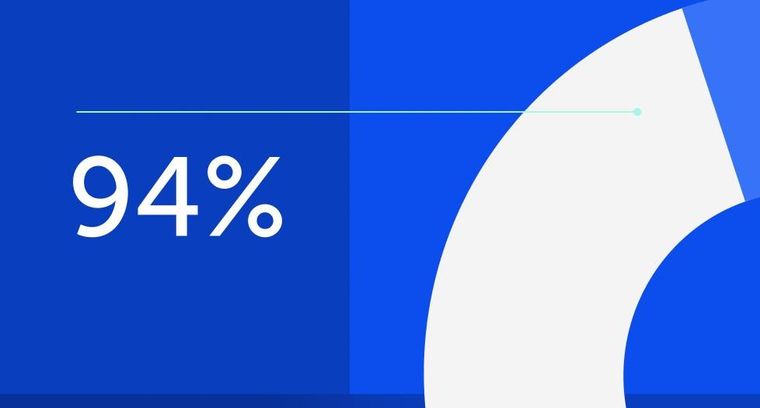
94% of researchers rate our articles as excellent or good
Learn more about the work of our research integrity team to safeguard the quality of each article we publish.
Find out more
ORIGINAL RESEARCH article
Front. Microbiol., 20 September 2022
Sec. Evolutionary and Genomic Microbiology
Volume 13 - 2022 | https://doi.org/10.3389/fmicb.2022.1007056
Sequencing of most Treponema pallidum genomes excludes repeat regions in tp0470 and the tp0433 gene, encoding the acidic repeat protein (arp). As a first step to understanding the evolution and function of these genes and the proteins they encode, we developed a protocol to nanopore sequence tp0470 and arp genes from 212 clinical samples collected from ten countries on six continents. Both tp0470 and arp repeat structures recapitulate the whole genome phylogeny, with subclade-specific patterns emerging. The number of tp0470 repeats is on average appears to be higher in Nichols-like clade strains than in SS14-like clade strains. Consistent with previous studies, we found that 14-repeat arp sequences predominate across both major clades, but the combination and order of repeat type varies among subclades, with many arp sequence variants limited to a single subclade. Although strains that were closely related by whole genome sequencing frequently had the same arp repeat length, this was not always the case. Structural modeling of TP0470 suggested that the eight residue repeats form an extended α-helix, predicted to be periplasmic. Modeling of the ARP revealed a C-terminal sporulation-related repeat (SPOR) domain, predicted to bind denuded peptidoglycan, with repeat regions possibly incorporated into a highly charged β-sheet. Outside of the repeats, all TP0470 and ARP amino acid sequences were identical. Together, our data, along with functional considerations, suggests that both TP0470 and ARP proteins may be involved in T. pallidum cell envelope remodeling and homeostasis, with their highly plastic repeat regions playing as-yet-undetermined roles.
In recent years, efforts to catalog genomic diversity and phylodynamics of the syphilis spirochete, Treponema pallidum subsp. pallidum, have resulted in a rapid increase in the amount of sequencing data and number of near-complete genome assemblies available in public databases (Pinto et al., 2016; Arora et al., 2017; Beale et al., 2019, 2021; Grillová et al., 2019; Chen et al., 2021; Lieberman et al., 2021; Taouk et al., 2022; Thurlow et al., 2022). Insights gained from these efforts, including the spread of azithromycin resistance (Beale et al., 2019) and high-resolution information on antigenic diversity (Lieberman et al., 2021), have aided our understanding of T. pallidum evolution and are invaluable to vaccine design. Although whole genome sequencing of low abundance T. pallidum DNA directly from clinical specimens is technically challenging due to the necessity of enrichment protocols such as hybrid capture with RNA or DNA baits (Pinto et al., 2016; Arora et al., 2017; Beale et al., 2019, 2021; Lieberman et al., 2021; Taouk et al., 2022), Dpn1 enrichment (Grillová et al., 2019), whole genome amplification (Chen et al., 2021; Thurlow et al., 2022), and/or the traditional technique of passage of clinical strains through rabbits, sufficient progress has been made in development of these techniques that sequencing throughput of samples on a scale appropriate for monitoring of vaccine trials is feasible.
