- 1Biorepository and Tissue Research Facility, University of Virginia School of Medicine, Charlottesville, VA, United States
- 2Department of Microbiology, Biochemistry and Molecular Genetics, Rutgers New Jersey Medical School, Newark, NJ, United States
A wide range of protozoan pathogens either transmitted by vectors (Plasmodium, Babesia, Leishmania and Trypanosoma), by contaminated food or water (Entamoeba and Giardia), or by sexual contact (Trichomonas) invade various organs in the body and cause prominent human diseases, such as malaria, babesiosis, leishmaniasis, trypanosomiasis, diarrhea, and trichomoniasis. Humans are frequently exposed to multiple pathogens simultaneously, or sequentially in the high-incidence regions to result in co-infections. Consequently, synergistic or antagonistic pathogenic effects could occur between microbes that also influences overall host responses and severity of diseases. The co-infecting organisms can also follow independent trajectory. In either case, co-infections change host and pathogen metabolic microenvironments, compromise the host immune status, and affect microbial pathogenicity to influence tissue colonization. Immunomodulation by protozoa often adversely affects cellular and humoral immune responses against co-infecting bacterial pathogens and promotes bacterial persistence, and result in more severe disease symptoms. Although co-infections by protozoa and viruses also occur in humans, extensive studies are not yet conducted probably because of limited animal model systems available that can be used for both groups of pathogens. Immunosuppressive effects of protozoan infections can also attenuate vaccines efficacy, weaken immunological memory development, and thus attenuate protection against co-infecting pathogens. Due to increasing occurrence of parasitic infections, roles of acute to chronic protozoan infection on immunological changes need extensive investigations to improve understanding of the mechanistic details of specific immune responses alteration. In fact, this phenomenon should be seriously considered as one cause of breakthrough infections after vaccination against both bacterial and viral pathogens, and for the emergence of drug-resistant bacterial strains. Such studies would facilitate development and implementation of effective vaccination and treatment regimens to prevent or significantly reduce breakthrough infections.
Introduction
Co-infections of protozoa-bacteria-viruses are an emerging phenomenon due to overlapping epidemiological niches or shared transmission routes. Co-infections can adversely affect host immune responses, pathogenicity of microbes and success of chemotherapy and vaccinations. Co-infection of Plasmodium species with multiple bacterial species have been reported including with Mycobacterium (Chukwuanukwu et al., 2017), Salmonella (Cunnington et al., 2011), non-typhoid (NT) Salmonella (Takem et al., 2014), in addition to viruses such as HIV (Alemu et al., 2013), SARS-CoV-2 (Anyanwu, 2021), and hepatitis viruses (Helegbe et al., 2018). Such protozoan co-infections with bacterial and viral infections have high prevalence in same endemic regions especially in Sub-Saharan Africa. Malaria patients have been shown to be susceptible to other infections, influencing the pathogenesis and prognosis of the disease. Other interactions include Babesia-Borrelia (Dunn et al., 2014) as well as les common Entamoeba spp.-Escherichia coli have also been reported (Fernandez-Lopez et al., 2019). Alteration in the host, for instance, due to HIV infection that compromises host immune system can render humans more susceptible to co-infection by other opportunistic pathogens (Ayoade and Joel Chandranesan, 2022). Co-infecting pathogens may also have epidemiological implications and alter the mortality or morbidity due to diseases they cause (Anyanwu, 2021). Increase in DNA uptake and genetic recombination includes transfer of antimicrobial resistance genes between the co-infecting agents (Marti et al., 2017) resulting in emergence of multi-drug resistant pathogens. Apart from the effect on the pathogens, co-infections may also impact the efficacy of vaccines and success of chemotherapeutic agents.
Bacterial-protozoan co-infections are a commonly occurring phenomenon due to overlapping ecological niches and inadequate disease control infrastructures and thus, requires an all-inclusive approach to develop preventative vaccines and effective chemotherapeutic agents (Cox, 2001). Unexplained decline in efficacy of vaccines that are routinely used, and emergence of breakthrough infections is a concerning trend in disease endemic regions and poses a threat to control of infections around the world. The success of antimicrobials and vaccinations in diseases control depends heavily on a vibrant immune system. The immunodynamics of co-infections with protozoa need considered scrutiny during development of vaccines and antibiotics because the changes have the potential to lead to emergence of antigenic variations, breakthrough infections and antibiotic resistance (Wait et al., 2020).
The rise in immunocompromised individual numbers and accompanying vaccination failures has created a favorable microenvironment for emergence of more virulent pathogens (Laufer et al., 2007). This has further increased the urgency to investigate the causes of reduced effectiveness of vaccines against bacterial and viral pathogens to facilitate formulation of more inclusive and rational corrective measures. Inequitable resource distribution and marginalization of the developing world coupled with poor nutrition and disease control infrastructure complicates the control of global disease burden as more resistant strains emerge. These pathogens are then carried throughout the globe by traveling populations in now highly interconnected world (Walsh, 1989). This scenario creates an existential threat to disease control and a paradigm shift in approaches to vaccine and drug development. Therefore, it is critically important that acute to chronic protozoan infections and their presence during co-infections are considered seriously when more severe disease manifestations are noticed, or reduced vaccines effectiveness are observed.
Based upon a comprehensive review of literature, we have summarized the impact of protozoan infections on pathogenesis of co-infecting bacterial and viral pathogens (Figure 1). We have also depicted the consequence of acute to chronic parasitic infection on emergence of drug-resistance in co-infecting pathogens and influence of protozoa on vaccines efficacy that could affect protection from infectious bacteria and viruses.
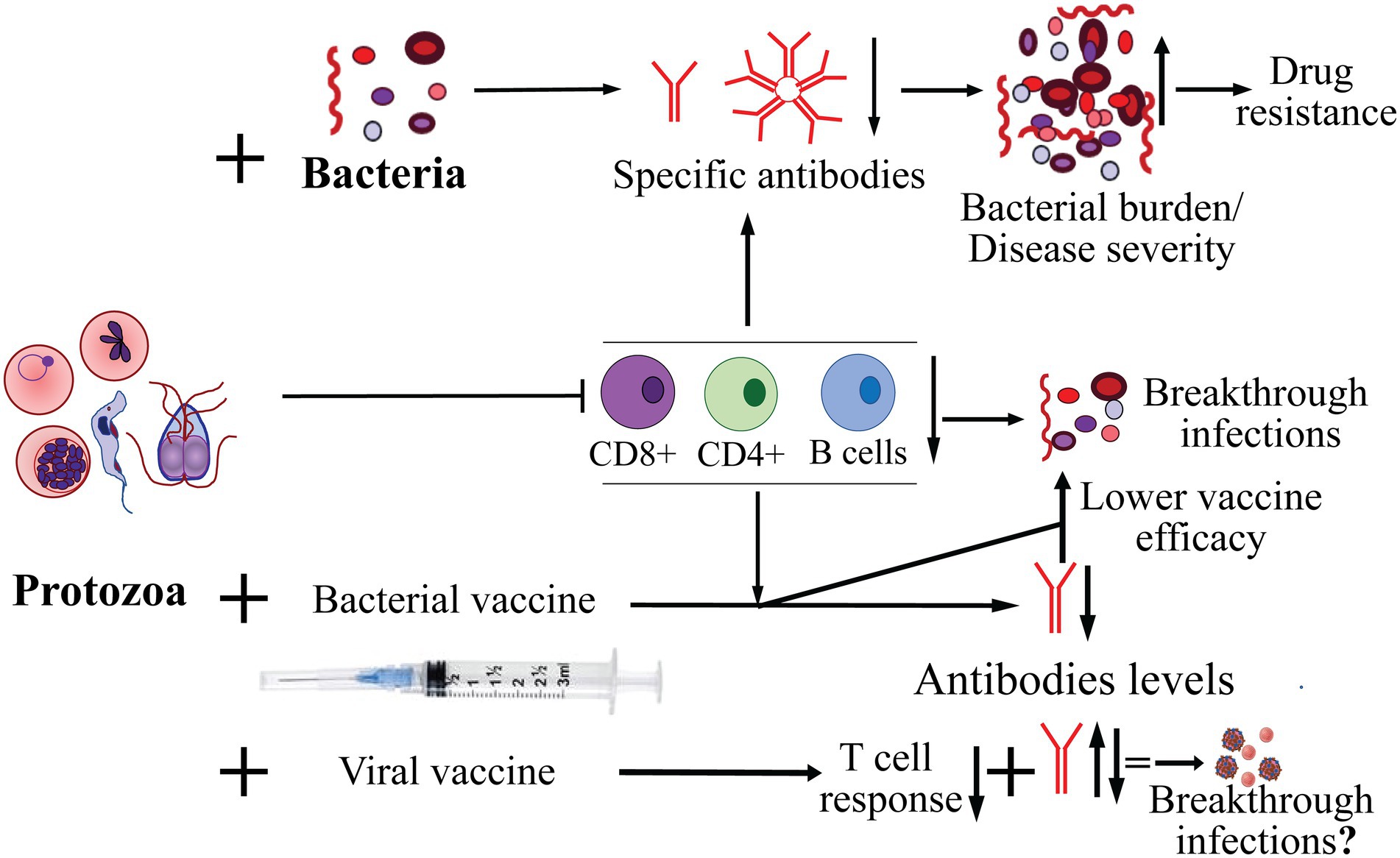
Figure 1. Effect of protozoan infection on adaptive immune response and co-infecting bacteria, and on effectiveness of bacterial and viral vaccines in protection from infection. The major pathogenic protozoa, such as Plasmodium, Babesia, Trichomonas and Trypanosoma, often cause suppression of adaptive immune response affecting both B and T cells. As a consequence, decrease in the specific antibody levels against co-infecting bacteria resulting in increase in burden of bacterial pathogens and exacerbation of severity of diseases they cause. Increased bacterial burden also enhances probability of emergence of resistance to antimicrobials. Diminished cellular and humoral immune response caused by acute to chronic infection by protozoa could also result in reduced efficacy/failure of vaccines against bacterial pathogens and may lead to breakthrough infections. Highly variable effects of protozoan infections with respect to antibody response to vaccines against different viral pathogens has been reported but their impact on breakthrough infections by the respective viruses remains to be investigated more thoroughly.
The epidemiology of protozoa-bacteria co-infections
Protozoa-bacteria co-infections are an emerging healthcare problem especially in the developing world due to geographical overlap between different pathogens. For example, the overlapping existence of Plasmodium spp. and Mycobacterium tuberculosis that cause malaria and tuberculosis (TB), respectively particularly in countries with poor healthcare infrastructure creates a perfect setting for co-infections (Page et al., 2005). Co-infections involving gastrointestinal pathogens also commonly occur in low-income countries with poor water-sewer infrastructure and hygienic environments and thus, allow a common pathway of transmission (fecal-oral) as reported for Campylobacter jejunum and intestinal protozoa (Bronowski et al., 2014). Co-infections with sexually transmittable pathogens also occur frequently in women with abnormal vaginal bacteriome. Bacterial vaginosis (BV), a common syndrome when quantity and quality of vaginal microbiota is perturbed, often involves Trichomonas vaginalis infection (Onderdonk et al., 2016). Clostridium perfringens and T. fetus are also a frequent occurrence in bacterial-protozoan vaginosis. Toxoplasma gondii infections have also been observed together with Clostridium perfringens during endometritis (Alsammani et al., 2012). The presence of infected companion feline hosts with susceptible humans serves as the most ardent predisposing factor for T. gondii infection cycle. For respiratory and gastrointestinal co-infections, other common predisposing conditions in humans is underlying immunocompromised status, such as during HIV infection/AIDS or the presence of other enteric pathogens like entero-viruses.
Common vector and reservoir host(s) harboring multiple pathogens have been observed frequently. Babesia species, which are protozoans transmitted by Ixodes scapularis ticks, are hemoparasites with life cycle and pathology similar to Plasmodium spp. and trigger comparable impact on mammalian hosts immune responses and show several overlapping diseases manifestations (Djokic et al., 2021). Lyme disease causing spirochetal bacteria belonging to Borrelia burgdorferi sensu lato are also transmitted to different hosts by ticks (Stewart and Bloom, 2020). Increasing rate of co-infections by these two pathogens have been reported in the endemic regions of the United States (Swanson et al., 2006; Diuk-Wasser et al., 2016; Wormser et al., 2019) and Europe (Olsthoorn et al., 2021). Thus, in addition to B. burgdorferi-Babesia co-infections, simultaneous Babesia-Anaplasma phagocytophilum infections are also reported because tick vector and white footed mouse, Peromyscus leucopus (and other reservoir hosts) harbor several pathogens together. While some protozoa have established a mutual relationship with bacteria in which each microbe benefits from the infected host, other examples may involve cases where the pathogen modulates the immune status to benefit itself at the expense of the host (Schmid-Hempel, 2009).
Protozoan infection and adaptive immune response
Following infection, protozoan parasites may either induce antibody, cell mediated immunity or stimulate both types of immune responses. These changes depend on the type of infection, localization of pathogen in the body, and the development stage of the organism. Various protozoan pathogens colonize different organs of the body, such as gastrointestinal tract (Amoeba), blood stream (Trypanosoma), within erythrocytes (Plasmodium and Babesia) and inside the macrophages (Leishmania spp., and T. gondii). Extracellular parasites generally induce and can often be controlled by antibody mediated killing by opsonophagocytosis while intracellular protozoan pathogens control requires cell mediated immune responses (Bretscher, 1992). Leishmania spp. are obligate intracellular protozoan, such that defense mechanisms against this parasite depend upon CD4+ T-lymphocytes stimulation that could also activate macrophages and induce Th1 cytokines production (Gupta et al., 2013). Some parasites can induce both humoral and cell mediated immunity depending on the developmental stage of the pathogen, for, e.g., in Plasmodium spp. (Beck et al., 1995). In addition, they may also deploy immune evasion mechanisms for survival in the hosts, such as escape from antibodies by changing their surface antigenic coats, i.e., antigen variation observed in Trypanosoma brucei (Márquez-Contreras, 2018). Trypanosoma brucei gambiense induces humoral immune response because of its extra-cellular existence; however, antigenic variation of the parasites is hallmark of its long-term persistence in the host because it enables the protozoan to evade the immune system-mediated elimination in many cases (Márquez-Contreras, 2018).
The pathogenesis of protozoan-bacterial co-infections
Protozoan-bacterial co-infections affects hosts in many ways. They can either lead to an antagonistic/deleterious or synergistic/advantageous pathogenic effect on the infected hosts. Antagonisms may be caused by resource competition or stimulation/suppression of innate or adaptive immune response that could negatively affect the co-infecting pathogen (Rigaud et al., 2010). During synergistic interactions, pathogens could suppress immune response of hosts resulting in high rates of replication of one or both pathogens. For example, protozoan infections can lead to apoptotic clearance of cells of the immune system creating a favorable environment for their own multiplication and potentially for proliferation of the co-infecting bacteria (Gigley et al., 2012). In addition, co-infecting pathogens can also modulate the gene expression in each other. Furthermore, synergism may also involve direct or indirect resources sharing, such that one microbe assists the co-infecting pathogen with regards to acquisition of nutrients (Birger et al., 2015). In this case, one pathogen may create favorable environment for colonization and growth of another pathogen, for, e.g., the presence of bacterial biofilm may promote proliferation of protozoa to stimulate synergism (Denoncourt et al., 2014). Alternatively, each pathogen takes on independent mechanisms of existence with minimal effect on diseases they cause. Several examples listed below demonstrate different effects of protozoan-bacterial co-infections on each other and on host(s).
Plasmodium and mycobacterium tuberculosis
Two major diseases, TB and malaria commonly occur together in patients due to overlapping geographical regions of infection for the two causative organisms (Baluku et al., 2019). Due to some similar initial subjective manifestations: flu-like illness, chills, fever, and fatigue, diagnostic approaches can miss some infections that leads to delayed therapeutic intervention. Thus, the lack of treatment for asymptomatic parasitic infection can apply selection pressure on TB by facilitating the emergence of drug-resistant strains (Murray et al., 2014). Interestingly, P. falciparum and P. vivax infections showed significant reduction in B and T (both CD4+ and CD8+) cells in patients (Kassa et al., 2006). M. tuberculosis infection has also been demonstrated to modulate the immune responses and confer immunological protection against severe malaria while weakened responses occur against bacteria. As a result, Plasmodium-M. tuberculosis co-infected patients show reduced/mild symptoms of malaria and a more severe symptoms of tuberculosis (Chukwuanukwu et al., 2017). In a murine model of infection, M. tuberculosis-induced potentiation of type 1 immune responses has been associated with protection against lethal malaria, which also appears to be related to induced production of IFN-γ and TNF-α in C57/BL6 mice (Page et al., 2005). Furthermore, mice sequentially infected with M. tuberculosis followed by P. yoelii were less capable in containing bacterial growth in lungs, spleen, and liver and resulted in increased mortality of mice (Scott et al., 2004). Also, increased M. tuberculosis burden were observed in lungs of mice co-infected with P. berghei (Mueller et al., 2014) or P. yoelii (Blank et al., 2016). While this co-existence exacerbates disease by M. tuberculosis, it is antagonistic to Plasmodium-induced illness. Interestingly, heat shock protein 70 (HSP70) from M. tuberculosis has been associated with the induction of a strong humoral and cellular response directed against P. falciparum (Page et al., 2005). During malaria, a marked increase in the production of the anti-inflammatory cytokines IL-10 and IL-4 (Chukwuanukwu et al., 2017) occurs and is thought to exacerbate TB pathology by reducing the protective Th1 bias and tilting immunity towards Th2 response. Additionally, co-infection with the non-lethal P. yoelii also resulted in more severe tuberculosis pathology with increased immune cells infiltration, and increased pro-and anti-inflammatory mediators, mainly IFN-γ, TNF-α, IL-6, IL-10, and IL-17. Moreover, higher TNF-α levels positively correlated with increased M. tuberculosis burden in lungs of co-infected mice (Blank et al., 2016).
Plasmodium and non-typhoid salmonella
Co-infections between Non-Typhoid Salmonella (NTS) and highly pathogenic Plasmodium species have also been reported (Mtove et al., 2011). Plasmodium infection causes extensive hemolysis and release of cellular heme, which can be toxic for organisms. The protozoan converts it to hemozoin, a novel non-DNA ligand for Toll-like receptor (TLR)9, which can be captured by cells of the reticuloendothelial system (RES) and can activate innate immune responses (Simoes et al., 2015). In response, mammalian host produces heme oxygenase-1 to degrade the heme and mitigate malaria pathology by limiting production of reactive oxygen species (ROS; Gozzelino et al., 2010); however, reduced ROS has counterproductive effect due to decrease in beneficial granulocyte oxidative burst function in clearing infections, and thus allows multiplication of co-infecting Salmonella in neutrophils (Cunnington et al., 2012; Harding et al., 2020). Activation of TLR9 also results in the production of pro-inflammatory cytokines, certain chemokines, and causes up-regulation of costimulatory molecules (Coban et al., 2005).
Plasmodium and other bacterial infections
Bacterial infections offer major complication during Plasmodium co-infections. During rodent P. yoelii infection, host immunity is impaired against diverse bacteria, including Streptococcus pneumoniae due to effects on innate immune response (Harding et al., 2020). A parasite-specific factor (haemozoin and bound bioactive molecules) directly contributes to Plasmodium-induced suppression of innate immune response against bacteria. P. yoelii infections also suppresses immune responses against Listeria monocytogenes by causing increased apoptosis of Listeria-specific T cells resulting in slower induction of cellular immune responses. Interaction between different strains of S. pneumoniae and the rodent malaria parasite P. chabaudi have also been shown to promote an antagonistic sequelae (Moens et al., 2012; Fairlie-Clarke et al., 2013). Studies have also shown that complement components, C1q and C3, interact with P. falciparum infected RBCs to initiate the complement cascade that leads to complement depletion (Nyakoe et al., 2009), which has been attributed to increased burden of S. pneumoniae during Plasmodium-S. pneumoniae co-infections (Harding et al., 2020).
Babesia spp. and Borrelia burgdorferi
Babesia-B. burgdorferi co-infections are a common occurrence due to shared reservoir host(s) and vector (Benach et al., 1985; Krause et al., 1996; Wormser et al., 2019). Studies in mice have demonstrated that when infected with B. burgdorferi, mice have been found to exhibit features similar to those of human Lyme disease (Barthold et al., 2010). During experimental co-infection of susceptible mice, B. microti infection causes splenomegaly and splenic dysfunction that results in a reduction in the levels of functional B and T cells. As a result, the production of specific antibodies against both pathogens are reduced causing poor control of B. burgdorferi infection (Djokic et al., 2019). Diminished adaptive immunity then exacerbates Lyme disease severity that is indicated by both; increased burden of B. burgdorferi in different organs of co-infected mice and more severe Lyme arthritis compared to those in mice infected with Lyme spirochetes alone (Parveen and Bhanot, 2019). These results agree with previous investigation showing that B. burgdorferi infection increased Lyme arthritis severity in co-infected Balb/c mice compared to singly infected mice (Moro et al., 2002). Limited human epidemiological studies have been conducted to determine outcomes of Babesia-Borrelia co-infection [reviewed (Knapp and Rice, 2015)] but overall, more diverse and persistent manifestations associated with B. burgdorferi infection were observed in co-infected patients (Krause et al., 1996). Thus, limited clinical studies have shown some overlapping features with those observed in mice; however, more thorough investigations are needed to fully determine the impact of co-infections on each disease severity.
Trypanosoma brucei and Brucella
During infection with T. brucei, phagocytosis of the protozoan has been found to be associated with an extensive production of cytokines. Cytokines IFN-γ and TNF-α were shown to be involved in exacerbation of anemia as mice lacking the respective genes exhibited protection from anemia, while anti-inflammatory cytokine, IL-10 counteracted the effects of Trypanosoma-induced anemia (Tabel et al., 2008; Musaya et al., 2015). Mice infected with T. brucei exhibit the characteristic parasitemia waves concurrently with the host expression of elevated levels of IFN-γ. Trypanosomes overcome host innate immune response and then cause significant immunosuppression allowing proliferation of this pathogen. The induction of pro-inflammatory IFN-γ by host in response to T. brucei infection has been shown to reduce splenic bacteria burdens in mice infected with either Brucella melitensis, B. abortus, or B. suis (Machelart et al., 2017). T. brucei-Brucella co-infection is therefore antagonistic for Brucella. In other cases, induction of IFN-γ is not sufficient to control selected bacterial infections, for, e.g., M. tuberculosis (Vilaplana et al., 2014; Musaya et al., 2015).
Trichomonas vaginalis, Mycoplasma hominis, Atopobium spp. and Gardnerella spp.
Trichomoniasis, a prevalent sexually transmitted infection (STI) is caused by the protozoan parasite T. vaginalis, which can establish a symbiotic relationship with M. hominis, a species implicated in bacterial vaginosis (Rappelli et al., 1998). M. hominis synergistically upregulates human monocytes pro-inflammatory response to T. vaginalis resulting in enhanced inflammation during trichomoniasis (Fiori et al., 2013). Due to influence of Trichomonas on change in vaginal pH, increase in infections in the urogenital tract also include other bacterial vaginosis associated bacteria. For example, co-existence of Atopobium and Gardnerella have also been found to cause synergistic enhancement of T. vaginalis induced production of chemokines (Onderdonk et al., 2016).
Protozoa-bacteria co-infection of gastrointestinal tract
Bacterial-protozoan co-infections also affect the gastrointestinal system with significant implications to the ensuing pathology. E. histolytica is a pathogenic protozoan related to intestinal and extraintestinal infections. In the large intestine, it co-exists with many resident microbiotas and results in asymptomatic infection or diarrhea (Stanley, 2003). The protozoan must compete with indigenous bacteria and may breach the mucus barrier. After binding to host cells, protozoan induces cell death, which causes amebic colitis and facilitates dissemination into extraintestinal organs. The interaction between E. histolytica and E. coli O55 causes substantial changes in their genes’ expression (Fernandez-Lopez et al., 2019). E. coli offers nutritional support for amoebic growth and helps the parasite to boost defenses against H2O2 induced oxidative stress to facilitate establishment of parasitic persistence in intestinal mucosa. As an example, oxaloacetate produced by E. coli protects E. histolytica against H2O2 induced oxidative stress while epithelial monolayers exposed to enteropathogenic bacteria are more susceptible to additional damage by E. histolytica. Phagocytosis of pathogenic/non-pathogenic bacteria promoted by amoebae further increased epithelial cells layer damage and exacerbated colitis severity (Galvan-Moroyoqui et al., 2008).
Murine studies have shown that Giardia intestinalis-enteroaggregative E. coli (EAEC) co-infection promotes bacterial growth impairment, microbiota-dependent delayed parasite clearance, microbial metabolic perturbations in the gut, and an alteration of localized host immune responses against EAEC (Bartelt et al., 2017). In contrast, G. muris reduces the symptoms of Citrobacter rodentium-induced colitis, by enhancing the production of mucosal antimicrobial peptides such as mouse β-defensin 3 and Trefoil factor 3 (Manko et al., 2017).
Helicobacter pylori (H. pylori) and Cryptosporidium spp. are well-known for their high prevalence in immunocompromised pediatric patients worldwide especially in developing countries (Ibrahim et al., 2019). H. pylori may support the colonization by Cryptosporidium spp. and vice versa. The interaction between H. pylori and intestinal parasites may have serious health consequences because Cryptosporidiosis results in increased intestinal permeability while H. pylori causes atrophic changes in the stomach. Together they may have a serious impact on tissue integrity and the balance of gut microbiome. Further investigation is warranted to unravel how this interaction affects the gut microbiome.
The epidemiology of protozoan parasite-viral co-infections
The interactions between viruses-protozoan co-infections and their complexities remain unexplored. These pathogens can reciprocally alter their epidemiology and/or host response to vaccines and therapies (Karp and Auwaerter, 2007). Virus-protozoan co-infections such as those caused by HIV and Plasmodium have been documented particularly in sub-Saharan Africa. Co-existence of these pathogens represents an emerging healthcare problem that has been causing significant morbidity and mortality, with more than 2 million deaths occurring annually (World Health Organization, 2017). Several other studies demonstrated that people living with HIV have more frequent and severe malaria manifestations (World Health Organization, 2015). HIV infected individuals are also at higher risk of exposure to leishmaniasis (Okwor and Uzonna, 2013; Diro et al., 2014) and have more efficient T. gondii infection with increased risks of deaths (Pott Jr. and Castelo, 2013). In addition, people with HIV have up to 21% more seroprevalences against amebiasis (Hung et al., 2012) and have significantly higher rates of trichomoniasis than HIV-negative individuals (36.4% vs. 21.3%) (Davis et al., 2016).
Other Plasmodium-viral co-infections are also frequently observed in sub-Saharan Africa. A study from Anastos et al., 2010 in Rwanda (East Africa) showed an association between Plasmodium infection and increases risk of cervical precancer in Human Papilloma Virus (HPV) infected patients (Anastos et al., 2010). In a recent study from Nigeria (West Africa), authors reported that Plasmodium infection coexists with Measles virus in 32.5% febrile children analyzed and they were under risk of serious consequences or even death (Aminu et al., 2021). Nigeria also has high rates of malaria-influenza co-existence among people refusing flu vaccinations. Influenza A and B were found in 54% of unvaccinated pregnant women having Plasmodium parasitemia (Anjorin and Nwammadu, 2020). Plasmodium spp. and hepatitis B Virus (HBV) infections are prone to co-exist in individuals living in the same regions. In a systematic review and meta-analysis conducted, 22 studies were analyzed and showed that overall co-infection prevalence between Plasmodium spp. and HBV is 6% worldwide with the highest prevalence rate (10%) in Gambia (Kotepui and Kotepui, 2020). Strong positive association was also found between seropositivity for Plasmodium and Ebola virus in residents from Gabon, Central Africa with co-infection prevalence of 10.2% (Abbate et al., 2020).
Cryptosporidium is one of the most important parasitic diarrheal agents affecting children in the developing countries (Tamomh et al., 2021). C. hominis, C. parvum and C. meleagridis have been implicated in diarrhoea. This protozoan has also emerged as a global opportunistic threat causing severe diarrhea (Ahmadpour et al., 2020). Cryptosporidium infection is common among HIV/AIDS patients (prevalence of 8,69%) worsening the protozoan infection associated symptoms causing severe diarrhea and eventually death because of low CD4+ T-cells counts (Wang et al., 2013; Fregonesi et al., 2015; Hailu et al., 2015; Yang et al., 2017; Wang et al., 2018). As a consequence of such co-infection and severe disease (Fregonesi et al., 2015; Alemu et al., 2018) parasitemia as high as 90% was observed (Ojurongbe et al., 2011).
Due to more recent emergence of infection by severe acute respiratory syndrome coronavirus 2 (SAR-CoV2) in humans, co-infections with different protozoan are not fully explored yet. Moreover, most of the available reports about SARS-CoV2 co-infections describe concomitant bacteria, fungus and other viral infections, especially associated with respiratory infections and pneumonia. In depth studies have not been conducted for SARS-CoV2 and protozoan co-infections; however a few reports are available showing co-infections occur with Toxoplasma (Montazeri et al., 2022), Plasmodium (Raham, 2021; Boonyarangka et al., 2022), Babesia (Jacobs and Siddon, 2021), Leishmania (Pikoulas et al., 2022) and Trypanosoma (Alberca et al., 2020). In the first year of the COVID-19 pandemic, worldwide malaria cases increased from 227 million in 2019 to 241 million in 2020 (World Health Organization, 2021) and a high rate of latent T. gondii infection was also found among COVID-19 patients with severe manifestations reported in the Middle East region (Montazeri et al., 2022). Therefore, more research is needed to fully understand the impact of SARS-CoV-2 infections on co-infecting protozoa, and vice versa.
The pathogenesis of protozoan-viral co-infections
Plasmodium and HIV
Due to the geographical overlap between Plasmodium and HIV, both pathogens can often infect humans with synergistic and adverse impact (Alemu et al., 2013). HIV infection increases the Plasmodium burden in patients, facilitating the increase in protozoan transmission. Conversely, infection with Plasmodium results in increase in number and activation state of CD4 + T cells, creating an ideal environment for HIV replication, increasing viremia. Other mechanisms of Plasmodium-infection induced HIV replication include the secretion of TNF-α that can act directly stimulate HIV replication (Ayouba et al., 2008). Additionally, infection with Plasmodium causes pro-inflammatory (T helper 1- type) immune response with activation of CD4+, CD4 + 5RO + T cells. These T cells are preferred target for HIV replication (Spina et al., 1997). CD14+ macrophages activated during acute malaria are also a source of migratory reservoirs of HIV-1 facilitating dissemination of virus to lymphocytes during cell–cell interactions promoting disease dissemination (Pantaleo and Koup, 2004). P. falciparum has also been shown to stimulate HIV-1 replication through the production of cytokines (IL-6 and TNF-α) that activate lymphocytes (Inion et al., 2003). Other studies have shown that exposure to soluble Plasmodium antigens and hemozoin induced HIV replication or reactivation via CD4 T-cell stimulation together with the production of pro-inflammatory cytokines, for, e.g., IL-1β, IL-6, and TNF-α (Froebel et al., 2004).
Co-infections with SARS-CoV2
It has been hypothesized that malaria may reduce the COVID-19 severity in endemic regions of sub-Saharan Africa (Gutman et al., 2020; Ssebambulidde et al., 2020; Osei et al., 2022). An inverse correlation between the incidence of COVID-19 and malaria with less probability of COVID-19 cases was found in malaria-endemic countries (Ssebambulidde et al., 2020). One possible explanation for this phenomenon is that malaria patients generate anti-GPI antibodies which eventually identify SARS-CoV-2 glycoproteins developing a protective response against COVID-19 improving the disease prognostic (Hussein et al., 2020). Conflicting results from a Malian longitudinal cohort study showed no association between malaria and COVID-19 seroconversion or effect on the symptoms reported for COVID-19 (Woodford et al., 2022). The identification of immunomodulatory effects provoked by malaria and helminth infections could lead us to better understanding of the factors involved in improvement of vaccine efficacy. It still remains unclear how efficacy of COVID-19 vaccines is affected by these parasites.
There are several reports showing association between Neglected Infectious Diseases (NTDs) and SARS-CoV2, in terms of how they affect the severity of COVID-19 clinical outcomes, vice versa and the development of trained immunity as occurs for helminth infections and malaria (Ssebambulidde et al., 2020; Anyanwu, 2021; Gluchowska et al., 2021; Wilairatana et al., 2021; Achan et al., 2022; Hussein et al., 2022). Our review of literature indicate that the immunomodulatory effects of COVID-19 and parasitic co-infections brought insights not by direct investigations but based upon lessons learned from other co-infections systems (Fonte et al., 2020; Gluchowska et al., 2021; Akelew et al., 2022; Woodford et al., 2022). Briefly, helminth co-infection was suggested to cause immunomodulation in COVID-19 patients to result in reduction of disease severity (Bradbury et al., 2020). This immunomodulation could be due parasite specific innate response and Th2 immune response with CD4+ T cells, eosinophils, and production of IL-4, IL-5, and IL-10, thereby reducing hyperinflammation in patients with severe COVID-19 (Rodriguez, 2020; Akelew et al., 2022). Reinforcing these observations, a recent study showed that patients co-infected with SARS-CoV2 and helminths had less severe COVID-19 due to reduced hyper-inflammation response (Wolday et al., 2021). In fact, an inverse correlation between COVID-19 existence and severity was observed in countries endemic for soil-transmitted helminths (Ssebambulidde et al., 2020).
Plasmodium and SARS-CoV-2
Plasmodium and SARS-CoV2 co-infections have been reported to occur across the endemic and non-endemic regions (Junaedi et al., 2020). Despite the rising incidence of COVID-19 disease in the world, an unremarkably lower prevalence has been observed in malaria endemic regions (Osei et al., 2022) suggesting that Plasmodium presence may offer some protection against SARS-CoV-2 infection. SARS-CoV-2 uses the angiotensin-converting enzyme 2 (ACE2) receptor to enter the host cells. However, a D-allele variant of ACEI/D polymorph has been described in a mild form of malaria. This ACE I/D polymorphism occurs in intron 16 reduces ACE2 expression. Reduced expression of ACE2 receptor in populations with this polymorphism may play a protective role against severe COVID-19. An increase of its substrate Ang II in plasma of these individuals have been demonstrated in people with African genetic background (Delanghe et al., 2020).
At the height of the COVID-19 outbreaks, relatively low prevalence rates were observed in areas known to have high malaria endemicity, prompting some interest on the role of malaria immunity in protecting COVID-19 infections (Vilaplana et al., 2014). Infections with Plasmodium induces both innate and adaptive immunity. Recent studies have shown that in addition to inducing adaptive B and T cells memory response, innate immune response to Plasmodium infection may also induce memory, a phenomenon known as trained immunity, which is capable of mounting a faster and more robust recall response and may provide cross protections against unrelated pathogens (Netea et al., 2011, 2020). Cross protection induced by trained immunity is a widely acknowledged phenomena and has been demonstrated by BCG vaccinations against M. tuberculosis because it provides cross protection against unrelated pathogens (Sohrabi et al., 2020). The major factors involved in innate immune response to malaria include natural killer (NK) cells, monocytes, macrophages, and pro- and anti-inflammatory cytokines (Hansen et al., 2007; Doolan et al., 2009). These responses can develop nonspecific trained immunity that can be effective against other pathogens like SARS-CoV-2, producing a faster and more effective protective response (Raham, 2021). Trained immunity against Plasmodium could also produce tolerance that tapers down the inflammatory response from innate immune cells, such as monocytes (Boutlis et al., 2006). Tolerance has the beneficial effect of reducing the harmful effect of excessive infection and disease (Nahrendorf et al., 2021), and cross-protection could occur by reduction of the inflammatory progression to unrelated disease, including SARS-CoV2. Such a response may explain the reduced COVID-19 severity in malaria community (Guha et al., 2020). Further research is needed to examine these concepts as relevant to COVID-19 in malaria endemic areas. For example, the innate immune factors (NK cells, Type 1 IFN, IgG) need to be evaluated in COVID-19 asymptomatic and symptomatic patients in malaria endemic areas.
Plasmodium and other viral co-infections
Co-infections by Plasmodium and hepatitis B and C (HBV and HCV) viruses often occur due to shared needles and blood transfusions etc. (World Health Statistics, 2022). A high incidence rate of malaria and hepatitis infection in the sub-Saharan population has been reported (Helegbe et al., 2018; Sevede et al., 2019). Co-infections with Plasmodium and hepatitis often results in changes in burdens of both pathogens such that individuals co-infected with Plasmodium spp. and HBV display lower parasitemia and higher viremia (Andrade et al., 2011). Both of these pathogens also have an antagonistic effect on anemia, while P. falciparum causes hemolytic anemia, HBV increases hemoglobin levels by releasing erythropoietin from regenerating hepatic tissues (Simon et al., 1982; Klassen and Spivak, 1990; Ifudu and Fowler, 2001). Conversely, during chronic HBV infection, cytokines released in response to P. falciparum infection could further activate the apoptosis of HBV-infected hepatocytes, and exacerbated liver damage as evidenced by the increase in pro-inflammatory cytokines, TNF-α, IL-1β, and IL-6 that are increased during Plasmodium and chronic HBV infection and reduction in anti-inflammatory cytokines IL-10 and IL-4 in pregnant women (Brown et al., 1992; Azizieh et al., 2018).
Historically, Ebola outbreaks have occurred in Western Africa, and it overlaps with malaria prevalence. Studies have shown that P. falciparum infection prior to infection with Ebola virus could induce an antiviral activity and a protective role against Ebola. Acute Plasmodium infection has been shown to promote IFN-γ-dependent resistance to Ebola virus infection (Rogers et al., 2020).
Outbreaks of measles have been reported in malaria endemic areas of sub-Saharan Africa generating some interest on the impact of one pathogen over the other albeit studies to-date are limited. One study has shown significantly lower parasitic prevalence and mean densities of malaria parasites were found in children up to 9 years of age who had measles or influenza than in asymptomatic control children (Rooth and Bjorkman, 1992).
Cryptosporidium and HIV
Pathogens belonging genus Cryptosporidium are transmitted by fecal-oral route causing gastrointestinal infection in various vertebrate species, including humans (Xiao et al., 2004; Wang et al., 2011) and has been associated to chronic to life-threatening diarrhea in immunocompromised individuals (Conner et al., 2019). Both innate and adaptive immune responses play a role in protection from cryptosporidiosis and resolution of infection; however, cell-mediated immunity is crucial for clearance of cryptosporidiosis (Borad and Ward, 2010). HIV/AIDS patients with lower CD4 counts are more susceptible to cryptosporidiosis and have greater severity of disease (Hunter and Nichols, 2002). In HIV/AIDS patients with active cryptosporidiosis, infected epithelial cells express high levels of the chemokine, CXCL10, and expression levels correlate with the parasite burden (Wang et al., 2007). Since CXCL10 increases the rate of HIV replication in vitro, elevated CXCL10 in cryptosporidiosis may contribute to enhanced destruction of CD4+ T cells due to HIV infection (Ahmadpour et al., 2020). Humoral immune responses have also been reported to play an important role in protection against Cryptosporidiosis since studies have suggested that that antibody responses to specific antigens were associated with protection from diarrhea in Cryptosporidium-infected HIV/AIDS patients (Ahmadpour et al., 2020). Specific serum IgG, IgM, and IgA production were evaluated in Cryptosporidium-HIV co-infection showing no difference among patients with or without diarrhea (Kaushik et al., 2009). The occurrence of diarrhea in HIV-positive individuals was not always observed during Cryptosporidium co-infections probably because antiretroviral therapy improved the immune system functionality (Irisarri-Gutierrez et al., 2017). Conversely, Cryptosporidium has been shown to stimulate periductal inflammation in the biliary tree, induces biliary epithelial cell apoptosis, and thus could contribute to the pathogenesis of AIDS-cholangiopathy (Chen and LaRusso, 2002).
Toxoplasma and HIV
Toxoplasma gondii, a coccidian protozoan obligate intracellular parasite, is the causative agent of toxoplasmosis which affects approximately 30% population worldwide. T. gondii infection results in malformation and life-threatening disease in developing fetuses and is one of the most prevalent causative agents of opportunistic infections in HIV/AIDS patients causing central nervous system toxoplasmosis (Montoya and Liesenfeld, 2004; Ayoade and Joel Chandranesan, 2022). According to a systematic review, toxoplasmosis co-infection prevalence in HIV patients varies greatly among different countries showing the highest numbers in Thailand (53.7%), North Sudan (75.0), Ethiopia (87.4%), Brazil (80.0%) and Iran (96.3%). These authors also observed that high prevalence of T. gondii-HIV co-infections in low-income countries (Wang et al., 2017). It has been shown that T. gondii-HIV co-infections lead to toxoplasmosis severity and increase mortality in patients who developed AIDS and were not properly treated (Da Cunha et al., 1994; Pott and Castelo, 2013; Agrawal et al., 2014). During HIV infection, depletion of CD4 cells, decreased production of cytokines and IFNγ, and impaired cytotoxic T-lymphocyte activity result in reactivation of latent Toxoplasma infection (Basavaraju, 2016), and is associated with persistence of IgG antibodies against T gondii (Robert-Gangneux and Darde, 2012). Additionally, low CD4 T lymphocyte count was associated with high frequency of encephalitis caused by toxoplasmosis in patients who developed AIDS (Lejeune et al., 2011). On the other hand, T. gondii co-infection also alters the immune response, clinical manifestation and transmission of the HIV infection (Welker et al., 1993; Bala et al., 1994). In individuals singly infected with HIV, for example, both numbers of plasmacytoid dendritic cells and IFN-α production are impaired (Feldman et al., 2001), which can be exacerbated during opportunistic Toxoplasma co-infection. Additionally, the exposure to T. gondii has been shown to potentiate CD4 positive T-cells and possibly monocytes to be more permissive for HIV replication (Subauste et al., 2004), suggesting that HIV/T. gondii co-infected individuals potentially exhibit more severe diseases.
Other protozoan-viral co-infections
Preliminary studies have shown that toxoplasmosis is a risk factor for acquiring SARS-CoV-2 infection and severe manifestations of COVID-19 (Fiori et al., 2013). T. gondii induces the shedding of mitochondrial outer membrane to promote its own growth. Intriguingly, the hijacking of host mitochondria has been shown to play a critical role in the pathogenesis of COVID-19 (Lo et al., 2014).
The relationship between HIV-1 infection and amoebiasis (Lowther et al., 2000; Hung et al., 2005, 2008) was demonstrated by the observation that HIV-infected men having sex with men (MSM) were at significantly higher risk of amebiasis than patients from other risk groups (Hung et al., 2008). One study has identified Amebic Liver Abscess (ALA) as the most common extraintestinal manifestation of invasive infection as an important condition in HIV-1-infected individuals and has been attributed to the ability of HIV-1 to suppress activity of regulatory T cells. This in turn suppresses E. histolytica-specific T-cell reactivity and cause increased susceptibility to invasive amebiasis in persons with early stage of HIV-1 infection (Hsieh et al., 2007).
Trichomoniasis is a highly prevalent STI among HIV-1-infected patients (Cu-Uvin et al., 2002). Previous investigation has demonstrated that T. vaginalis infection enhances HIV-1 transmission (Schwebke, 2005). Proposed mechanisms by which T. vaginalis infection may increase HIV-1 infection include: induction of inflammatory response in vaginal, exocervix, and urethral epithelia; disruption of mucosal barrier function; recruitment of CD4 lymphocytes and macrophages; development of microhemorrhages; degradation of secretory leukocyte protease inhibitors; and enhancement of susceptibility to bacterial vaginosis or other abnormal vaginal flora that all may increase the risk of HIV-1 acquisition (Sorvillo et al., 2001).
Rotavirus often contribute to the Cryptosporidium co- infection in farm animals and humans (Izzo et al., 2011; Mokomane et al., 2018; Praharaj et al., 2019). Differences in clinical manifestation between lambs infected with Cryptosporidium alone or together with rotavirus have been detected; however, some reports showed no differences during these two situations. More research is needed to reveal the influence of simultaneous occurrence of different pathogens, including C. parvum, which may either facilitate or antagonize concurrent infections. Some co-infections with Cryptosporidium species may not exert any response but needs to be investigated thoroughly.
The impact of protozoan-bacterial co-infections on chemotherapeutic interventions
The emergence of co-infections could have a modulating effect the on the success or failure of chemotherapy by playing a role in the emergence of antibiotic resistant strains (Birger et al., 2015). Co-infecting pathogens are often misdiagnosed due to overlapping symptomatology, delay in treatment to allow excessive proliferation of the microbe(s) that can render the host immune response insufficient to clear infection. Increase burden can facilitate development of drug resistance in one or both pathogens (U.S. Department of Health and Human Services, 2018). Targeted treatment against one pathogen may also remove a competitor and could lead to active growth of the remaining pathogen increasing the probability of evolution of drug resistant variants as seen in malaria and TB (Colombatti et al., 2011). During synergistic infections, reduced immune system-mediated killing of pathogens may allow their replication, increasing the possibility of the emergence of de novo resistance.
Rifampicin is an antitubercular drug that also exhibits potent anti-malarial activity against P. vivax in humans (Pukrittayakamee et al., 1994). Continual usage of rifampicin against TB may have resulted in discontinuing its use against malaria parasite since the therapeutic doses for the two pathogens are different. Impaired immunological control of tuberculosis due to the presence of the co-infecting Plasmodium spp. may also increase the danger of a recrudescence of partially resistant pathogen populations after therapy has ended (Okeke, 2003). An indirect effect of antimicrobials to bioavailability of drugs, can be due to a physical hindrance provided by one pathogen, such as by forming a biofilm, leading to suboptimal drug concentration at the colonization site. The presence of co-infection may also increase the abundance of antibiotic-target pathogen, and thus aiding the focal infection.
Development of antimicrobials targeting common metabolic pathways of co-infecting pathogens is a promising field of research that could limit the establishment of multidrug resistance. For example, improvement in design of sulfonamide drugs to target the de novo folate synthesis pathway, which is used by both Plasmodium spp. and M. tuberculosis is an attractive idea for consideration in drug design. In fact, sulfonamide class of antibiotics, initially developed as antibacterial agents, have been central in the development of antifolate-based combinational drugs against malaria. Co-trimoxazole as an antibacterial prophylactic agent can also prevent the incidence of malaria. The long safety history of co-trimoxazole when used in pregnancy (to treat bacterial infections) and its antimalarial prophylactic properties have led to the evaluation of this combination to also prevent malaria during pregnancy.
The impact of protozoan infections on the efficacy of bacterial/viral vaccines
Vaccines, like infections, involve participation of the innate and adaptive immune system which encompasses, phagocytosis, cytokine/chemokine secretion and activation of the antigen-specific adaptive immune response with subsequent immunological memory development. An effective adaptive immunity development involves activation of specific subsets of T lymphocytes, and stimulation of B lymphocytes to differentiate into antibody-secreting plasma cells followed by creation of protective immunological memory (Vetter et al., 2018). The critical starting point in the development of an effective vaccine requires identification of potent antigens that are appropriately presentable by professional antigen-presenting cells. Changes in the target antigens can impair development of an effective immune response. These changes may result from genetic variations arising as a consequence of co-infections resulting in failure of recognition by the adaptive immune response and occurrence of breakthrough infections (Bretsche et al., 2001). A previous meta-analysis study showed that parasitic infections such as those caused by helminths, protozoa and viruses at the time of vaccination were associated with worse immunological responses, tending to overcome infection less efficiently after post vaccination challenge. Multiple factors determine how parasitic infections impact the outcome of immunizations. These include: the type of parasite involved, immune response induced, vaccine formulation, route of administration, the target antigen, vaccine type (e.g., live attenuated, inactivated organism), study design and the timing of infection relative to vaccination (Wait et al., 2020).
The association between parasitic infections and impaired immune responses to vaccine antigens has been demonstrated for a diverse group of pathogens [(Wait et al., 2020) and Table 1]. For example, immune response induced by vaccines against Haemophilus influenzae and diphtheria (Malhotra et al., 2015), Bacille Calmette-Guerin (BCG) and tetanus toxoid (McGregor, 1962; Greenwood et al., 1972; Elliott et al., 2010; Alvarez-Larrotta et al., 2019), S. typhi (Williamson and Greenwood, 1978), acellular diphtheria-tetanus and pertussis vaccine (DTPa; Radwanska et al., 2008), HIV (Robinson et al., 2004), and potentially against SARS-CoV-2 too could be affected (Fonte et al., 2020; Gluchowska et al., 2021; Akelew et al., 2022). The impact of helminths on different vaccines outcomes has also been reported previously against pneumococcus (Apiwattanakul et al., 2014), BCG (Elias et al., 2008) and HIV-1C (Da’dara and Harn, 2010). Additionally, reduced vaccine efficacy has been associated with chronic helminth infections when compared to acute infections (Wait et al., 2020). In this review, we have focused on the impact of protozoan infections on the efficacy of vaccines against bacterial and viral infections.
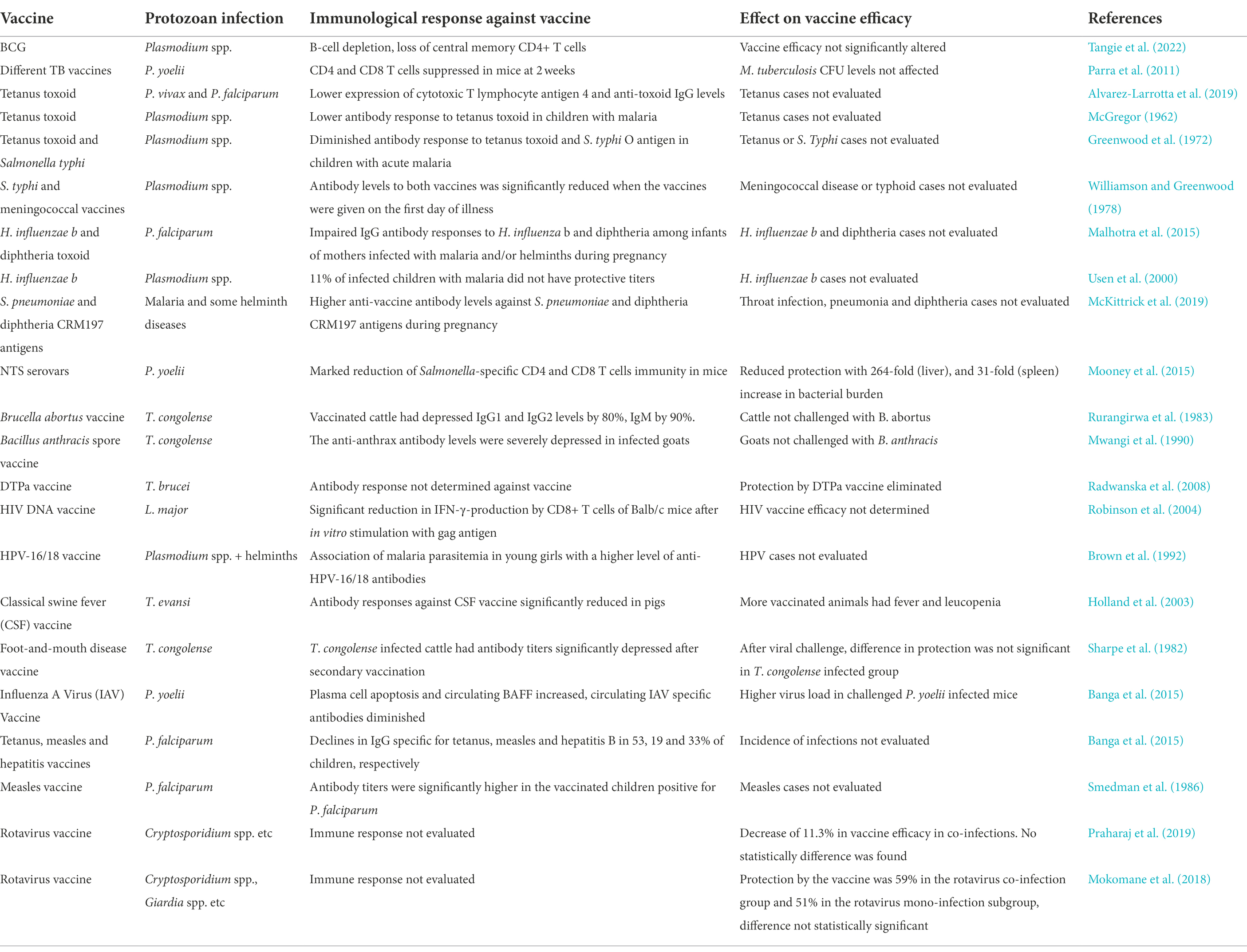
Table 1. The most common protozoa co-infections and its effects on bacterial/viral vaccines efficacy.
Protozoan parasites and vaccines against bacterial pathogens
Immunosuppression by protozoan pathogens could interfere with the immune response generated by vaccines, creating a negative correlation between parasitic infections and efficacy of vaccine in protection. Conversely, there is also evidence that vaccines may induce trained immunity and non-specific response against protozoa (Welsh and Selin, 2002; Selin et al., 2006; Agrawal, 2019) including those causing malaria and babesiosis (Clark et al., 1976, 1977; Garly et al., 2003; Walk et al., 2019). In fact, BCG vaccination, which can enhance non-specific protection to unrelated infections especially by activation of NK cells with non-specific memory by production of pro-inflammatory cytokines is provides an example (Kleinnijenhuis et al., 2014); however, parasitic infections often lead to lower antibody and IFN-γ levels, which represent a decrease in the quality of the humoral and cellular immune responses (Rowe et al., 2000).
Malaria is a highly prevalent disease in settings where poor responses to unrelated vaccines has been reported to occur (Natukunda, 2020). Several studies have shown that Plasmodium infection might impair vaccine induced protective immunity against other pathogens (Dietz et al., 1997; Cunnington and Riley, 2010). Diminished vaccines efficacy has been attributed to different factors, such as human and bacterial genotypes, exposure to environmental microbes, climate and geographical location and prevalence of co-infections (Tangie et al., 2022). A decreased protection against murine typhoid in P. yoelii-infected mice vaccinated with S. typhimurium antigens correlated with suppression of CD4 and CD8 T cell effector responses and increased anti-inflammatory IL-10 cytokine production (Mooney et al., 2015). Plasmodium spp. induced immunosuppression could be an important factor responsible for weak response to routine immunizations in malaria-endemic communities (McGregor, 1962; Gilles et al., 1983). For example, in a study in south coast of Kenya showed an impaired IgG antibody response to H. influenza b and diphtheria among infants of mothers infected with malaria and/or helminths during pregnancy compared to infants of uninfected mothers. The authors discussed that transplacental exposure of the fetus to parasite antigens in prenatal maternal malaria or helminth infections could lead an in-utero alteration of fetal immune responses affecting the response to vaccines (Malhotra et al., 2015). These observations were also confirmed in another study where Gambian children with malaria also had low antibodies titers to a H. influenza b conjugate vaccines than healthy controls, suggesting that the child cytokine profile at the time of antigen presentation likely modifies the immune response (Usen et al., 2000). Immune responses to both Salmonella typhi and meningococcal vaccines were also evaluated in Nigerian children infected with Plasmodium. Thus, S. typhi vaccination given on the first day of malaria infection had the immune responsiveness depressed, which rapidly recovered following malaria treatment while immune response to meningococcal vaccine was still impaired after a month of infection (Williamson and Greenwood, 1978). In contrast to all these findings, malaria and some helminth diseases during pregnancy were associated with minor, mostly enhancing effects on infant anti-vaccine antibody levels against S. pneumoniae and diphtheria CRM197 antigens (McKittrick et al., 2019).
Submicroscopic infection by Plasmodium spp. has been shown to be associated with a decrease in the levels of IgG against tetanus toxoid (McGregor, 1962; Greenwood et al., 1972; Alvarez-Larrotta et al., 2019), however, tetanus and diphtheria toxoids continue to offer protection in these individuals suggesting that functional immunity was sufficiently protective. Chronic protozoan infections can result in persistence of stimulating antigen that could lead to exhausted T cells with less robust effector functions, and alterations of the differentiation and sustenance of memory T cells (Costa-Madeira et al., 2022). Up-regulation and co-expression of multiple inhibitory receptors and failure to produce antigen-independent memory T cells are also observed in these cases (Schietinger and Greenberg, 2014).
Some studies showed that pre-existing Plasmodium infections did not affect the efficacy of vaccines against bacterial or viral pathogens, for instance, P. yoelii infection did not affect different formulations of TB vaccine ability to control pulmonary growth of an acute virulent M. tuberculosis infection (Parra et al., 2011). The immunological response to two doses of tetanus toxoid in groups of pregnant Kenyan women showed that the presence of Plasmodium parasitemia does not interfere with primary or secondary immune response during chemoprophylaxis against malaria (Dietz et al., 1997). Moreover, two doses of tetanus toxoid in 2-year-old Gambian children also showed that chloroquine or pyrimethamine chemoprophylaxis lead to more protective immune response when compared with children who were not treated for malaria (McGregor, 1962).
African trypanosomiasis caused by T. brucei infection inhibits protective immune responses against bacterium, Bordetella pertussis when immunized with a trivalent human vaccine, DTPa in mice (Radwanska et al., 2008). Other species of Trypanosoma can also prejudice the protective immune responses to bacterial or viral infections in animals. In an earlier study, cattle previously infected by Trypanosoma congolense when vaccinated against Brucella abortus had 80% reduction in IgG1 and IgG2 immunoglobulins (Rurangirwa et al., 1983). Furthermore, anti-anthrax antibody levels were severely depressed in T. congolense infected goats after immunization with Bacillus anthracis inactivated spore vaccine (Mwangi et al., 1990). Reduced antibody responses in infected pigs were also suggested to occur due to suppression of helper T cell caused by the concurrent T. evansi infection. In both mice and cattle, T cell proliferation was inhibited upon mitogenic stimulation and was mediated by macrophage-like suppressor cells, with reduction in IL-2 secretion together with impaired expression of the IL-2 receptor (Sileghem et al., 1989; Sileghem and Flynn, 1992). T. brucei infection also results in a rapid loss of B cells by apoptosis, reducing humoral immunity that further prevents the development of protective memory responses and thus, impair the ability of the host to recall vaccine-induced memory responses (Radwanska et al., 2008).
Protozoan parasites and vaccines against viral pathogens
Studies in mice suggested that Plasmodium infection is deleterious to pre-existing levels of heterologous antibodies. Specifically, P. chabaudi blood-stage infection of influenza-immune inbred and outbred mice resulted in a transient drop in influenza-specific antibodies and antibody secreting cells in the bone marrow (Ng et al., 2014). These results were confirmed by another study in which Malian children with pre-existing tetanus, measles, HBV vaccination had an accelerated decline in vaccine-specific IgG after acute malaria episodes (Banga et al., 2015) due to binding of Plasmodium-infected erythrocytes to bone marrow stromal cells that may disrupt the survival signals of long-lived plasma cells (Rogers et al., 2000; Kinyanjui et al., 2007; Banga et al., 2015). Additionally, BAFF receptor, which promotes survival of antibody secreting plasma cells, was found to be downregulated in splenic and bone marrow plasma cells, while circulating BAFF levels and apoptotic plasma cells increased in the bone marrow of Plasmodium-infected mice. These results were confirmed by the observation that Plasmodium-induced polyclonal B cell activation and elevated levels of immunoglobulins results in apoptosis of long-lived plasma cells through a CD32-dependent mechanism [reported in (Banga et al., 2015)]. Surprisingly, modest positive effects of pre-existing Plasmodium and helminths infections on vaccines efficacy of HPV vaccine was observed among young girls displaying Plasmodium parasitemia (Brown et al., 2014). Additionally, post-immunization measles antibody titers were significantly higher in the vaccinated children positive for P. falciparum infection than those without malaria parasites in the blood (Smedman et al., 1986).
Leishmania major is another protozoan that affects the viral vaccines-induced immune response. In BALB/c mice, infection with L. major reduced the CD8+ T cell-specific immune response induced by HIV-1 DNA vaccine suggesting that Th2 cell response caused by L. major infection can negatively affect vaccine efficacy (Robinson et al., 2004). Limited studies have been conducted to indicate contribution of Trypanosomes on viral vaccines. Foot-and-mouth disease virus vaccine was evaluated in cattle infected with T. congolense and despite significant depression in antibodies titers, their subsequent response to live virus challenge was not significantly different from the uninfected controls suggesting persistence of functional immunity (Sharpe et al., 1982). Antibody responses against classical swine fever virus vaccine were significantly decreased in T. evansi-infected pigs as compared to uninfected animals (Holland et al., 2003). Thus, Trypanosoma affects antibody responses against viral vaccine antigens but may or may not affect protection offered by the vaccines.
The Rotavirus vaccine efficacy was evaluated in diarrhea-associated co-infections in India, including those caused by Cryptosporidium. Vaccine efficacy decreased from 49.6 to 60.6% in the presence of co-infections (Praharaj et al., 2019). In another study, enteric co-infections including those by Cryptosporidium and Giardia did not affect the effectiveness of rotavirus vaccine. Therefore, lower vaccine effectiveness reported in low-income countries could not be explained only because of co-infection with Cryptosporidium (Mokomane et al., 2018).
Overall, it appears that the effect of protozoan infections on antibodies protection against vaccine antigens of different viruses is variable but the correlation of change in antibody levels with the failure or success of the vaccines in protection against the specific viral infection remains to be investigated more extensively.
Concluding remarks
Mammalian hosts encounter protozoan and other pathogens that are either transmitted consecutively or simultaneously. The impact of immunosuppression by protozoan pathogens often increases the co-infecting bacterial burden in the affected organs, thus increasing the severity of diseases they cause. It would not be surprising if chronic protozoan infection and the resulting sustained immunosuppression can activate latent infection, such as by M. tuberculosis in malaria endemic regions. Therefore, efforts to manage, treat bacterial and protozoan infections or develop novel vaccines need to consider the presence of co-infections because that could have a dramatic influence on host susceptibility to disease and the design of treatment approaches. We document variable effects of protozoan infections on bacterial and viral vaccines with significant effects on reduction in efficacy of bacterial vaccines. Some viral vaccine response remains unaltered by the presence of infecting protozoan, while antibody titer is either increased or decreased in other cases albeit their impact on protection from infection remains unclear. Therefore, booster doses of vaccines may be needed when breakthrough infections start appearing in a particular geographic region, such as tetanus toxoid booster is often required under specific circumstances. In fact, vaccines are often recommended to protect from recurrence of viral diseases such as shingles in otherwise healthy and middle-aged adults who were infected with chickenpox as children. Furthermore, a more targeted treatment approach needs to be developed for specific co-infections to avoid toxicity due to excessive use of chemotherapeutics. For example, common metabolic pathways of co-infecting pathogens can maximize antimicrobials efficacy, reduce toxicity due to excessive drug use, and curb multidrug resistance emergence. Studies in Africa under World Health Organization examined antibody titer against measles in vaccinated infants with malaria parasite presence/absence. Extension of such studies in older children and adults in regions with chronic protozoan infection could reveal the full picture of their impact on viral vaccines and would lead to better understanding of vaccines efficacy, help document causes of breakthrough infections and employ approaches for improvement of protection by vaccines.
Abbreviations and descriptions
Bacille Calmette-Guerin (BCG) is a vaccine against tuberculosis disease in regions where this disease is highly prevalent.
Bacterial vaginosis (BV) is caused by a localized inflammatory response against overgrowing natural microbiota of vagina.
Enteroaggregative E. coli (EAEC) is a pathogenic E. coli species that causes chronic diarrhea and is known because of its ability to form aggregates on intestinal mucosal surface.
Cross-Reactive-Material-197 (CRM197) is a mutant version of the diphtheria toxin rending a protein non-toxic.
Diphtheria-tetanus-acellular pertussis (DTPa) is a trivalent vaccine against three bacteria, Corynebacterium diphtheriae, Clostridium tetani and Bordetella pertussis given to children to prevent from serious diseases by these pathogens. Diphtheria causes breathing problem, tetanus results in tightening of muscles while pertussis is contagious disease that is also known as whooping cough.
Heat shock protein 70 (HSP70) is a universally expressed conserved protein in almost all living organisms that serves as chaperone for proteins for transport across membrane and also have been shown to function as potent stimulators of the innate immune system.
Non-Typhoid Salmonella (NTS) are group of Salmonella species that are not usually human pathogens unlike Salmonella enterica serovar Typhi that causes typhoid fever.
Reactive oxygen species (ROS) are oxygen containing oxygen containing reactive species produced during aerobic respiration. ROS produced by neutrophils and other phagocytes in mammalian hosts can kill organisms by causing permanent damage to DNA.
Tuberculosis (TB) is disease caused by respiratory bacterial pathogen, Mycobacterium tuberculosis that can be visualized under the microscope after acid-fast staining.
Author contributions
LA and SR wrote the initial draft and prepared Table 1. NP prepared Figure 1. All authors edited and read the final version and approved it for submission.
Funding
This work was supported by the National Institutes of Health (R01AI137425) grant to NP.
Conflict of interest
The authors declare that the research was conducted in the absence of any commercial or financial relationships that could be construed as a potential conflict of interest.
Publisher’s note
All claims expressed in this article are solely those of the authors and do not necessarily represent those of their affiliated organizations, or those of the publisher, the editors and the reviewers. Any product that may be evaluated in this article, or claim that may be made by its manufacturer, is not guaranteed or endorsed by the publisher.
References
Abbate, J. L., Becquart, P., Leroy, E., Ezenwa, V. O., and Roche, B. (2020). Exposure to Ebola virus and risk for infection with malaria parasites, rural Gabon. Emerg. Infect. Dis. 26, 229–237. doi: 10.3201/eid2602.181120
Achan, J., Serwanga, A., Wanzira, H., Kyagulanyi, T., Nuwa, A., Magumba, G., et al. (2022). Current malaria infection, previous malaria exposure, and clinical profiles and outcomes of COVID-19 in a setting of high malaria transmission: an exploratory cohort study in Uganda. Lancet Microbe 3, e62–e71. doi: 10.1016/S2666-5247(21)00240-8
Agrawal, B. (2019). Heterologous immunity: role in natural and vaccine-induced resistance to infections. Front. Immunol. 10:2631. doi: 10.3389/fimmu.2019.02631
Agrawal, S. R., Singh, V., Ingale, S., and Jain, A. P. (2014). Toxoplasmosis of spinal cord in acquired immunodeficiency syndrome patient presenting as paraparesis: a rare entity. J. Glob. Infect. Dis. 6, 178–181. doi: 10.4103/0974-777X.145248
Ahmadpour, E., Safarpour, H., Xiao, L., Zarean, M., Hatam-Nahavandi, K., Barac, A., et al. (2020). Cryptosporidiosis in HIV-positive patients and related risk factors: A systematic review and meta-analysis. Parasite 27:27. doi: 10.1051/parasite/2020025
Akelew, Y., Andualem, H., Ebrahim, E., Atnaf, A., and Hailemichael, W. (2022). Immunomodulation of COVID-19 severity by helminth co-infection: implications for COVID-19 vaccine efficacy. Immun. Inflamm. Dis. 10:e573. doi: 10.1002/iid3.573
Alberca, R. W., Yendo, T. M., Leuzzi Ramos, Y. A., Fernandes, I. G., Oliveira, L. M., Teixeira, F. M. E., et al. (2020). Case report: COVID-19 and Chagas disease in two coinfected patients. Am. J. Trop. Med. Hyg. 103, 2353–2356. doi: 10.4269/ajtmh.20-1185
Alemu, G., Alelign, D., and Abossie, A. (2018). Prevalence of opportunistic intestinal parasites and associated factors among HIV patients while receiving ART at Arba Minch Hospital in Southern Ethiopia: A cross-sectional study. Ethiop. J. Health Sci. 28, 147–156. doi: 10.4314/ejhs.v28i2.6
Alemu, A., Shiferaw, Y., Addis, Z., Mathewos, B., and Birhan, W. (2013). Effect of malaria on HIV/AIDS transmission and progression. Parasit. Vectors 6:18. doi: 10.1186/1756-3305-6-18
Alsammani, M. A., Ahmed, S. R., Alsheeha, M. A., Saadia, Z., and Khairi, S. A. (2012). Co-infection with toxoplasma gondii and Clostridium perfringens in a postpartum woman with uterine gas gangrene: a case report. J. Obstet. Gynaecol. Res. 38, 1024–1027. doi: 10.1111/j.1447-0756.2011.01817.x
Alvarez-Larrotta, C., Agudelo, O. M., Duque, Y., Gavina, K., Yanow, S. K., Maestre, A., et al. (2019). Submicroscopic plasmodium infection during pregnancy is associated with reduced antibody levels to tetanus toxoid. Clin. Exp. Immunol. 195, 96–108. doi: 10.1111/cei.13213
Aminu, M., Parason, Y. I., Abdullahi, U., Tahir, M. I., Olaniyan, F. A., and Usman, M. A. (2021). Detection of measles IgM and malaria parasite among febrile children in Zaria, Nigeria. J. Infect. Dis. Epidemiol. 7:189. doi: 10.23937/2474-3658/1510189
Anastos, K., Hoover, D. R., Burk, R. D., Cajigas, A., Shi, Q., Singh, D. K., et al. (2010). Risk factors for cervical precancer and cancer in HIV-infected, HPV-positive Rwandan women. PLoS One 5:e13525. doi: 10.1371/journal.pone.0013525
Andrade, B. B., Santos, C. J., Camargo, L. M., Souza-Neto, S. M., Reis-Filho, A., Clarencio, J., et al. (2011). Hepatitis B infection is associated with asymptomatic malaria in the Brazilian Amazon. PLoS One 6:e19841. doi: 10.1371/journal.pone.0019841
Anjorin, A. A. A., and Nwammadu, J. E. (2020). Seroepidemiology of seasonal influenza virus among unvaccinated pregnant women in Lagos Nigeria. Infez Med 28, 407–415.
Anyanwu, M. U. (2021). The association between malaria prevalence and COVID-19 mortality. BMC Infect. Dis. 21:975. doi: 10.1186/s12879-021-06701-8
Apiwattanakul, N., Thomas, P. G., Iverson, A. R., and McCullers, J. A. (2014). Chronic helminth infections impair pneumococcal vaccine responses. Vaccine 32, 5405–5410. doi: 10.1016/j.vaccine.2014.07.107
Ayoade, F., and Joel Chandranesan, A. S. (2022). “HIV-1 associated toxoplasmosis,” in StatPearls (Treasure Island, FL).
Ayouba, A., Badaut, C., Kfutwah, A., Cannou, C., Juillerat, A., Gangnard, S., et al. (2008). Specific stimulation of HIV-1 replication in human placental trophoblasts by an antigen of plasmodium falciparum. AIDS 22, 785–787. doi: 10.1097/QAD.0b013e3282f560ee
Azizieh, F., Dingle, K., Raghupathy, R., Johnson, K., VanderPlas, J., and Ansari, A. (2018). Multivariate analysis of cytokine profiles in pregnancy complications. Am. J. Reprod. Immunol. 79:e12818. doi: 10.1111/aji.12818
Bala, S., Englund, G., Kovacs, J., Wahl, L., Martin, M., Sher, A., et al. (1994). Toxoplasma gondii soluble products induce cytokine secretion by macrophages and potentiate in vitro replication of a monotropic strain of HIV. J. Eukaryot. Microbiol. 41:7S.
Baluku, J. B., Nassozi, S., Gyagenda, B., Namanda, M., Andia-Biraro, I., Worodria, W., et al. (2019). Prevalence of malaria and TB coinfection at a National Tuberculosis Treatment Centre in Uganda. J. Trop. Med. 2019:3741294. doi: 10.1155/2019/3741294
Banga, S., Coursen, J. D., Portugal, S., Tran, T. M., Hancox, L., Ongoiba, A., et al. (2015). Impact of acute malaria on pre-existing antibodies to viral and vaccine antigens in mice and humans. PLoS One 10:e0125090. doi: 10.1371/journal.pone.0125090
Bartelt, L. A., Bolick, D. T., Mayneris-Perxachs, J., Kolling, G. L., Medlock, G. L., Zaenker, E. I., et al. (2017). Cross-modulation of pathogen-specific pathways enhances malnutrition during enteric co-infection with Giardia lamblia and enteroaggregative Escherichia coli. PLoS Pathog. 13:e1006471. doi: 10.1371/journal.ppat.1006471
Barthold, S. W., Cadavid, D., and Philipp, M. T. (2010) in Borrelia: Molecular biology, host interaction, and pathogenesis. eds. D. S. Samuels and J. D. Radolf (Norfolk, UK: Caister Academic), 359–411.
Basavaraju, A. (2016). Toxoplasmosis in HIV infection: an overview. Trop. Parasitol. 6, 129–135. doi: 10.4103/2229-5070.190817
Beck, H. P., Felger, I., Genton, B., Alexander, N., al-Yaman, F., Anders, R. F., et al. (1995). Humoral and cell-mediated immunity to the Plasmodium falciparum ring-infected erythrocyte surface antigen in an adult population exposed to highly endemic malaria. Infect. Immun. 63, 596–600. doi: 10.1128/iai.63.2.596-600.1995
Benach, J. L., Coleman, J. L., Habicht, G. S., MacDonald, A., Grunwaldt, E., and Giron, J. A. (1985). Serological evidence for simultaneous occurrences of Lyme disease and babesiosis. J. Infect. Dis. 152, 473–477. doi: 10.1093/infdis/152.3.473
Birger, R. B., Kouyos, R. D., Cohen, T., Griffiths, E. C., Huijben, S., Mina, M. J., et al. (2015). The potential impact of coinfection on antimicrobial chemotherapy and drug resistance. Trends Microbiol. 23, 537–544. doi: 10.1016/j.tim.2015.05.002
Blank, J., Eggers, L., Behrends, J., Jacobs, T., and Schneider, B. E. (2016). One episode of self-resolving plasmodium yoelii infection transiently exacerbates chronic mycobacterium tuberculosis infection. Front. Microbiol. 7:152. doi: 10.3389/fmicb.2016.00152
Boonyarangka, P., Phontham, K., Sriwichai, S., Poramathikul, K., Harncharoenkul, K., Kuntawunginn, W., et al. (2022). Co-infection with plasmodium vivax and COVID-19 in Thailand. Trop. Med. Infect. Dis. 7:145. doi: 10.3390/tropicalmed7080145
Borad, A., and Ward, H. (2010). Human immune responses in cryptosporidiosis. Future Microbiol. 5, 507–519. doi: 10.2217/fmb.09.128
Boutlis, C. S., Yeo, T. W., and Anstey, N. M. (2006). Malaria tolerance--for whom the cell tolls? Trends Parasitol. 22, 371–377. doi: 10.1016/j.pt.2006.06.002
Bradbury, R. S., Piedrafita, D., Greenhill, A., and Mahanty, S. (2020). Will helminth co-infection modulate COVID-19 severity in endemic regions? Nat. Rev. Immunol. 20:342. doi: 10.1038/s41577-020-0330-5
Bretsche, P. A., Ismail, N., Menon, J. N., Power, C. A., Uzonna, J., and Wei, G. (2001). Vaccination against and treatment of tuberculosis, the leishmaniases and AIDS: perspectives from basic immunology and immunity to chronic intracellular infections. Cell. Mol. Life Sci. 58, 1879–1896. doi: 10.1007/pl00000824
Bretscher, P. A. (1992). An hypothesis to explain why cell-mediated immunity alone can contain infections by certain intracellular parasites and how immune class regulation of the response against such parasites can be subverted. Immunol. Cell Biol. 70, 343–351. doi: 10.1038/icb.1992.44
Bronowski, C., James, C. E., and Winstanley, C. (2014). Role of environmental survival in transmission of Campylobacter jejuni. FEMS Microbiol. Lett. 356, 8–19. doi: 10.1111/1574-6968.12488
Brown, J., Baisley, K., Kavishe, B., Changalucha, J., Andreasen, A., Mayaud, P., et al. (2014). Impact of malaria and helminth infections on immunogenicity of the human papillomavirus-16/18 AS04-adjuvanted vaccine in Tanzania. Vaccine 32, 611–617. doi: 10.1016/j.vaccine.2013.11.061
Brown, A. E., Mongkolsirichaikul, D., Innis, B., Snitbhan, R., and Webster, H. K. (1992). Falciparum malaria modulates viremia in chronic hepatitis B virus infection. J. Infect. Dis. 166, 1465–1466. doi: 10.1093/infdis/166.6.1465
Chen, X. M., and LaRusso, N. F. (2002). Cryptosporidiosis and the pathogenesis of AIDS-cholangiopathy. Semin. Liver Dis. 22, 277–290. doi: 10.1055/s-2002-34505
Chukwuanukwu, R. C., Onyenekwe, C. C., Martinez-Pomares, L., Flynn, R., Singh, S., Amilo, G. I., et al. (2017). Modulation of the immune response to mycobacterium tuberculosis during malaria/M. tuberculosis co-infection. Clin. Exp. Immunol. 187, 259–268. doi: 10.1111/cei.12861
Clark, I. A., Allison, A. C., and Cox, F. E. (1976). Protection of mice against Babesia and plasmodium with BCG. Nature 259, 309–311. doi: 10.1038/259309a0
Clark, I. A., Wills, E. J., Richmond, J. E., and Allison, A. C. (1977). Suppression of babesiosis in BCG-infected mice and its correlation with tumor inhibition. Infect. Immun. 17, 430–438. doi: 10.1128/iai.17.2.430-438.1977
Coban, C., Ishii, K. J., Kawai, T., Hemmi, H., Sato, S., Uematsu, S., et al. (2005). Toll-like receptor 9 mediates innate immune activation by the malaria pigment hemozoin. J. Exp. Med. 201, 19–25. doi: 10.1084/jem.20041836
Colombatti, R., Penazzato, M., Bassani, F., Vieira, C. S., Lourenco, A. A., Vieira, F., et al. (2011). Malaria prevention reduces in-hospital mortality among severely ill tuberculosis patients: a three-step intervention in Bissau, Guinea-Bissau. BMC Infect. Dis. 11:57. doi: 10.1186/1471-2334-11-57
Conner, M., Joshi, T., Veerisetty, S. S., and Hutchings, J. (2019). Coinfection of cytomegalovirus and cryptosporidiosis in a patient with AIDS. ACG Case Rep. J. 6:e00225. doi: 10.14309/crj.0000000000000225
Costa-Madeira, J. C., Trindade, G. B., Almeida, P. H. P., Silva, J. S., and Carregaro, V. (2022). T lymphocyte exhaustion during human and experimental visceral Leishmaniasis. Front. Immunol. 13:835711. doi: 10.3389/fimmu.2022.835711
Cox, F. E. (2001). Concomitant infections, parasites and immune responses. Parasitology 122, S23–S38. doi: 10.1017/S003118200001698X
Cunnington, A. J., de Souza, J. B., Walther, M., and Riley, E. M. (2011). Malaria impairs resistance to salmonella through heme- and heme oxygenase-dependent dysfunctional granulocyte mobilization. Nat. Med. 18, 120–127. doi: 10.1038/nm.2601
Cunnington, A. J., Njie, M., Correa, S., Takem, E. N., Riley, E. M., and Walther, M. (2012). Prolonged neutrophil dysfunction after plasmodium falciparum malaria is related to hemolysis and heme oxygenase-1 induction. J. Immunol. 189, 5336–5346. doi: 10.4049/jimmunol.1201028
Cunnington, A. J., and Riley, E. M. (2010). Suppression of vaccine responses by malaria: insignificant or overlooked? Expert Rev. Vaccines 9, 409–429. doi: 10.1586/erv.10.16
Cu-Uvin, S., Ko, H., Jamieson, D. J., Hogan, J. W., Schuman, P., Anderson, J., et al. (2002). Prevalence, incidence, and persistence or recurrence of trichomoniasis among human immunodeficiency virus (HIV)-positive women and among HIV-negative women at high risk for HIV infection. Clin. Infect. Dis. 34, 1406–1411. doi: 10.1086/340264
Da Cunha, S., Ferreira, E., Ramos, I., Martins, R., De Freitas, L., Borges, J. L., et al. (1994). Cerebral toxoplasmosis after renal transplantation. Case report and review. Acta Medica Port. 7, S61–S66.
Da’dara, A. A., and Harn, D. A. (2010). Elimination of helminth infection restores HIV-1C vaccine-specific T cell responses independent of helminth-induced IL-10. Vaccine 28, 1310–1317. doi: 10.1016/j.vaccine.2009.11.019
Davis, A., Dasgupta, A., Goddard-Eckrich, D., and El-Bassel, N. (2016). Trichomonas vaginalis and human immunodeficiency virus coinfection among women under community supervision: A call for Expanded T. vaginalis screening. Sex. Transm. Dis. 43, 617–622. doi: 10.1097/OLQ.0000000000000503
Delanghe, J. R., Speeckaert, M. M., and De Buyzere, M. L. (2020). The host’s angiotensin-converting enzyme polymorphism may explain epidemiological findings in COVID-19 infections. Clin. Chim. Acta 505, 192–193. doi: 10.1016/j.cca.2020.03.031
Denoncourt, A. M., Paquet, V. E., and Charette, S. J. (2014). Potential role of bacteria packaging by protozoa in the persistence and transmission of pathogenic bacteria. Front. Microbiol. 5:240. doi: 10.3389/fmicb.2014.00240
Dietz, V., Galazka, A., van Loon, F., and Cochi, S. (1997). Factors affecting the immunogenicity and potency of tetanus toxoid: implications for the elimination of neonatal and non-neonatal tetanus as public health problems. Bull. World Health Organ. 75, 81–93.
Diro, E., Lynen, L., Ritmeijer, K., Boelaert, M., Hailu, A., and van Griensven, J. (2014). Visceral Leishmaniasis and HIV coinfection in East Africa. PLoS Negl. Trop. Dis. 8:e2869. doi: 10.1371/journal.pntd.0002869
Diuk-Wasser, M. A., Vannier, E., and Krause, P. J. (2016). Coinfection by Ixodes tick-borne pathogens: ecological, epidemiological, and clinical consequences. Trends Parasitol. 32, 30–42. doi: 10.1016/j.pt.2015.09.008
Djokic, V., Akoolo, L., Primus, S., Schlachter, S., Kelly, K., Bhanot, P., et al. (2019). Protozoan parasite Babesia microti subverts adaptive immunity and enhances Lyme disease severity. Front. Microbiol. 10:1596. doi: 10.3389/fmicb.2019.01596
Djokic, V., Rocha, S. C., and Parveen, N. (2021). Lessons learned for pathogenesis, immunology, and disease of Erythrocytic parasites: plasmodium and Babesia. Front. Cell. Infect. Microbiol. 11:685239. doi: 10.3389/fcimb.2021.685239
Doolan, D. L., Dobano, C., and Baird, J. K. (2009). Acquired immunity to malaria. Clin. Microbiol. Rev. 22, 13–36, Table of Contents. doi: 10.1128/CMR.00025-08
Dunn, J. M., Krause, P. J., Davis, S., Vannier, E. G., Fitzpatrick, M. C., Rollend, L., et al. (2014). Borrelia burgdorferi promotes the establishment of Babesia microti in the northeastern United States. PLoS One 9:e115494. doi: 10.1371/journal.pone.0115494
Elias, D., Britton, S., Aseffa, A., Engers, H., and Akuffo, H. (2008). Poor immunogenicity of BCG in helminth infected population is associated with increased in vitro TGF-beta production. Vaccine 26, 3897–3902. doi: 10.1016/j.vaccine.2008.04.083
Elliott, A. M., Mawa, P. A., Webb, E. L., Nampijja, M., Lyadda, N., Bukusuba, J., et al. (2010). Effects of maternal and infant co-infections, and of maternal immunisation, on the infant response to BCG and tetanus immunisation. Vaccine 29, 247–255. doi: 10.1016/j.vaccine.2010.10.047
Fairlie-Clarke, K. J., Allen, J. E., Read, A. F., and Graham, A. L. (2013). Quantifying variation in the potential for antibody-mediated apparent competition among nine genotypes of the rodent malaria parasite plasmodium chabaudi. Infect. Genet. Evol. 20, 270–275. doi: 10.1016/j.meegid.2013.09.013
Feldman, S., Stein, D., Amrute, S., Denny, T., Garcia, Z., Kloser, P., et al. (2001). Decreased interferon-alpha production in HIV-infected patients correlates with numerical and functional deficiencies in circulating type 2 dendritic cell precursors. Clin. Immunol. 101, 201–10. doi: 10.1006/clim.2001.5111
Fernandez-Lopez, L. A., Gil-Becerril, K., Galindo-Gomez, S., Estrada-Garcia, T., Ximenez, C., Leon-Coria, A., et al. (2019). Entamoeba histolytica interaction with Enteropathogenic Escherichia coli increases parasite virulence and inflammation in Amebiasis. Infect. Immun. 87:e00279–19. doi: 10.1128/IAI.00279-19
Fiori, P. L., Diaz, N., Cocco, A. R., Rappelli, P., and Dessi, D. (2013). Association of Trichomonas vaginalis with its symbiont mycoplasma hominis synergistically upregulates the in vitro proinflammatory response of human monocytes. Sex. Transm. Infect. 89, 449–454. doi: 10.1136/sextrans-2012-051006
Fonte, L., Acosta, A., Sarmiento, M. E., Ginori, M., Garcia, G., and Norazmi, M. N. (2020). COVID-19 lethality in sub-Saharan Africa and helminth immune modulation. Front. Immunol. 11:574910. doi: 10.3389/fimmu.2020.574910
Fregonesi, B. M., Suzuki, M. N., Machado, C. S., Tonani, K. A., Fernandes, A. P., Monroe, A. A., et al. (2015). Emergent and re-emergent parasites in HIV-infected children: immunological and socio-environmental conditions that are involved in the transmission of giardia spp. and cryptosporidium spp. Rev. Soc. Bras. Med. Trop. 48, 753–758. doi: 10.1590/0037-8682-0119-2015
Froebel, K., Howard, W., Schafer, J. R., Howie, F., Whitworth, J., Kaleebu, P., et al. (2004). Activation by malaria antigens renders mononuclear cells susceptible to HIV infection and re-activates replication of endogenous HIV in cells from HIV-infected adults. Parasite Immunol. 26, 213–217. doi: 10.1111/j.0141-9838.2004.00701.x
Galvan-Moroyoqui, J. M., Del Carmen Dominguez-Robles, M., Franco, E., and Meza, I. (2008). The interplay between Entamoeba and enteropathogenic bacteria modulates epithelial cell damage. PLoS Negl. Trop. Dis. 2:e266. doi: 10.1371/journal.pntd.0000266
Garly, M. L., Martins, C. L., Bale, C., Balde, M. A., Hedegaard, K. L., Gustafson, P., et al. (2003). BCG scar and positive tuberculin reaction associated with reduced child mortality in West Africa. A non-specific beneficial effect of BCG? Vaccine 21, 2782–2790. doi: 10.1016/s0264-410x(03)00181-6
Gigley, J. P., Bhadra, R., Moretto, M. M., and Khan, I. A. (2012). T cell exhaustion in protozoan disease. Trends Parasitol. 28, 377–384. doi: 10.1016/j.pt.2012.07.001
Gilles, H. M., Greenwood, B. M., Greenwood, A. M., Bradley, A. K., Blakebrough, I., Pugh, R. N., et al. (1983). The Malumfashi project--an epidemiological, clinical and laboratory study. Trans. R. Soc. Trop. Med. Hyg. 77, 24–31. doi: 10.1016/0035-9203(83)90005-6
Gluchowska, K., Dzieciatkowski, T., Sedzikowska, A., Zawistowska-Deniziak, A., and Mlocicki, D. (2021). The new status of parasitic diseases in the COVID-19 pandemic-risk factors or protective agents? J. Clin. Med. 10. doi: 10.3390/jcm10112533
Gozzelino, R., Jeney, V., and Soares, M. P. (2010). Mechanisms of cell protection by heme oxygenase-1. Annu. Rev. Pharmacol. Toxicol. 50, 323–354. doi: 10.1146/annurev.pharmtox.010909.105600
Greenwood, B. M., Bradley-Moore, A. M., Bryceson, A. D., and Palit, A. (1972). Immunosuppression in children with malaria. Lancet 299, 169–172. doi: 10.1016/s0140-6736(72)90569-7
Guha, R., Mathioudaki, A., Doumbo, S., Doumtabe, D., Skinner, J., Arora, G., et al. (2020). Plasmodium falciparum malaria drives epigenetic reprogramming of human monocytes toward a regulatory phenotype. PLoS Pathog. 17:e1009430 doi: 10.1371/journal.ppat.1009430
Gupta, G., Oghumu, S., and Satoskar, A. R. (2013). Mechanisms of immune evasion in leishmaniasis. Adv. Appl. Microbiol. 82, 155–184. doi: 10.1016/B978-0-12-407679-2.00005-3
Gutman, J. R., Lucchi, N. W., Cantey, P. T., Steinhardt, L. C., Samuels, A. M., Kamb, M. L., et al. (2020). Malaria and parasitic neglected tropical diseases: potential Syndemics with COVID-19? Am. J. Trop. Med. Hyg. 103, 572–577. doi: 10.4269/ajtmh.20-0516
Hailu, A. W., S, G. S., Merid, Y., Gebru, A. A., Ayene, Y. Y., and Asefa, M. K. (2015). The case control studies of HIV and intestinal parasitic infections rate in active pulmonary tuberculosis patients in Woldia general hospital and health Center in North Wollo, Amhara region Ethiopia. Int. J. Pharma. Sci. 5, 1092–1099.
Hansen, D. S., D’Ombrain, M. C., and Schofield, L. (2007). The role of leukocytes bearing natural killer complex receptors and killer immunoglobulin-like receptors in the immunology of malaria. Curr. Opin. Immunol. 19, 416–423. doi: 10.1016/j.coi.2007.07.011
Harding, C. L., Villarino, N. F., Valente, E., Schwarzer, E., and Schmidt, N. W. (2020). Plasmodium impairs antibacterial innate immunity to systemic infections in part through Hemozoin-bound bioactive molecules. Front. Cell. Infect. Microbiol. 10:328. doi: 10.3389/fcimb.2020.00328
Helegbe, G. K., Aryee, P. A., Mohammed, B. S., Wemakor, A., Kolbila, D., Abubakari, A. W., et al. (2018). Seroprevalence of malaria and hepatitis B coinfection among pregnant women in tamale Metropolis of Ghana: A cross-sectional study. Can. J. Infect. Dis. Med. Microbiol. 2018:5610981. doi: 10.1155/2018/5610981
Holland, W. G., Do, T. T., Huong, N. T., Dung, N. T., Thanh, N. G., Vercruysse, J., et al. (2003). The effect of Trypanosoma evansi infection on pig performance and vaccination against classical swine fever. Vet. Parasitol. 111, 115–123. doi: 10.1016/s0304-4017(02)00363-1
Hsieh, S. M., Chen, M. Y., Pan, S. C., Hung, C. C., and Chang, S. C. (2007). Aberrant induction of regulatory activity of CD4+CD25+ T cells by dendritic cells in HIV-infected persons with amebic liver abscess. J. Acquir. Immune Defic. Syndr. 44, 6–13. doi: 10.1097/01.qai.0000242457.43392.61
Hung, C. C., Chang, S. Y., and Ji, D. D. (2012). Entamoeba histolytica infection in men who have sex with men. Lancet Infect. Dis. 12, 729–736. doi: 10.1016/S1473-3099(12)70147-0
Hung, C. C., Deng, H. Y., Hsiao, W. H., Hsieh, S. M., Hsiao, C. F., Chen, M. Y., et al. (2005). Invasive amebiasis as an emerging parasitic disease in patients with human immunodeficiency virus type 1 infection in Taiwan. Arch. Intern. Med. 165, 409–415. doi: 10.1001/archinte.165.4.409
Hung, C. C., Ji, D. D., Sun, H. Y., Lee, Y. T., Hsu, S. Y., Chang, S. Y., et al. (2008). Increased risk for Entamoeba histolytica infection and invasive amebiasis in HIV seropositive men who have sex with men in Taiwan. PLoS Negl. Trop. Dis. 2:e175. doi: 10.1371/journal.pntd.0000175
Hunter, P. R., and Nichols, G. (2002). Epidemiology and clinical features of cryptosporidium infection in immunocompromised patients. Clin. Microbiol. Rev. 15, 145–154. doi: 10.1128/CMR.15.1.145-154.2002
Hussein, M. I. H., Albashir, A. A. D., Elawad, O., and Homeida, A. (2020). Malaria and COVID-19: unmasking their ties. Malar. J. 19:457. doi: 10.1186/s12936-020-03541-w
Hussein, R., Guedes, M., Ibraheim, N., Ali, M. M., El-Tahir, A., Allam, N., et al. (2022). Impact of COVID-19 and malaria coinfection on clinical outcomes: a retrospective cohort study. Clin. Microbiol. Infect. 28, 1152.e1–1152.e6. doi: 10.1016/j.cmi.2022.03.028
Ibrahim, A., Ali, Y. B. M., Abdel-Aziz, A., and El-Badry, A. A. (2019). Helicobacter pylori and enteric parasites co-infection among diarrheic and non-diarrheic Egyptian children: seasonality, estimated risks, and predictive factors. J. Parasit. Dis. 43, 198–208. doi: 10.1007/s12639-018-1075-y
Ifudu, O., and Fowler, A. (2001). Hepatitis B virus infection and the response to erythropoietin in end-stage renal disease. ASAIO J. 47, 569–572. doi: 10.1097/00002480-200109000-00035
Inion, I., Mwanyumba, F., Gaillard, P., Chohan, V., Verhofstede, C., Claeys, P., et al. (2003). Placental malaria and perinatal transmission of human immunodeficiency virus type 1. J. Infect. Dis. 188, 1675–1678. doi: 10.1086/379737
Irisarri-Gutierrez, M. J., Mingo, M. H., de Lucio, A., Gil, H., Morales, L., Segui, R., et al. (2017). Association between enteric protozoan parasites and gastrointestinal illness among HIV- and tuberculosis-infected individuals in the Chowke district, southern Mozambique. Acta Trop. 170, 197–203. doi: 10.1016/j.actatropica.2017.03.010
Izzo, M. M., Kirkland, P. D., Mohler, V. L., Perkins, N. R., Gunn, A. A., and House, J. K. (2011). Prevalence of major enteric pathogens in Australian dairy calves with diarrhoea. Aust. Vet. J. 89, 167–173. doi: 10.1111/j.1751-0813.2011.00692.x
Jacobs, J. W., and Siddon, A. J. (2021). Concurrent COVID-19 and babesiosis in an older, splenectomized patient. Blood 138:2154. doi: 10.1182/blood.2021013947
Junaedi, M., Katu, S., Ilyas, M., Daud, N., Saleh, S., and Nurjannah Lihawa, H. (2020). Case report: Covid-19 and severe malaria co-infection. Eur. J. Mol. Clin. Med. 7, 961–968.
Karp, C. L., and Auwaerter, P. G. (2007). Coinfection with HIV and tropical infectious diseases protozoal pathogens. Clin. Infect. Dis. 45, 1208–1213. doi: 10.1086/522181
Kassa, D., Petros, B., Mesele, T., Hailu, E., and Wolday, D. (2006). Characterization of peripheral blood lymphocyte subsets in patients with acute Plasmodium falciparum and P. vivax malaria infections at Wonji sugar estate, Ethiopia. Clin. Vaccine Immunol. 13, 376–379. doi: 10.1128/CVI.13.3.376-379.2006
Kaushik, K., Khurana, S., Wanchu, A., and Malla, N. (2009). Serum immunoglobulin G, M and A response to Cryptosporidium parvum in cryptosporidium-HIV co-infected patients. BMC Infect. Dis. 9:179. doi: 10.1186/1471-2334-9-179
Kinyanjui, S. M., Conway, D. J., Lanar, D. E., and Marsh, K. (2007). IgG antibody responses to plasmodium falciparum merozoite antigens in Kenyan children have a short half-life. Malar. J. 6:82. doi: 10.1186/1475-2875-6-82
Klassen, D. K., and Spivak, J. L. (1990). Hepatitis-related hepatic erythropoietin production. Am. J. Med. 89, 684–686. doi: 10.1016/0002-9343(90)90190-o
Kleinnijenhuis, J., Quintin, J., Preijers, F., Joosten, L. A., Jacobs, C., Xavier, R. J., et al. (2014). BCG-induced trained immunity in NK cells: role for non-specific protection to infection. Clin. Immunol. 155, 213–219. doi: 10.1016/j.clim.2014.10.005
Knapp, K. L., and Rice, N. A. (2015). Human coinfection with Borrelia burgdorferi and Babesia microti in the United States. J. Parasitol. Res. 2015:587131. doi: 10.1155/2015/587131
Kotepui, K. U., and Kotepui, M. (2020). Prevalence of and risk factors for plasmodium spp. co-infection with hepatitis B virus: a systematic review and meta-analysis. Malar. J. 19:368. doi: 10.1186/s12936-020-03428-w
Krause, P. J., Telford, S. R. 3rd, Spielman, A., Sikand, V., Ryan, R., Christianson, D., et al. (1996). Concurrent Lyme disease and babesiosis: Evidence for increased severity and duration of illness. JAMA 275, 1657–1660. doi: 10.1001/jama.1996.03530450047031
Laufer, M. K., van Oosterhout, J. J., Thesing, P. C., Dzinjalamala, F. K., Hsi, T., Beraho, L., et al. (2007). Malaria treatment efficacy among people living with HIV: the role of host and parasite factors. Am. J. Trop. Med. Hyg. 77, 627–632. doi: 10.4269/ajtmh.2007.77.627
Lejeune, M., Miro, J. M., De Lazzari, E., Garcia, F., Claramonte, X., Martinez, E., et al. (2011). Restoration of T cell responses to toxoplasma gondii after successful combined antiretroviral therapy in patients with AIDS with previous toxoplasmic encephalitis. Clin. Infect. Dis. 52, 662–670. doi: 10.1093/cid/ciq197
Lo, Y. C., Ji, D. D., and Hung, C. C. (2014). Prevalent and incident HIV diagnoses among Entamoeba histolytica-infected adult males: a changing epidemiology associated with sexual transmission--Taiwan, 2006–2013. PLoS Negl. Trop. Dis. 8:e3222. doi: 10.1371/journal.pntd.0003222
Lowther, S. A., Dworkin, M. S., and Hanson, D. L. (2000). Entamoeba histolytica/Entamoeba dispar infections in human immunodeficiency virus-infected patients in the United States. Clin. Infect. Dis. 30, 955–959. doi: 10.1086/313811
Machelart, A., Van Vyve, M., Potemberg, G., Demars, A., De Trez, C., Tima, H. G., et al. (2017). Trypanosoma infection favors Brucella elimination via IL-12/IFNgamma-dependent pathways. Front. Immunol. 8:903. doi: 10.3389/fimmu.2017.00903
Malhotra, I., McKibben, M., Mungai, P., McKibben, E., Wang, X., Sutherland, L. J., et al. (2015). Effect of antenatal parasitic infections on anti-vaccine IgG levels in children: a prospective birth cohort study in Kenya. PLoS Negl. Trop. Dis. 9:e0003466. doi: 10.1371/journal.pntd.0003466
Manko, A., Motta, J. P., Cotton, J. A., Feener, T., Oyeyemi, A., Vallance, B. A., et al. (2017). Giardia co-infection promotes the secretion of antimicrobial peptides beta-defensin 2 and trefoil factor 3 and attenuates attaching and effacing bacteria-induced intestinal disease. PLoS One 12:e0178647. doi: 10.1371/journal.pone.0178647
Márquez-Contreras, M. E. (2018). Mechanisms of immune evasion by Trypanosoma brucei. Microbiol. Curr. Res. 02, 39–44. doi: 10.4066/2591-8036.18-303
Marti, H., Kim, H., Joseph, S. J., Dojiri, S., Read, T. D., and Dean, D. (2017). Tet(C) gene transfer between chlamydia suis strains occurs by homologous recombination after co-infection: implications for spread of tetracycline-resistance among Chlamydiaceae. Front. Microbiol. 8:156. doi: 10.3389/fmicb.2017.00156
McGregor, I. A. A. B. M. (1962). Antibody response to tetanus toxoid inoculation in malarious and non-malarious Gambian children. Trans. R. Soc. Trop. Med. Hyg. 56, 364–367. doi: 10.1016/0035-9203(62)90005-6
McKittrick, N. D., Malhotra, I. J., Vu, D. M., Boothroyd, D. B., Lee, J., Krystosik, A. R., et al. (2019). Parasitic infections during pregnancy need not affect infant antibody responses to early vaccination against Streptococcus pneumoniae, diphtheria, or Haemophilus influenzae type B. PLoS Negl. Trop. Dis. 13:e0007172. doi: 10.1371/journal.pntd.0007172
Moens, L., Wuyts, G., Verhaegen, J., and Bossuyt, X. (2012). Cross-protective immunity against heterologous Streptococcus pneumoniae. Infect. Immun. 80, 1944–1945; author reply 1946. doi: 10.1128/IAI.06397-11
Mokomane, M., Tate, J. E., Steenhoff, A. P., Esona, M. D., Bowen, M. D., Lechiile, K., et al. (2018). Evaluation of the influence of gastrointestinal coinfections on rotavirus vaccine effectiveness in Botswana. Pediatr. Infect. Dis. J. 37, e58–e62. doi: 10.1097/INF.0000000000001828
Montazeri, M., Nakhaei, M., Fakhar, M., Pazoki, H., Pagheh, A. S., Nazar, E., et al. (2022). Exploring the association between latent toxoplasma gondii infection and COVID-19 in hospitalized patients: first registry-based study. Acta Parasitol. 67, 1172–1179. doi: 10.1007/s11686-022-00559-9
Montoya, J. G., and Liesenfeld, O. (2004). Toxoplasmosis. Lancet 363, 1965–1976. doi: 10.1016/S0140-6736(04)16412-X
Mooney, J. P., Lee, S. J., Lokken, K. L., Nanton, M. R., Nuccio, S. P., McSorley, S. J., et al. (2015). Transient loss of protection afforded by a live attenuated non-typhoidal salmonella vaccine in mice co-infected with malaria. PLoS Negl. Trop. Dis. 9:e0004027. doi: 10.1371/journal.pntd.0004027
Moro, M. H., Zegarra-Moro, O. L., Bjornsson, J., Hofmeister, E. K., Bruinsma, E., Germer, J. J., et al. (2002). Increased arthritis severity in mice coinfected with Borrelia burgdorferi and Babesia microti. J. Infect. Dis. 186, 428–431. doi: 10.1086/341452
Mtove, G., Amos, B., Nadjm, B., Hendriksen, I. C., Dondorp, A. M., Mwambuli, A., et al. (2011). Decreasing incidence of severe malaria and community-acquired bacteraemia among hospitalized children in Muheza, North-Eastern Tanzania, 2006-2010. Malar. J. 10:320. doi: 10.1186/1475-2875-10-320
Mueller, A. K., Behrends, J., Blank, J., Schaible, U. E., and Schneider, B. E. (2014). An experimental model to study tuberculosis-malaria coinfection upon natural transmission of mycobacterium tuberculosis and plasmodium berghei. J. Vis. Exp. 84:e50829. doi: 10.3791/50829
Murray, C. J., Ortblad, K. F., Guinovart, C., Lim, S. S., Wolock, T. M., Roberts, D. A., et al. (2014). Global, regional, and national incidence and mortality for HIV, tuberculosis, and malaria during 1990-2013: a systematic analysis for the global burden of disease study 2013. Lancet 384, 1005–1070. doi: 10.1016/S0140-6736(14)60844-8
Musaya, J., Matovu, E., Nyirenda, M., and Chisi, J. (2015). Role of cytokines in Trypanosoma brucei-induced anaemia: A review of the literature. Malawi Med. J. 27, 45–50. doi: 10.4314/mmj.v27i2.3
Mwangi, D. M., Munyua, W. K., and Nyaga, P. N. (1990). Immunosuppression in caprine trypanosomiasis: effects of acute Trypanosoma congolense infection on antibody response to anthrax spore vaccine. Trop. Anim. Health Prod. 22, 95–100. doi: 10.1007/BF02239832
Nahrendorf, W., Ivens, A., and Spence, P. J. (2021). Inducible mechanisms of disease tolerance provide an alternative strategy of acquired immunity to malaria. elife 10:e63838. doi: 10.7554/eLife.63838
Natukunda, A. E. A. (2020). Effect of intermittent preventive treatment for malaria with dihydroartemisinin-piperaquine on immune responses to vaccines among rural Ugandan adolescents: randomized controlled trial protocol B for the “population differences in vaccine responses,” (POPVAC) program. BMJ Open 11:e040427. doi: 10.1136/bmjopen-2020-040427
Netea, M. G., Dominguez-Andres, J., Barreiro, L. B., Chavakis, T., Divangahi, M., Fuchs, E., et al. (2020). Defining trained immunity and its role in health and disease. Nat. Rev. Immunol. 20, 375–388. doi: 10.1038/s41577-020-0285-6
Netea, M. G., Quintin, J., and van der Meer, J. W. (2011). Trained immunity: a memory for innate host defense. Cell Host Microbe 9, 355–361. doi: 10.1016/j.chom.2011.04.006
Ng, D. H., Skehel, J. J., Kassiotis, G., and Langhorne, J. (2014). Recovery of an antiviral antibody response following attrition caused by unrelated infection. PLoS Pathog. 10:e1003843. doi: 10.1371/journal.ppat.1003843
Nyakoe, N. K., Taylor, R. P., Makumi, J. N., and Waitumbi, J. N. (2009). Complement consumption in children with plasmodium falciparum malaria. Malar. J. 8:7. doi: 10.1186/1475-2875-8-7
Ojurongbe, O., Raji, O. A., Akindele, A. A., Kareem, M. I., Adefioye, O. A., and Adeyeba, A. O. (2011). Cryptosporidium and other enteric parasitic infections in HIV-seropositive individuals with and without diarrhoea in Osogbo Nigeria. Br. J. Biomed. Sci. 68, 75–78. doi: 10.1080/09674845.2011.11730327
Okeke, I. N. (2003). “Factors contributing to the emergence of resistance,” in The resistance phenomenon in microbes and infectious disease vectors: Implications for human health and strategies for containment: Workshop summary. eds. L. S. K. SL and M. Najafi, et al. (United States: National Acaemies Press)
Okwor, I., and Uzonna, J. E. (2013). The immunology of Leishmania/HIV co-infection. Immunol. Res. 56, 163–171. doi: 10.1007/s12026-013-8389-8
Olsthoorn, F., Sprong, H., Fonville, M., Rocchi, M., Medlock, J., Gilbert, L., et al. (2021). Occurrence of tick-borne pathogens in questing Ixodes ricinus ticks from wester Ross Northwest Scotland. Parasit Vect. 14:430. doi: 10.1186/s13071-021-04946-5
Onderdonk, A. B., Delaney, M. L., and Fichorova, R. N. (2016). The human microbiome during bacterial vaginosis. Clin. Microbiol. Rev. 29, 223–238. doi: 10.1128/CMR.00075-15
Osei, S. A., Biney, R. P., Anning, A. S., Nortey, L. N., and Ghartey-Kwansah, G. (2022). Low incidence of COVID-19 case severity and mortality in Africa; could malaria co-infection provide the missing link? BMC Infect. Dis. 22:78. doi: 10.1186/s12879-022-07064-4
Page, K. R., Jedlicka, A. E., Fakheri, B., Noland, G. S., Kesavan, A. K., Scott, A. L., et al. (2005). Mycobacterium-induced potentiation of type 1 immune responses and protection against malaria are host specific. Infect. Immun. 73, 8369–8380. doi: 10.1128/IAI.73.12.8369-8380.2005
Pantaleo, G., and Koup, R. A. (2004). Correlates of immune protection in HIV-1 infection: what we know, what we don’t know, what we should know. Nat. Med. 10, 806–810. doi: 10.1038/nm0804-806
Parra, M., Derrick, S. C., Yang, A., Tian, J., Kolibab, K., Oakley, M., et al. (2011). Malaria infections do not compromise vaccine-induced immunity against tuberculosis in mice. PLoS One 6:e28164. doi: 10.1371/journal.pone.0028164
Parveen, N., and Bhanot, P. (2019). Babesia microti-Borrelia Burgdorferi coinfection. Pathogens 8:117. doi: 10.3390/pathogens8030117
Pikoulas, A., Piperaki, E. T., Spanakos, G., Kallianos, A., Mparmparousi, D., Rentziou, G., et al. (2022). Visceral leishmaniasis and COVID-19 coinfection – A case report. IDCases 27:e01358. doi: 10.1016/j.idcr.2021.e01358
Pott, H., and Castelo, A. (2013). Isolated cerebellar toxoplasmosis as a complication of HIV infection. Int. J. STD AIDS 24, 70–72. doi: 10.1258/ijsa.2012.012189
Praharaj, I., Platts-Mills, J. A., Taneja, S., Antony, K., Yuhas, K., Flores, J., et al. (2019). Diarrheal etiology and impact of coinfections on rotavirus vaccine efficacy estimates in a clinical trial of a monovalent human-bovine (116E) Oral rotavirus vaccine, Rotavac, India. Clin. Infect. Dis. 69, 243–250. doi: 10.1093/cid/ciy896
Pukrittayakamee, S., Viravan, C., Charoenlarp, P., Yeamput, C., Wilson, R. J., and White, N. J. (1994). Antimalarial effects of rifampin in plasmodium vivax malaria. Antimicrob. Agents Chemother. 38, 511–514. doi: 10.1128/AAC.38.3.511
Radwanska, M., Guirnalda, P., De Trez, C., Ryffel, B., Black, S., and Magez, S. (2008). Trypanosomiasis-induced B cell apoptosis results in loss of protective anti-parasite antibody responses and abolishment of vaccine-induced memory responses. PLoS Pathog. 4:e1000078. doi: 10.1371/journal.ppat.1000078
Raham, T. F. (2021). Influence of malaria endemicity and tuberculosis prevalence on COVID-19 mortality. Public Health 194, 33–35. doi: 10.1016/j.puhe.2021.02.018
Rappelli, P., Addis, M. F., Carta, F., and Fiori, P. L. (1998). Mycoplasma hominis parasitism of trichomonas vaginalis. Lancet 352:1286. doi: 10.1016/S0140-6736(98)00041-5
Rigaud, T., Perrot-Minnot, M. J., and Brown, M. J. (2010). Parasite and host assemblages: embracing the reality will improve our knowledge of parasite transmission and virulence. Proc. Biol. Sci. 277, 3693–3702. doi: 10.1098/rspb.2010.1163
Robert-Gangneux, F., and Darde, M. L. (2012). Epidemiology of and diagnostic strategies for toxoplasmosis. Clin. Microbiol. Rev. 25, 264–296. doi: 10.1128/CMR.05013-11
Robinson, T. M., Nelson, R., Artis, D., Scott, P., and Boyer, J. D. (2004). Experimental Leishmania major infection suppresses HIV-1 DNA vaccine induced cellular immune response. Cells Tissues Organs 177, 185–188. doi: 10.1159/000079992
Rodriguez, C. (2020). The global helminth belt and Covid-19: the new eosinophilic link. Qeios. doi: 10.32388/IWKQH9.2
Rogers, N. J., Hall, B. S., Obiero, J., Targett, G. A., and Sutherland, C. J. (2000). A model for sequestration of the transmission stages of plasmodium falciparum: adhesion of gametocyte-infected erythrocytes to human bone marrow cells. Infect. Immun. 68, 3455–3462. doi: 10.1128/IAI.68.6.3455-3462.2000
Rogers, K. J., Shtanko, O., Vijay, R., Mallinger, L. N., Joyner, C. J., Galinski, M. R., et al. (2020). Acute plasmodium infection promotes interferon-gamma-dependent resistance to Ebola virus infection. Cell Rep. 30:e 4044, 4041–4051.e4. doi: 10.1016/j.celrep.2020.02.104
Rooth, I. B., and Bjorkman, A. (1992). Suppression of plasmodium falciparum infections during concomitant measles or influenza but not during pertussis. Am. J. Trop. Med. Hyg. 47, 675–681. doi: 10.4269/ajtmh.1992.47.675
Rowe, J., Macaubas, C., Monger, T. M., Holt, B. J., Harvey, J., Poolman, J. T., et al. (2000). Antigen-specific responses to diphtheria-tetanus-acellular pertussis vaccine in human infants are initially Th2 polarized. Infect. Immun. 68, 3873–3877. doi: 10.1128/IAI.68.7.3873-3877.2000
Rurangirwa, F. R., Musoke, A. J., Nantulya, V. M., and Tabel, H. (1983). Immune depression in bovine trypanosomiasis: effects of acute and chronic Trypanosoma congolense and chronic Trypanosoma vivax infections on antibody response to Brucella abortus vaccine. Parasite Immunol. 5, 267–276. doi: 10.1111/j.1365-3024.1983.tb00743.x
Schietinger, A., and Greenberg, P. D. (2014). Tolerance and exhaustion: defining mechanisms of T cell dysfunction. Trends Immunol. 35, 51–60. doi: 10.1016/j.it.2013.10.001
Schmid-Hempel, P. (2009). Immune defence, parasite evasion strategies and their relevance for ‘macroscopic phenomena’ such as virulence. Philos. Trans. R. Soc. Lond. Ser. B Biol. Sci. 364, 85–98. doi: 10.1098/rstb.2008.0157
Schwebke, J. R. (2005). Trichomoniasis in adolescents: a marker for the lack of a public health response to the epidemic of sexually transmitted diseases in the United States. J. Infect. Dis. 192, 2036–2038. doi: 10.1086/498221
Scott, C. P., Kumar, N., Bishai, W. R., and Manabe, Y. C. (2004). Short report: modulation of mycobacterium tuberculosis infection by plasmodium in the murine model. Am. J. Trop. Med. Hyg. 70, 144–148. doi: 10.4269/ajtmh.2004.70.144
Selin, L. K., Brehm, M. A., Naumov, Y. N., Cornberg, M., Kim, S. K., Clute, S. C., et al. (2006). Memory of mice and men: CD8+ T-cell cross-reactivity and heterologous immunity. Immunol. Rev. 211, 164–181. doi: 10.1111/j.0105-2896.2006.00394.x
Sevede, D., Doumbia, M., Kouakou, V., Djehiffe, V., Pineau, P., and Dosso, M. (2019). Increased liver injury in patients with chronic hepatitis and IgG directed against hepatitis E virus. EXCLI J. 18, 955–961. doi: 10.17179/excli2019-1827
Sharpe, R. T., Langley, A. M., Mowat, G. N., Macaskill, J. A., and Holmes, P. H. (1982). Immunosuppression in bovine trypanosomiasis: response of cattle infected with Trypanosoma congolense to foot-and-mouth disease vaccination and subsequent live virus challenge. Res. Vet. Sci. 32, 289–293. doi: 10.1016/S0034-5288(18)32382-8
Sileghem, M., Darji, A., Remels, L., Hamers, R., and De Baetselier, P. (1989). Different mechanisms account for the suppression of interleukin 2 production and the suppression of interleukin 2 receptor expression in Trypanosoma brucei-infected mice. Eur. J. Immunol. 19, 119–124. doi: 10.1002/eji.1830190119
Sileghem, M., and Flynn, J. N. (1992). Suppression of interleukin 2 secretion and interleukin 2 receptor expression during tsetse-transmitted trypanosomiasis in cattle. Eur. J. Immunol. 22, 767–773. doi: 10.1002/eji.1830220321
Simoes, M. L., Goncalves, L., and Silveira, H. (2015). Hemozoin activates the innate immune system and reduces plasmodium berghei infection in Anopheles gambiae. Parasit. Vectors 8:12. doi: 10.1186/s13071-014-0619-y
Simon, P., Boffa, G., Ang, K. S., and Menault, M. (1982). Polycythaemia in a haemodialyzed anephric patient with hepatitis. Demonstration of erythropoietin secretion (author’s transl). Nouv. Press. Med. 11, 1401–1403.
Smedman, L., Silva, M. C., Gunnlaugsson, G., Norrby, E., and Zetterstrom, R. (1986). Augmented antibody response to live attenuated measles vaccine in children with plasmodium falciparum parasitaemia. Ann. Trop. Paediatr. 6, 149–153. doi: 10.1080/02724936.1986.11748428
Sohrabi, Y., Dos Santos, J. C., Dorenkamp, M., Findeisen, H., Godfrey, R., Netea, M. G., et al. (2020). Trained immunity as a novel approach against COVID-19 with a focus on bacillus Calmette-Guerin vaccine: mechanisms, challenges and perspectives. Clin. Transl. Immunol. 9:e 1228. doi: 10.1002/cti2.1228
Sorvillo, F., Smith, L., Kerndt, P., and Ash, L. (2001). Trichomonas vaginalis, HIV, and African-Americans. Emerg. Infect. Dis. 7, 927–932. doi: 10.3201/eid0706.010603
Spina, C. A., Prince, H. E., and Richman, D. D. (1997). Preferential replication of HIV-1 in the CD45RO memory cell subset of primary CD4 lymphocytes in vitro. J. Clin. Invest. 99, 1774–1785. doi: 10.1172/JCI119342
Ssebambulidde, K., Segawa, I., Abuga, K. M., Nakate, V., Kayiira, A., Ellis, J., et al. (2020). Parasites and their protection against COVID-19- ecology or immunology? Med. Rxiv. doi: 10.1101/2020.05.11.20098053
Stewart, P. E., and Bloom, M. E. (2020). Sharing the ride: Ixodes scapularis symbionts and their interactions. Front. Cell. Infect. Microbiol. 10:142. doi: 10.3389/fcimb.2020.00142
Subauste, C. S., Wessendarp, M., Portilllo, J. A., Andrade, R. M., Hinds, L. M., Gomez, F. J., et al. (2004). Pathogen-specific induction of CD154 is impaired in CD4+ T cells from human immunodeficiency virus-infected patients. J. Infect. Dis. 189, 61–70. doi: 10.1086/380510
Swanson, S. J., Neitzel, D., Reed, K. D., and Belongia, E. A. (2006). Coinfections acquired from ixodes ticks. Clin. Microbiol. Rev. 19, 708–727. doi: 10.1128/CMR.00011-06
Tabel, H., Wei, G., and Shi, M. (2008). T cells and immunopathogenesis of experimental African trypanosomiasis. Immunol. Rev. 225, 128–139. doi: 10.1111/j.1600-065X.2008.00675.x
Takem, E. N., Roca, A., and Cunnington, A. (2014). The association between malaria and non-typhoid salmonella bacteraemia in children in sub-Saharan Africa: a literature review. Malar. J. 13:400. doi: 10.1186/1475-2875-13-400
Tamomh, A. G., Agena, A. M., Elamin, E., Suliman, M. A., Elmadani, M., Omara, A. B., et al. (2021). Prevalence of cryptosporidiosis among children with diarrhoea under five years admitted to Kosti teaching hospital, Kosti City, Sudan. BMC Infect. Dis. 21:349. doi: 10.1186/s12879-021-06047-1
Tangie, E., Walters, A., Hsu, N. J., Fisher, M., Magez, S., Jacobs, M., et al. (2022). BCG-mediated protection against M. tuberculosis is sustained post-malaria infection independent of parasite virulence. Immunology 165, 219–233. doi: 10.1111/imm.13431
U.S. Department of Health and Human Services (2018). Report of the other tick-borne diseases and co-infections subcommittee to the tick-borne disease working group, HHS.Gov. U.S. Department of Health and Human Services.
Usen, S., Milligan, P., Ethevenaux, C., Greenwood, B., and Mulholland, K. (2000). Effect of fever on the serum antibody response of Gambian children to Haemophilus influenzae type b conjugate vaccine. Pediatr. Infect. Dis. J. 19, 444–449. doi: 10.1097/00006454-200005000-00010
Vetter, V., Denizer, G., Friedland, L. R., Krishnan, J., and Shapiro, M. (2018). Understanding modern-day vaccines: what you need to know. Ann. Med. 50, 110–120. doi: 10.1080/07853890.2017.1407035
Vilaplana, C., Prats, C., Marzo, E., Barril, C., Vegue, M., Diaz, J., et al. (2014). To achieve an earlier IFN-gamma response is not sufficient to control mycobacterium tuberculosis infection in mice. PLoS One 9:e100830. doi: 10.1371/journal.pone.0100830
Wait, L. F., Dobson, A. P., and Graham, A. L. (2020). Do parasite infections interfere with immunisation? A review and meta-analysis. Vaccine 38, 5582–5590. doi: 10.1016/j.vaccine.2020.06.064
Walk, J., de Bree, L. C. J., Graumans, W., Stoter, R., van Gemert, G. J., van de Vegte-Bolmer, M., et al. (2019). Outcomes of controlled human malaria infection after BCG vaccination. Nat. Commun. 10:874. doi: 10.1038/s41467-019-08659-3
Walsh, J. A. (1989). Disease problems in the third world. Ann. N. Y. Acad. Sci. 569, 1–16. doi: 10.1111/j.1749-6632.1989.tb27354.x
Wang, H. C., Dann, S. M., Okhuysen, P. C., Lewis, D. E., Chappell, C. L., Adler, D. G., et al. (2007). High levels of CXCL10 are produced by intestinal epithelial cells in AIDS patients with active cryptosporidiosis but not after reconstitution of immunity. Infect. Immun. 75, 481–487. doi: 10.1128/IAI.01237-06
Wang, R. J., Li, J. Q., Chen, Y. C., Zhang, L. X., and Xiao, L. H. (2018). Widespread occurrence of cryptosporidium infections in patients with HIV/AIDS: epidemiology, clinical feature, diagnosis, and therapy. Acta Trop. 187, 257–263. doi: 10.1016/j.actatropica.2018.08.018
Wang, Z. D., Wang, S. C., Liu, H. H., Ma, H. Y., Li, Z. Y., Wei, F., et al. (2017). Prevalence and burden of toxoplasma gondii infection in HIV-infected people: a systematic review and meta-analysis. Lancet HIV 4, e177–e188. doi: 10.1016/S2352-3018(17)30005-X
Wang, L., Zhang, H., Zhao, X., Zhang, L., Zhang, G., Guo, M., et al. (2013). Zoonotic cryptosporidium species and Enterocytozoon bieneusi genotypes in HIV-positive patients on antiretroviral therapy. J. Clin. Microbiol. 51, 557–563. doi: 10.1128/JCM.02758-12
Wang, R., Zhang, X., Zhu, H., Zhang, L., Feng, Y., Jian, F., et al. (2011). Genetic characterizations of cryptosporidium spp. and giardia duodenalis in humans in Henan, China. Exp. Parasitol. 127, 42–45. doi: 10.1016/j.exppara.2010.06.034
Welker, Y., Molina, J. M., Poirot, C., Ferchal, F., Decazes, J. M., Lagrange, P., et al. (1993). Interaction between human immunodeficiency virus and toxoplasma gondii replication in dually infected monocytoid cells. Infect. Immun. 61, 1596–1598. doi: 10.1128/iai.61.4.1596-1598.1993
Welsh, R. M., and Selin, L. K. (2002). No one is naive: the significance of heterologous T-cell immunity. Nat. Rev. Immunol. 2, 417–426. doi: 10.1038/nri820
Wilairatana, P., Masangkay, F. R., Kotepui, K. U., Milanez, G. J., and Kotepui, M. (2021). Prevalence and characteristics of malaria among COVID-19 individuals: A systematic review, meta-analysis, and analysis of case reports. PLoS Negl. Trop. Dis. 15:e0009766. doi: 10.1371/journal.pntd.0009766
Williamson, W. A., and Greenwood, B. M. (1978). Impairment of the immune response to vaccination after acute malaria. Lancet 1, 1328–1329. doi: 10.1016/s0140-6736(78)92403-0
Wolday, D., Gebrecherkos, T., Arefaine, Z. G., Kiros, Y. K., Gebreegzabher, A., Tasew, G., et al. (2021). Effect of co-infection with intestinal parasites on COVID-19 severity: A prospective observational cohort study. E Clin. Med. 39:101054. doi: 10.1016/j.eclinm.2021.101054
Woodford, J., Sagara, I., Diawara, H., Assadou, M. H., Katile, A., Attaher, O., et al. (2022). Recent malaria does not substantially impact COVID-19 antibody response or rates of symptomatic illness in communities with high malaria and COVID-19 transmission in Mali, West Africa. Front. Immunol. 13:959697. doi: 10.3389/fimmu.2022.959697
World Health Organization (2015). “Guidelines for the treatment of malaria ” 3d ed. (Geneva: World Health Organization).
World Health Statistics (2022). “Monitoring health for the SDGs, sustainable development goals ”. (Geneva: World Health Organization).
Wormser, G. P., McKenna, D., Scavarda, C., Cooper, D., El Khoury, M. Y., Nowakowski, J., et al. (2019). Co-infections in persons with early Lyme disease, New York, USA. Emerg. Infect. Dis. 25, 748–752. doi: 10.3201/eid2504.181509
Xiao, L., Fayer, R., Ryan, U., and Upton, S. J. (2004). Cryptosporidium taxonomy: recent advances and implications for public health. Clin. Microbiol. Rev. 17, 72–97. doi: 10.1128/CMR.17.1.72-97.2004
Keywords: protozoa, bacterial infection, co-infection, immune response, vaccine efficacy
Citation: Akoolo L, Rocha SC and Parveen N (2022) Protozoan co-infections and parasite influence on the efficacy of vaccines against bacterial and viral pathogens. Front. Microbiol. 13:1020029. doi: 10.3389/fmicb.2022.1020029
Edited by:
Laurent Rénia, Nanyang Technological University, SingaporeReviewed by:
Jyoti Bhardwaj, Indiana University Hospital, United StatesPanagiotis Karanis, University of Nicosia, Cyprus
Copyright © 2022 Akoolo, Rocha and Parveen. This is an open-access article distributed under the terms of the Creative Commons Attribution License (CC BY). The use, distribution or reproduction in other forums is permitted, provided the original author(s) and the copyright owner(s) are credited and that the original publication in this journal is cited, in accordance with accepted academic practice. No use, distribution or reproduction is permitted which does not comply with these terms.
*Correspondence: Nikhat Parveen, UGFydmVlbmlAbmptcy5ydXRnZXJzLmVkdQ==
†These authors have contributed equally to this work