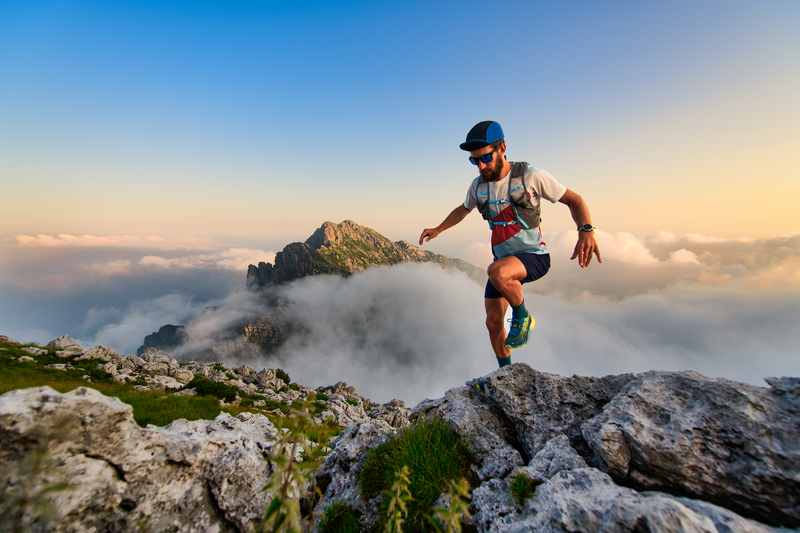
95% of researchers rate our articles as excellent or good
Learn more about the work of our research integrity team to safeguard the quality of each article we publish.
Find out more
ORIGINAL RESEARCH article
Front. Microbiol. , 18 November 2022
Sec. Evolutionary and Genomic Microbiology
Volume 13 - 2022 | https://doi.org/10.3389/fmicb.2022.1009919
Microbial symbionts can influence a myriad of insect behavioral and physiological traits. However, how microbial communities may shape or be shaped by insect interactions with plants and neighboring species remains underexplored. The fig-fig wasp mutualism system offers a unique model to study the roles of microbiome in the interactions between the plants and co-habiting insects because a confined fig environment is shared by two fig wasp species, the pollinator wasp (Eupristina altissima and Eupristina verticillata) and the cheater wasp (Eupristina sp1 and Eupristina sp2). Here, we performed whole genome resequencing (WGS) on 48 individual fig wasps (Eupristina spp.) from Yunnan, China, to reveal the phylogenetic relationship and genetic divergence between pollinator and congeneric cheater wasps associated with the Ficus trees. We then extracted metagenomic sequences to explore the compositions, network structures, and functional capabilities of microbial communities associated with these wasps. We found that the cheaters and pollinators from the same fig species are sister species, which are highly genetically divergent. Fig wasps harbor diverse but stable microbial communities. Fig species dominate over the fig wasp genotype in shaping the bacterial and fungal communities. Variation in microbial communities may be partially explained by the filtering effect from fig and phylogeny of fig wasps. It is worth noting that cheaters have similar microbial communities to their sister pollinators, which may allow cheaters to coexist and gain resources from the same fig species. In terms of metabolic capabilities, some bacteria such as Desulfovibrio and Lachnospiraceae are candidates involved in the nutritional uptake of fig wasps. Our results provide novel insights into how microbiome community and metabolic functions may couple with the fig-wasp mutualistic systems.
Microorganisms represent a predominant component of biodiversity and help maintain the function and stability of ecological systems (Weinbauer and Wenderoth, 2002; Maron et al., 2018; Escalas et al., 2019; Ratzke et al., 2020). Thus, exploring the composition and function of microbial communities in ecosystems contributes to understanding of ecological factors shaping biodiversity and species interactions (Zilber-Rosenberg and Rosenberg, 2008; Gibbons and Gilbert, 2015; Nazir et al., 2019), particularly those associated with herbivore insects and plants (Mithöfer and Boland, 2012; Casteel and Hansen, 2014; Zhu et al., 2014; Wielkopolan and Obrępalska-Stęplowska, 2016). For instance, microorganisms associated with herbivore insects can modify plant phytohormones and antiherbivore defenses (Casteel and Hansen, 2014). Microbial manipulation of plant chemistry and immunity could also affect plant susceptibility to herbivore insects (Lazebnik et al., 2014; Koduru, 2021). These examples demonstrate that the microorganisms can play critical roles in modulating plant-herbivore interactions.
In addition, insect-associated microorganisms could drive insect diversification and speciation (Vavre and Kremer, 2014). For instance, Wolbachia are widely associated with insects and can cause hybrid incompatibility in many species (Bordenstein et al., 2001; Serbus et al., 2008; Vavre and Kremer, 2014). Moreover, gut bacteria associated with Drosophila have been shown to affect the kin recognition of host insects and may change the reproductive investment of insects (Lizé et al., 2014). Co-speciation between host insects and their gut symbionts implies that parallel evolutionary processes might exist in the insects-bacteria system (Groussin et al., 2020). However, research has mainly focused on the crops and generalist insects.
Here, we explored the microbial diversity and potential metabolic functions in fig-wasp mutualism, which is a classic example of coevolution and obligatory pollinator system. Each fig species is pollinated by a specific pollinating fig wasp species (Janzen, 1979; Herre, 1989) and the fig wasps solely oviposit and develop within the figs (Ware and Compton, 1994). Generally, when the figs are in the receptive stage, the narrow ostiole is opened to allow the pollinator fig wasps access (Ware and Compton, 1994; Souza et al., 2015), and after their entry, the ostiole is closed (Nefdt and Compton, 1996). The pollinator fig wasps lay eggs or pollination inside of figs (mature offspring will fly away the maternal figs and enter a new round cycle), which is a relatively confined and stable environment for the development of fig wasps. The specific micro-habitat reduces the uncertain disturbances from surrounding environment and provides a unique model for studying the associated microorganisms and their potential roles in driving plant-insect interactions in obligatory mutualism system. However, due to the extremely tiny size of the fig wasps, so far, there was no further study on the specific regions of the microbe communities associated, and even fewer reports about the microbe community on the fig wasp. For a long time, the relationship between the fig and pollinator wasp was thought to be strictly species-specific. Namely, each specific fig wasp species provides one fig species with the pollination service, and in turn, the fig wasp completes its life cycle in the specific fig species (Wiebes, 1979; Rasplus, 1996). Moreover, cheater wasp species can also exist (Peng et al., 2008; Jandér and Herre, 2010; Zhang et al., 2019). The cheater species were found in two fig-wasp systems in Yunnan Province, China: Eupristina altissima (Hymenoptera: Chalcidoidea; pollinator) and Eupristina sp1 (Hymenoptera: Chalcidoidea; cheater) on Ficus altissima Blume (Ficus, Moraceae; Peng et al., 2008). Eupristina verticillata (pollinator) and Eupristina sp2 (cheater) on Ficus microcarpa Linnaeus (Martinson et al., 2014; Zhang et al., 2019, 2021). The pollinator and their pairs cheater are sister species on per fig species, and the coexistence between pollinator and cheater is common (Peng et al., 2008; Zhang et al., 2021). The diversity and functions of microorganisms associated with the different pollinator and wasp species are largely unknown.
In this study, we conducted illumina sequencing on various samples of fig-wasp systems to reveal the phylogenetic relationship and genetic divergence between pollinator and congeneric cheater wasps associated with the Ficus trees. We extracted microbial reads not mapped to the wasp genomes to explore (1) the diversity, compositions, and potential functions of microbial community associated with each fig wasp species; (2) microbial co-occurrence network patterns; (3) the assemblage patterns of microbial communities associated with pollinator and cheater fig wasps. The genomic data provides new insights on potential contributions of microbiome to fig-fig wasp mutualism and coevolution.
From March to October 2020, figs (nearly phase that fig wasp offspring will emerge from figs) of Ficus microcarpa and F. altissima, were sampled at Xishuangbanna and nearby areas in Yunnan, China (Supplementary Table S1; Figures 1A,B). They mainly from three locations: Menglun town (20 individuals in total), Lushui (21 individuals in total), Chuxiong (7 individuals) with the distance of 500 and 280 kilometers. In order to get a well representative sampling of the fig wasps, for each location we sampled two crops, and we random picked figs covered several fig trees, we collected only one female pollinator or cheater wasp from each fig to ensure that each individual came from different foundress females. We cleaned the fig wasps with sterile water, and identified the pollinator and cheater species under a light microscope and stored them individually into tubes with 100% ethanol at −20°C. All the materials used for the sample collection were aseptically treated to avoid contamination. Genomic DNA was extracted from the whole body of each fig wasps using the QIAamp DNA Micro Kit (Qiagen, Hilden, Germany), and paired-end sequencing libraries with an insert size of 350 bp were constructed according to the Illumina library preparation protocol. Sequencing was carried out on the Illumina HiSeq 2,500 platform (Illumina Inc., San Diego, United States) with a target coverage of 20×. The sequencing data have been deposited in the NCBI, associated BioProject ID is PRJNA893401.
Figure 1. Phylogenetic, population genetic, and microbial similarity analysis of four fig wasp species. (A) Ficus altissima and its associated fig wasp; (B) F. microcarpa and its associated fig wasp. (C) Faststructure plot depicting ancestry proportions of each species in K = 5. (D) Genetic clusters identified based on the PC1 and PC2, PC2 and PC3 from the smartPCA analysis. (E) NJ tree constructed using the SNPhylo, the colors represented each species. (F) The Non-metric multidimensional scaling (NMDS) ordination analysis based on the composition of microbial communities. (Left) the Non-metric multidimensional scaling ordination (stress = 0.15, p < 0.01) produced with the Bray–Curtis dissimilarity distance metric. (Right) the same results of Left represent from different host figs. Fa_C represents the cheater of F. altissima; Fa_P represents the pollinator of F. altissima; Fm_C represents the cheater of F. microcarpa; Fm_P represents the pollinator of F. microcarpa.