Most genomic analyses of T. pallidum have excluded portions of the genome difficult to resolve by short-read sequencing, including the T. pallidum repeat (tpr) family of paralogous genes, the number of 60 bp tandem near-perfect repeats in the gene encoding the acidic repeat protein (arp) (tp0433), and the number of 24 bp tandem repeats in the gene encoding the tetratricopeptide repeat protein TP0470 (tp0470). Arp repeat length has been interrogated extensively using the CDC (Pillay et al., 1998) and enhanced CDC (Marra et al., 2010) typing schemes. This has allowed monitoring of local strain composition over time (Marra et al., 2010; Flasarová et al., 2012; Grimes et al., 2012) and of strains circulating worldwide (Sutton et al., 2001; Pillay et al., 2002; Pope et al., 2005; Liu et al., 2020), and identification of subtypes enriched in neurosyphilis (Molepo et al., 2007; Marra et al., 2010). However, these approaches focus on obtaining the number of arp repeats, rather than providing sequence information on the repeats, which is necessary for more robust genotyping. Our sequencing-based approach starts to fill this important knowledge gap.
Little is known about the role of tp0470 in syphilis pathogenesis, though tetratricopeptide repeat proteins in other bacterial species act as scaffolds for protein-protein interactions and are often critical to functionality of virulence factors (Cerveny et al., 2013). The highly charged motif “EAEEARRK,” encoded by the 24 bp repeat in tp0470, occurs C-terminal to the predicted tetratricopeptide repeat domain. This motif is repeated between 4 and 29 times in publicly available T. pallidum subsp. pallidum complete genomes, and up to 37 times in the T. pallidum subsp. pertenue strain CDC-2. The role of the arp gene is similarly understudied. It encodes an antigenic (Liu et al., 2007) protein containing at least four types of the 60 bp repeat that have been previously identified in T. pallidum subsp. pallidum, with differences confined to six positions, all of which result in amino acid substitutions (Liu et al., 2007; Harper et al., 2008). Repeat lengths between 2 and 22 have been previously observed in T. pallidum subsp. pallidum clinical specimens (Harper et al., 2008). Although the role of the arp in pathogenesis and colonization of various anatomic sites is unknown, some samples collected from whole blood had a lower number of arp tandem repeats than in patient-matched lesion swabs (Mikalová et al., 2013), and late stage syphilis samples are reported to have fewer arp repeats on average than early stage (Harper et al., 2008). Importantly, arp and tp0470 are thought to evolve via intra-strain recombination (Grillová et al., 2019; Noda et al., 2022).
To date, most closed T. pallidum genomes have relied on Sanger sequencing of arp and tp0470 (Cejková et al., 2012; Pětrošová et al., 2012; Zobaníková et al., 2013; Grillová et al., 2019), requiring extensive manual curation of data as well as ample starting material. Herein, we describe new bench and bioinformatic protocols for highly multiplexed nanopore sequencing of arp and tp0470, reducing the quantity of sample needed, as well as per-sample cost and hands-on time. These methodological improvements allowed us to gain insight into the evolution of both tp0470 and arp and formulate hypotheses that pave the way for functional studies to understand the role of these putative virulence factors in syphilis pathogenesis.
All human samples were collected and deidentified following protocols established at each institution. All Institutional Review Board (IRB) information from samples collected in Japan, Italy, Ireland, Maryland, United States, Madagascar, Peru, Papua New Guinea, and Nanjing, China has been previously published (Hopkins et al., 2004; Lukehart et al., 2004; Van Damme et al., 2009; Hook et al., 2010; Marra et al., 2010; Lieberman et al., 2021). Collection of additional samples was covered by the following IRBs: Malawi: National Health Sciences Research Committee Ministry of Health and Population (IRB Approval Number 2252); Colombia: Centro Internacional de Entrenamiento e Investigaciones Medicas (CIDEIM) Institutional Human Research Ethics Committee (CIEIH) (IRB protocol number 1289); Guangzhou, China: Dermatology Hospital of Southern Medical University (SMU) Medical Ethic Committee [IRB protocol number GDDHLS-20181202(R3)]; Chapel Hill, United States: University of North Carolina IRB Protocol Number 19-0311. Sequencing of deidentified strains was covered by the University of Washington Institutional Review Board (IRB) protocol number STUDY00000885 and University of North Carolina IRB protocol number 19-0311.
