidt, S., and Dilling, S. (2008). Discovery of a ferredoxin:NAD+-oxidoreductase (Rnf) in Acetobacterium woodii: a novel potential coupling site in acetogens. Ann. N. Y. Acad. Sci. 1125, 137–146. doi: 10.1196/annals.1419.011
Murphy, J. N., and Saltikov, C. W. (2007). The cymA gene, encoding a tetraheme c-type cytochrome, is required for arsenate respiration in Shewanella species. J. Bacteriol. 189, 2283–2290. doi: 10.1128/JB.01698-06
Norambuena, J., Flores, R., Cardenas, J. P., Quatrini, R., Chavez, R., and Levican, G. (2012). Thiol/disulfide system plays a crucial role in redox protection in the acidophilic iron-oxidizing bacterium Leptospirillum ferriphilum. PLoS One 7:e44576. doi: 10.1371/journal.pone.0044576
Ordonez, E., Van Belle, K., Roos, G., De Galan, S., Letek, M., Gil, J. A., et al. (2009). Arsenate reductase, mycothiol, and mycoredoxin concert thiol/disulfide exchange. J. Biol. Chem. 284, 15107–15116. doi: 10.1074/jbc.M900877200
Pandey, S., Rai, R., and Rai, L. C. (2012). Proteomics combines morphological, physiological and biochemical attributes to unravel the survival strategy of anabaena sp. PCC7120 under arsenic stress. J. Proteome 75, 921–937. doi: 10.1016/j.jprot.2011.10.011
Pandey, S., Shrivastava, A. K., Singh, V. K., Rai, R., Singh, P. K., Rai, S., et al. (2013). A new arsenate reductase involved in arsenic detoxification in anabaena sp. PCC7120. Funct. Integr. Genomics 13, 43–55. doi: 10.1007/s10142-012-0296-x
Peters, J. W., Beratan, D. N., Bothner, B., Dyer, R. B., Harwood, C. S., Heiden, Z. M., et al. (2018). A new era for electron bifurcation. Curr. Opin. Chem. Biol. 47, 32–38. doi: 10.1016/j.cbpa.2018.07.026
Poudel, S., Dunham, E. C., Lindsay, M. R., Amenabar, M. J., Fones, E. M., Colman, D. R., et al. (2018). Origin and evolution of flavin-based electron bifurcating enzymes. Front. Microbiol. 9:1762. doi: 10.3389/fmicb.2018.01762
Punta, M., Coggill, P. C., Eberhardt, R. Y., Mistry, J., Tate, J., Boursnell, C., et al. (2012). The Pfam protein families database. Nucleic Acids Res. 40, D290–D301. doi: 10.1093/nar/gkr1065
Qiu, D., Zeng, X., Zeng, L., Li, G., and Shao, Z. (2021). Fusibacter ferrireducens sp. nov., an anaerobic, Fe(III)- and Sulphur-reducing bacterium isolated from mangrove sediment. Int. J. Syst. Evol. Microbiol. 71. doi: 10.1099/ijsem.0.004952
Ravot, G., Magot, M., Fardeau, M. L., Patel, B. K., Thomas, P., Garcia, J. L., et al. (1999). Fusibacter paucivorans gen. Nov., sp. nov., an anaerobic, thiosulfate-reducing bacterium from an oil-producing well. Int. J. Syst. Bacteriol. 49, 1141–1147.
Rosen, B. P., and Liu, Z. (2009). Transport pathways for arsenic and selenium: a minireview. Environ. Int. 35, 512–515. doi: 10.1016/j.envint.2008.07.023
Schuchmann, K., and Müller, V. (2016). Energetics and application of heterotrophy in acetogenic bacteria. Appl. Environ. Microbiol. 82, 4056–4069. doi: 10.1128/aem.00882-16
Serrano, A. E., Escudero, L. V., Tebes-Cayo, C., Acosta, M., Encalada, O., Fernandez-Moroso, S., et al. (2017). First draft genome sequence of a strain from the genus Fusibacter isolated from Salar de Ascotan in northern Chile. Stand. Genomic Sci. 12:9. doi: 10.1186/s40793-017-0252-4
Sigrist, C. J., Cerutti, L., Hulo, N., Gattiker, A., Falquet, L., Pagni, M., et al. (2002). PROSITE: a documented database using patterns and profiles as motif descriptors. Brief. Bioinform. 3, 265–274. doi: 10.1093/bib/3.3.265
Slyemi, D., and Bonnefoy, V. (2012). How prokaryotes deal with arsenic. Environ. Microbiol. Rep. 4, 571–586. doi: 10.1111/j.1758-2229.2011.00300.x
Smii, L., Ben Hania, W., Cayol, J.-L., Joseph, M., Hamdi, M., Ollivier, B., et al. (2015). Fusibacter bizertensis sp. nov., isolated from a corroded kerosene storage tank. Int. J. Syst. Evol. Microbiol. 65, 117–121. doi: 10.1099/ijs.0.066183-0
Tatusov, R. L., Fedorova, N. D., Jackson, J. D., Jacobs, A. R., Kiryutin, B., Koonin, E. V., et al. (2003). The COG database: an updated version includes eukaryotes. BMC Bioinform. 4:41. doi: 10.1186/1471-2105-4-41
Tremblay, P. L., Zhang, T., Dar, S. A., Leang, C., and Lovley, D. R. (2012). The Rnf complex of clostridium ljungdahlii is a proton-translocating ferredoxin:NAD+ oxidoreductase essential for autotrophic growth. MBio 4, e00406–e00412. doi: 10.1128/mBio.00406-12
van Lis, R., Nitschke, W., Duval, S., and Schoepp-Cothenet, B. (2013). Arsenics as bioenergetic substrates. Biochim. Biophys. Acta 1827, 176–188. doi: 10.1016/j.bbabio.2012.08.007
Vera, M., Pagliai, F., Guiliani, N., and Jerez, C. A. (2008). The chemolithoautotroph Acidithiobacillus ferrooxidans can survive under phosphate-limiting conditions by expressing a C-P lyase operon that allows it to grow on phosphonates. Appl. Environ. Microbiol. 74, 1829–1835. doi: 10.1128/AEM.02101-07
Villadangos, A. F., Van Belle, K., Wahni, K., Tamu Dufe, V., Freitas, S., Nur, H., et al. (2011). Corynebacterium glutamicum survives arsenic stress with arsenate reductases coupled to two distinct redox mechanisms. Mol. Microbiol. 82, 998–1014. doi: 10.1111/j.1365-2958.2011.07882.x
Wang, L. Y., Bradstock, P., Li, C., McInerney, M. J., and Krumholz, L. R. (2016). The role of Rnf in ion gradient formation in Desulfovibrio alaskensis. Peerj 4. doi: 10.7717/peerj.1919
Weghoff, M. C., Bertsch, J., and Müller, V. (2015). A novel mode of lactate metabolism in strictly anaerobic bacteria. Environ. Microbiol. 17, 670–677. doi: 10.1111/1462-2920.12493
Westphal, L., Wiechmann, A., Baker, J., Minton, N. P., and Müller, V. (2018). The Rnf complex is an energy-coupled transhydrogenase essential to reversibly link cellular NADH and ferredoxin pools in the acetogen Acetobacterium woodii. J. Bacteriol. 200. doi: 10.1128/JB.00357-18
Keywords: Fusibacter, arsenic respiration, electron bifurcation, Rnf complex, ferredoxin, thioredoxin, etf, Northern Chile
Citation: Acosta-Grinok M, Vázquez S, Guiliani N, Marín S and Demergasso C (2022) Looking for the mechanism of arsenate respiration of Fusibacter sp. strain 3D3, independent of ArrAB. Front. Microbiol. 13:1029886. doi: 10.3389/fmicb.2022.1029886
Edited by:
Hans Karl Carlson, Berkeley Lab (DOE), United StatesReviewed by:
Victor Manuel Reyes-Umaña, University of California, Berkeley, United StatesWolfgang Buckel, University of Marburg, Germany
Copyright © 2022 Grinok, Vázquez, Guiliani, Marín and Demergasso. This is an open-access article distributed under the terms of the Creative Commons Attribution License (CC BY). The use, distribution or reproduction in other forums is permitted, provided the original author(s) and the copyright owner(s) are credited and that the original publication in this journal is cited, in accordance with accepted academic practice. No use, distribution or reproduction is permitted which does not comply with these terms.
*Correspondence: Cecilia Demergasso, Y2RlbWVyZ2FAdWNuLmNs