The quality of raw sequence reads from fig wasps was assessed using FastQC.1 For raw sequencing reads, we used Trimmomatic (Bolger et al., 2014) to remove adapter sequences and cut off bases from either the start or the end of reads when the base quality was <20 We discarded the reads with fewer than 36 bases remaining after trimming. We then mapped all reads to the Eupristina verticillata reference genome (Zhang et al., 2020) with default parameters implemented in bwa-0.7.10 using the BWA-MEM algorithm (Li, 2013). Local realignment, and simultaneous SNP and indel discovery was performed using RealignerTargetCreator, IndelRealigner, and the HaplotypeCaller in GATK v3.2.2 (DePristo et al., 2011). We used MarkDuplicates in Picard2 to remove the potential PCR duplicates. After that, genotypes (in gVCF files) of all individuals were joined together by using the default hard filtering parameters as prescribed by GATK v3.2.2 best practices. Only sites with mapping quality ≥30 and base quality ≥30 were considered for calling variants in HaplotypeCaller (Supplementary Table S1). We used Vcftools (Danecek et al., 2011) to further filter low quality SNPs according to the following criteria: (1) only biallelic SNPs that were at least 5 bp away from any indel were retained; (2) genotypes with a genotype quality score (GQ) < 20, mapping quality score (MQ) < 40, and sites with sequencing depths (DP) < 10 were treated as missing; (3) SNPs with a genotype missing call rate ≥ 20% across the whole sample set were removed; (4) filtered the sites with minor alleles frequency (MAF) <0.05 sites, and removed the site where the proportion of heterozygous genotypes was >70% across the whole sample set. After these filtering steps, 397,502 SNPs were retained from all individuals for genetic analyses. To investigate the genetic structure of the fig wasps, we conducted three sets of analyses. (1) We ran fastSTRUCTURE (model-based clustering algorithm) with K = 1–20 and repeated the process 20 times with random seeds (Raj et al., 2014). Since linkage disequilibrium might violate the statistical assumptions involved in this test, we take one SNP from every 100 bp using the - thin in vcftools resulting in a dataset with 74,396 SNPs. (2) We assessed the distribution of genetic variance by principal component analysis (smartPCA) as implemented in EIGENSOFT version 6.0 (Patterson et al., 2006). (3) We constructed Neighbor-Joining (NJ) tree using SNPhylo (Lee et al., 2014), and with one individual from Kradibia gibbosae (pollinator of F. tinctoria) as the outgroup. Additionally, in order to check the genetic relationships of the fig wasps in this study with the samples in Hainan reported in previous study (Sun et al., 2011), we downloaded the 24 COI gene sequences of Eupristina verticillata from the paper Sun et al., 2011 (GenBankID:HM582244-HM582267) and chosen E. koningsbergeri (MK543378) and E. altissima (MK559358) as outgroups. We de novo assembled 23 mitochondrial genomes of E. verticillata using GetOrganelle (Jin et al., 2020), then extracted the COI gene in Geneious (Kearse et al., 2012), using BS-18 (HM582254) as the reference. We deposited the 23 COI genes in the National Center for Biotechnology Information GenBank under the accession number GenBank OP715733-OP715755. We reconstructed a maximum-likelihood (ML) phylogenetic tree based on the COI genes of a total of 47 E. verticillata and two outgroups (E. koningsbergeri and E. altissima). We used MAFFT (Katoh and Standley, 2013) to align 49 COI genes and ambiguously aligned fragments were removed by Gblocks (Talavera and Castresana, 2007). We used modelFinder (Kalyaanamoorthy et al., 2017) to select the best-fit nucleotide substitution model: TIM + F + I, then reconstructed ML tree by IQ-TREE (Nguyen et al., 2015). We inferred the support for the phylogenetic tree by bootstrapping with 10,000 ultrafast bootstraps (Minh et al., 2013).
The analysis pipeline of the bacterial and fungal communities was composed of three steps. First, we used bwa v0.7.173 to get the unmapped raw reads using the completely assembled reference genome of E. verticillata (Zhang et al., 2020). The unmapped reads were extracted from the mapping result files and estimated the quality reads via QualiMap v2.2.1 (Okonechnikov et al., 2016) and then individually paired-end assembled using Pandaseq (Masella et al., 2012). Finally, based on the blast v2.12.04 with an expect value of <10 e−10, we aligned the unmapped reads to the SILVA database5 and Ribosomal Database Project6 to obtain the bacterial and fungal identifications. The blast outputs were performed analyses related to microbial diversity and function.
To estimate the similarity of microbial communities in the different samples, we conducted the non-parametric analysis of similarities analysis (ANOSIM), Non-metric multidimensional scaling (NMDS) with the Bray-Curtis distance and hierarchical clustering analysis with the ward.D2 algorithm (Murtagh and Legendre, 2014) on Euclidean distances. To characterize the corresponding relation in microbial communities and the phylogenetic structure of fig wasps, we visualized the topology structures of clusters of microbial communities and phylogenetic tree of fig wasps. Subsequently, we detected significance of the corresponding relation bycomparing to the null model (algorithm: quasiswap) based on 1,000 permutation samples in PACo package (Balbuena et al., 2013). Linear discriminant analysis effect size (LEfSe)7 was conducted to identify marker features that differentiate between from the pollinator and cheater fig wasp microbiomes, the different groups at the species level were determined using to explore whether the Wolbachia strains from the pollinator and cheater fig wasps show are genetically distinct, we conducted cluster analysis according to the similarity of Wolbachia compositions detected in the fig wasps associated with F. microcarpa.
To decipher the alpha-diversity and abundance of the fig wasp-associated bacterial and fungal communities, we calculated Shannon-Wiener and Chao 1 diversity indices of the fig wasp-associated microbial communities using the Vegan package (Oksanen et al., 2018). The compositions at the phylum and genus levels were visualized by stacked column charts. To evaluate the sampling effort, the microbial rarefaction and prediction curves were calculated by species richness in iNEXT (Hsieh et al., 2016) package.
To explore potential co-occurrence patterns and interactions in fig wasps-associated microbial communities, we performed network analysis using igraph package in the R programming environment (Csardi and Nepusz, 2006; R Development Core Team, 2018). Combining the fungal and bacterial communities from the same samples, we calculated the Spearman’s correlation coefficients and p value. Correlation coefficient lower than 0.8 and p value larger than 0.05 were filtered to generate a robust co-occurrence network. To describe the structure and parameters of the networks, we measured several network properties including connectance, average degree, diameter, modularity, and robustness. Connectance is the proportion of observed links among all possible interactions in the network, which descripts the complexity extent of network (Newman and Girvan, 2004; Landi et al., 2018). Average degree means the mean value of edges each node in the microbial network (Fornito et al., 2016). Diameter is defined as the largest length of the shortest paths between all pairs of vertices, and a measure of efficiency for the transmission resources across entire network (Luke, 2015). Modularity reflects the clustering extents to which compositions evaluates the clustering in microbial network (Newman et al., 2006). The modularity value ranges from −0.5 to 1, the value closer to 1 represents the network more clustering divided into density subgroups (Luke, 2015). Robustness evaluates the stability and resistance facing some species loss in the network (Dunne et al., 2002; Landi et al., 2018). Further, to evaluate the statistical significance of links in networks observed in samples, we compared the observed values to the null model generated by 500 times randomized network using the r2dtable algorithm (Dormann et al., 2009).
The functional annotation of fig wasp-associated fungi was performed using the FUNGuild (Nguyen et al., 2016) Guilds_v1.1.py script. To consider the confidence scores of predictions, we only included the “highly probable” and “probable” two identification levels in our function guilds. For the fig wasp-associated bacteria, we conducted the Tax4Fun2 to predict the bacterial metabolic functions (Wemheuer et al., 2020), and the FAPROTAX to detect the ecological function by collapse_table.py (Louca et al., 2016). The functional composition and enrichment were visualized with the bar plots and heatmaps.
The cross-validation procedure from the fastSTRUCTURE analysis identified K = 5 as the best parameter for the number of clusters in the samples. The five genetic clusters fit well with the species identification based on prior species category (Figure 1C). The four fig wasps were genetically independent and no genetic admix was observed between the pollinator and cheater wasps, and no further subgroups was appeared within each wasp species (Figure 1C) The genetic structure among the four fig wasp species was also supported by the Principal Component Analysis (PCA) and phylogenetic tree (Figures 1D,E). Together, the genetic analyses showed that individuals from each species showed a consistent genetic identity and the microbe compositions will not be affected by the genetic variation within species.
The ML tree based on COI gene of the pollinator and cheater wasps in this study showed that the pollinator wasps clustered with clade 1 (identified in Hainan islands), whereas the cheater wasps clustered with. Clade 2 (identified in Hainan; Supplementary Figure S1), and no wasp species in this study clustered with clade 3 (a unique cryptic species group in Hainan).
The NMDS plot and ANOSIM analysis reveal significant microbiome segregation among the different fig wasps, largely driven by the fig species (Figure 1F; stress = 0.15, p < 0.01) Microbial communities of F. altissima-associated wasps clustered separately from F. microcarpa-associated wasps. However, microbial communities are not significantly different between the cheater and pollinator wasps from the same host fig species (F. altissima: stress = 0.18, p = 0.06; F. microcarpa: stress = 0.11, p = 0.28). The same situation was also revealed by the co-phylogeny of the fig wasp and microbial communities. The NJ tree of the fig wasps revealed clearly species-specific clusters. However, the cluster dendrogram of microbial communities showed two clusters, the first one cluster consisting primarily of the microbial communities associated with the cheater and pollinator from F. microcarpa, and the second cluster consisting primarily of the microbial communities associated with the wasps from F. altissima, but admixed with two cheaters and pollinators from F. microcarpa (Figure 2). The certain congruence branches also were supported by the corresponding relation test, in which the microbial communities and phylogenetic topology have significant interaction intimacy (m2XY = 0.72, p < 0.001, n = 1,000) than the null model.