To obtain T. pallidum genomic DNA, in general, lesions were gently squeezed to release an exudate, then swabbed and placed in a 1.5 mL tube. Swab shafts were subsequently cut, and lysis buffer AL added, following the QiaAMP protocol (Qiagen, Germantown, MD, United States). Samples were quality assessed by tp47 and β-globin qPCR, sequenced and genomes assembled as previously described (Lieberman et al., 2021) or, for samples from Colombia, Malawi, Guangzhou, China, and Chapel Hill, United States, consensus sequences were assembled as previously described with minor modifications (Chen et al., 2021; Parr, 2021). Alignment, recombination masking and generation of the maximum likelihood phylogeny were performed as previously described (Lieberman et al., 2021).
Repeat regions of arp and tp0470 were amplified using 96 combinations of forward and reverse barcoded primers containing a 24 bp index (Supporting Information). Input ranged between approximately 100–10,000 genomes based on tp47 copies (0.5–8 μL) depending on genome copy number and amount of sample available. Samples were amplified by one of two methods, both using the Takara PrimeSTAR GXL polymerase in a 25 μL reaction: Those with low volume were first amplified with non-barcoded primers (98°C for 2 min, 35 cycles of 98°C for 10 s, 62°C for 15 s, 68°C for 2 min, then held at 68°C for 10 min before storing at 4°C). Then, the amplicon cleaned with 0.8× AMPure XP beads, diluted 1:100, and then 1 μL template barcoded with 14 additional cycles of PCR using the indexed primers, using a 65°C annealing temperature. Alternatively, samples were amplified directly from the genomic DNA using barcoded primers. Both methods produced equivalent results and technical replicates for each method agreed with each other (Supporting Information “Methods Comparison”).
PCR products were electrophoresed using 1 or 2% TAE agarose gels and purified with 0.6× or 0.8× volumes of AMPure XP beads for arp and tp0470 amplicons, respectively. Clean PCR products were quantified using Qubit 1X dsDNA HS buffer (Invitrogen, Waltham, MA, United States).
Following barcoding PCR and purification/quantification, amplicons were pooled to meet recommended input amounts (<1 μg DNA in 47 μL) for Oxford Nanopore (ONT) Adapter ligation kit (SQK-LSK109). Because most amplicons fell in the range of 2–10 ng/uL, we chose to maximize efficiency by pooling an equivalent volume per amplicon per run: Pooling of 0.5 μL allows 94 amplicons to be sequenced per run.
DNA end repair of the pool was performed as outlined in the ONT protocol for SQK-LSK109 using both ONT and NEBNext reagents, then purified with AMPure XP beads at a 1:1 ratio. Adapter ligation proceeded according to the manufacturer’s protocol, with room temperature incubation for 10 min, followed by purification with a ratio of 0.8× AMPure XP beads, washing with kit short fragment buffer (SFB), and elution in 47 μL water. The resulting pool was then quantified on Qubit (1X high sensitivity dsDNA kit) to calculate the total molecular weight of DNA. Molarity was calculated assuming an average fragment size of 800 bp.
Amplicons were sequenced on Flongle flow cells via the MinION mk1B platform. ONT MinKNOW software interfaced with the MinION to perform pre-run flow cell checks and initiate/monitor the sequencing experiment. Each Flongle was primed and loaded with 20 fmol DNA per SQK-LSK109 and Flongle sequencing expansion (EXP-FSE001).
Sequencing was run for 24 h, selecting “SQK-LSK109” for the DNA amplicon kit with high accuracy basecalling and other default parameters. Basecalling was performed in MinKNOW (v21.11.7) running Guppy (v.5.1.12) (Wick et al., 2019). Reads with an average phred score greater than nine passed the quality filter.
Fastq files that passed the quality filter were processed through Porechop (Wick et al., 2017) twice: First, using a customized adapters.py script that contained forward barcodes, using the default stringency of up to five mismatches in the 24 bp barcodes. Barcodes were modeled after those used previously in a dual-indexing protocol (Currin et al., 2019). Second round demultiplexing proceeded using reverse primers with the same parameters in the adapters2.py script, but ensuring no additional bases were trimmed past the barcodes.
Demultiplexed reads were aligned with bwa mem 0.7.17 (Li and Durbin, 2009) using the nanopore preset to reference files containing various numbers of tandem arp or tp0470 repeats and flanking regions of several hundred bases. Per-reference statistics were extracted with the BBtools v38.18 (Bushnell, 2014) utility pileup.sh, and the reference assigned the most reads determined for each sample using a custom R script. Consensus sequences were extracted using the sam2consensus utility script.1 Predicted number of repeats and resultant band size were then cross referenced with the agarose gel images, and the automated call either confirmed or overridden.
Locations of predicted signal peptides and lipidation sites were determined in “slow” mode in SignalP 6.0 (Teufel et al., 2022). Default settings were used for PSORT analysis (Yu et al., 2010). Conserved domains were determined using the NCBI Conserved Domain Database (Lu et al., 2020). Following removal of predicted secretion sequences, full length protein sequences for TP0470 and ARP were modeled in trRosetta (Yang et al., 2020; Du et al., 2021) with default settings and AlphaFold (Jumper et al., 2021; Varadi et al., 2022) using the pdb70 database for homology modeling and otherwise default settings. Protein models were visualized in PyMOL, and electrostatic surface potential shown with the Adaptive Poisson-Boltzmann Solver (APBS) plugin.
All statistical analysis was performed in R v4.0.3. Phylogenetic trees and tp0470 and arp variants were visualized with the R packages ggtree (Yu et al., 2017), treeio (Wang et al., 2020), and ggplot (Wickham, 2016), and multiple sequence alignments by R package ggmsa (Zhou et al., 2022). Bash, R, and python scripts for all data processing are available at https://github.com/greninger-lab/TP_genome_finishing.
We have previously reported the near-complete genomes of 196 T. pallidum strains, of which 191 were T. pallidum subsp. pallidum (Lieberman et al., 2021). Herein, we have updated the T. pallidum subsp. pallidum whole genome phylogeny to include 16 additional strains collected in Colombia, China, Malawi, and United States. With the exception of one strain collected from Malawi (TPVMW082H), all newly added strains fit within the subclades defined previously (Lieberman et al., 2021) (Figure 1A; Supporting Information). Malawi strain TPVMW082H appears to have diverged from the lineage that gave rise to the Nichols B and Nichols C subclades, but the maximum likelihood support values were below 0.95; therefore the phylogenetic relationship between TPVMW082H and Nichols B and C could not be clearly delineated. To demonstrate the fact that strain TPVMW082H is only distantly related to other samples included in this phylogeny, we have assigned this strain its own subclade, Nichols X.
Figure 1. Variation in tp0470 repeat length. (A) Recombination masked whole genome phylogeny (left) with the number of tp0470 repeats for each strain (right). Sequence variant number is included as text to the right of each length bar. All data are also included in a tabular representation in Supporting Information. Number of tp0470 repeats by subclade (B) or country (C).
To date, no systematic analysis of full-length T. pallidum tandem repeat genes has been performed, in part due to the fact that the short-read Illumina data used to generate most whole genomes cannot resolve the many 24- and 60-bp repeats found in tp0470 and arp, respectively. Although Sanger sequencing can be employed to examine repeats from both genes, this approach is labor intensive and relies on the a priori assumption that the tandem repeats comprise fewer than ∼800 bp, the length limit for Sanger sequencing; lengths of up to 22 repeats (1,320 bases) have been reported for arp (Harper et al., 2008). For tp0470, the DAL1 strain has 29 repeats of 24 bases (696 bases total) (Cejková et al., 2012), and the T. pallidum subsp. pertenue strain CDC-2 has 37 24 base repeats (888 bases total) with no upper limit known. Therefore, we developed bench and bioinformatic protocols for highly multiplexed, long read sequencing of the arp and tp0470 loci. We amplified portions of the arp and tp0470 genes using dual-indexed primers, allowing up to 192 amplicons on a single nanopore Flongle, followed by demultiplexing, reference mapping, and consensus calling. Across six Flongle runs to generate arp and tp0470 data on 212 strains, an average of 408,286 reads passing filter were generated. Following demultiplexing of forward and reverse barcodes, an average of 3,327 reads were assigned to each sample, comprising both arp and tp0470 reads. Reads were then mapped to tp0470 and arp reference files containing between 1–60 and 1–24 tandem repeats, respectively, using bwa mem (Li and Durbin, 2009) with the Oxford Nanopore preset (-ont2d) to account for low fidelity reads.
To validate our method, we cross referenced the band size seen on gel electrophoresis with the automated call from our pipeline. Supplementary Figures 1, 2 show the gel electrophoresis bands from a subset of samples and histograms of the distribution of mapping to the number of repeats for tp0470 and arp, respectively. We found 89% (183/206) concordance between the tp0470 pipeline call and band size, with discordance likely due to considerable low molecular weight byproducts produced during amplification of tp0470 repeats, which are 75% GC-rich. However, as is clear for sample China11, the correct repeat number was readily apparent in these samples upon inspection of the mapping distribution (boxed region and arrows, Supplementary Figure 1B). Technical replicates of select samples agreed with each other in 100% of cases, however, amplicons produced using a two-stage amplification (see Section “Materials and methods”) required more manual comparison with band size to eliminate the low molecular weight byproducts, which were unsurprisingly more likely to appear during two stage barcoding. For each sample, the proportion of aligned reads aligning to the top hit are shown as a distribution in Supplementary Figure 1C, highlighting the wide variability of byproducts generated during tp0470 PCR. Replicates generated using both methods gave the same result (Supporting Information “Methods Comparison”).
At 98.5% (200/203), concordance between the number of arp repeats determined automatically by our pipeline and the electrophoresis band size was extremely high (Supplementary Figure 2). Sanger sequencing was used to validate tp0470 repeat lengths of five samples with concentration exceeding 5 ng/μL and band sizes less than 700 bp, with 100% concordance seen. Confirmation of novel arp repeat types, as well as linkage between adjacent 60 bp repeats, was performed by manual inspection of Illumina WGS reads for all unique arp variants. Technical replicates and replicates using each barcoding method gave the same result (Supporting Information).
We first examined the consensus sequence and number of tandem 24 bp repeats in the tp0470 gene in the context of the recombination-masked whole genome phylogeny we had previously determined (Lieberman et al., 2021). Unlike arp, which has some sequence variation at six positions per 60 bp repeat [see below; (Liu et al., 2007; Harper et al., 2008)], we did not find any tp0470 sequence changes in any of the 233 strains we examined, which included 211 strains we successfully sequenced by nanopore and the remainder from public databases. However, we did note wide variability in the number of repeats (Figure 1A), ranging between 4 and 51, with Nichols-like strains generally having more repeats than SS14-like strains [mean (median) repeats for Nichols and SS14 25.7 (27) and 15.3 (14), respectively; p < 2.2 × 10–16, Welch’s t-test] (Figure 1B), although this effect was difficult to disentangle from the strong location-specific effects we observed (Figure 1C, p < 2 × 10–16, ANOVA).
We then examined the number of arp repeats in each sample in the whole genome phylogeny. Among the 226 TPA strains with arp sequence information, including 203 strains we sequenced and the remainder from public databases, the majority (170, 83.7%) had 14 tandem repeats, 28 (13.8%) had ten repeats, and the remainder had between 4 and 24 repeats (Figure 2A, Supporting Information), consistent with previous reports of the prevalence of repeat lengths (Pillay et al., 1998; Harper et al., 2008; Marra et al., 2010). No relationship between tp0470 repeat length and arp repeat length was observed (Supplementary Figure 3, Pearson coefficient = 0.008), consistent with prior studies (Šmajs et al., 2018), even when strains containing 14 repeat arp sequences were removed (Pearson coefficient = 0.196). With the exception of the Nichols-like clade Laboratory Strain “Chicago” (NC_017268), which was excluded due to a known sequencing error in the reference sequence, all variants were in frame and SNVs limited to the six positions per 60 bp repeat previously recognized to be variable, resulting in four amino acid substitutions. Subclade-specific repeat length variation was noted (p < 2 × 10–16, ANOVA), with all but five samples in Nichols subclade B having ten repeats, three of the four strains in Nichols C with 19 repeats, and 15 or 16 repeats in the SS14 Mexico subclade (Figures 2A,B). When samples from Madagascar, which had a bimodal distribution of arp repeats, were removed from analysis, no repeat length variation was found among different countries (Figure 2C, p > 0.05, ANOVA). Among the 170 samples with 14 ARP repeats, nine different gene sequences, which we have named A–I in order of decreasing prevalence, were represented (Figure 2D), with different usage patterns in different subclades. For example, ARP14 variant A was found in 103 samples in SS14 subclades exclusively. ARP14 variants B and C, which differ by only three nucleotides within a single 60 bp repeat, are found exclusively within the Nichols A subclade, variant E found exclusively in Nichols B, and variant D found in Nichols subclades C, E, and the subclade containing the laboratory strains, Nichols D.
Figure 2. Variation in arp repeat length. (A) Recombination masked whole genome phylogeny (left) with the number of arp repeats for each strain (right). Sequence variant number is included as text to the right of each length bar. All data are also included in a tabular representation in Supplementary Table 2. Number of arp repeats by subclade (B) or country (C). (D) Multiple sequence alignment of the nine variants with 14 arp repeats. Variant positions are highlighted and bases colored red, blue, yellow, or green for A, C, G, or T, respectively. The number of strains with each variant sequence is included in the bar graph to the right of the multiple sequence alignment.
We also characterized the pattern of the modular 60 bp near-identical repeats. Three types of arp repeat (Type I, II, and III) were originally identified by Liu et al. (2007). A fourth type, Type II/III, which likely formed by recombination of Types II and III between the two sets of three variable positions to form a chimera, was discovered in a larger analysis of laboratory and clinical strains (Harper et al., 2008). In addition to these “canonical” types of arp repeat, we found three additional repeat Types that had not been previously described (Figure 3A): Type I/III, which appears to be a chimera of Types I and III and found only in a single Peruvian strain with seven arp repeats; Type III/I, which is a chimera of Type III and either Type I or II and found in a 14-repeat arp variant found in 17 samples from Madagascar as well as the Cuban strain CW83 (Grillová et al., 2019, found in 17); and Type IIIG, found in a single sample from the United States, which shares the Type III sequence at the first four variable positions and likely recombination to match Types I or II at the final two variable positions. All repeat Types generated unique amino acid sequences (Figure 3B). Consistent with previous reports that non-venereal T. pallidum subspecies use only Type II repeats (Harper et al., 2008; Cejková et al., 2012; Staudová et al., 2014), the single Lihir Island T. pallidum subsp. pertenue strain and four Japan T. pallidum subsp. endemicum strains included in our previous study (Lieberman et al., 2021) had only four Type II repeats, or ten or eleven Type II repeats, respectively (Supporting Information).
Figure 3. arp repeat type usage. (A) Nucleotide sequence of the arp repeat module types. Variable positions are highlighted. (B) Amino acid sequence of the arp repeat module types. