- 1Department of Molecular Evolution, Centro de Astrobiología (CAB), CSIC-INTA, Madrid, Spain
- 2Centro de Investigación en Recursos Naturales y Sustentabilidad (CIRENYS), Universidad Bernardo O’Higgins, Santiago, Chile
The microorganisms that thrive in Antarctica, one of the coldest environments on the planet, have developed diverse adaptation mechanisms to survive in these extreme conditions. Through functional metagenomics, in this work, 29 new genes related to cold tolerance have been isolated and characterized from metagenomic libraries of microorganisms from the rhizosphere of two Antarctic plants. Both libraries were hosted in two cold-sensitive strains of Escherichia coli: DH10B ΔcsdA and DH10B ΔcsdA Δrnr. The csdA gene encodes a DEAD-box RNA helicase and rnr gene encodes an exoribonuclease, both essential for cold-adaptation. Cold-tolerance tests have been carried out in solid and liquid media at 15°C. Among the cold-tolerance genes identified, 12 encode hypothetical and unknown proteins, and 17 encode a wide variety of different proteins previously related to other well-characterized ones involved in metabolism reactions, transport and membrane processes, or genetic information processes. Most of them have been connected to cold-tolerance mechanisms. Interestingly, 13 genes had no homologs in E. coli, thus potentially providing entirely new adaptation strategies for this bacterium. Moreover, ten genes also conferred resistance to UV-B radiation, another extreme condition in Antarctica.
Introduction
Antarctica is considered, on average, to be the coldest, driest, and windiest continent on Earth and it has the highest average altitude. In fact, its temperatures are really extreme, reaching values between −20 and −60°C in winter, and its average temperature is always below 0°C. Other extreme conditions that limit the development of life in Antarctica are strong winds, periodic freeze–thaw cycles, high sublimation and evaporation rates, long periods of darkness, or high exposures to ultraviolet radiation (Duarte et al., 2018).
Despite these drastic conditions, many organisms have been able to develop different molecular and morphological strategies that allow them to thrive optimally in these cold environments. They are collectively named psychrophiles or cold-adapted organisms (Dhaulaniya et al., 2019). Microorganisms such as bacteria, archaea, algae, fungi, or protists prevail in these cold habitats and have a key role in the transport of energy, nutrient recycling, and mineralization processes, which are vital for the functioning of these terrestrial and aquatic ecosystems (Ruisi et al., 2007). However, drastic low temperatures affect their cell envelope properties and functions by reducing membrane permeability and restricting its flexibility and diffusion rates. Moreover, embedded proteins also decrease their mobility and function, and turgor pressure is normally increased by ice formation or freeze–thaw cycles (Collins and Margesin, 2019). To counteract these deleterious effects, cold-adapted microorganisms usually introduce some changes in their membrane compositions favoring an increase in their fluidity and, therefore, their functionality under low temperatures (Kahlke and Thorvaldsen, 2012). In addition, upregulation of membrane transport proteins has been detected in some psychrophiles, facilitating increased rates of diffusion and transport rates (De Maayer et al., 2014).
Enzymes and proteins are also negatively affected at low temperatures (Dhaulaniya et al., 2019) and their reaction rates might be considerably reduced under these conditions. However, psychrophiles have adapted their enzymes to extreme cold conditions by decreasing their structural (Sindhu et al., 2017) and thermal stability (Maiangwa et al., 2015), favoring its flexibility, specificity, and kinetics by increasing enzyme concentration, and evolving new alternative enzymes whose reaction rate is only controlled by diffusion and is completely independent of temperature (Georlette et al., 2004). In addition, protein and RNA/DNA chaperones play an important role in cold-adaptation counteracting protein misfolding and aggregation as well as stabilizing RNA/DNA secondary structures. In this way, they are responsible for maintaining efficient transcription, translation, and DNA replication rates (Lim et al., 2000).
Cold adaptation is also related to various metabolic adjustments that favor reduced ROS production and the conservation of energy for long-term survival. These metabolic adjustments include the downregulation of primary metabolism pathways, the activation of some alternative secondary pathways, and the metabolism and accumulation of reserve compounds, such as polyhydroxyalkanoate (PHA), which can be used as a dynamic reserve of carbon, nitrogen, and energy, but may also play a key role in cryoprotection, oxidative stress resistance, cell motility, or maintenance of cellular redox balance (Methé et al., 2005; Tribelli et al., 2015; Tribelli and Lopez, 2018).
Furthermore, subzero temperatures normally cause ice formation in the extracellular space and, therefore, osmotic stress, dehydration, cryoinjury, or even cell rupture and death (Fonseca et al., 2016). Cold-adapted microorganisms respond to these deleterious effects by producing different types of cryoprotectants such as compatible solutes (Goordial et al., 2016), ice-binding proteins (antifreeze and ice-nucleating proteins) (Lorv et al., 2014; Voets, 2017), biosurfactants (Kitamoto et al., 2001; Perfumo et al., 2018), or extracellular polymeric substances (Caruso et al., 2018).
All of the cellular cold-adaptation strategies described above have already been well-characterized. Nonetheless, further research effort is needed. The research on psychrophiles is really interesting not only to increase our knowledge about cold-tolerant molecular mechanisms but also to describe new cold-active enzymes with new properties that could confer some advantages for many biotechnological processes (Duarte et al., 2018). Some enzymes from these microorganisms have been used in the food industry to improve milk fermentation and meat and dough quality, in agriculture as biofertilizers, or in textile industry for dye removal or bleaching processes (Gurung et al., 2013; Nigam, 2013). On the other hand, psychrophilic microorganisms have strict requirements for their growth, so reproducing these conditions in the laboratory is quite difficult and many strains are not culturable under standard laboratory conditions. To overcome this problem and with the aim of searching for novel cold-tolerant genes and increasing our knowledge about the molecular mechanisms of cold adaptation, we used a functional metagenomic approach in this work. Through this methodology, we were able to access the entire genetic potential of microorganisms in the rhizosphere of two Antarctic plants: Deschampsia antarctica and Colobanthus quitensis. This procedure allows a culture-independent analysis of functional genes related to a cold response of microbial genes isolated from the Antarctic samples. As a result, 29 novel cold-tolerant genes were isolated and characterized in this study. Seventeen of them were similar to previously described genes and few of them had already been related to cold-acclimation processes, whereas others had not previously been linked to cold tolerance. Furthermore, 12 hypothetical or unknown proteins were also described in this study. This is a valuable finding since these genes could be related to novel cold-tolerance mechanisms not described until now. All these results offered us a broader view of the cold-adaptation mechanisms that microorganisms have developed under these extreme environments.
Materials and methods
Bacterial strains and growth conditions
Three strains of Escherichia coli have been used in this study: E. coli DH10B (Invitrogen) and two cold-sensitive mutants that we specifically constructed for this screening: E. coli DH10B ΔcsdA and E. coli DH10B ΔcsdA Δrnr. E. coli DH10B was used as a host to construct and to maintain the metagenomic libraries, while DH10B ΔcsdA and DH10B ΔcsdA Δrnr strains were used as hosts for screening for cold-tolerance genes. These three strains were routinely grown in Luria-Bertani (LB) medium (Conda) at 37°C. The growth medium for transformed E. coli strains was supplemented with 50 mg/ml ampicillin (Ap) to maintain the pBluescript SKII (+) plasmid. When required, the working concentration of 5-bromo-4-chloro-3-indolyl-β-galactopyranoside (X-Gal) was 40 μg/ml and of isopropyl β-D-1-thiogalactopyranoside (IPTG) was 100 μM.
Construction of Escherichia coli cold-sensitive mutant strains
Two cold-sensitive strains (E. coli DH10B ΔcsdA and E. coli DH10B ΔcsdA Δrnr) were constructed from E. coli DH10B cells (Invitrogen). The Counter-Selection BAC Modification Kit (Gene Bridges) was used to obtain both cold-sensitive mutants utilizing the Red/ET recombination technique (Gene Bridges) that allows the homologous recombination in vivo and, therefore, the specific exchange of our gene of interest (csdA or rnr) by an antibiotic resistance cassette (kanamycin was selected to obtain the DH10B ΔcsdA strain and chloramphenicol for DH10B ΔcsdA Δrnr). Specific DNA cassettes were designed and synthetized including the corresponding antibiotic resistance gene, restriction enzymes sites (BamHI and XbaI), and flanking fragments specific for the UTRs regions of csdA and rnr genes (Integrated DNA Technologies, IDT). E. coli DH10B cells were first transformed with the pRedET plasmid (Gene Bridges), which carries the λ phage γβα operon under the control of the arabinose-inducible pBAD promoter and that confers tetracycline resistance. Then, we introduced our insert (previously amplified by PCR (primers are described in Supplementary Table S1) and linearized by BamHI/XbaI digestion) into these cells and homologous recombination was induced by adding 10% arabinose to the medium and incubating for 1 h at 37°C. Selection of positive clones was performed by adding kanamycin (15 μg/ml) or chloramphenicol (20 μg/ml) to the LB-agar media. Correct insertion of the resistance cassette in the bacterial chromosome and the elimination of our gene of interest were verified by PCR (Supplementary Table S1).
Construction of metagenomic libraries
Two metagenomic libraries have been constructed using DNA isolated from rhizosphere samples of two Antarctic plants: Colobanthus quitensis (library C) and Deschampsia antarctica (library D). Samples were recovered during the austral summer (February 2011) at Admiralty Bay in King George Island, located in the South Shetland archipelago in the north of Antarctic Peninsula. Deschampsia rhizosphere samples were obtained close to the Brazilian Antarctic Station (Estação Antártica Comandante Ferraz; GPS coordinates: 62°03′40″S–58°23′30″W), while the Colobanthus samples were collected in the Antarctic Specially Protected Area (ASPA N° 151; GPS coordinates: 62°08′00″S–58°28′10″W) adjacent to the Arctowski Polish Station.
Roots with soil particles tightly adhered to them were collected at a depth of 5 cm and immediately kept in 50 ml tubes containing RNA Later (Sigma) solution. All samples were collected in triplicate and stored frozen at –80°C. In order to extract high-quality and intact metagenomic DNA from the rhizosphere samples, they were thawed and aseptically processed with the BIO101 FastDNA Spin kit for soil (Qbiogene), obtaining 20 μg of DNA per gram of sample. Libraries were constructed using the high-copy number pBluescript II SK (+) vector and the E. coli DH10B strain as a host, as previously described (Mirete et al., 2007; González-Pastor and Mirete, 2010). Briefly, the metagenomic DNA was partially digested using Sau3AI and the fragments from 1 to 8 Kbp were isolated by a 0.8% low-melting-point agarose gel electrophoresis with the QIAquick Gel Extraction kit (QIAGEN). The metagenomic DNA fragments were then ligated into the BamHI digested and dephosphorylated pBluescript II SK (+) vector. DNA (100–125 ng) purified from the gel was mixed with the vector in a 1:1 molar ratio. Ligation mixtures were incubated overnight at 16°C using T4 DNA ligase (Roche) and used to transform E. coli DH10B cells (Invitrogen) by electroporation with a MicroPulser (Bio-Rad) according to the manufacturer’s instructions (Mirete et al., 2007; González-Pastor and Mirete, 2010). To estimate the average insert size of the library, the plasmids from ten random recombinant clones were isolated on LB-Ap plates and digested using either EcoRI or XbaI and XhoI restriction enzymes (Roche). To amplify these libraries, cells were grown at 37°C for 24 h on LB-agar plates containing ampicillin (approximately 1.3 × 104 cells per plate). Then, cells from each plate were recovered, mixed with LB and 10% glycerol (w/v), and stored at −80°C.
Screening for cold-tolerant clones in the metagenomic libraries
Recombinant plasmids from the metagenomic libraries constructed in E. coli DH10B cells were extracted using the QIAprep Spin Miniprep kit (Qiagen) and 100 ng of them were used to transform electrocompetent cells of E. coli DH10B ΔcsdA and DH10B ΔcsdA Δrnr, which had been previously prepared according to Dower et al., 1988. To amplify the libraries, after electroporation, the transformed cells were grown in liquid LB-Ap medium at 37°C to increase the number of viable cells around 104 times. To select for cold-tolerant clones, a total of 3 × 106 recombinant clones from each library were spread on LB agar supplemented with Ap, X-Gal, and IPTG (around 105 cells per plate) and incubated at 15°C during 3–5 days until the appearance of colonies. To ensure that the resistance phenotype was not due to the presence of spontaneous chromosomal mutations, resistant colonies were pooled, and their plasmid DNA were isolated and used to transform DH10B ΔcsdA or DH10B ΔcsdA Δrnr cells. The new retransformed clones were grown again at 15°C on LB agar (Ap, X-Gal, IPTG) medium. Cold-tolerant clones were selected and their isolated recombinant plasmids were digested with XhoI and XbaI (Roche) to identify those which are unique in their restriction patterns.
In silico analysis of cold-tolerant clones
The DNA inserts cloned into pBluescript II SK (+) plasmids isolated from cold-tolerant clones were sequenced on both strands with universal primers M13F/M13R and with other primers specifically designed for primer walking by using the ABI PRISM dye terminator cycle-sequencing ready-reaction kit (PerkinElmer, Waltham, MA, United States) and an ABI PRISM 377 sequencer (PerkinElmer). Sequences were assembled and analyzed with the ApE program. Prediction of potential open reading frames (ORFs) was conducted using the online source ORF Finder, which is available at the NCBI webpage.1 The bacterial code was selected by allowing ATG, CTG, GTG, and TTG as alternative start codons for translation to protein sequences. All the predicted ORFs longer than 90 bp were translated and used as queries in BlastP. Their putative function was annotated based on their similarities to protein family domains by using NCBI’s Conserved Domain Database and Pfam (Protein Families).2 Those translated ORFs with an E-value higher than 0.001 in the BlastP searches were considered unknown proteins. For DNA-binding domain prediction, two different programs were used with default settings: DPP-PseAAC (Rahman et al., 2018)3 and DNABIND (Liu and Hu, 2013).4
Cold-tolerance tests
All the positive clones isolated from the cold screening were tested in liquid and solid media at 15°C. Two controls were introduced in each experiment: a positive control (DH10B strain transformed with the empty pBluescript II SK (+) vector) and a negative control (DH10B ΔcsdA or DH10B ΔcsdA Δrnr strains transformed with the empty pBluescript II SK (+) vector). Solid medium tests were carried out by drop assay using cell cultures grown overnight at 37°C in LB-Ap liquid medium and whose OD600nm values were adjusted to 1. The following serial dilutions were made: 1, 1/5, 1/10, 1/50, 1/100, and 1/1,000 and they were distributed as drops of 10 μl on LB-Ap solid medium. Plates were incubated at 15°C for 10 days. The results were confirmed by repeating these tests at least three times using independent cultures. We also checked that all the strains had initially been adjusted to a similar cell density and that all of them have a similar viability by carrying out the same drop assay at 37°C (without a cold stress). In this control assay, we made the following serial dilutions: 1, 10−1, 10−2, 10−3, 10−4, and 10−5, to observe possible cell viability differences between cold-tolerant clones and control strains.
Liquid medium tests were used to confirm the cold-tolerance results obtained in solid medium tests. They were performed in 96-well plates with cell cultures whose OD600nm values had previously been adjusted to 0.03. Cells were cultured in LB-Ap liquid medium at 15°C and 80 rpm for 7 days. Growth curves were constructed by measuring OD600nm values four times per day using the microplate reader SPECTROstar Nano (BMG Labtech). Non-inoculated wells served as blanks and their values were subtracted from those obtained in inoculated wells. Growth curves were built using the DMFit online tool included in the Combase website,5 according to Baranyi and Roberts model (Baranyi and Roberts, 1994). After obtaining the growth curves, we calculated two kinetic parameters for all of them: the specific growth rate (μ, h−1) and the generation time (Tg, h). The μ was defined as the slope of the exponential phase of the growth curve (linear phase) and the Tg was calculated using the following formula: Tg = (Ln 2)/μ. Liquid medium tests were repeated at least three times using independent cultures to confirm the results and six replicates of each strain were introduced in each plate. Moreover, growth curves were also built at 30°C to confirm that all the strains were able to grow at the same rate independently of the metagenomic insert that they contain. These control curves were obtained by measuring OD600nm values every hour for 30 or 40 h in the TECAN Infinite M Nano microplate reader.
Subcloning of independent genes to identify those that confer cold tolerance
To determine which genes could be directly involved in cold resistance in those recombinant plasmids containing more than one gene, all of them were cloned individually in the pBluescript SKII (+) vector. Therefore, fragments containing these genes and a region of ∼ 200 bp located upstream of the start codon, which probably incorporates native expression sequences (promoters and ribosome binding sites), were amplified by PCR using M13 primers and specific primers (Supplementary Table S1). The following reaction mixture was used in each PCR assay: 100 ng of plasmid DNA, 500 μM of each of the four dNTPs, 2.5 U of Pfu Turbo DNA Polymerase (Agilent), and 200 nM of each forward and reverse primers up to a total volume of 50 μl. The PCR amplification program used was as follows: 1 cycle of 5 min at 95°C, 30 cycles of 45 s at 95°C, 30 s at 52°C and 10 min at 72°C, and finally, 1 cycle of 10 min at 72°C. PCR amplification products were excised from agarose gels and purified using the QIAquick Gel Extraction kit (Qiagen). Purified PCR products were digested with the appropriate restriction enzymes (XbaI/XhoI, XbaI/KpnI, or XbaI/SalI, Roche) and ligated into pBluescript II SK (+; using a 1:6 ratio) previously digested with the same restriction enzymes. DH10B ΔcsdA or DH10B ΔcsdA Δrnr cells were transformed with these recombinant plasmids and their response was compared to the original clones carrying complete environmental DNA fragments by cold tests in solid and liquid media. All genes were subcloned in the same orientation as the original clone.
Overexpression of some Escherichia coli homologs in the cold-sensitive mutant strains
The E. coli homologs for some of the cold-tolerant genes isolated in this work were overexpressed in the E. coli DH10B ΔcsdA strain. These genes were selected from the genome of the E. coli DH10B strain by Blast (NCBI) and only those that shared a considerable similarity with the cold-tolerance genes (%ID > 25% and E-values < 0.001) were overexpressed. These E. coli genes were amplified by PCR using the genomic DNA of the DH10B strain as a template (the primers are described in Supplementary Table S1) and similarly subcloned into pBluescript II SK (+) vector as described in the previous section. E. coli DNA was isolated using the Wizard Genomic DNA Purification Kit as recommended by the manufacturer (Promega). E. coli DH10B ΔcsdA cells were transformed with these recombinant plasmids and the resulting strains overexpressing cold-tolerant homologs were tested for cold tolerance at 15°C in solid and liquid media as described above.
Ultraviolet-radiation resistance test by drop assay
In order to investigate whether the cold-tolerant clones may be resistant to other types of stress conditions, they were exposed to UV-B radiation. First, overnight cultures of these clones in LB-Ap liquid medium were adjusted to an OD600 nm of 1 and serial dilutions were made (1/2, 1/4, 1/8, 1/10, and 1/100). Then, a 10 μl drop of each dilution was inoculated on LB-Ap solid medium. As negative controls, the cold-sensitive strains DH10B ΔcsdA and DH10B ΔcsdA Δrnr, carrying the empty pBluescript II SK (+) vector, were used. Cells were irradiated with UV-B radiation at a dose of 4 mJ/cm2 and incubated overnight at 37°C. Then, their UV-resistance was evaluated by comparing the growth of cold-tolerant clones with negative controls. A control assay was performed with the same strains but they were not irradiated to verify their similar viability regardless of the applied treatment. The assay was repeated under the same conditions for the clones most resistant to UV-B radiation and their respective subclones were also tested to identify which genes were responsible for the UV-resistance observed in the complete clones.
Results
Screening for genes involved in cold tolerance from the rhizosphere of Antarctic plants
In order to search for genes that could confer cold tolerance to E. coli from microorganisms of the rhizosphere of Antarctic plants, two metagenomic libraries were constructed using metagenomic DNA from rhizosphere samples of the Colobanthus quitensis (library C) and Deschampsia antarctica (library D) plants. Approximately 1,100,000 recombinant clones were obtained from each library. The average size of the DNA fragments cloned in the pBluescript II SK (+) vector used for the libraries was 4.5 kb. Each library was amplified as described in Experimental Procedures. The DH10B strain used as a host for these libraries can grow at low temperatures, and was therefore not suitable for cold screening of metagenomic libraries. To avoid this problem, two cold-sensitive strains were constructed, DH10B ΔcsdA and DH10B ΔcsdA Δrnr, and the metagenomic libraries (C and D) were transferred to these strains. The csdA gene encodes a DEAD-box RNA helicase that is essential for the cold-acclimation process (Phadtare, 2011) and the rnr gene encodes an exoribonuclease that is also induced at low temperatures. A single mutant for the rnr gene is not cold sensitive but the double mutant ΔcsdA Δrnr grows more slowly at 30°C and cannot grow at all at moderate low temperatures (20°C) (Awano et al., 2007; Phadtare, 2011). The growth of these two mutant strains was tested at 15°C in solid and liquid medium, and it was observed that they started to grow later (higher lag phases) and slower (longer generation times) than the parental strain DH10B (Figures 1, 2; Supplementary Table S2). Therefore, to detect genes conferring cold tolerance, the metagenomic libraries in DH10B ΔcsdA and DH10B ΔcsdA Δrnr strains were grown on solid LB-Ap-medium at 15°C for a variable period of time between 3 and 5 days, conditions in which the same strains harboring empty pBluescript II SK (+) plasmids did not grow.
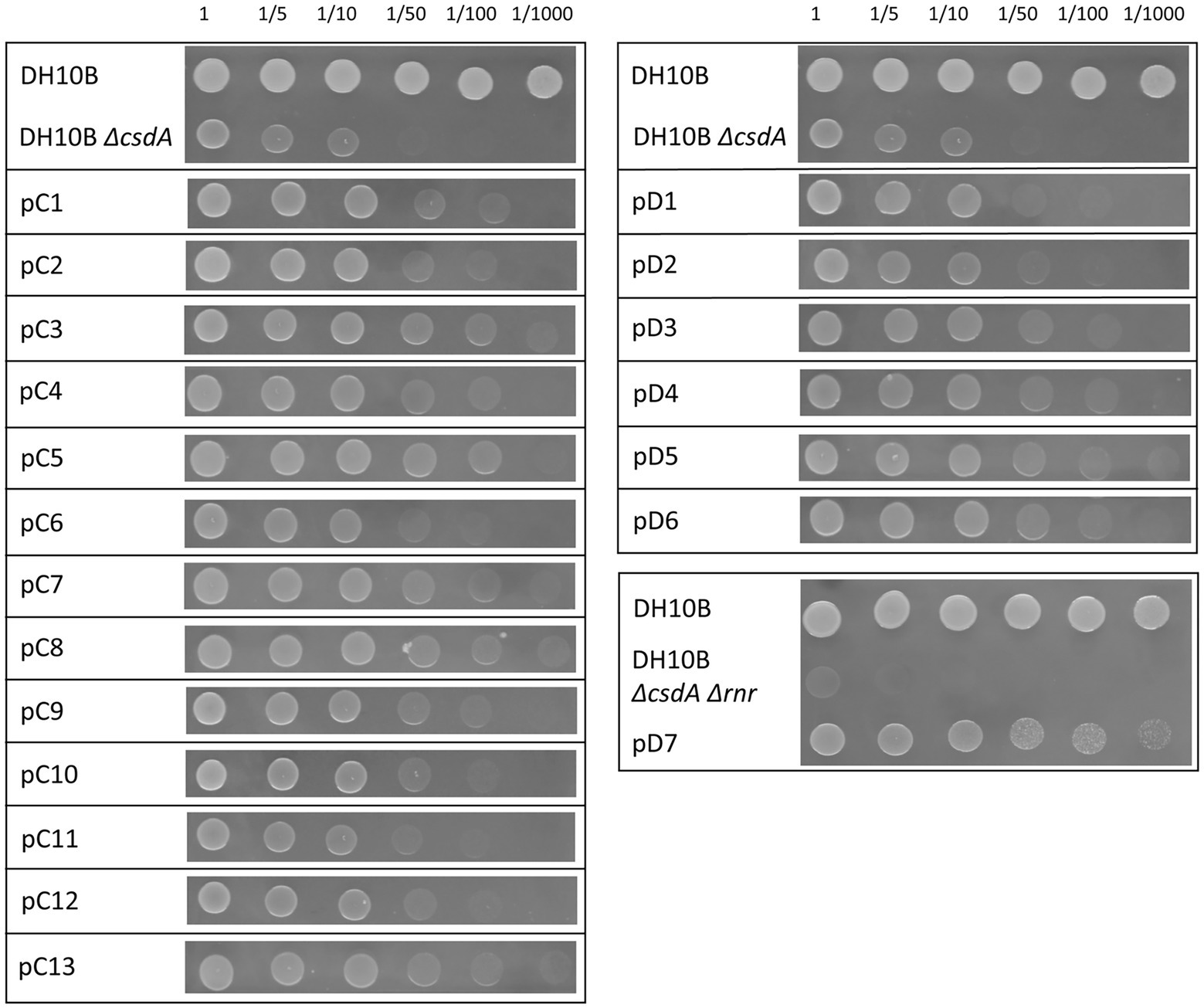
Figure 1. Drop assay of the 20 cold-resistant clones isolated from Antarctic metagenomic libraries: library C constructed from Colobanthus quitensis rhizosphere samples and library D from Deschampsia antarctica rhizosphere samples. The DH10B strain carrying an empty pBluescript vector was used as a positive control and both cold-sensitive strains (DH10B ΔcsdA and DH10B ΔcsdA Δrnr) also carrying empty pBluescript vectors were used as negative controls. The OD600 nm of overnight cultures was adjusted to values of 1.0 and serial dilutions were performed. 10 μl drops were inoculated on LB-Ap50 plates and grown at 15°C for 10 days. Each experiment was repeated at least three times using independent cultures.