Figure 2. Left: the cluster dendrogram analysis based on the ward.D2 method using Euclidean distances of compositions of microbial communities in samples. The tip labels in the dendrogram are the sample codes. Right: the phylogenetic structure of fig wasps, (the corresponding relation: m2XY = 0.72, p < 0.001, n = 1,000). Fa_C represents the cheater of F. altissima; Fa_P represents the pollinator of F. altissima; Fm_C represents the cheater of F. microcarpa; Fm_P represents the pollinator of F. microcarpa.
The results from LEfSe analysis showed that Saccharomycetaceae, Cyanobacteria Chloroplast Nicotiana tomentosiformis, Cyanobacteria Chloroplast Gossypium hirsutum, and Cyanobacteria Chloroplast Phaseolus acutifolius were significantly more abundant in the pollinator wasps of F. altissima and we observed that 21 groups such as Proteobacteria, Enterobacteriales, Sphingobacteriales, Vibrio, and Streptococcus were significantly associated with the cheater of F. altissima (Supplementary Figure S2 A). Furthermore, 10 groups such as Burkholderiaceae, Rickettsiales Mitochondria Hordeum, Prevotellaceae, and Wolbachia were significantly more abundant in the cheater wasps of F. microcarpa (Supplementary Figure S2 B), and 35 groups were significantly abundant in the pollinator wasps of F. microcarpa.
Interestingly, Wolbachia was detected in F. microcarpa-associated fig wasps but not in F. altissima-associated fig wasps. Cluster analysis revealed two clusters, the first including 22.22 percent pollinators and 77.78 percent cheaters of F. microcarpa and the second containing 66.67 percent pollinators and 33.33 percent cheaters (Supplementary Figure S3).
In terms of fungal communities, alpha diversity indices were generally not different among the different fig wasps (Supplementary Figures S4 A,B). The Shannon diversity index (mean value) ranges from 1.12 (cheater of F. altissima) to 1.59 (pollinator of F. microcarpa), whereas the Chao 1 diversity index was not significant differences among samples. The bacterial alpha diversity also showed minimal differences among the fig wasp species (Supplementary Figures S4 C,D). The samples of F. altissima pollinator had a significant lower Chao 1 index than those samples from F. microcarpa. In terms of taxonomic composition, the fungal communities were dominated by 7 phyla: Ascomycota, Basidiomycota, Entomophthoromycota, Zygomycota, Cryptomycota, Chytridiomycota, and Glomeromycota (Figures 3A,B). The Ascomycota was the most abundant group (70.39–95.10%) followed by Basidiomycota (3.43–5.04%). The Saccharomyces was the dominant genus in the samples from cheater (65.11%), pollinator (69.85%) of F. altissima, and pollinator of F. microcarpa (23.03%). Whereas the Stereopsis genus was most prominent in the sample from cheater of F. microcarpa (40.99%).
Figure 3. The relative abundance of fungal and bacterial communities in four different samples from fig wasps-associated microbial communities. (A) Fungal compositions at phylum level. (B) Fungal compositions at genus level. (C) Bacterial compositions at phylum level. (D) Bacterial compositions at genus level. The percentage is the relative abundance, the values less than 3% were not shown in panel. Fa_C represents the cheater of F. altissima; Fa_P represents the pollinator of F. altissima; Fm_C represents the cheater of F. microcarpa; Fm_P represents the pollinator of F. microcarpa.
More bacterial phyla were detected and the top 5 were Proteobacteria, Firmicutes, Actinobacteria, Chloroflexi, and Acidobacteria (Figures 3C,D). The relative abundance of Proteobacteria was higher in cheaters (41.71%), pollinators (32.45%) of F. altissima, and pollinators of F. microcarpa (30.45%), whereas the dominant phylum was Firmicutes in cheaters of F. microcarpa (35.47%). At bacterial genus level, except for cheaters of F. microcarpa, Propionibacterium was the most abundant genus in all other samples. Wolbachia was only observed in cheaters (5.95%) and pollinators (8.52%) of F. microcarpa.
Moreover, the individual microbial compositions at phylum level were visualized (Supplementary Figures S5 A,B), indicating microbial compositions among samples were generally consistent. The rarefaction curves indicated that samples in this study did not reach the asymptotic platform (Supplementary Figures S6 A,B).
Across all networks from our samples that integrated the bacterial and fungal communities, the r2dtable algorithm showed that network links were non-random and mainly positive significant interactions (Supplementary Figure S7), which may reflect the microbial groups trend to cooperation with each other in microenvironment of fig. The network from the cheater wasps of F. altissima consisted of 228 nodes (genera), 1,333 positive edges and 1 negative edge. While the network in pollinator of F. altissima had 244 nodes and 1,006 positive interactions. The network from cheater of F. microcarpa was composed of 441 nodes, 6,649 positive links and 2 negative links, whereas 410 nodes and 2,745 positive links formed the network in pollinator from F. microcarpa. The co-occurrence network analysis showed that Ascomycota, Proteobacteria, and Firmicutes are the most abundant nodes, this is consistent with results from the relative abundance of microbial communities. For each co-occurrence network, the maximum connectance and robustness were 0.21 and 0.99, respectively (Table 1). Furthermore, the highest average degree was observed in microbial communities from cheater of F. microcarpa (35.10). Samples from cheater of F. microcarpa showed the lowest modularity (0.37) and the network diameters of samples from F. altissima were less than samples from F. microcarpa.
Based on the fungal guild classification identified by the FUNGuild, 7 ecological functional guilds were detected (Figure 4A). The guilds were comprised of wood saprotroph, undefined saprotroph, plant pathogen, ectomycorrhizal, arbuscular mycorrhizal, animal pathogen, and animal endosymbiont. The most abundant guild was undefined saprotroph, followed by animal pathogen. The least significant difference (LSD) test showed that the animal pathogen and plant pathogen guilds were significantly more abundant in the samples from pollinator of F. microcarpa than in samples from cheater of F. altissima. The remaining ecological functional guilds showed non-significant difference among the samples.
Figure 4. The function prediction of microbial communities. (A) Heatmap plot of fungal functional guilds produced by FUNGuild analysis. (B) Bar plots of the prediction Kyoto Encyclopedia of Genes and Genomes (KEGG) pathway level 1 obtained from Tax4Fun2 for each sample of bacterial community. (C) Heatmap of the functional abundances at the KEGG metabolic pathway level 2 obtained from Tax4Fun2 for each sample of bacterial community. The color bar reflects the value of functional abundance. The letters in each panel were calculated by the least significant difference (LSD) test, the same letter represents no differ significantly. The relative abundance of pathways with 0.05 cutoff (not shown). Fa_C represents the cheater of F. altissima; Fa_P represents the pollinator of F. altissima; Fm_C represents the cheater of F. microcarpa; Fm_P represents the pollinator of F. microcarpa.
All samples showed highly similar distribution patterns of the Kyoto Encyclopedia of Genes and Genomes (KEGG) pathways level 1 and 2 (Figures 4B,C). The bacterial communities in samples revealed that the predicted KEGG pathway level 1 includes metabolism, genetic information processing, environmental information processing, cellular processes, organismal systems, and human diseases. We set a cutoff of relative abundance > 0.05 to present the pathways in the results. At the KEGG metabolic pathway level 2, the pathways related to the metabolism (such as carbohydrate metabolism, energy metabolism and amino acid metabolism), genetic information processing, and environmental information processing were highly represented. No significant difference in KEGG pathways was detected among the different wasp bacterial communities. The ecological functions of bacteria via the FAPROTAX dataset showed that 74 functional groups were observed across the samples, with chemoheterotrophy, animal parasites or symbionts, aerobic chemoheterotrophy, and fermentation being the most abundant functional groups (Supplementary Figure S8).
In fig-fig wasp systems, coexisting cheaters and pollinators have similar morphological features but different pollination behavior. Whether these cheaters are phylogenetically unrelated from the pollinator or they evolved from the pollinator lineage is an controversial topic of research for biologists (Kong, 2014; Zhang et al., 2019). The first cheater case (Ceratosolen galili) found in Ficus sycomorus from Africa was due to the host plant shifting of fig wasps (Compton et al., 1991). Zhang et al. (2021) suggested that the cheaters and pollinators of F. micorcarpa and F. altissima were two distinct events but the cheater and pollinator from each host fig were closely related species, based on their mitochondria COI gene. However, this result was based on single gene evidence. In this study, the whole genome-wide sequence provides strong support that the cheaters and pollinators from the same fig species are sister species and are highly divergent. In nature, coexistence of cheaters and pollinators is also found in the yucca and yucca moth system (Pellmyr et al., 1996; Pellmyr and Leebens-Mack, 1999), where extensive hybridization serves as a general mechanism limiting the cheaters’ expansion (Segraves et al., 2005). However, no admixture between the cheaters and pollinators was found in our study, suggesting that they are completely reproductive-isolated. Our samples showed significant interspecific divergence but strong intraspecific genetic consistency in Xishuangbanna and the neighboring area.
The common bacterial phyla associated with insects are reported to be Protobacteria, Firmicutes, Bacteroidetes, and Actinobacteria (Warnecke et al., 2007; Colman et al., 2012; Jones et al., 2013; Receveur et al., 2020). Ascomycota is a particularly predominant phylum in fungal communities (Douglas, 2015; Sharma et al., 2018; Morales-Rodriguez et al., 2019). Our results also support the previous findings with common microorganisms associated with other insects, and all the common phyla mentioned above are detected in this study.
Moreover, our results revealed more diverse and complex composition of fig wasp-associated microbial communities than documented. A previous report based on unmapped raw genomic sequence data of Ceratosolen solmsi (pollinator of Ficus hispida) identified 158 genera of fungi belonging to two phyla: Ascomycota (92.3%) and Basidiomycota (7.7%; Niu et al., 2015a). Except for the consistent with the results on dominant two phyla, we detected extra five fungal phyla more than 200 genera in fungal communities associated with our fig wasp specimens. Furthermore, compared to the results from Niu et al. (2015b), we documented greater variation in bacterial composition. They found the bacterial communities consisted of 43 genera belonging to 6 phyla from four fig wasp species (Ceratosolen solmsi Mayr, Apocrypta bakeri Joseph, Philotrypesis pilosa Mayr, and Philotrypesis sp. Forster), which differ in their phylogenetic relationships and diets but coexist in Ficus hispida. Among them, the pollinator wasp C. solmsi is dominated by Protobacteria (65.3%) and Actinobacteria (23.9%) in the associated bacterial community, using the Amplified ribosomal DNA restriction analysis (ARDRA) method (Niu et al., 2015b). A total of more than 400 genera, representing 19 different bacterial phyla were collected across our fig wasp samples, whereas Protobacteria, Firmicutes, and Actinobacteria were the most abundant bacterial groups in different samples. Overall, we observed more microbial groups which have not been identified in previous reports on fig wasps, such as the Entomophthoromycota, Zygomycota, Chloroflexi, Cyanobacteria, and Nitrospirae.
We argue that these differences in microbial composition could be attributed to several reasons. Previous studies showed that ARDRA has a much lower resolution than high throughput sequencing that is more sensitive and effective and may fail to capture diverse and abundant microbial taxa (Smit et al., 1997; De Mandal et al., 2015; Bailón-Salas et al., 2017; Hu et al., 2021). The different sexes of wasps used in the different studies may also contribute to the different results. Niu et al. (2015a) only detected Ascomycota and Basidiomycota strains in fungi groups associated with male individuals, whereas females were sampled in this study. The female and male are dimorphic in morphology and behavior in fig wasps, generally, wingless male mate within their natal fig fruits and never leave, whereas winged males disperse to mate (Weiblen, 2002). However, females must fly away natal fig to search proper fig to start a new life cycle, which means high movement ability may provide females more chance to contact extra microbial groups.
Several studies have suggested that host genotype has a bigger influence than environment in shaping microbial communities (Brucker and Bordenstein, 2012; Sanders et al., 2014). In this study, we observed that microbial taxa significantly varied among samples from two different Ficus tree species. As an obligate mutualism system, the figs are associated with their specific pollinator and non-pollinating wasps (Cook and Segar, 2010), whereas only few non-pollinating wasp species can enter the syconium for oviposition (West et al., 1996; Peng et al., 2005). The host fig might influence the microbial community of the pollinator wasps within the fig syconium. In addition, even in the same syconia, the bacterial community was also affected by the phylogeny and diet structure of host wasp (Sun et al., 2011). The fig wasps in this study are all phytophagous, and both pollinator wasps Eupristina verticillata (host fig: F. microcapa) and Eupristina altissima (host fig: F. altissima) belong to the genus Eupristina (Agaonidae). They have been separated for at least 28.83 Mya (Cruaud et al., 2022), indicating bacterial communities might have introduced and diverged through the long evolutionary time scale. Thus, we indicate that the microbial communities of fig wasps were influenced by the environment (syconia of different fig species) and the phylogenetic relationship of the host fig wasps themselves.
However, fig species has more dominating influence than fig wasp genotype in shaping the microbial communities. The correlations with the cluster dendrograms of microbial community compositions and the NJ tree dendrograms of fig wasps further confirmed this idea. Although a few of Fm samples are clustered into the Fa cluster (Figure 2), this suggests that the microbial compositions associated with fig wasps may (not completely accurate) represent the evolutionary relationship of fig wasps and also be impacted by the figs. The microbial communities showed more similarities between the pollinator and cheater wasps from the same fig species, which can be attributed to three potential reasons: First, the figs play filtering roles in avoiding external disturbances. The pollinator and their pair cheater can be observed in one fig, which means they can have contact with one other, leading to the horizontal transmission of the microorganisms, though coexistence in the same syconia is considered rare (~9%; Peng et al., 2008; Zhang et al., 2019). Second, the divergence time between the cheater and pollinator was 3.13 Mya in the F. altissima and 0.91 Mya in the F. microcarpa, both were significantly shorter than the separation time between wasps associated with different host fig species (28.83 Mya; Jie Gao et al., unpublished work). The third possible factor is that the cheater may mimic the pollinator’s microbial composition, which helps cheater to hide against recognition of figs. Figs have the ability to abort syconia with too many non-pollinator wasp offspring, thereby sanctioning cheaters and ensuring better pollination services from pollinators. This means the host figs trend to allocate more resource to beneficial pollinators (Jandér and Herre, 2016). In turn, this similarity in microbial community may be critical to cheaters to gain more resources from figs. Research in Xishuangbanna demonstrated that local F. microcarpa lack the host sanctions (Zhang et al., 2021). We speculated that the microbial communities of pollinator wasps might interact with the fig to affect the chemical signals of figs and inhibit of host sanction because the fig wasps rely on volatile compounds for locating the host figs (Ware et al., 1993) Bacteria associated with oviposition resources have been shown to influence the oviposition preference of flies (Zheng et al., 2013). Furthermore, the fungal compositions are markedly diverse at different developmental stages of figs, especially, in the stages during and after entering pollinators, indicating that fungi may provide volatile cues to fig wasps to track figs (Martinson et al., 2012). The exact role of microorganisms in mediating the fig’s recognition and oviposition behaviors of fig wasp merits further investigation.
The low to nonexistent host sanctions on the non-pollinator wasps promote the evolution of cheaters, causing the wasp undergo considerable morphological and genomic evolution to adapt to the extreme environment in the closed syconium (Zhang et al., 2021). We observed that the cheater and pollinators both have a stable network structure of microbial community with non-random network and high positive relationships. This is interesting because the prevalence of competition interaction is typically higher than cooperative in microbial communities (Palmer and Foster, 2022). The positive interactions may hold the key for stable mutualism between the fig, fig wasp., and microbial communities (Barberán et al., 2012; Chow et al., 2014; Gould et al., 2018). However, we also found that the network parameters of microbial communities from F. altissima are not exactly the same for the parameters from F. microcarpa. For instance, the high average degree and low modularity were observed in microbial communities of cheaters from F. microcarpa, which represents that each node has more links with other nodes but communities with low clustering density. We argued that this situation is due to the low effective nodes, which was supported by visualization (Supplementary Figure S7). Moreover, lower node number also results in higher diameter value (Scardoni and Laudanna, 2012), and the high network diameter presents lower efficiency for the transmission information and resources across entire microbial community in F. microcarpa. It is consistent with the smaller fig size in F. microcarpa than in F. altissima (observation results), implying fig wasps (such as cheaters and pollinators) have less coexistence chance in former than in latter.
Microorganisms associated with insects can have major roles in insect nutrition and metabolism (Dillon and Dillon, 2004; Douglas, 2018; Jang and Kikuchi, 2020). The main functions of bacteria predicted in fig wasps include metabolism, fermentation and chemoheterotrophy, while the fungal guilds comprised of both pathogen and endosymbiont. Since the fig wasps lay eggs in the figs and incubate until they emerge from the gall flowers to mate, they can only obtain limited food from the figs, especially after mating (Janzen, 1979). Some groups of Proteobacteria, Firmicutes, Acidobacteria, and Bacteroidetes might contribute to nutrient provisioning to fig wasps, and may be involved in metabolic processes including nitrogen recycling and fixation (Hongoh, 2010; Desai and Brune, 2012; Köhler et al., 2012). The genus Desulfovibrio detected in our study could reduce sulfate (Kuhnigk et al., 1996), (Arias-Cordero et al., 2012) and participates in metabolic processes involving sulfate and acetate (Kuhnigk et al., 1996). Notably, some bacteria from genus Enterobacter are capable of degrade cell walls in the host plant (Xia et al., 2017), which might enhance utilization efficiency of fig resources for the fig wasps. The Lachnospiraceae that were identified at low abundance in our samples have high proteolytic and cellulolytic activities. They are considered an ecologically important constituent of the bacterial population under certain dietary conditions (Cotta and Forster, 2006). Another study in broad-headed bug demonstrated that infection of genus Burkholderia, common symbiotic bacteria, enhances insecticide resistance and fitness of host bug (Kikuchi et al., 2007, 2012).
Moreover, Klebsiella, Enterocuccus, and Serratia are capable of producing aggregation pheromone, a type of chemical compound that has been shown to cause locust swarming (Dillon et al., 2002). Fungi associated with the bark beetles can also synthesize specific aggregation pheromone 2-Methyl-3-buten-2-ol (Zhao et al., 2015). Other genera, including Pseudomonas, Staphylococcus, Acinetobacter, and Pantoea, contain numerous pathogenic species and can attract predators via chemical signaling (Chansang et al., 2010; Engel and Moran, 2013; Khan et al., 2014). This may account for the existence of diverse non-pollinator fig wasps, which some non-pollinator fig wasps tend to oviposit in figs that have been visited by pollinator fig wasps (Peng et al., 2005). Given that the microbial community composition and functions in the fig wasp system is severely underexplored, our study provides preliminary evidence on microbial community functions and their potential contributions to fig wasp physiology and health for further functional validation.
Wolbachia are the most common intracellular bacteria in arthropods and nematodes, and can affect host speciation through cytoplasmic incompatibility (Walker et al., 2021), sex pheromones, and mating behavior (Sharon et al., 2010). The incidence of Wolbachia infection is found to be pervasive in fig wasp (Dewayne Shoemaker et al., 2002; Haine and Cook, 2005; Ahmed et al., 2013). Earlier studies on a large-scale survey of the Wolbachia and their host fig wasps have pointed out that convergent incidence of Wolbachia is found in different fig wasp species, and suggested neither phylogeny nor ecological association among host species is consistent to the phylogenetic affinities of the Wolbachia infection (Dewayne Shoemaker et al., 2002; Haine and Cook, 2005). The Wolbachia cluster dendrogram in our study did not support absolute isolation between the cheater and pollinator wasps. However, a strong phylogenetic correlation between the pollinators and Wolbachia strains have been reported on the F. microcapa of Hainan islands (Sun et al., 2011). Their study showed that three clades (cryptic species, but the authors did not consider the existence of cheaters in F. microcarpa) of the pollinator wasps corresponded to three Wobachia strains. We further extracted the COI gene in our fig wasps and constructed the phylogenetic relationship with the three clades samples from Sun et al., 2011. The results showed that clade 1 was clustered with the pollinator wasps, and clade 2 corresponded to the cheater wasps (Supplementary Figure S1), indicating that the clade2 are very likely the cheaters of F. microcarpa.. Therefore, the effect of Wolbachia on speciation of fig wasps from F. microcarpa should be furtherly tested. Interestingly, the presence of Wolbachia infection was not observed in cheaters and pollinators from F. altissima. Although the reason of absence of Wolbachia infection is uncertain, the sampling individuals without Wolbachia infection, and lacking specific amplification primers of Wolbachia may account for failing to detect Wolbachia sequences from unmapped data.
In the obligate pollinating systems, the cheater visitors are rare. However, the role of microorganism in this system still remains unknown. We analyzed the phylogenetic relationship between cheaters and pollinators, then explored diversity and contribution of microorganisms associated with cheater and pollinator wasps. Our study showed that the cheater and pollinator wasps are highly divergent genetic lineages. And their association microbial groups are extremely diverse, which may play essential roles in maintaining nutrient, health, and speciation of fig wasps. Besides, fig species dominate over the fig wasp genotype in shaping the microbial communities, which may help cheater to coexist with pollinators in figs.
The data presented in the study are deposited in the NCBI repository, accession number GenBank OP715733-OP715755. And the WGS data associated Bioproject number is PRJNA893401.
JG, YD, and Y-QP conceived the study. JG, YD, and J-FH collected the samples. Z-RZ analyzed the genetic part data. YD analyzed the microbiome data. YD and JG wrote the manuscript. J-FH, Y-QP, SM, AW, and BW gave suggestions during the manuscript writing. All authors contributed to the article and approved the submitted version.
This study was supported by grants from West Light Foundation of the Chinese Academy of Sciences and the National Natural Science Foundation of China (NSFC 32271591 and 32070487).
We appreciate the linguistic assistance provided by Sawyer Adams during the preparation of this manuscript. We would also like to thank Huayan Chen for providing the photos of fig wasps. Genomic data processing and analyses were conducted at the High Performance Computing Cluster from the Institutional Center for Shared Technologies and Facilities of Xishuangbanna Tropical Botanical Garden, CAS.
The authors declare that the research was conducted in the absence of any commercial or financial relationships that could be construed as a potential conflict of interest.
All claims expressed in this article are solely those of the authors and do not necessarily represent those of their affiliated organizations, or those of the publisher, the editors and the reviewers. Any product that may be evaluated in this article, or claim that may be made by its manufacturer, is not guaranteed or endorsed by the publisher.
The Supplementary material for this article can be found online at: https://www.frontiersin.org/articles/10.3389/fmicb.2022.1009919/full#supplementary-material
SUPPLEMENTARY FIGURE S1 | The ML tree of the pollinator and cheater wasps in this study (the Electric blue background) with the Eupristina species found in F. microcarpa in Hainan islands based on COI gene.
SUPPLEMENTARY FIGURE S2 | The features of microbial communities. (A) LEfSe plot of biomarkers in communities from F. altissima. (B) LEfSe plot of biomarkers in communities from F. microcarpa. The threshold of discriminant score was 2.0. Fa_C represents the cheater of F. altissima; Fa_P represents the pollinator of F. altissima; Fm_C represents the cheater of F. microcarpa; Fm_P represents the pollinator of F. microcarpa.
SUPPLEMENTARY FIGURE S3 | The cluster dendrogram produced by the ward.D2 method using Euclidean distances based on the compositions of Wolbachia bacteria in samples. The tip labels in the dendrogram are the sample codes; Fm_C represents the cheater of F. microcarpa; Fm_P represents the pollinator of F. microcarpa.
SUPPLEMENTARY FIGURE S4 | The diversity indices patterns of fig wasps-associated fungi and bacteria. (A,B) The Shannon and Chao 1 diversity index of fungal communities. (C,D) The Shannon and Chao 1 diversity index of bacterial communities. The letters at the top of each panel were calculated by the least significant difference (LSD) test, the same letter represents no significant difference. Fa_C represents the cheater of F. altissima; Fa_P represents the pollinator of F. altissima; Fm_C represents the cheater of F. microcarpa; Fm_P represents the pollinator of F. microcarpa.
SUPPLEMENTARY FIGURE S5 | The relative abundance of fungal and bacterial communities at phylum level in different individual samples. (A) Fungal compositions at phylum level. (B) Bacterial compositions at phylum level. Fa_C represents the cheater of F. altissima; Fa_P represents the pollinator of F. altissima; Fm_C represents the cheater of F. microcarpa; Fm_P represents the pollinator of F. microcarpa.
SUPPLEMENTARY FIGURE S6 | The rarefaction curves of fungal community (A) and bacterial (B) among four different samples. Fa_C represents the cheater of F. altissima; Fa_P represents the pollinator of F. altissima; Fm_C represents the cheater of F. microcarpa; Fm_P represents the pollinator of F. microcarpa.
SUPPLEMENTARY FIGURE S7 | The microbial co-occurrence networks based on filtered correlation coefficient (Spearman’s r > 0.8 and p value <0.05). (A) The co-occurrence network from the cheater of F. altissima. (B) The co-occurrence network from the pollinator of F. altissima. (C) The co-occurrence network from the cheater of F. microcarpa. (D) The co-occurrence network from the pollinator of F. microcarpa. The nodes in the co-occurrence network are the microbial genera, the color of nodes represents the phylum that the genus belongs to, the links in the network are the significant correlation in two nodes, the width of link line reflects the absolute value of correlation coefficient and the color of link line represents the positive (gray) and negative (red) correlation coefficient. Fa_C represents the cheater of F. altissima; Fa_P represents the pollinator of F. altissima; Fm_C represents the cheater of F. microcarpa; Fm_P represents the pollinator of F. microcarpa.
SUPPLEMENTARY FIGURE S8 | Heatmap of the functional groups obtained from FAPROTAX for each sample of bacterial community. The color bar reflects the value of functional abundance. Fa_C represents the cheater of F. altissima; Fa_P represents the pollinator of F. altissima; Fm_C represents the cheater of F. microcarpa; Fm_P represents the pollinator of F. microcarpa.
1. ^http://www.bioinformatics.babraham.ac.uk/projects/fastqc/
2. ^http://picard.sourceforge.net
3. ^https://github.com/sghignone/bwa
4. ^http://blast.ncbi.nlm.nih.gov/Blast.cgi?PAGE_TYPE=BlastDocs
Ahmed, M. Z., Greyvenstein, O. F., Erasmus, C., Welch, J. J., and Greeff, J. M. (2013). Consistently high incidence of Wolbachia in global fig wasp communities. Ecological Entomol. 38, 147–154. doi: 10.1111/een.12002
Arias-Cordero, E., Ping, L., Reichwald, K., Delb, H., Platzer, M., and Boland, W. (2012). Comparative evaluation of the gut microbiota associated with the below-and above-ground life stages (larvae and beetles) of the forest cockchafer, Melolontha hippocastani. PLoS One 7:e51557. doi: 10.1371/journal.pone.0051557
Bailón-Salas, A. M., Medrano-Roldán, H., Valle-Cervantes, S., Ordaz-Díaz, L. A., Urtiz-Estrada, N., and Rojas-Contreras, J. A. (2017). Review of molecular techniques for the identification of bacterial communities in biological effluent treatment facilities at pulp and paper mills. Bioresources 12, 4384–4409. doi: 10.15376/biores.12.2.Bailon_Salas
Balbuena, J. A., Míguez-Lozano, R., and Blasco-Costa, I. (2013). PACo: a novel procrustes application to cophylogenetic analysis. PLoS One 8:e61048. doi: 10.1371/journal.pone.0061048
Barberán, A., Bates, S. T., Casamayor, E. O., and Fierer, N. (2012). Using network analysis to explore co-occurrence patterns in soil microbial communities. ISME J. 6, 343–351. doi: 10.1038/ismej.2011.119
Bolger, A. M., Lohse, M., and Usadel, B. (2014). Trimmomatic: a flexible trimmer for Illumina sequence data. Bioinformatics 30, 2114–2120. doi: 10.1093/bioinformatics/btu170
Bordenstein, S. R., O'Hara, F. P., and Werren, J. H. (2001). Wolbachia-induced incompatibility precedes other hybrid incompatibilities in Nasonia. Nature 409, 707–710. doi: 10.1038/35055543
Brucker, R. M., and Bordenstein, S. R. (2012). The roles of host evolutionary relationships (genus: Nasonia) and development in structuring microbial communities. Evolution: international journal of organic. Evolution 66, 349–362. doi: 10.1111/j.1558-5646.2011.01454.x
Casteel, C. L., and Hansen, A. K. (2014). Evaluating insect-microbiomes at the plant-insect interface. J. Chem. Ecol. 40, 836–847. doi: 10.1007/s10886-014-0475-4
Chansang, U., Mulla, M. S., Chantaroj, S., and Sawanpanyalert, P. (2010). The eye fly Siphunculina funicola (Diptera: Chloropidae) as a carrier of pathogenic bacteria in Thailand. Southeast Asian J. Trop. Med. Public Health 41, 61–71.
Chow, C. E. T., Kim, D. Y., Sachdeva, R., Caron, D. A., and Fuhrman, J. A. (2014). Top-down controls on bacterial community structure: microbial network analysis of bacteria, T4-like viruses and protists. ISME J. 8, 816–829. doi: 10.1038/ismej.2013.199
Colman, D. R., Toolson, E. C., and Takacs-Vesbach, C. (2012). Do diet and taxonomy influence insect gut bacterial communities? Mol. Ecol. 21, 5124–5137. doi: 10.1111/j.1365-294X.2012.05752.x
Compton, S., Holton, K., Rashbrook, V., Van Noort, S., and Vincent, S. (1991). Studies of Ceratosolen galili, a non-pollinating agaonid fig wasp. Biotropica 23, 188–194. doi: 10.2307/2388305
Cook, J. M., and Segar, S. T. (2010). Speciation in fig wasps. Ecological Entomol. 35, 54–66. doi: 10.1111/j.1365-2311.2009.01148.x
Cotta, M., and Forster, R. (2006). The family Lachnospiraceae, including the genera Butyrivibrio, Lachnospira and Roseburia. PRO 4, 1002–1021. doi: 10.1007/0-387-30744-3_35
Cruaud, A., Rønsted, N., Chantarasuwan, B., Chou, L. S., Clement, W. L., Couloux, A., et al. (2022). An extreme case of plant-insect codiversification: figs and fig-pollinating wasps. Syst Biol. 61, 1029–1047. doi: 10.1093/sysbio/sys068
Csardi, G., and Nepusz, T. (2006). The igraph software package for complex network research. InterJournal, Complex Systems 1695, 1–9.
Danecek, P., Auton, A., Abecasis, G., Albers, C. A., Banks, E., DePristo, M. A., et al. (2011). The variant call format and VCFtools. Bioinformatics 27, 2156–2158. doi: 10.1093/bioinformatics/btr330
De Mandal, S., Panda, A., Bisht, S., and Kumar, N. (2015). Microbial ecology in the era of next generation sequencing. Next Generat Sequenc & Applic S 1, 1–6. doi: 10.4172/2469-9853.S1-001
DePristo, M. A., Banks, E., Poplin, R., Garimella, K. V., Maguire, J. R., Hartl, C., et al. (2011). A framework for variation discovery and genotyping using next-generation DNA sequencing data. Nat. Genet. 43, 491–498. doi: 10.1038/ng.806
Desai, M. S., and Brune, A. (2012). Bacteroidales ectosymbionts of gut flagellates shape the nitrogen-fixing community in dry-wood termites. ISME J. 6, 1302–1313. doi: 10.1038/ismej.2011.194
Dewayne Shoemaker, D., Machado, C. A., Molbo, D., Werren, J. H., Windsor, D. M., and Herre, E. A. (2002). The distribution of Wolbachia in fig wasps: correlations with host phylogeny, ecology and population structure. Proceedings of the Royal Society of London. Series B: Biological Sciences, 269, 2257–2267.
Dillon, R. J., and Dillon, V. (2004). The gut bacteria of insects: nonpathogenic interactions. Annu. Rev. Entomol. 49, 71–92. doi: 10.1146/annurev.ento.49.061802.123416
Dillon, R., Vennard, C., and Charnley, A. (2002). A note: gut bacteria produce components of a locust cohesion pheromone. J. Appl. Microbiol. 92, 759–763. doi: 10.1046/j.1365-2672.2002.01581.x
Dormann, C. F., Fründ, J., Blüthgen, N., and Gruber, B. (2009). Indices, graphs and null models: analyzing bipartite ecological networks. Open Ecology J. 2, 7–24. doi: 10.2174/1874213000902010007
Douglas, A. E. (2015). Multiorganismal insects: diversity and function of resident microorganisms. Annu. Rev. Entomol. 60, 17–34. doi: 10.1146/annurev-ento-010814-020822
Douglas, A. E. (2018). Omics and the metabolic function of insect-microbial symbioses. Current Opinion Insect Sci. 29, 1–6. doi: 10.1016/j.cois.2018.05.012
Dunne, J. A., Williams, R. J., and Martinez, N. D. (2002). Network structure and biodiversity loss in food webs: robustness increases with connectance. Ecol. Lett. 5, 558–567. doi: 10.1046/j.1461-0248.2002.00354.x
Engel, P., and Moran, N. A. (2013). The gut microbiota of insects-diversity in structure and function. FEMS Microbiol. Rev. 37, 699–735. doi: 10.1111/1574-6976.12025
Escalas, A., Hale, L., Voordeckers, J. W., Yang, Y., Firestone, M. K., Alvarez-Cohen, L., et al. (2019). Microbial functional diversity: from concepts to applications. Ecol. Evol. 9, 12000–12016. doi: 10.1002/ece3.5670
Fornito, A., Zalesky, A., and Bullmore, E. (2016). Fundamentals of brain network analysis. United States: Academic Press.
Gibbons, S. M., and Gilbert, J. A. (2015). Microbial diversity-exploration of natural ecosystems and microbiomes. Curr. Opin. Genet. Dev. 35, 66–72. doi: 10.1016/j.gde.2015.10.003
Gould, A. L., Zhang, V., Lamberti, L., Jones, E. W., Obadia, B., Korasidis, N., et al. (2018). Microbiome interactions shape host fitness. Proc. Natl. Acad. Sci. 115, E11951–E11960. doi: 10.1073/pnas.1809349115
Groussin, M., Mazel, F., and Alm, E. J. (2020). Co-evolution and co-speciation of host-gut bacteria systems. Cell Host Microbe 28, 12–22. doi: 10.1016/j.chom.2020.06.013
Haine, E. R., and Cook, J. M. (2005). Convergent incidences of Wolbachia infection in fig wasp communities from two continents. Proc. R. Soc. B Biol. Sci. 272, 421–429. doi: 10.1098/rspb.2004.2956
Herre, E. A. (1989). Coevolution of reproductive characteristics in 12 species of New World figs and their pollinator wasps. Experientia 45, 637–647. doi: 10.1007/BF01975680
Hongoh, Y. (2010). Diversity and genomes of uncultured microbial symbionts in the termite gut. Biosci. Biotechnol. Biochem. 74, 1145–1151. doi: 10.1271/bbb.100094
Hsieh, T. C., Ma, K. H., and Chao, A. (2016). iNEXT: an R package for rarefaction and extrapolation of species diversity (hill numbers). Methods Ecol. Evol. 7, 1451–1456. doi: 10.1111/2041-210X.12613
Hu, T., Chitnis, N., Monos, D., and Dinh, A. (2021). Next-generation sequencing technologies: an overview. Hum. Immunol. 82, 801–811. doi: 10.1016/j.humimm.2021.02.012
Jandér, K. C., and Herre, E. A. (2010). Host sanctions and pollinator cheating in the fig tree–fig wasp mutualism. Proc. R. Soc. B Biol. Sci. 277, 1481–1488. doi: 10.1098/rspb.2009.2157
Jandér, K. C., and Herre, E. A. (2016). Host sanctions in Panamanian Ficus are likely based on selective resource allocation. Am. J. Bot. 103, 1753–1762. doi: 10.3732/ajb.1600082
Jang, S., and Kikuchi, Y. (2020). Impact of the insect gut microbiota on ecology, evolution, and industry. Current Opinion Insect Sci. 41, 33–39. doi: 10.1016/j.cois.2020.06.004
Janzen, D. H. (1979). How to be a fig. Annu. Rev. Ecol. Syst. 10, 13–51. doi: 10.1146/annurev.es.10.110179.000305
Jin, J. J., Yu, W. B., Yang, J. B., Song, Y., DePamphilis, C. W., Yi, T. S., et al. (2020). GetOrganelle: a fast and versatile toolkit for accurate de novo assembly of organelle genomes. Genome Biol. 21, 1–31. doi: 10.1186/s13059-020-02154-5
Jones, R. T., Sanchez, L. G., and Fierer, N. (2013). A cross–taxon analysis of insect-associated bacterial diversity. PLoS One 8:e61218. doi: 10.1371/journal.pone.0061218
Kalyaanamoorthy, S., Minh, B. Q., Wong, T. K., Von Haeseler, A., and Jermiin, L. S. (2017). ModelFinder: fast model selection for accurate phylogenetic estimates. Nat. Methods 14, 587–589. doi: 10.1038/nmeth.4285
Katoh, K., and Standley, D. M. (2013). MAFFT multiple sequence alignment software version 7: improvements in performance and usability. Mol. Biol. Evol. 30, 772–780. doi: 10.1093/molbev/mst010
Kearse, M., Moir, R., Wilson, A., Stones-Havas, S., Cheung, M., Sturrock, S., et al. (2012). Geneious basic: an integrated and extendable desktop software platform for the organization and analysis of sequence data. Bioinformatics 28, 1647–1649. doi: 10.1093/bioinformatics/bts199
Khan, I. A., Khan, A., Asif, H., Jiskani, M. M., Mühlbach, H. P., and Azim, M. K. (2014). Isolation and 16S rDNA sequence analysis of bacteria from dieback affected mango orchards in southern Pakistan. Pak. J. Bot. 46, 1431–1435.
Kikuchi, Y., Hayatsu, M., Hosokawa, T., Nagayama, A., Tago, K., and Fukatsu, T. (2012). Symbiont-mediated insecticide resistance. Proc. Natl. Acad. Sci. 109, 8618–8622. doi: 10.1073/pnas.1200231109
Kikuchi, Y., Hosokawa, T., and Fukatsu, T. (2007). Insect-microbe mutualism without vertical transmission: a stinkbug acquires a beneficial gut symbiont from the environment every generation. Appl. Environ. Microbiol. 73, 4308–4316. doi: 10.1128/AEM.00067-07
Koduru, S. K. (2021). Herbivore-associated microorganisms in the modulation of plant responses: an overview. Recent Develop. Applied Microbiol. Biochemistry 2, 221–228. doi: 10.1016/B978-0-12-821406-0.00020-5
Köhler, T., Dietrich, C., Scheffrahn, R. H., and Brune, A. (2012). High-resolution analysis of gut environment and bacterial microbiota reveals functional compartmentation of the gut in wood-feeding higher termites (Nasutitermes spp.). Appl. Environ. Microbiol. 78, 4691–4701. doi: 10.1128/AEM.00683-12
Kong, Y. (2014). The structure and function of fig wasp community on Ficus microcarpa in different locations in Yunnan. [Master’s thesis]. [Yunnan]: Xishuangbanna Tropical Botanical Garden, Chinese Academy of Sciences.
Kuhnigk, T., Branke, J., Krekeler, D., Cypionka, H., and König, H. (1996). A feasible role of sulfate-reducing bacteria in the termite gut. Syst. Appl. Microbiol. 19, 139–149. doi: 10.1016/S0723-2020(96)80039-7
Landi, P., Minoarivelo, H. O., Brännström, Å., Hui, C., and Dieckmann, U. (2018). Complexity and stability of ecological networks: a review of the theory. Popul. Ecol. 60, 319–345. doi: 10.1007/s10144-018-0628-3
Lazebnik, J., Frago, E., Dicke, M., and van Loon, J. J. A. (2014). Phytohormone mediation of interactions between herbivores and plant pathogens. J. Chem. Ecol. 40, 730–741. doi: 10.1007/s10886-014-0480-7
Lee, T. H., Guo, H., Wang, X., Kim, C., and Paterson, A. H. (2014). SNPhylo: a pipeline to construct a phylogenetic tree from huge SNP data. BMC Genomics 15:162. doi: 10.1186/1471-2164-15-162
Li, H. (2013). Aligning sequence reads, clone sequences and assembly contigs with BWA-MEM. arXiv preprint arXiv:1303.3997.
Lizé, A., McKay, R., and Lewis, Z. (2014). Kin recognition in Drosophila: the importance of ecology and gut microbiota. The ISME journal 8, 469–477.
Louca, S., Parfrey, L. W., and Doebeli, M. (2016). Decoupling function and taxonomy in the global ocean microbiome. Science 353, 1272–1277. doi: 10.1126/science.aaf4507
Maron, P. A., Sarr, A., Kaisermann, A., Lévêque, J., Mathieu, O., Guigue, J., et al. (2018). High microbial diversity promotes soil ecosystem functioning. Appl. Environ. Microbiol. 84, e02738–e02717. doi: 10.1128/AEM.02738-17
Martinson, E. O., Herre, E. A., Machado, C. A., and Arnold, A. E. (2012). Culture-free survey reveals diverse and distinctive fungal communities associated with developing figs (Ficus spp.) in Panama. Microb. Ecol. 64, 1073–1084. doi: 10.1007/s00248-012-0079-x
Martinson, E. O., Jandér, K. C., Peng, Y. Q., Chen, H. H., Machado, C. A., Arnold, A. E., et al. (2014). Relative investment in egg load and poison sac in fig wasps: implications for physiological mechanisms underlying seed and wasp production in figs. Acta Oecol. 57, 58–66. doi: 10.1016/j.actao.2013.07.009
Masella, A. P., Bartram, A. K., Truszkowski, J. M., Brown, D. G., and Neufeld, J. D. (2012). PANDAseq: paired-end assembler for illumina sequences. BMC Bioinformatics 13, 1–7. doi: 10.1186/1471-2105-13-31
Minh, B. Q., Nguyen, M. A. T., and von Haeseler, A. (2013). Ultrafast approximation for phylogenetic bootstrap. Mol. Biol. Evol. 30, 1188–1195. doi: 10.1093/molbev/mst024
Mithöfer, A., and Boland, W. (2012). Plant defense against herbivores: chemical aspects. Annu. Rev. Plant Biol. 63, 431–450. doi: 10.1146/annurev-arplant-042110-103854
Morales-Rodriguez, C., Sferrazza, I., Aleandri, M., Dalla Valle, M., Mazzetto, T., Speranza, S., et al. (2019). Fungal community associated with adults of the chestnut gall wasp Dryocosmus kuriphilus after emergence from galls: taxonomy and functional ecology. Fungal Biol. 123, 905–912. doi: 10.1016/j.funbio.2019.09.009
Murtagh, F., and Legendre, P. (2014). Ward’s hierarchical agglomerative clustering method: which algorithms implement Ward’s criterion? J. Classif. 31, 274–295. doi: 10.1007/s00357-014-9161-z
Nazir, R., Rehman, S., Nisa, M., and Ali Baba, U. (2019). “Exploring bacterial diversity: from cell to sequence” in Freshwater Microbiology. eds. S. A. Bandh, S. Shafi, and N. Shameem (Cambridge, MA: Academic Press), 263–306.
Nefdt, R. J., and Compton, S. G. (1996). Regulation of seed and pollinator production in the fig–fig wasp mutualism. J. Anim. Ecol. 65, 170–182. doi: 10.2307/5720
Newman, M. E., Barabási, A. L. E., and Watts, D. J. (2006). The structure and dynamics of networks. United States: Princeton University Press.
Newman, M. E., and Girvan, M. (2004). Finding and evaluating community structure in networks. Phys. Rev. E 69:026113. doi: 10.1103/PhysRevE.69.026113
Nguyen, L. T., Schmidt, H. A., Von Haeseler, A., and Minh, B. Q. (2015). IQ-TREE: a fast and effective stochastic algorithm for estimating maximum-likelihood phylogenies. Mol. Biol. Evol. 32, 268–274. doi: 10.1093/molbev/msu300
Nguyen, N. H., Song, Z., Bates, S. T., Branco, S., Tedersoo, L., Menke, J., et al. (2016). FUNGuild: an open annotation tool for parsing fungal community datasets by ecological guild. Fungal Ecol. 20, 241–248. doi: 10.1016/j.funeco.2015.06.006
Niu, L. H., Song, X. F., He, S. M., Zhang, P., Wang, N. X., Li, Y., et al. (2015a). New insights into the fungal community from the raw genomic sequence data of fig wasp Ceratosolen solmsi. BMC Microbiol. 15, 1–11. doi: 10.1186/s12866-015-0370-3
Niu, L. H., Xiao, J., Murphy, R., Li, Y., Huang, D. W., Niu, L., et al. (2015b). Host phylogeny and diet structure its bacterial community: a case study of various fig wasps coexisting in Ficus hispida. Res. Rev.: J. Microbiol. Biotechn. 4, 44–51. Available at: https://www.rroij.com/open-access/host-phylogeny-and-diet-structure-its-bacterial-community-a-case-study-of-various-fig-wasps-coexisting-in-ficus-hispida.php?aid=61416
Okonechnikov, K., Conesa, A., and García-Alcalde, F. (2016). Qualimap 2: advanced multi–sample quality control for high–throughput sequencing data. Bioinformatics 32, 292–294. doi: 10.1093/bioinformatics/btv566
Oksanen, J., Blanchet, F. G., Friendly, M., Kindt, R., Legendre, P., McGlinn, D., et al. (2018). Vegan: community ecology package. R package version 2.5-1. Available online at: https://CRAN.R–project.org/package=vegan
Palmer, J. D., and Foster, K. R. (2022). Bacterial species rarely work together. Science 376, 581–582. doi: 10.1126/science.abn5093
Patterson, N., Price, A. L., and Reich, D. (2006). Population structure and eigenanalysis. PLoS Genet. 2:e190. doi: 10.1371/journal.pgen.0020190
Pellmyr, O., and Leebens-Mack, J. (1999). Forty million years of mutualism: evidence for Eocene origin of the yucca-yucca moth association. Proc. Natl. Acad. Sci. 96, 9178–9183. doi: 10.1073/pnas.96.16.9178
Pellmyr, O., Leebens-Mack, J., and Huth, C. J. (1996). Non-mutualistic yucca moths and their evolutionary consequences. Nature 380, 155–156. doi: 10.1038/380155a0
Peng, Y. Q., Duan, Z. B., Yang, D. R., and Rasplus, J. Y. (2008). Co-occurrence of two Eupristina species on Ficus altissima in Xishuangbanna, SW China. Symbiosis 45, 9–14. Available at: https://dalspace.library.dal.ca/bitstream/handle/10222/78312/VOLUME%2045-NUMBERS%201-3-2008-PAGE%209.pdf?sequence=1
Peng, Y. Q., Yang, D. R., and Wang, Q. Y. (2005). Quantitative tests of interaction between pollinating and non-pollinating fig wasps on dioecious Ficus hispida. Ecological Entomol. 30, 70–77. doi: 10.1111/j.0307-6946.2005.00650.x
R Development Core Team (2018). R: A language and environment for statistical computing. R Foundation for Statistical Computing. Vienna, Austria.
Raj, A., Stephens, M., and Pritchard, J. K. (2014). fastSTRUCTURE: variational inference of population structure in large SNP data sets. Genetics 197, 573–589. doi: 10.1534/genetics.114.164350
Rasplus, J. Y. (1996). The one-to-one species specificity of the Ficus-Agaoninae mutualism: How casual? The biodiversity of African plants, pp. 639–649. Springer, Dordrecht.
Ratzke, C., Barrere, J., and Gore, J. (2020). Strength of species interactions determines biodiversity and stability in microbial communities. Nature Ecology & Evolution 4, 376–383. doi: 10.1038/s41559-020-1099-4
Receveur, J. P., Fenoglio, S., and Benbow, M. E. (2020). Insect-associated bacterial communities in an alpine stream. Hydrobiologia 847, 331–344. doi: 10.1007/s10750-019-04097-w
Sanders, J. G., Powell, S., Kronauer, D. J., Vasconcelos, H. L., Frederickson, M. E., and Pierce, N. E. (2014). Stability and phylogenetic correlation in gut microbiota: lessons from ants and apes. Mol. Ecol. 23, 1268–1283. doi: 10.1111/mec.12611
Scardoni, G., and Laudanna, C. (2012). “Centralities based analysis of complex networks. New Frontiers in graph theory” in InTech. ed. Y. Zhang (London: INTECH Open Access Publisher), 323–348.
Segraves, K. A., Althoff, D. M., and Pellmyr, O. (2005). Limiting cheaters in mutualism: evidence from hybridization between mutualist and cheater yucca moths. Proc. R. Soc. B Biol. Sci. 272, 2195–2201. doi: 10.1098/rspb.2005.3201
Serbus, L. R., Casper-Lindley, C., Landmann, F., and Sullivan, W. (2008). The genetics and cell biology of Wolbachia–host interactions. Annu. Rev. Genet. 42, 683–707. doi: 10.1146/annurev.genet.41.110306.130354
Sharma, L., Gonçalves, F., Oliveira, I., Torres, L., and Marques, G. (2018). Insect-associated fungi from naturally mycosed vine mealybug Planococcus ficus (Signoret)(Hemiptera: Pseudococcidae). Biocontrol Sci. Tech. 28, 122–141. doi: 10.1080/09583157.2018.1428733
Sharon, G., Segal, D., Ringo, J. M., Hefetz, A., Zilber-Rosenberg, I., and Rosenberg, E. (2010). Commensal bacteria play a role in mating preference of Drosophila melanogaster. Proc. Natl. Acad. Sci. 107, 20051–20056. doi: 10.1073/pnas.1009906107
Smit, E., Leeflang, P., and Wernars, K. (1997). Detection of shifts in microbial community structure and diversity in soil caused by copper contamination using amplified ribosomal DNA restriction analysis. FEMS Microbiol. Ecol. 23, 249–261. doi: 10.1111/j.1574-6941.1997.tb00407.x
Souza, C. D., Pereira, R. A., Marinho, C. R., Kjellberg, F., and Teixeira, S. P. (2015). Diversity of fig glands is associated with nursery mutualism in fig trees. Am. J. Bot. 102, 1564–1577. doi: 10.3732/ajb.1500279
Sun, X. J., Xiao, J. H., Cook, J. M., Feng, G., and Huang, D. W. (2011). Comparisons of host mitochondrial, nuclear and endosymbiont bacterial genes reveal cryptic fig wasp species and the effects of Wolbachia on host mtDNA evolution and diversity. BMC Evol. Biol. 11, 1–8. doi: 10.1186/1471-2148-11-86
Talavera, G., and Castresana, J. (2007). Improvement of phylogenies after removing divergent and ambiguously aligned blocks from protein sequence alignments. Syst. Biol. 56, 564–577. doi: 10.1080/10635150701472164
Vavre, F., and Kremer, N. (2014). Microbial impacts on insect evolutionary diversification: from patterns to mechanisms. Current Opinion Insect Sci. 4, 29–34. doi: 10.1016/j.cois.2014.08.003
Walker, T., Quek, S., Jeffries, C. L., Bandibabone, J., Dhokiya, V., Bamou, R., et al. (2021). Stable high-density and maternally inherited Wolbachia infections in anopheles moucheti and anopheles demeilloni mosquitoes. Curr. Biol. 31:e2315, 2310–2320. doi: 10.1016/j.cub.2021.03.056
Ware, A. B., and Compton, S. G. (1994). Dispersal of adult female fig wasps: 1. Arrivals and departures. Entomol. Exp. Appl. 73, 221–229. doi: 10.1111/j.1570-7458.1994.tb01859.x
Ware, A. B., Kaye, P. T., Compton, S. G., and Van Noort, S. (1993). Fig volatiles: their role in attracting pollinators and maintaining pollinator specificity. Plant Syst. Evol. 186, 147–156. doi: 10.1007/BF00940794
Warnecke, F., Luginbühl, P., Ivanova, N., Ghassemian, M., Richardson, T. H., Stege, J. T., et al. (2007). Metagenomic and functional analysis of hindgut microbiota of a wood–feeding higher termite. Nature 450, 560–565. doi: 10.1038/nature06269
Weiblen, G. D. (2002). How to be Afigwasp. Annu. Rev. Entomol. 47, 299–330. doi: 10.1146/annurev.ento.47.091201.145213
Weinbauer, M. G., and Wenderoth, D. F. (2002). Microbial diversity and ecosystem functions–the unmined riches. Electron. J. Biotechnol. 5, 19–20. doi: 10.2225/vol5-issue1-fulltext-11
Wemheuer, F., Taylor, J. A., Daniel, R., Johnston, E., Meinicke, P., Thomas, T., et al. (2020). Tax4Fun2: prediction of habitat-specific functional profiles and functional redundancy based on 16S rRNA gene sequences. Environmental Microbiome 15, 1–12. doi: 10.1186/s40793-020-00358-7
West, S. A., Herre, E. A., Windsor, D. M., and Green, P. R. (1996). The ecology and evolution of the New World non-pollinating fig wasp communities. J. Biogeogr. 23, 447–458. doi: 10.1111/j.1365-2699.1996.tb00006.x
Wiebes, J. (1979). Co-evolution of figs and their insect pollinators. Annu. Rev. Ecol. Syst. 10, 1–12. doi: 10.1146/annurev.es.10.110179.000245
Wielkopolan, B., and Obrępalska-Stęplowska, A. (2016). Three-way interaction among plants, bacteria, and coleopteran insects. Planta 244, 313–332. doi: 10.1007/s00425-016-2543-1
Xia, X., Gurr, G. M., Vasseur, L., Zheng, D., Zhong, H., Qin, B., et al. (2017). Metagenomic sequencing of diamondback moth gut microbiome unveils key holobiont adaptations for herbivory. Front. Microbiol. 8:663. doi: 10.3389/fmicb.2017.00663
Zhang, T., Jandér, K. C., Huang, J. F., Wang, B., Zhao, J. B., Miao, B. G., et al. (2021). The evolution of parasitism from mutualism in wasps pollinating the fig, Ficus microcarpa, in Yunnan Province, China. Proc. Natl. Acad. Sci. 118:e2021148118. doi: 10.1073/pnas.2021148118
Zhang, T., Miao, B. G., Wang, B., Peng, Y. Q., and Darwell, C. T. (2019). Non-pollinating cheater wasps benefit from seasonally poor performance of the mutualistic pollinating wasps at the northern limit of the range of Ficus microcarpa. Ecological Entomol. 44, 844–848. doi: 10.1111/een.12749
Zhang, X., Wang, G., Zhang, S., Chen, S., Wang, Y., Wen, P., et al. (2020). Genomes of the banyan tree and pollinator wasp provide insights into fig-wasp coevolution. Cells 183:e817, 875–889.e17. doi: 10.1016/j.cell.2020.09.043
Zhao, T., Axelsson, K., Krokene, P., and Borg Karlson, A. K. (2015). Fungal symbionts of the spruce bark beetle synthesize the beetle aggregation pheromone 2-methyl-3-buten-2-ol. J. Chem. Ecol. 41, 848–852. doi: 10.1007/s10886-015-0617-3
Zheng, L., Crippen, T. L., Holmes, L., Singh, B., Pimsler, M. L., Benbow, M. E., et al. (2013). Bacteria mediate oviposition by the black soldier fly, Hermetia illucens (L.), (Diptera: Stratiomyidae). Sci. Rep. 3, 1–8. doi: 10.1038/srep02563
Zhu, F., Poelman, E. H., and Dicke, M. (2014). Insect herbivore-associated organisms affect plant responses to herbivory. New Phytol. 204, 315–321. doi: 10.1111/nph.12886
Keywords: co-occurrence network, fig wasp, microbial community, whole-genome resequencing, interactions
Citation: Dong Y, Zhang Z-R, Mishra S, Wong AC-N, Huang J-F, Wang B, Peng Y-Q and Gao J (2022) Diversity and metabolic potentials of microbial communities associated with pollinator and cheater fig wasps in fig-fig wasp mutualism system. Front. Microbiol. 13:1009919. doi: 10.3389/fmicb.2022.1009919
Received: 02 August 2022; Accepted: 14 October 2022;
Published: 18 November 2022.
Edited by:
Ludmila Chistoserdova, University of Washington, United StatesReviewed by:
Ellen Martinson, University of New Mexico, United StatesCopyright © 2022 Dong, Zhang, Mishra, Wong, Huang, Wang, Peng and Gao. This is an open-access article distributed under the terms of the Creative Commons Attribution License (CC BY). The use, distribution or reproduction in other forums is permitted, provided the original author(s) and the copyright owner(s) are credited and that the original publication in this journal is cited, in accordance with accepted academic practice. No use, distribution or reproduction is permitted which does not comply with these terms.
*Correspondence: Yan-Qiong Peng, cGVuZ3lxQHh0YmcuYWMuY24=; Jie Gao, Z2FvamllQHh0Ymcub3JnLmNu
Disclaimer: All claims expressed in this article are solely those of the authors and do not necessarily represent those of their affiliated organizations, or those of the publisher, the editors and the reviewers. Any product that may be evaluated in this article or claim that may be made by its manufacturer is not guaranteed or endorsed by the publisher.
Research integrity at Frontiers
Learn more about the work of our research integrity team to safeguard the quality of each article we publish.