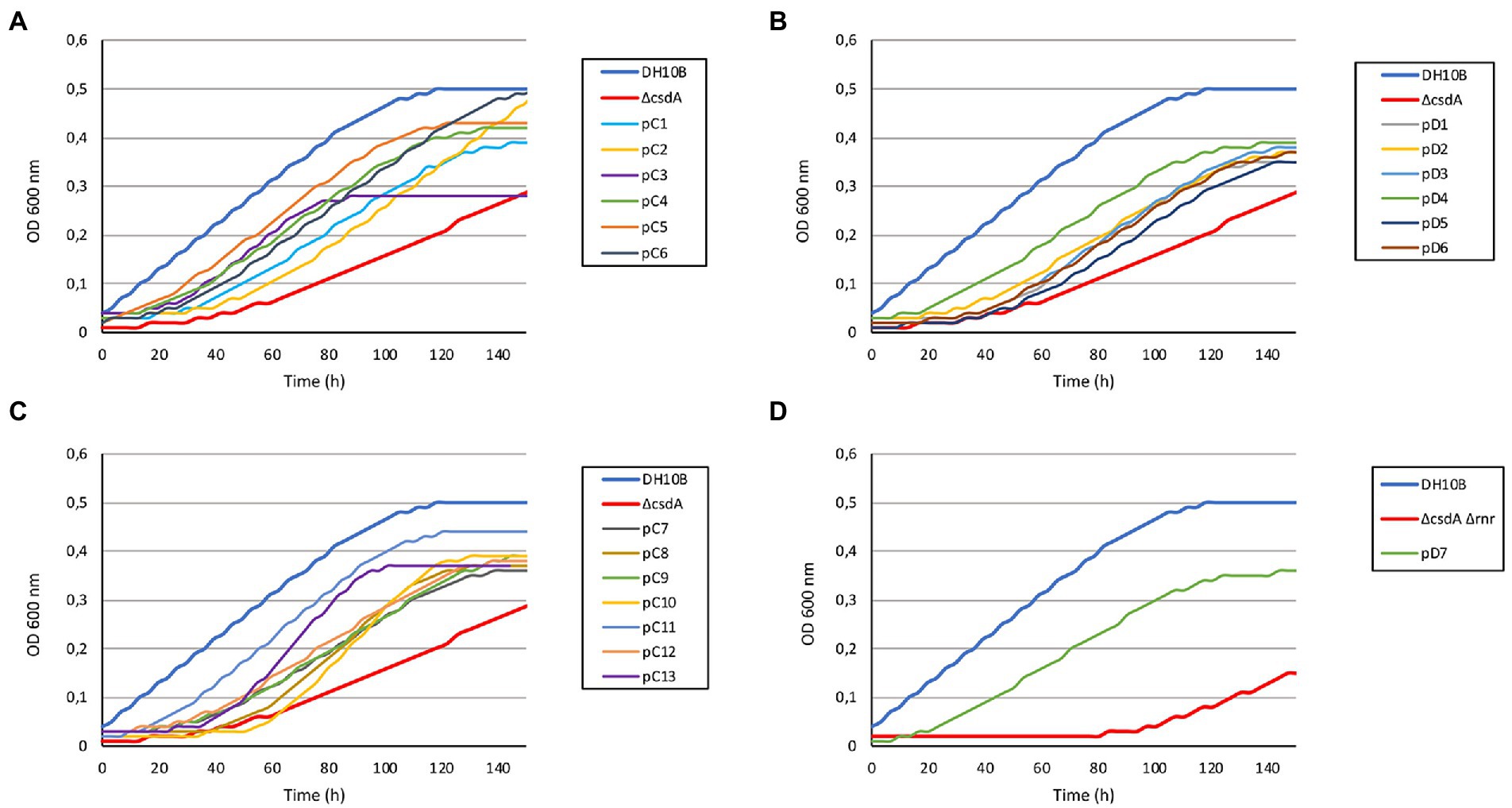
Figure 2. Growth curves of the 20 cold-resistant clones isolated from Antarctic metagenomic libraries. The DH10B strain carrying an empty pBluescript vector was used as a positive control and both cold-sensitive strains (DH10B ΔcsdA and DH10B ΔcsdA Δrnr) also carrying empty pBluescript vectors were used as negative controls. All the clones were grown during 5 h at 37°C with slight agitation and then their OD600 nm values were adjusted to 0.03. They were grown in 96-well microtiter plates during 140 h at 15°C with a slight agitation (80 rpm) and OD measures were taken in the SPECTROstar Nano (BMG Labtech) four times per day. Six replicates per clone were introduced in each assay and each experiment was repeated at least three times using independent cultures to corroborate the results. (A) Results obtained for 6 cold-resistant clones (pC1–pC6) isolated from library C (Colobanthus quitensis) in the screening performed with the single cold-sensitive mutant (DH10B ΔcsdA). (B) Results obtained from the remaining 7 cold-resistant clones isolated from library C (pC7–pC13) in the screening performed with the single cold-sensitive mutant. (C) Results obtained for the 6 cold-resistant clones (pD1–pD6) isolated from library D (Deschampsia antarctica) in the screening performed with the single cold-sensitive mutant. (D) Results obtained for the cold-resistant clone pD7 isolated from library D in the screening performed with the cold-sensitive double mutant (DH10B ΔcsdA Δrnr).
A total of 60 cold-tolerant colonies (32 from library C and 28 from library D) were obtained from both libraries hosted in the cold-sensitive strain DH10B ΔcsdA. To ensure that the resistance phenotype was not due to the presence of spontaneous mutations, all these colonies were pooled and their recombinant plasmids were isolated and used to transform DH10B ΔcsdA cells. Finally, after re-isolating under cold conditions and studying the restriction patterns (obtained with XhoI and XbaI restriction enzymes) of 40 colonies from each library, we found 13 different clones from library C (pC1–pC13) and 6 clones from library D (pD1–pD6) that showed a clear cold-tolerance phenotype (Figure 1). On the other hand, in the screening performed with the double mutant DH10B ΔcsdA Δrnr, which is more sensitive to low temperatures than DH10B ΔcsdA, only one cold-tolerant colony was obtained from library D (pD7) after retransforming and analyzing 30 colonies from each library, following the same protocol described for the screening performed in the single mutant DH10B ∆csdA (Figure 1).
The DH10B ΔcsdA and DH10B ΔcsdA Δrnr strains transformed with the recombinant plasmids also showed a better growth rate under low temperatures (15°C) in liquid media (Figure 2) than the cold-sensitive strains carrying an empty vector. Growth parameters calculated from these tests are compiled in Supplementary Table S2, showing that cold-tolerant clones were able to grow faster (lower generation time) or start to grow earlier than cold-sensitive mutants. On the other hand, no differences in growth rates were observed when cells were grown at 37°C on solid media (see Supplementary Figure S1) or when they were grown at 30°C in liquid media (Supplementary Figure S2). These results supported the idea that the environmental genes cloned in the pBluescript II SK (+) vector specifically contributed to an improvement of E. coli growth at low temperatures but not to a general cellular development as differences were not detected under optimal (37°C) or suboptimal (30°C) conditions.
Identification of genes conferring cold tolerance
The metagenomic DNA fragments of each plasmid were sequenced by prime walking and a total of 29 genes were predicted in the 13 clones (pC1–pC13) isolated from library C and in the 6 clones (pD1-pD6) from library D in the screening performed with the DH10B ΔcsdA strain (Table 1; Figure 3). Moreover, two genes were predicted in the cold-tolerant pD7 clone isolated in the screening performed with the double mutant (Table 1; Figure 3). Sequence analyses of these environmental DNA fragments revealed the presence of a single open reading frame (ORF) in pC4, pC5, pC7, pC8, pC13, pD1, pD3, pD4, pD5, and pD6; two ORFs in pC1, pC2, pC3, pC6, pC9, pC10, pC12, pD2, and pD7; and three ORFs in pC11. The G + C content of these DNA fragments ranged from 39.2 to 76.1%, indicating their diverse phylogenetic origin. Most of the genes analyzed in this study encode amino acid sequences similar to bacterial proteins. Using the BLASTp tool (NCBI website), we tried to relate them with previously characterized proteins, but 8 of them were similar to conserved hypothetical proteins (28%) and 4 were classified as unknown proteins (14%) as no similarity was found between them and previously annotated proteins (Table 1; Figure 3). Using the NCBI Conserved Domain Database (CDD) and the Pfam Database to functionally categorize the recovered genes, a large variety of domains could be predicted (Table 1). In addition, DNA-binding domains were predicted in some sequences using two bioinformatics programs (Table 1).
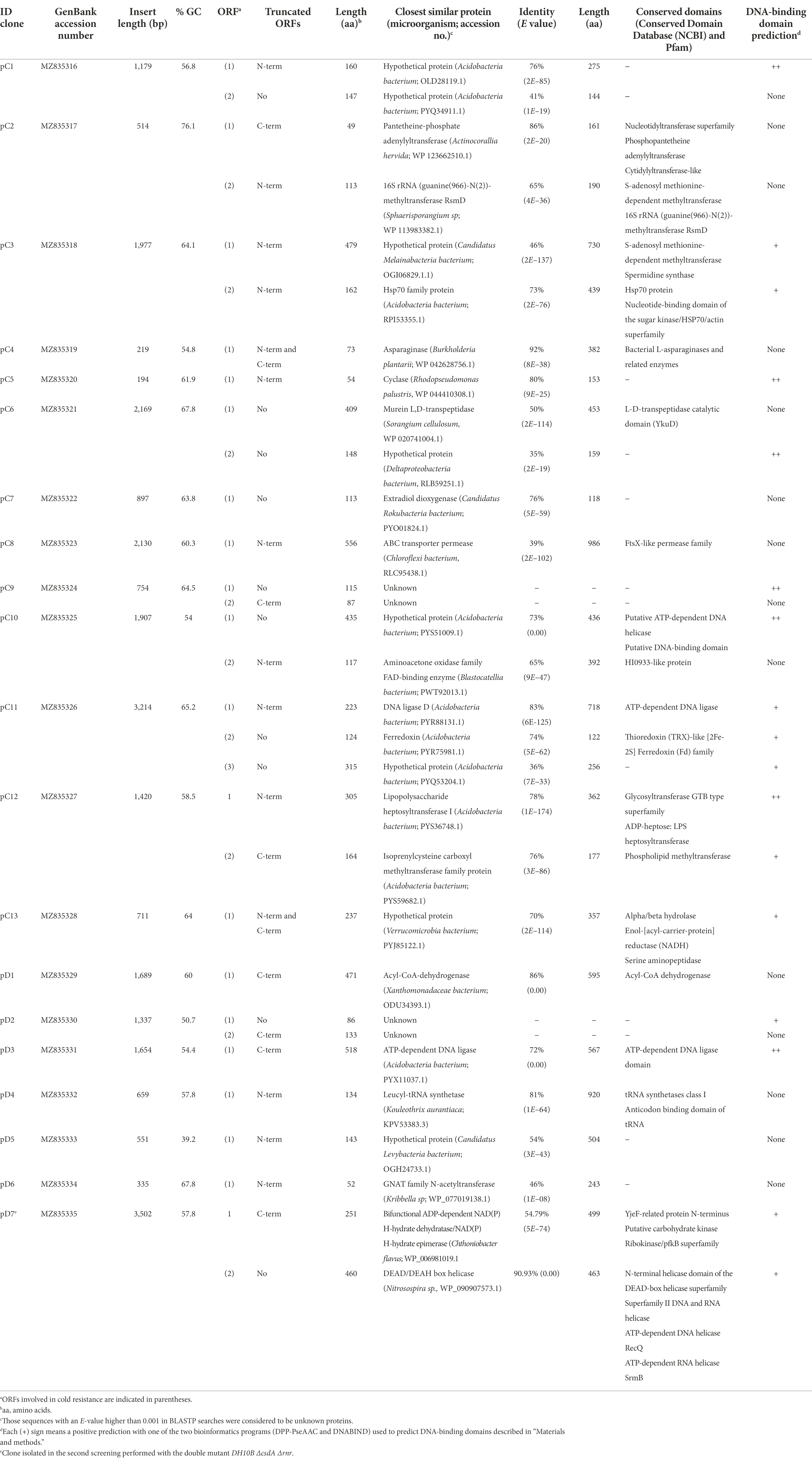
Table 1. Description of cold-resistance clones isolated from metagenomic libraries C and D using DH10B csdA and DH10B ΔcsdA Δrnr strains as hosts, similarities with other known sequences and conserved domains found in them.
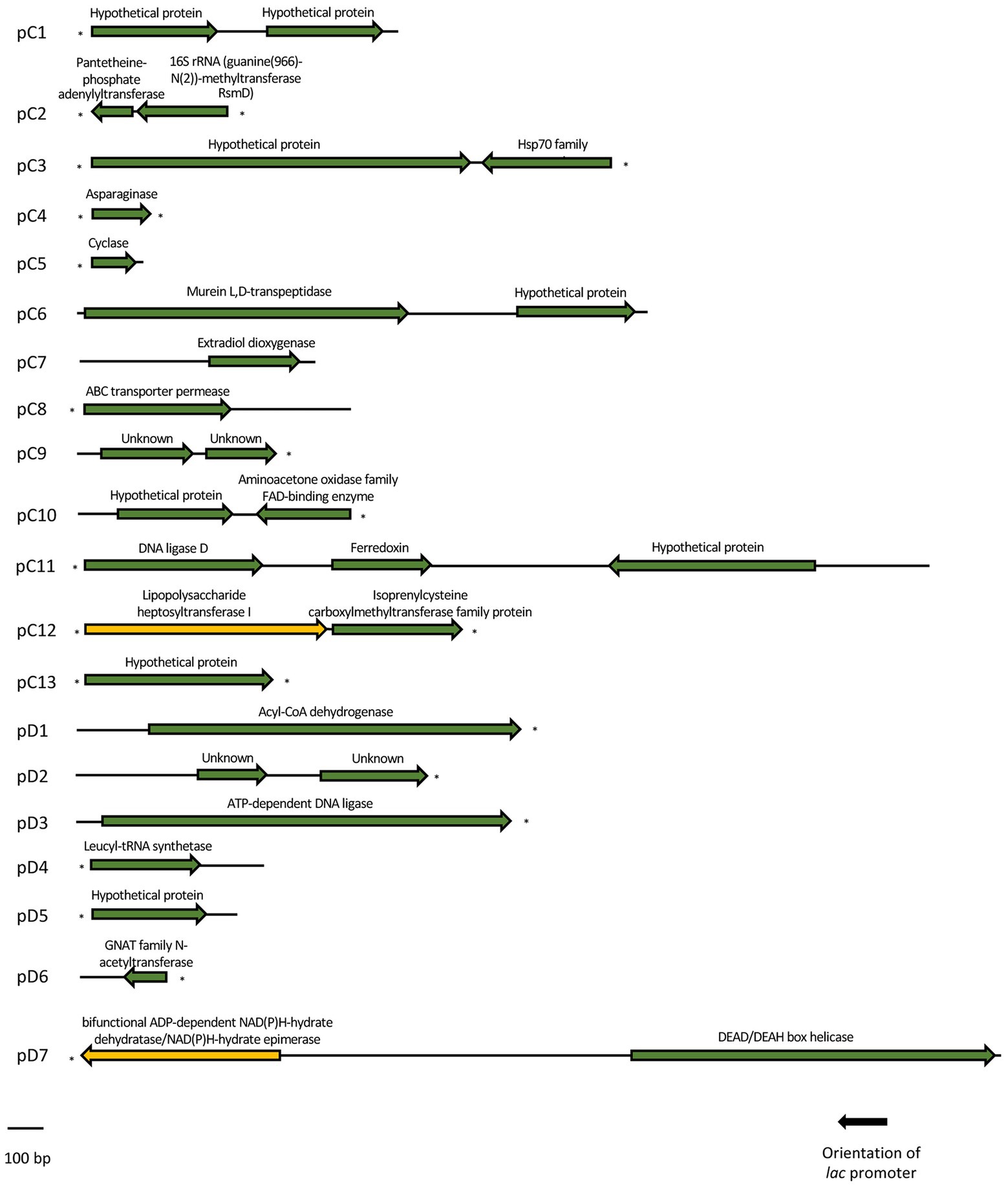
Figure 3. Schematic organization of the ORFs identified in each of the 20 cold-resistant clones. Arrows indicate the location and the transcriptional orientation of all the putative genes, being marked in green the ones related to a cold-tolerant phenotype and in yellow the ones that were not related to cold-tolerance. Truncated ORFs are indicated with an asterisk.
The recombinant plasmids pC4, pC5, pC7, pC8, pC13, pD1, pD3, pD4, pD5, and pD6 contained only one ORF each, which are responsible for their cold-tolerance phenotype (Table 1; Figures 1, 2). These clones encode diverse proteins, most of them previously annotated in different organisms. Briefly, pC4 encodes an asparaginase, pC5 a cyclase, pC7 an extradiol dioxygenase, pC8 an ABC transporter permease, pC13 a hypothetical protein, pD1 an acyl-CoA-dehydrogenase, pD3 an ATP-dependent DNA ligase, pD4 a leucyl-tRNA synthetase, pD5 a hypothetical protein, and pD6 a GNAT family N-acetyltransferase (Table 1). However, the rest of the clones contained recombinant plasmids with two or more ORFs that were independently subcloned to define the putative genes responsible for cold tolerance. The results from solid and liquid tests comparing the tolerance of each subclone to the complete clone and to control strains are compiled in Supplementary Figures S3, S4. The DNA insert of pC1 contains two ORFs, both encoding hypothetical proteins. Both genes showed cold tolerance when they were subcloned independently and were able to grow similarly to the complete clone pC1 at 15°C in solid and liquid media (Supplementary Figures S3, S4A). In the case of the DNA insert from pC2, two ORFs were identified: orf1 encoding a pantetheine-phosphate adenylyltransferase and orf2 encoding a 16S rRNA methyltransferase. The clones harboring each one of these ORFs were cold resistant compared to our negative control and they were able to grow faster at 15°C in solid and liquid media than the DH10B ΔcsdA strain and even the complete clone (Supplementary Figures S3, S4B). The DNA insert of pC3 contains two ORFs: orf1 encodes a hypothetical protein and orf2 encodes a Hsp70 family protein. Both ORFs clearly conferred tolerance to low temperatures but orf2 is slightly more resistant than orf1 when subcloned independently (Supplementary Figures S3, S4C). In the case of the DNA insert of pC6, two ORFs were identified, encoding a murein L,D-transpeptidase and a hypothetical protein, respectively. Both genes showed cold tolerance when they were subcloned independently (Supplementary Figures S3, S4D). The DNA insert of pC9 also contains two ORFs, both encoding unknown proteins. Both ORFs conferred cold tolerance when independently subcloned (Supplementary Figures S3, S4E). pC10 clone contained a recombinant insert with two possible ORFs, encoding a hypothetical protein and an aminoacetone oxidase, respectively. Both ORFs showed a similar response to low temperatures when independently subcloned and this response was also similar to that observed for the complete clone, thus both subclones were related to cold tolerance (Supplementary Figures S3, S4F). Three ORFs have been described in the DNA insert of pC11: orf1 encoding a DNA ligase, orf2 encoding a ferredoxin and orf3 encoding a hypothetical protein. These three ORFs showed a similar response to cold when they were subcloned independently and it was very similar to the cold tolerance showed by the complete clone (Supplementary Figures S3, S4G). pC12 clone contained a recombinant insert with two ORFs: orf1 encodes a lipopolysaccharide heptosyltransferase and orf2 encodes an isoprenylcysteine carboxyl methyltransferase. In this case, orf2 seemed to be the main responsible for the cold tolerance showed by pC12, as orf1 grew considerably slower than orf2 at 15°C (Supplementary Figure S4H). This difference was best detected in the liquid test. The DNA insert of pD2 also contains two ORFs, both encoding unknown proteins. Both ORFs seemed to be responsible for cold tolerance (Supplementary Figures S3, S4I). Finally, pD7 clone isolated from the double mutant screening was also formed by two ORFs: orf1 encodes a bifunctional ADP-dependent NAD(P)H-hydrate dehydratase/NAD(P)H-hydrate epimerase and orf2 encodes a DEAD/DEAH box helicase. The second one was clearly related to cold tolerance (Supplementary Figures S3, S4J), whereas the orf1 did not confer cold tolerance when independently subcloned.
Evaluation of cold tolerance conferred by overexpressed Escherichia coli homologs in the cold-sensitive mutant DH10B ΔcsdA
We found E. coli homologs for 16 of the 29 cold-tolerant genes described in this work (55% of the genes; Table 2). For the rest of the genes (45% of the genes), we did not find any homologs in the E. coli genome, so they suppose totally new functions for our cold-sensitive strain. Of the 16 E. coli homologs, only 10 were identified in the genome of the DH10B strain. Seven of them were overexpressed in the DH10B ΔcsdA strain and their cold tolerance was tested in solid and liquid medium at 15°C in comparison with their respective genes identified in this study (Figures 4, 5). We excluded from these overexpression tests the E. coli homologs for the pC10 orf1 and pC13 genes, since they encode hypothetical proteins, and also for pD7 orf2, which encodes an RNA helicase that could be complementing the csdA mutation in the cold-sensitive E. coli strain (Table 1). The overexpression of the seven E. coli homologs also increased cold tolerance and these transformed clones were able to grow similarly to the cold-resistant clones originally isolated from the Antarctic libraries (Figures 4, 5). These results suggest that the proteins encoded by these E. coli genes might be directly or indirectly involved in cold-adaptation processes of this bacterium and, therefore, the overexpression of their environmental homologs also provided an advantage to grow at low temperatures.
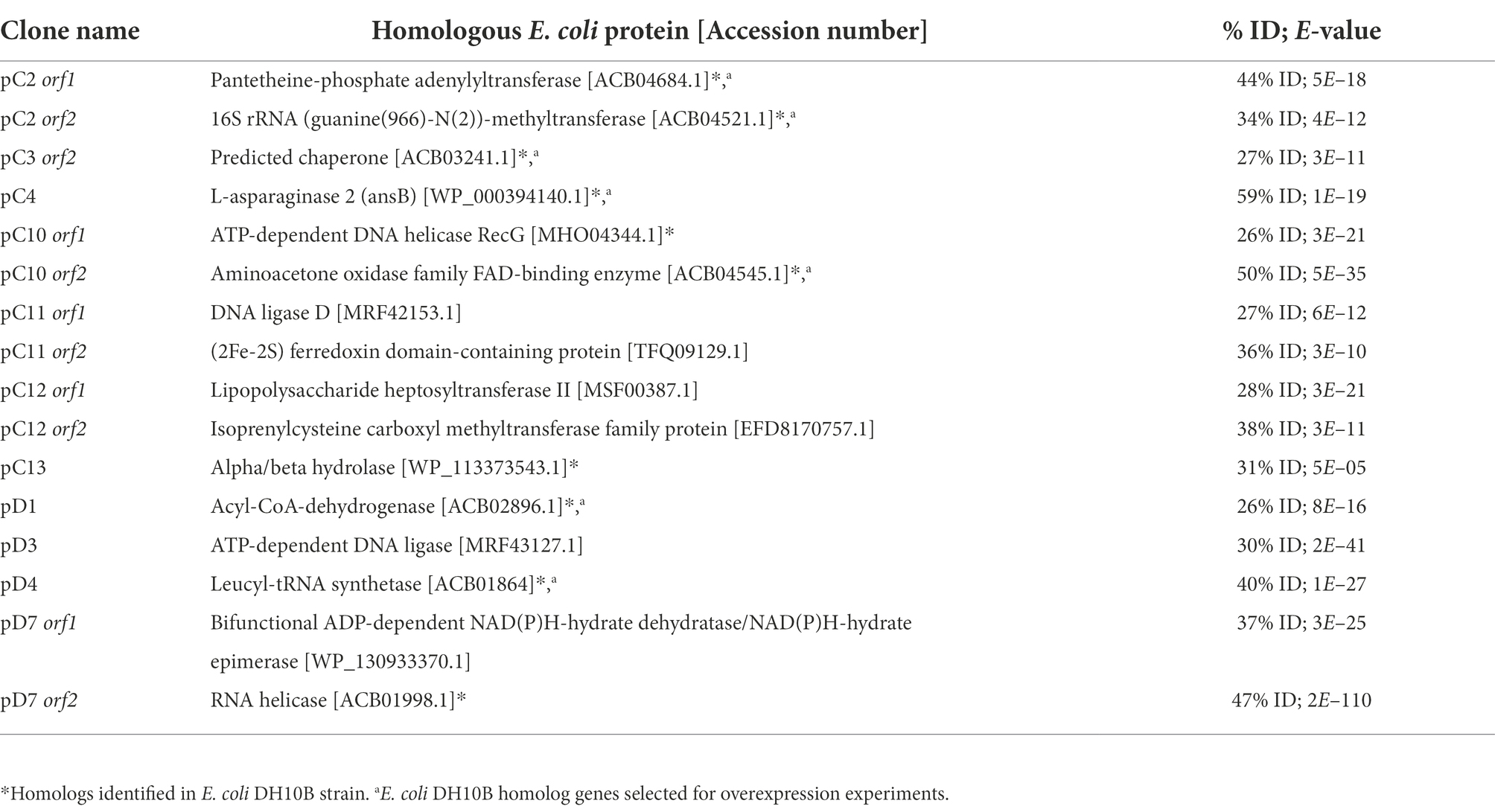
Table 2. Escherichia coli proteins homologous to cold-tolerant proteins encoded by the genes isolated from Antarctic metagenomic libraries.
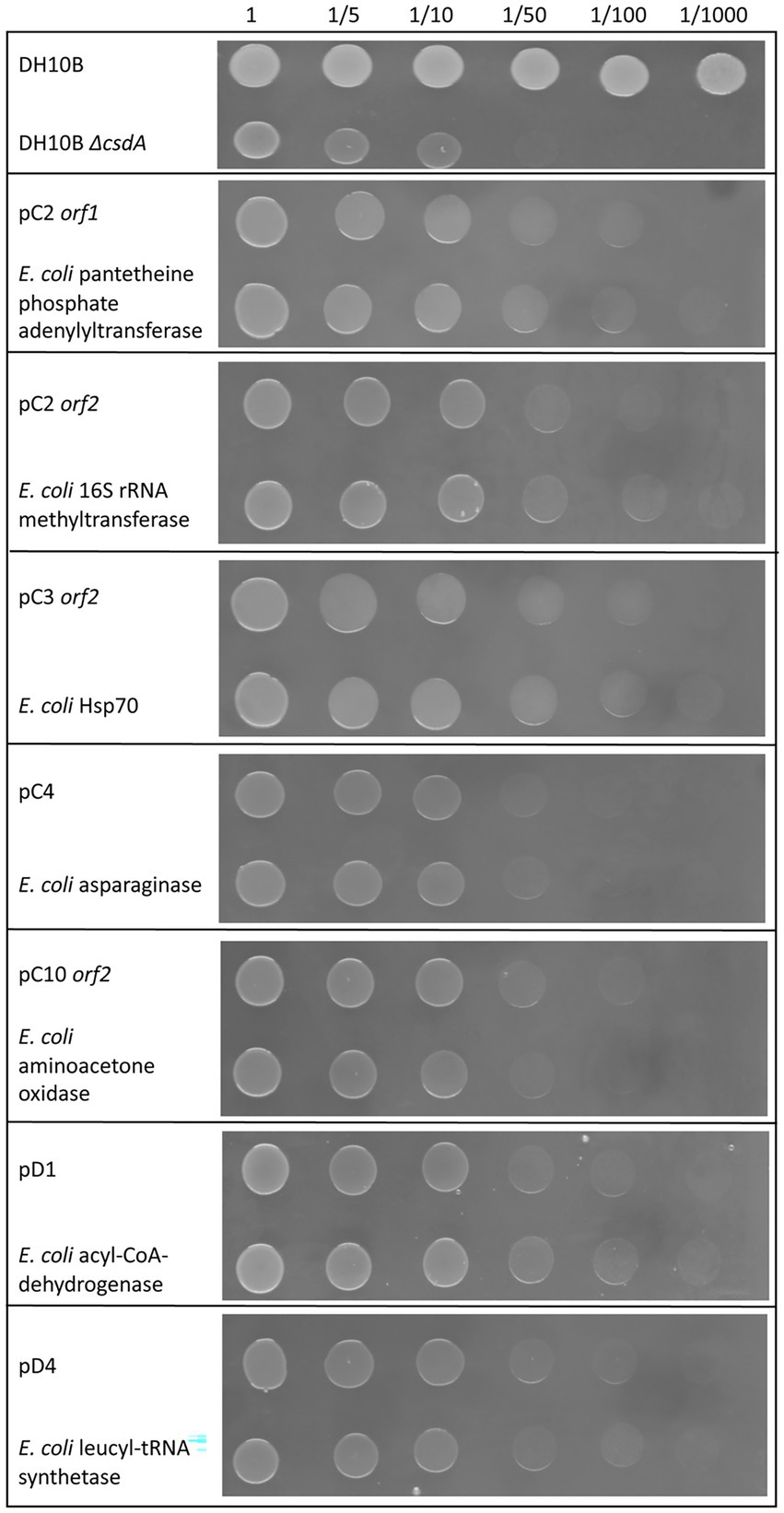
Figure 4. Comparative drop assay performed with some of the cold-tolerant genes characterized in this study and their homologous genes in Escherichia coli (see Table 2) expressed in the DH10B ΔcsdA strain. DH10B strain carrying an empty pBluescript vector was used as a positive control and the single cold-sensitive mutant (DH10B ΔcsdA) also carrying an empty pBluescript vector was used as a negative control. The cell density of overnight cultures was adjusted to OD600 nm values of 1.0, serial dilutions were performed and 10 μl drops of each dilution were inoculated on LB-Ap50 plates and grown at 15°C for 10 days. Each experiment was repeated at least three times using independent cultures.
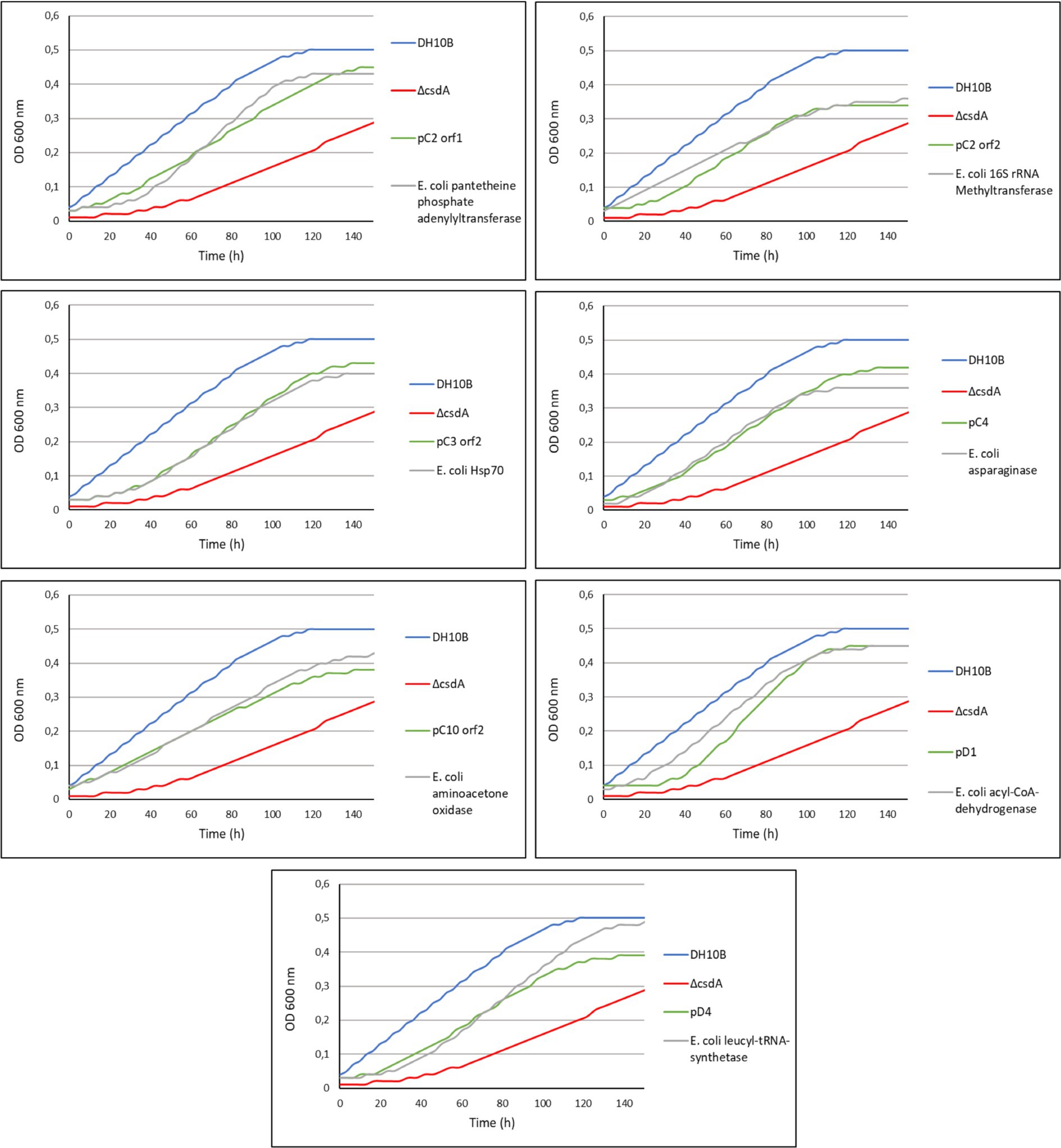
Figure 5. Comparison of the growth curves of some of the cold-tolerant genes characterized in this study and their homologous genes in E. coli. DH10B strain carrying an empty pBluescript vector was used as a positive control and the single cold-sensitive mutant (DH10B ΔcsdA) also carrying an empty pBluescript vector was used as a negative control. All clones were grown during 5 h at 37°C with a slight agitation and then their OD600 nm values were adjusted to 0.03. They were grown in 96-well microtiter plates during 140 h at 15°C with a slight agitation (80 rpm) and OD measures were taken in the SPECTROstar Nano (BMG Labtech) four times per day. Six replicates per clone were introduced in each assay and each experiment was repeated at least three times using independent cultures to corroborate the results.
Testing the resistance to UV-radiation of the cold-tolerant clones: Looking for cross-resistance
After exposing the cold-tolerant clones to UV-B radiation (4 mJ/cm2), we observed that 8 of the 20 clones were considerably resistant to this type of stress conditions, approximately one order of magnitude higher compared to the negative controls (DH10B ΔcsdA or DH10B ΔcsdA Δrnr strains; Figure 6). These UV-resistant clones were the following: pC2, pC3, pC6, pC9, pC13, pD5, pD6, and pD7. Of them, pC13, pD5, and pD6 are composed of a single putative ORF, thus they were considered to be responsible for the UV-resistance. pC13 encodes a hypothetical protein that contains different conserved motifs: alpha/beta hydrolase, enol-[acyl-carrier-protein] reductase (NADH) and serine aminopeptidase). pD5 encodes a hypothetical protein but, in this case, it does not have any conserved motif. Finally, pD6 encodes a GNAT family N-acetyltransferase. For the other clones that contained two ORFs (Table 1), the UV-resistance test was done with each ORF subcloned independently to know which one is directly involved in UV-resistance (Figure 6). In the case of pC2 clone, it seemed that orf2 was clearly related to UV-resistance. It encodes for a protein that is similar (65% ID) to a 16S rRNA methyltransferase (Table 1) and that contains a conserved motif of a S-adenosyl-methionine-dependent methyltransferase. In the case of pC3 and pC6 clones, neither of their ORFs was clearly involved in UV-resistance when independently subcloned, therefore, both ORFs seemed to be needed together to protect cells from UV-radiation (Figure 6). pC9 clone also contained two ORFs and orf2 was clearly the one involved in UV-resistance. It encodes for an unknown protein, not previously characterized, and without any known conserved motif. Finally, in the case of the pD7 clone isolated in the screening performed with the double mutant, orf2 might be the one involved in UV-resistance but its response was much less intense than that observed for the complete clone (Figure 6). This orf2 encodes a complete DEAD/DEAH box helicase (91% ID) and showed the typical conserved motifs of these family proteins (Table 1). The results of the control assay performed with the same clones and subclones not irradiated with UV-B radiation are compiled in Supplementary Figure S5.
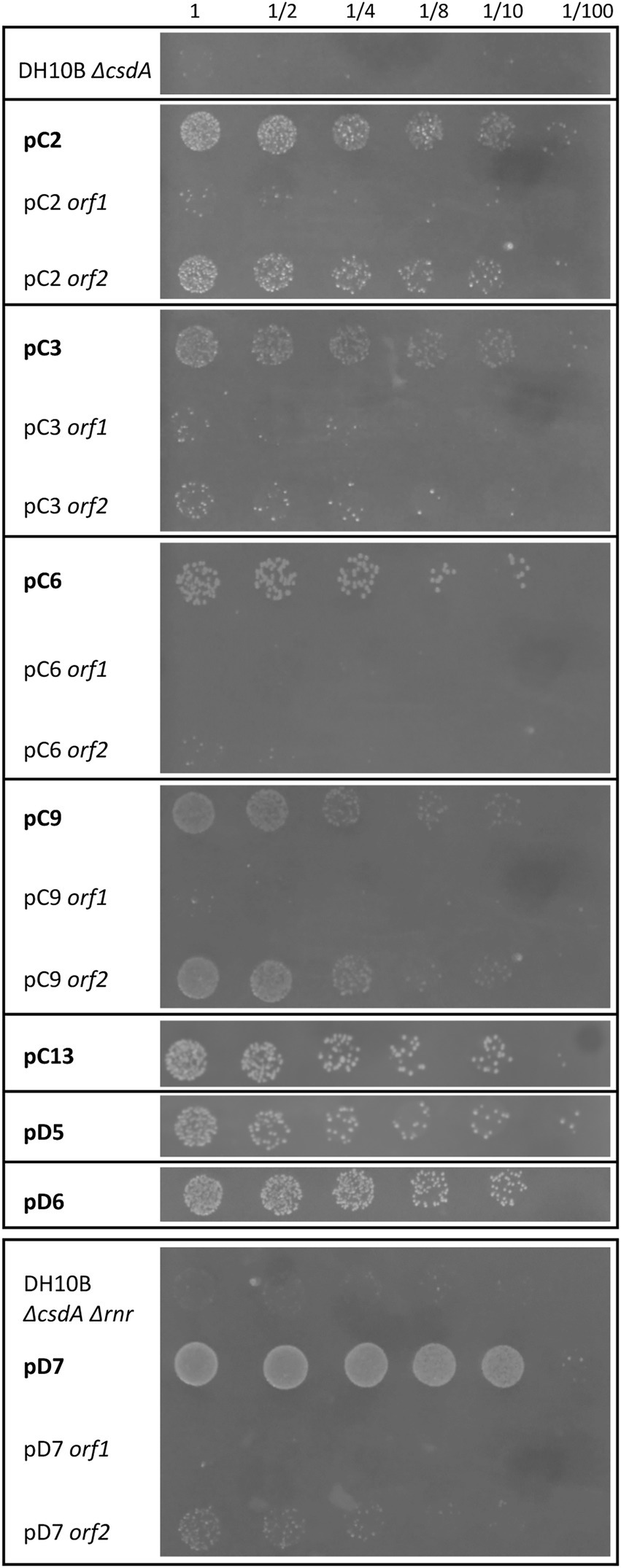
Figure 6. Drop assay of the cold-resistant clones and subclones that were also resistant to UV-B radiation. Both cold-sensitive strains (DH10B ΔcsdA and DH10B ΔcsdA Δrnr) carrying empty pBluescript vector were used as negative controls. The cell density of overnight cultures was adjusted to OD600 nm values of 1.0, serial dilutions were performed and 10 μl drops of each dilution were inoculated on LB-Ap50 plates and irradiated with a dose of UV-B of 4 mJ/cm2. UV-B resistance was evaluated after growing cells overnight at 37°C. Each experiment was repeated at least three times using independent cultures.
Discussion
Most of the molecular mechanisms of cold adaptation have been investigated in cultured microorganisms. Therefore, in order to expand the knowledge of these strategies, in this study, we proposed to identify new genes involved in cold adaptation from rhizosphere microorganisms of two Antarctic plants: Colobanthus quitensis and Deschampsia antarctica. To our knowledge, this is the first study applying a functional metagenomics approach (as culture-independent technique) to isolate cold-tolerance genes from environmental microorganisms. This strategy has been successfully used to study mechanisms of adaptation to diverse extreme conditions, such as acidic pH (Guazzaroni et al., 2013), metals (Mirete et al., 2007; Morgante et al., 2014), high salinity (Kapardar et al., 2010; Culligan et al., 2012, 2013; Mirete et al., 2015), UV-radiation (Lamprecht-Grandío et al., 2020), or perchlorate (Díaz-Rullo et al., 2021).
In this work, a total of 29 genes that enhanced the growth of E. coli cold-sensitive strains at low temperatures were identified and characterized. In this way, 21 cold-tolerant genes were isolated from Colobanthus quitensis library (C) and 8 from Deschampsia antarctica library (D). Twelve of these cold-tolerant genes (41%) were identified as genes encoding conserved hypothetical proteins (7 from library C; 1 from library D) and unknown proteins (no similarity to other proteins) (2 from library C; 2 from library D) that could be involved in new cold-tolerance mechanisms not described until now. On the other hand, 17 genes (59%) encode proteins similar to others previously characterized, including a wide variety of different potential functions that can be classified into four categories: (i) metabolic reactions (6 from library C; 1 from library D), (ii) transport and cellular processes (2 from library C), (iii) genetic information processing (3 from library C; 4 from library D); and (iv) non-totally defined function (1 from library C). Only one protein was included in the latter category: a cyclase partially encoded by the truncated gene isolated in the pC5 clone. It is difficult to identify the function of the cyclase isolated in this work, since it is truncated, and no conserved motifs have been described in it.
Of the 29 cold-tolerant genes isolated from the Antarctic metagenomic libraries, E. coli homologs were found for only 16 of them (10 in the specific case of the DH10B strain genome) (Table 2). The remaining 13 genes provided totally new functions for this bacterium, optimizing its growth at low temperatures. Seven of the 10 E. coli DH10B homologs were overexpressed in the cold-sensitive mutant DH10B ΔcsdA and all conferred increased cold tolerance (Figures 4, 5). In fact, all these genes have been related to cold-acclimation processes in other organisms as explained in detail below. Furthermore, some transcriptomic and proteomic analysis performed with E. coli cultures have confirmed an upregulation of some of them or other related genes after cold shock (Mihoub et al., 2003; Phadtare and Inouye, 2004; Vidovic et al., 2011). Therefore, these E. coli genes, most related to DNA repair and replication, translation, protein folding or acetyl-CoA metabolism, are involved in cold stress response and their overexpression contribute to better growth of the cold-sensitive strain in a similar way as their environmental homologs (Figures 4, 5).
Cold-tolerant genes encoding proteins involved in metabolic reactions
Regarding the category of “metabolic reactions,” seven genes were identified that code for: pantetheine-phosphate adenylyltransferase (pC2 orf1), asparaginase (pC4), murein L,D-transpeptidase (pC6 orf1), extradiol dioxygenase (pC7), aminoacetone oxidase family FAD-binding enzyme (pC10 orf2), ferredoxin (pC11 orf2), and acyl-CoA dehydrogenase (pD1). Although these metabolic proteins have very diverse functions, many of them could be clearly connected to cold-tolerance mechanisms of adaptation. In fact, overexpression experiments performed with E. coli homologs for pantetheine-phosphate adenylyltransferase, asparaginase, aminoacetone oxidase, and acyl-CoA-dehydrogenase also conferred greater cold tolerance to the cold-sensitive strain (Figures 4, 5).
The pantetheine-phosphate adenylyltransferase (PPAT) and the acyl-CoA dehydrogenase (ACAD) are two proteins related to CoA metabolism. PPAT is a nucleotidyltransferase that participates in the biosynthesis of CoA from the pantothenic acid (Rubio et al., 2008) while ACAD enzyme catalyzes the first reaction of each catabolic β-oxidation cycle, favoring the release of a variable number of acetyl-CoA molecules from a fatty acid molecule until its complete dissociation (Swigonová et al., 2009). Therefore, both proteins are directly involved in increasing CoA levels, an essential cofactor in numerous biosynthetic, degradative, and energy obtaining pathways. Some authors have reported that proteins related to the biosynthesis of acetyl-CoA and ketone bodies reutilization (MetF, ScoB or MmsA) are induced at subzero temperatures in the Antarctic bacterium Psychrobacter sp. (Koh et al., 2017). Ting et al. (2010) detected a higher expression of the ACAD enzyme at 10°C compared to the levels detected at 30°C in the proteomic study performed with the marine bacterium Sphingopyxis alaskensis. Finally, acetyl-CoA is a precursor of fatty acid synthesis, and ACAD enzymes might participate in the modification of lipid membrane composition. At low temperatures, shorter and more unsaturated fatty acids conferred a greater fluidity to membranes, as it was described in the marine cyanobacteria Synechococcus sp. (Koh et al., 2017; Pittera et al., 2018).
As a result of the increased acetyl-CoA/CoA ratio during cold acclimation, other metabolic pathways, such as the methylglyoxal (MGO) metabolism, are also affected (Raymond-Bouchard et al., 2017). A protective role of the aminoacetone oxidase enzyme under stress conditions in the bacterium Streptococcus oligofermentans has been described (Molla et al., 2014). Aminoacetone is a pro-oxidative compound that can be oxidized to the toxic MGO. However, aminoacetone oxidase may protect cells by acting as an antioxidant agent, reducing cellular aminoacetone contents under stress conditions, and avoiding the production of the toxic MGO (Tressel et al., 1986; Molla et al., 2014). On the other hand, ferredoxins are electron transfer proteins that are ubiquitous in biological redox systems across all domains of life. They constitute a highly diverse family of iron–sulfur proteins that links important biochemical pathways for energy transduction, nutrient assimilation, and primary metabolism (Atkinson et al., 2016). Ferredoxins show typical characteristics of cold-adapted enzymes, such as an increased structural flexibility, a low stability at moderate temperatures, and high activity rates at low temperatures (Cvetkovska et al., 2018).
The extradiol dioxygenases constitute a large and diverse group of metalloenzymes that catalyze the rupture of aromatic rings in many different aerobic catabolic pathways (Vaillancourt et al., 2004). To our knowledge, these enzymes have not previously been related to cold adaptation in a specific way. Our results have demonstrated that this enzyme may benefit the cell growth under low temperatures since is fully linked to catabolic reactions. Moreover, extradiol dioxygenases were also related to the acetyl-CoA metabolism, which has an essential role in cold adaptation as we have previously explained (Nogales et al., 2007; Pérez-Pantoja et al., 2010).
Many amino acid metabolism reactions are altered during cold adaptation with the aim of contributing to cell survival (Sundareswaran et al., 2010; Bocian et al., 2015). The L-asparaginase is one of these enzymes whose expression is induced under low temperatures in different organisms (Phadtare and Inouye, 2004; Cho et al., 2007; Sundareswaran et al., 2010; Bocian et al., 2015). Moreover, L-asparaginase activity has been described as beneficial for cold adaptation in the psychrophilic bacteria Pseudomonas syringae (Sundareswaran et al., 2010). We have also isolated a cold-tolerant gene related to cell envelope metabolism that encodes a murein L,D-transpeptidase. This enzyme participates in the biosynthesis of the bacterial peptidoglycan that is part of bacterial cell walls. A thicker peptidoglycan layer and an upregulation of peptidoglycan biosynthesis have been described in different studies conducted at low temperatures (Rodrigues et al., 2008; Chen et al., 2009; Raymond-Bouchard et al., 2017; Collins and Margesin, 2019). A proteomic analysis performed with the psychrophilic bacteria Planococcus halocryophilus showed an increased expression of a DD-transpeptidase at −10°C of more than 9-fold compared to the values obtained at 23°C. Likewise, other enzymes related to peptidoglycan biosynthesis (MurA and MurB) also increased their expression levels at subzero temperatures (Mykytczuk et al., 2013; Raymond-Bouchard et al., 2017).
Cold-tolerant genes encoding proteins related to transport and membrane processes
Two cold-tolerant genes possibly encoding an ABC transporter permease (pC8) and an isoprenylcysteine carboxyl methyltransferase (pC12 orf2) are included in the second category of “transport and cellular processes.” Both proteins may favor cellular growth under low temperatures through different mechanisms. ABC transporters participate in a wide range of physiological processes. Specifically, previous transcriptomic and proteomic analysis have demonstrated the relevant role of ABC transporters on cold adaptation due to their ability to import/export a variety of different molecules across the cytoplasmic and outer membranes that may be reused for de novo synthesis of different nutrients and metabolites that are essential for cold acclimation (Phadtare and Inouye, 2004; Mykytczuk et al., 2011; Koh et al., 2017). On the other hand, isoprenylcysteine carboxyl methyltransferases (ICMTs) are proteins responsible for the last step of a post-translational modification termed prenylation that can affect numerous proteins, favoring a better association of proteins to cellular membranes, protein–protein interactions, and protein stability (Zhang and Casey, 1996; Hála and Žárský, 2019). Recently, plant studies supported a possible connection between protein prenylation and its response to both abiotic and biotic stress conditions (Hála and Žárský, 2019).
Cold-tolerant genes encoding proteins responsible for genetic information processing
Interestingly, seven cold-tolerant genes isolated in this work are included in the third category of “genetic information processing”: a 16S rRNA methyltransferase (pC2 orf2), a Hsp70 family protein (pC3 orf2), a DNA ligase (encoded by two clones: pC11 orf1 and pD3), a leucyl-tRNA synthetase (pD4), a GNAT family N-acetyltransferase (pD6), and a DEAD/DEAH box helicase (pD7). In addition, overexpression experiments performed with E. coli homologs for 16S rRNA methyltransferase, Hsp70 family protein, and leucyl-tRNA synthetase also conferred higher cold tolerance to the cold-sensitive strain, which confirms its direct relation with cold acclimation (Figures 4, 5).
16S rRNA (guanine-966-N2)-methyltransferase is responsible for the methylation of the 16S rRNA molecule at the N2 position of its G966 nucleotide (Baldridge and Contreras, 2013). Nucleotide modifications are considered essential for ribosome maturation, but recent studies have also related them to response to stressful conditions such as temperature changes or antibiotic presence (Lesnyak et al., 2007; Sergiev et al., 2007). Studies performed with E. coli knockout mutants for methyltransferase genes have shown reduced growth rate under thermal stress compared to the wild-type strain.
Other enzymes related to RNA processing are aminoacyl-tRNA synthetases. These enzymes catalyze the binding of each amino acid to its tRNA molecule in a very specific reaction (McClain, 1993). A proteomic analysis performed with the psychrophile Planococcus halocryophilus showed that a higher number of proteins related to amino acid and nucleotide recycling are detected at low temperatures. In fact, a higher abundance of some tRNA synthetases (for alanine and glutamic) was detected at –10°C (Raymond-Bouchard et al., 2017). On the other hand, studies with inactive pear embryos during its dormant phase evaluated the activity of aminoacyl-tRNA synthetases and they confirmed a higher activity of these enzymes after cold treatment (Tao and Khan, 1974).
Molecular chaperones such as those of the Hsp70 family can interact with different proteins and favor their proper folding to achieve a specific functional conformation. They also participate in other cellular processes such as: molecule transport, disaggregation and degradation of different proteins, being important for many different processes related to DNA replication, cell division, and metabolism (Maillot et al., 2019). Recent studies have supported the induction of heat-shock proteins in response to low temperatures, since correct folding and refolding of cold-damaged proteins are vital after cold shock (Lelivelt and Kawula, 1995; Chow and Tung, 1998; Phadtare and Inouye, 2004; Stetina et al., 2015; Maillot et al., 2019). In fact, cell recovery rate after a freezing period at −80°C was related to the abundance of heat-shock proteins previously accumulated in E. coli cells (Chow and Tung, 1998).
Two cold-tolerant genes isolated in the Antarctic libraries encode DNA ligases, which are DNA-joining enzymes that are essential for survival of all organisms due to their critical roles in DNA replication and repair (Berg et al., 2019). Some genomic studies performed with Psychrobacillus strains have suggested an evolution of their pan-genome structures to allow their adaptation to extremely cold habitats, consisting of changing their genome contents to gain higher capacity for DNA repair, translation, and membrane transport (Choi et al., 2020). Similarly, some proteomic analyses carried out with different organisms have also supported the idea that an upregulation of proteins related to DNA repair, transcription, and translation is required to adapt to cold stress conditions (Raymond-Bouchard et al., 2017; Liu et al., 2020).
Another gene totally related to cold acclimation is the one encoding a DEAD/DEAH box helicase (pD7 orf2), isolated from library D in the screening performed with the double mutant DH10B ∆csdA ∆rnr. This gene could be complementing the mutation in the csdA gene that encodes a highly conserved DEAD box RNA helicase essential for cold acclimation in E. coli, and therefore would allow the growth of this mutant strain at 15°C. DEAD box helicases such as CsdA participate in ribosome subunit biogenesis, translation initiation, cell transport, gene regulation, and stabilization/degradation of mRNAs. Alternatively, they may prevent or resolve misfolded proteins, providing assistance to rRNAs to reach their active conformation (Awano et al., 2007; Phadtare, 2012). All of these actions are essential during cold adaptation, where secondary structures of RNA are stably formed under low temperatures and helicases are required for their unwinding, for ribosome binding, and, therefore, for translation initiation (Yamanaka and Inouye, 2001; Phadtare, 2012).
Finally, clone pD6 encodes a N-terminal truncated GNAT. GNATs (general control non-repressible 5-related N-terminal acetyltransferases) constitute an important family of proteins that includes more than 10,0000 members among eukaryotes, bacteria, and archaea (Favrot et al., 2016). Acetylation appears as a major regulatory post-translational modification and is as widespread as phosphorylation. Specifically, GNAT transfers an acetyl group from acetyl-CoA to a wide diversity of substrates, from small molecules such as aminoglycoside antibiotics to macromolecules. In this way, these acetyltransferases are known to be involved in several cellular processes such as peptidoglycan recycling, detoxification pathways, production of virulence factors, iron acquisition, or redox balance (Favrot et al., 2016). Therefore, although they have not been directly connected to cold tolerance in prokaryotes, since they participate in many processes, they could also be involved in it.
New hypothetical and unknown proteins related to cold tolerance
In addition to all these cold-tolerant genes that are similar to previously characterized genes (17 of 29 genes) and that can be connected to a cold response, some genes encoding conserved hypothetical (8) and unknown (4) proteins have also been isolated from the Antarctic metagenomic libraries. Some of these hypothetical proteins showed conserved domains that may relate them to specific functions (Table 1). In this way, the hypothetical protein encoded by the pC3 orf1 gene might act as a spermidine synthase or as a S-adenosyl methionine-dependent methyltransferase. The hypothetical protein encoded by the pC10 orf1 gene may be a putative ATP-dependent DNA helicase and the one encoded by the single gene of the pC13 clone might act as an alpha/beta hydrolase. For the remaining hypothetical and unknown proteins, no conserved motifs have been identified. However, some of them seem to have DNA-binding domains (predicted by both bioinformatic programs used in this work, see Materials and methods section) as the ones encoded by pC1 orf1, pC6 orf2, pC9 orf1 or pC10 orf1 (Table 1). Therefore, some of these proteins could be related to DNA repair or protection mechanisms or genetic information processing, both essential during cold acclimation. In conclusion, regardless of their still unknown specific function, these hypothetical or unknown proteins provide totally new functions to E. coli cells, favoring their growth under low temperatures and could be of interest for future research.
Resistance to UV-B radiation of some of the cold-tolerant clones: A cross-protection against other type of stress conditions
Exposure to low temperatures produces a multitude of alterations in cells, such as decreased solute and nutrient solubility, reduced diffusion rates, increased osmotic stress, and desiccation. In addition, the formation of ice crystals can cause cell damage. An increase in solvent viscosity and gas solubility and thus higher solubility of oxygen and reactive oxygen species (ROS) are also typical in cold habitats (Collins and Margesin, 2019). In this way, continuous low temperatures are totally connected with oxidative stress conditions and cold-adapted organisms have probably also developed adaptation mechanisms for them.
According to our experiments, eight of the twenty cold-tolerant clones isolated in the Antarctic metagenomic libraries also showed a considerably resistance to UV-B radiation exposure, involving ten of the genes contained in these clones [pC2 orf2, pC3 (orf1 and orf2 acting together), pC6 (orf1 and orf2 acting together), pC9 orf2, pC13, pD5, pD6 and pD7 orf2] (Figure 6). Five of these genes encode hypothetical (pC3 orf1, pC6 orf2, pC13, pD5) or unknown (pC9 orf2) proteins with an undefined function, but in many of them, a DNA-binding domain was predicted with at least one of the two bioinformatic programs used in this work (Table 1). Therefore, they could be involved in the protection or repair of DNA damage, normally caused by UV-radiation (Lamprecht-Grandío et al., 2020). The other five cold-tolerant genes that also conferred UV-resistance are similar to previously characterized genes. In fact, four of them (pC2 orf2, pC3 orf2, pD6, and pD7 orf2) have been related to genetic information processing, so they could be also connected to UV protection since they encode proteins normally induced under different stress conditions, favoring translation initiation, ribosome biogenesis, gene expression regulation, stabilization/degradation of mRNA molecules, or proper protein folding (Table 1; Figure 6). Finally, the last UV-resistance gene encodes a murein L,D-transpeptidase that participates in the biosynthesis of the peptidoglycan layer, a physical barrier that can also protect cells from UV-radiation as an indirect mechanism (Liu et al., 2010).
Conclusion
The isolation of 29 novel cold-tolerant genes in this work supports the use of a functional metagenomic approach to characterize new genes related to extreme temperature tolerance mechanisms. This work has revealed a wide variety of processes that could be related to cold-tolerance, such as different metabolic reactions, transport and membrane processes, or genetic information processing. Moreover, some of the proteins encoded by the novel cold-tolerant genes described in this work are conserved hypothetical or unknown proteins that have not previously been related to cold tolerance. Further characterization of the identified genes will improve understanding of the molecular mechanisms and metabolic pathways involved in low temperature acclimatization. On the other hand, the genes discovered in this work could be used in different biotechnological applications that require low temperatures.
Data availability statement
The datasets presented in this study can be found in online repositories. The names of the repository/repositories and accession number(s) can be found at: https://www.ncbi.nlm.nih.gov/genbank/, MZ835316-MZ835335.
Author contributions
PF and JG-P designed the experiments and wrote the manuscript. VM participated in the revision of the manuscript and constructed the metagenomic library. VM and JG-P collected the environmental samples. PF performed the screening, the bioinformatic analysis, and the different tests of the tolerant clones. All authors contributed to the article and approved the submitted version.
Funding
PF was supported by a postdoctoral fellowship from the Consejería de Educación, Juventud y Deporte, Comunidad de Madrid, European Social Fund and Youth Employment Initiative (YEI) (PEJD-2017-POST/BIO-4333). VM was supported by a postdoctoral fellowship granted by Becas Chile 2010 Program, from the Chilean government. This research was supported by: (i) grants PGC2018-096956-B-C42, CTM2009-08648-E/ANT, and CTM2011-14777-E/ANT by the Spanish Ministry of Science and Innovation/State Agency of Research MCIN/AEI/10.13039/501100011033 and by “ERDF A way of making Europe,” and (ii) the European Commission, Horizon 2020 Framework Programme, Call: H2020-LEIT-BIO-2015-1, Project: METAFLUIDICS, GA 685474.
Acknowledgments
We thank Carolina González de Figueras from the Centro de Astrobiología (CAB), for technical assistance, and also to Angeles Aguilera Bazán (CAB), PI of the Antarctic projects, for organizing the campaign and her support.
Conflict of interest
The authors declare that the research was conducted in the absence of any commercial or financial relationships that could be construed as a potential conflict of interest.
Publisher’s note
All claims expressed in this article are solely those of the authors and do not necessarily represent those of their affiliated organizations, or those of the publisher, the editors and the reviewers. Any product that may be evaluated in this article, or claim that may be made by its manufacturer, is not guaranteed or endorsed by the publisher.
Supplementary material
The Supplementary material for this article can be found online at: https://www.frontiersin.org/articles/10.3389/fmicb.2022.1026463/full#supplementary-material
Footnotes
1. ^https://www.ncbi.nlm.nih.gov/orffinder/
2. ^https://www.ebi.ac.uk/Tools/hmmer/results/95FCB862-EF40-11E9-932A%20ACDA53F04F9B/score
3. ^http://77.68.43.135:8080/DPP-PseAAC/
References
Atkinson, J. T., Campbell, I., Bennett, G. N., and Silberg, J. J. (2016). Cellular assays for ferredoxins: a strategy to understand electron flow through protein carriers that link metabolic pathways. Biochemistry 55, 7047–7064. doi: 10.1021/acs.biochem.6b00831
Awano, N., Xu, C., Ke, H., Inoue, K., Inouye, M., and Phadtare, S. (2007). Complementation analysis of the cold-sensitive phenotype of the Escherichia coli csdA deletion strain. J. Bacteriol. 189, 5808–5815. doi: 10.1128/JB.00655-07
Baldridge, K. C., and Contreras, L. M. (2013). Functional implications of ribosomal RNA methylation in response to environmental stress. Crit. Rev. Biochem. Mol. Biol. 49, 69–89. doi: 10.3109/10409238.2013.859229
Baranyi, J., and Roberts, T. A. (1994). A dynamic approach to predicting bacterial growth in food. Int. J. Food Microbiol. 23, 277–294. doi: 10.1016/0168-1605(94)90157-0
Berg, K., Leiros, I., and Williamson, A. (2019). Temperature adaptation of DNA ligases from psychrophilic organisms. Extremophiles 23, 305–317. doi: 10.1007/s00792-019-01082-y
Bocian, A., Zwierzykowski, Z., Rapacz, M., Koczyk, G., Ciesiołka, D., and Kosmala, A. (2015). Metabolite profiling during cold acclimation of Lolium perenne genotypes distinct in the level of frost tolerance. J. Appl. Genet. 56, 439–449. doi: 10.1007/s13353-015-0293-6
Caruso, C., Rizzo, C., Mangano, S., Poli, A., Di Donato, P., Finore, I., et al. (2018). Production and biotechnological potential of extracellular polymeric substances from sponge-associated Antarctic bacteria. Appl. Environ. Microbiol. 84, e01624–e01617. doi: 10.1128/AEM.01624-17
Chen, S. Y., Jane, W. N., Chen, Y. S., and Wong, H. C. (2009). Morphological changes of Vibrio parahaemolyticus under cold starvation stresses. Int. J. Food Microbiol. 129, 157–165. doi: 10.1016/j.ijfoodmicro.2008.11.009
Cho, C. W., Lee, H. J., Chung, E., Kim, K. M., Heo, J. E., Kim, J. I., et al. (2007). Molecular characterization of the soybean L-asparaginase gene induced by low temperature stress. Mol. Cells 23, 280–286.
Choi, J. Y., Kim, S. C., and Lee, P. C. (2020). Comparative genome analysis of Psychrobacillus strain PB01, isolated from an iceberg. J. Microbiol. Biotechnol. 30, 237–243. doi: 10.4014/jmb.1909.09008
Chow, K. C., and Tung, W. L. (1998). Overexpression of dnaK/dnaJ and groEL confers freeze tolerance to Escherichia coli. Biochem. Biophys. Res. Commun. 253, 502–505. doi: 10.1006/bbrc.1998.9766
Collins, T., and Margesin, R. (2019). Psychrophilic lifestyles: mechanisms of adaptation and biotechnological tools. Appl. Microbiol. Biotechnol. 103, 2857–2871. doi: 10.1007/s00253-019-09659-5
Culligan, E. P., Sleator, R. D., Marchesi, J. R., and Hill, C. (2012). Functional metagenomics reveals novel salt tolerance loci from the human gut microbiome. ISME J. 6, 1916–1925. doi: 10.1038/ismej.2012.38
Culligan, E. P., Sleator, R. D., Marchesi, J. R., and Hill, C. (2013). Functional environmental screening of a metagenomic library identifies stlA; a unique salt tolerance locus from the human gut microbiome. PLoS One 8:e82985. doi: 10.1371/journal.pone.0082985
Cvetkovska, M., Szyszka-Mroz, B., Possmayer, M., Pittock, P., Lajoie, G., Smith, D. R., et al. (2018). Characterization of photosynthetic ferredoxin from the Antarctic alga Chlamydomonas sp. UWO241 reveals novel features of cold adaptation. New Phytol. 219, 588–604. doi: 10.1111/nph.15194
De Maayer, P., Anderson, D., Cary, C., and Cowan, D. A. (2014). Some like it cold: understanding the survival strategies of psychrophiles. EMBO Rep. 15, 508–517. doi: 10.1002/embr.201338170
Dhaulaniya, A. S., Balan, B., Kumar, M., Agrawal, P. K., and Singh, D. K. (2019). Cold survival strategies for bacteria, recent advancement and potential industrial applications. Arch. Microbiol. 201, 1–16. doi: 10.1007/s00203-018-1602-3
Díaz-Rullo, J., Rodríguez-Valdecantos, G., Torres-Rojas, F., Cid, L., Vargas, I. T., González, B., et al. (2021). Mining for perchlorate resistance genes in microorganisms from sediments of a hypersaline pond in Atacama Desert. Chile. Front Microbiol 12:723874. doi: 10.3389/fmicb.2021.723874
Dower, W. J., Miller, J. F., and Ragsdale, C. W. (1988). High efficiency transformation of E. coli by high voltage electroporation. Nucleic Acids Res. 16, 6127–6145. doi: 10.1093/nar/16.13.6127
Duarte, A. W. F., Dos Santos, J., Vianna, M. V., Vieira, J. M. F., Mallagutti, V. H., Inforsato, F. J., et al. (2018). Cold-adapted enzymes produced by fungi from terrestrial and marine Antarctic environments. Crit. Rev. Biotechnol. 38, 600–619. doi: 10.1080/07388551.2017.1379468
Favrot, L., Blanchard, J. S., and Vergnolle, O. (2016). Bacterial GCN5-related N-acetyltransferases: from resistance to regulation. Biochemistry 55, 989–1002. doi: 10.1021/acs.biochem.5b01269
Fonseca, F., Meneghel, J., Cenard, S., Passot, S., and Morris, G. J. (2016). Determination of intracellular vitrification temperatures for unicellular microorganisms under conditions relevant for cryopreservation. PLoS One 11:e0152939. doi: 10.1371/journal.pone.0152939
Georlette, D., Blaise, V., Collins, T., D’Amico, S., Grantia, E., Hoyoux, A., et al. (2004). Some like it cold: biocatalysis at low temperatures. FEMS Microbiol. Rev. 28, 25–42. doi: 10.1016/j.femsre.2003.07.003
González-Pastor, J. E., and Mirete, S. (2010). “Novel metal resistance genes from microorganisms: a functional metagenomic approach,” in Molecular Methods in Metagenomics. eds. R. Daniely and W. Streit (Berlin: Springer Verlag).
Goordial, J., Raymond-Bouchard, I., Zolotarov, Y., de Bethencourt, L., Ronholm, J., Shapiro, N., et al. (2016). Cold adaptive traits revealed by comparative genomic analysis of the eurypsychrophile Rhodococcus sp. JG3 isolated from high elevation McMurdo Dry Valley permafrost, Antarctica. FEMS Microbiol Ecol 92:fiv154. doi: 10.1093/femsec/fiv154
Guazzaroni, M. E., Morgante, V., Mirete, S., and González-Pastor, J. E. (2013). Novel acid resistance genes from the metagenome of the Tinto River, an extremely acidic environment. Environ. Microbiol. 15, 1088–1102. doi: 10.1111/1462-2920.12021
Gurung, N., Ray, S., Bose, S., and Rai, V. (2013). A broader view: microbial enzymes and their relevance in industries, medicine, and beyond. Biomed. Res. Int. 2013:329121. doi: 10.1155/2013/329121
Hála, M., and Žárský, V. (2019). Protein Prenylation in plant stress responses. Molecules 24:E3906. doi: 10.3390/molecules24213906
Kahlke, T., and Thorvaldsen, S. (2012). Molecular characterization of cold adaptation of membrane proteins in the Vibrionaceae core-genome. PLoS One 7:e51761. doi: 10.1371/journal.pone.0051761
Kapardar, R. K., Ranjan, R., Grover, A., Puri, M., and Sharma, R. (2010). Identification and characterization of genes conferring salt tolerance to Escherichia coli from pond water metagenome. Bioresour. Technol. 101, 3917–3924. doi: 10.1016/j.biortech.2010.01.017
Kitamoto, D., Yanagishita, H., Endo, A., Nakaiwa, M., Nakane, T., and Akiya, T. (2001). Remarkable anti-agglomeration effect of a yeast biosurfactant, diacylmannosylerythritol, on ice-water slurry for cold thermal storage. Biotechnol. Prog. 17, 362–365. doi: 10.1016/j.biortech.2010.01.017
Koh, H. Y., Park, H., Lee, J. H., Han, S. J., Sohn, Y. C., and Lee, S. G. (2017). Proteomic and transcriptomic investigations on cold-responsive properties of the psychrophilic Antarctic bacterium Psychrobacter sp. PAMC 21119 at subzero temperatures. Environ. Microbiol. 19, 628–644. doi: 10.1111/1462-2920.13578
Lamprecht-Grandío, M., Cortesão, M., Mirete, S., de la Cámara, M. B., de Figueras, C. G., Pérez-Pantoja, D., et al. (2020). Novel genes involved in resistance to both ultraviolet radiation and perchlorate from the metagenomes of hypersaline environments. Front. Microbiol. 11:453. doi: 10.3389/fmicb.2020.00453
Lelivelt, M. J., and Kawula, T. H. (1995). Hsc66, an Hsp70 homolog in Escherichia coli, is induced by cold shock but not by heat shock. J. Bacteriol. 177, 4900–4907. doi: 10.1128/jb.177.17.4900-4907.1995
Lesnyak, D. V., Osipiuk, J., Skarina, T., Sergiev, P. V., Bogdanov, A. A., Edwards, A., et al. (2007). Methyltransferase that modifies guanine 966 of the 16S rRNA: functional identification and tertiary structure. J. Biol. Chem. 282, 5880–5887. doi: 10.1074/jbc.M608214200
Lim, J., Thomas, T., and Cavicchioli, R. (2000). Low temperature regulated DEAD-box RNA helicase from the Antarctic archaeon, Methanococcoides burtonii. J. Mol. Biol. 297, 553–567. doi: 10.1006/jmbi.2000.3585
Liu, P., Duan, W., Wang, Q., and Li, X. (2010). The damage of outer membrane of Escherichia coli in the presence of TiO2 combined with UV light. Colloids Surf. B Biointerfaces 78, 171–176. doi: 10.1016/j.colsurfb.2010.02.024
Liu, R., and Hu, J. (2013). DNABind: a hybrid algorithm for structure-based prediction of DNA-binding residues by combining machine learning-and template-based approaches. Proteins 81, 1885–1899. doi: 10.1002/prot.24330
Liu, S., Ma, Y., Zheng, Y., Zhao, W., Zhao, X., Luo, T., et al. (2020). Cold-stress response of probiotic lactobacillus plantarum K25 by iTRAQ proteomic analysis. J. Microbiol. Biotechnol. 30, 187–195. doi: 10.4014/jmb.1909.09021
Lorv, J. S., Rose, D. R., and Glick, B. R. (2014). Bacterial ice crystal controlling proteins. Scientifica 2014:976895. doi: 10.1155/2014/976895
Maiangwa, J., Ali, M. S. M., Salleh, A. B., Rahman, R. N. Z. R. A., Shariff, F. M., and Leow, T. C. (2015). Adaptational properties and applications of cold-active lipases from psychrophilic bacteria. Extremophiles 19, 235–247. doi: 10.1007/s00792-014-0710-5
Maillot, N. J., Honoré, F. A., Byrne, D., Méjean, V., and Genest, O. (2019). Cold adaptation in the environmental bacterium Shewanella oneidensis is controlled by a J-domain co-chaperone protein network. Commun Biol 2:323. doi: 10.1038/s42003-019-0567-3
McClain, W. H. (1993). Rules that govern tRNA identity in protein synthesis. J. Mol. Biol. 234, 257–280. doi: 10.1006/jmbi.1993.1582
Methé, B. A., Nelson, K. E., Deming, J. W., Momen, B., Melamud, E., Zhang, X., et al. (2005). The psychrophilic lifestyle as revealed by the genome sequence of Colwellia psychrerythraea 34H through genomic and proteomic analyses. Proc. Natl. Acad. Sci. U. S. A. 102, 10913–10918. doi: 10.1073/pnas.0504766102
Mihoub, F., Mistou, M. Y., Guillot, A., Leveau, J. Y., Boubetra, A., and Billaux, F. (2003). Cold adaptation of Escherichia coli: microbiological and proteomic approaches. Int. J. Food Microbiol. 89, 171–184. doi: 10.1016/s0168-1605(03)00119-3
Mirete, S., De Figueras, C. G., and González-Pastor, J. E. (2007). Novel nickel resistance genes from the rhizosphere metagenome of plants adapted to acid mine drainage. Appl. Environ. Microbiol. 73, 6001–6011. doi: 10.1128/AEM.00048-07
Mirete, S., Mora-Ruiz, M. R., Lamprecht-Grandío, M., de Figueras, C. G., Rosselló-Móra, R., and González-Pastor, J. E. (2015). Salt resistance genes revealed by functional metagenomics from brines and moderate-salinity rhizosphere within a hypersaline environment. Front. Microbiol. 6:1121. doi: 10.3389/fmicb.2015.01121
Molla, G., Nardini, M., Motta, P., D’Arrigo, P., Panzeri, W., and Pollegioni, L. (2014). Aminoacetone oxidase from streptococcus oligofermentans belongs to a new three-domain family of bacterial flavoproteins. Biochem. J. 464, 387–399. doi: 10.1042/BJ20140972
Morgante, V., Mirete, S., de Figueras, C. G., Postigo-Cacho, M., and González-Pastor, J. E. (2014). Exploring the diversity of arsenic resistance genes from acid mine drainage microorganisms. Environ. Microbiol. 17, 1910–1925. doi: 10.1111/1462-2920.12505
Mykytczuk, N. C., Foote, S. J., Omelon, C. R., Southam, G., Greer, C. W., and Whyte, L. G. (2013). Bacterial growth at-15°C: molecular insights from the permafrost bacterium Planococcus halocryophilus Or1. ISME J. 7, 1211–1226. doi: 10.1038/ismej.2013.8
Mykytczuk, N. C., Trevors, J. T., Foote, S. J., Leduc, L. G., Ferroni, G. D., and Twine, S. M. (2011). Proteomic insights into cold adaptation of psychrotrophic and mesophilic Acidithiobacillus ferrooxidans strains. Anthonie van Leewenhoek 100, 259–277. doi: 10.1007/s10482-011-9584-z
Nigam, P. S. (2013). Microbial enzymes with special characteristics for biotechnological applications. Biomol. Ther. 3, 597–611. doi: 10.3390/biom3030597
Nogales, J., Macchi, R., Franchi, F., Barzaghi, D., Fernández, C., García, J. L., et al. (2007). Characterization of the last step of the aerobic phenylacetic acid degradation pathway. Microbiology 153, 357–365. doi: 10.1099/mic.0.2006/002444-0
Pérez-Pantoja, D., González, B., and Pieper, D. H. (2010). “Aerobic degradation of aromatic hydrocarbons,” in Handbook of Hydrocarbon and Lipid Microbiology. ed. K. N. Timmis (Berlin, Heidelberg: Springer-Verlag), 799–837.
Perfumo, A., Banat, I. M., and Marchant, R. (2018). Going green and cold: biosurfactants from low-temperature environments to biotechnology applications. Trends Biotechnol. 36, 277–289. doi: 10.1016/j.tibtech.2017.10.016
Phadtare, S. (2011). Unwinding activity of cold shock proteins and RNA metabolism. RNA Biol. 8, 394–397. doi: 10.4161/rna.8.3.14823
Phadtare, S. (2012). Escherichia coli cold-shock gene profiles in response to overexpression/deletion of CsdA, RNase R and PNPase and relevance to low-temperature RNA metabolism. Genes Cells 17, 850–874. doi: 10.1111/gtc.12002
Phadtare, S., and Inouye, M. (2004). Genome-wide transcriptional analysis of the cold shock response in wild-type and cold-sensitive, quadruple-csp-deletion strains of Escherichia coli. J. Bacteriol. 186, 7007–7014. doi: 10.1128/JB.186.20.7007-7014.2004
Pittera, J., Jouhet, J., Breton, S., Garczarek, L., Partensky, F., Maréchal, É., et al. (2018). Thermoacclimation and genome adaptation of the membrane lipidome in marine Synechococcus. Environ. Microbiol. 20, 612–631. doi: 10.1111/1462-2920.13985
Rahman, M. S., Shatabda, S., Saha, S., Kaykobad, M., and Rahman, M. S. (2018). DPP-PseAAC: a DNA-binding protein prediction model using Chou's general PseAAC. J. Theor. Biol. 452, 22–34. doi: 10.1016/j.jtbi.2018.05.006
Raymond-Bouchard, I., Chourey, K., Altshuler, I., Iyer, R., Hettich, R. L., and Whyte, L. G. (2017). Mechanisms of subzero growth in the cryophile Planococcus halocryophilus determined through proteomic analysis. Environ. Microbiol. 19, 4460–4479. doi: 10.1111/1462-2920.13893
Rodrigues, D., Ivanova, N., He, Z., Huebner, M., Zhou, J., and Tiedje, J. M. (2008). Architecture of thermal adaptation in an Exiguobacterium sibiricum strain isolated from 3 million year old permafrost: a genome and transcriptome approach. BMC Genomics 9:547. doi: 10.1186/1471-2164-9-547
Rubio, S., Whitehead, L., Larson, T. R., Graham, I. A., and Rodríguez, P. L. (2008). The coenzyme a biosynthetic enzyme phosphopantetheine adenylyltransferase plays a crucial role in plant growth, salt/osmotic stress resistance, and seed lipid storage. Plant Physiol. 148, 546–556. doi: 10.1104/pp.108.124057
Ruisi, S., Barreca, D., Selbmann, L., Zucconi, L., and Onofri, S. (2007). Fungi in Antartica. Rev. Environ. Sci. Biotechnol. 6, 127–141. doi: 10.1007/s11157-006-9107-y
Sergiev, P. V., Bogdanov, A. A., and Dontsova, O. A. (2007). Ribosomal RNA guanine-(N2)-methyltransferases and their targets. Nucleic Acids Res. 35, 2295–2301. doi: 10.1093/nar/gkm104
Sindhu, R., Binod, P., Madhavan, A., Beevi, U. S., Mathew, A. K., Abraham, A., et al. (2017). Molecular improvements in microbial α-amylases for enhanced stability and catalytic efficiency. Bioresour. Technol. 245, 1740–1748. doi: 10.1016/j.biortech.2017.04.098
Stetina, T., Koštál, V., and Korbelová, J. (2015). The role of inducible Hsp70, and other heat shock proteins, in adaptive complex of cold tolerance of the fruit fly (Drosophila melanogaster). PLoS One 10:e0128976. doi: 10.1371/journal.pone.0128976
Sundareswaran, V. R., Singh, A. K., Dube, S., and Shivaji, S. (2010). Aspartate aminotransferase is involved in cold adaptation in psychrophilic Pseudomonas syringae. Arch. Microbiol. 192, 663–672. doi: 10.1007/s00203-010-0591-7
Swigonová, Z., Mohsen, A. W., and Vockley, J. (2009). Acyl-CoA dehydrogenases: dynamic history of protein family evolution. J. Mol. Evol. 69, 176–193. doi: 10.1007/s00239-009-9263-0
Tao, K. L., and Khan, A. A. (1974). Increases in activities of aminoacyl-tRNA synthetases during cold-treatment of dormant pear embryo. Biochem. Biophys. Res. Commun. 59, 764–770. doi: 10.1016/s0006-291x(74)80045-8
Ting, L., Williams, T. J., Cowley, M. J., Lauro, F. M., Guilhaus, M., Raftery, M. J., et al. (2010). Cold adaptation in the marine bacterium, Sphingopyxis alaskensis, assessed using quantitative proteomics. Environ. Microbiol. 12, 2658–2676. doi: 10.1111/j.1462-2920.2010.02235.x
Tressel, T., Thompson, R., Zieske, L. R., Menendez, M. I., and Davis, L. (1986). Interaction between L-threonine dehydrogenase and aminoacetone synthetase and mechanism of aminoacetone production. J. Biol. Chem. 261, 16428–16437. doi: 10.1016/S0021-9258(18)66584-6
Tribelli, P. M., and Lopez, N. I. (2018). Reporting key features in cold-adapted bacteria. Life (Basel) 8:8. doi: 10.3390/life8010008
Tribelli, P. M., Venero, E. C. S., Ricardi, M. M., Gómez-Lozano, M., Iustman, L. J. R., Molin, S., et al. (2015). Novel essential role of ethanol oxidation genes at low temperature revealed by transcriptome analysis in the Antarctic bacterium pseudomonas extremaustralis. PLoS One 10:e0145353. doi: 10.1371/journal.pone.0145353
Vaillancourt, F. H., Bolin, J. T., and Eltis, L. D. (2004). “Ring-cleavage dioxygenases,” in Pseudomonas. ed. J. L. Ramos (New York, NY: Kluwer Academic/Plenum Publishers), 359–395.
Vidovic, S., Mangalappalli-Illathu, A. K., and Korber, D. R. (2011). Prolonged cold stress response of Escherichia coli O157 and the role of rpoS. Int. J. Food Microbiol. 146, 163–169. doi: 10.1016/j.ijfoodmicro.2011.02.018
Voets, I. K. (2017). From ice-binding proteins to bio-inspired antifreeze materials. Soft Matter 13, 4808–4823. doi: 10.1039/c6sm02867e
Yamanaka, K., and Inouye, M. (2001). Selective mRNA degradation by polynucleotide phosphorylase in cold shock adaptation in Escherichia coli. J. Bacteriol. 183, 2808–2816. doi: 10.1128/JB.183.9.2808-2816.2001
Keywords: cold-tolerance, UV-resistance, oxidative stress, functional metagenomics, extreme environments, Antarctic
Citation: de Francisco Martínez P, Morgante V and González-Pastor JE (2022) Isolation of novel cold-tolerance genes from rhizosphere microorganisms of Antarctic plants by functional metagenomics. Front. Microbiol. 13:1026463. doi: 10.3389/fmicb.2022.1026463
Edited by:
Baolei Jia, Chung-Ang University, South KoreaReviewed by:
Deepak Sharma, Case Western Reserve University, United StatesRam Karan, King Abdullah University of Science and Technology, Saudi Arabia
Copyright © 2022 de Francisco Martínez, Morgante and González-Pastor. This is an open-access article distributed under the terms of the Creative Commons Attribution License (CC BY). The use, distribution or reproduction in other forums is permitted, provided the original author(s) and the copyright owner(s) are credited and that the original publication in this journal is cited, in accordance with accepted academic practice. No use, distribution or reproduction is permitted which does not comply with these terms.
*Correspondence: José Eduardo González-Pastor, Z29uemFsZXpwamVAY2FiLmludGEtY3NpYy5lcw==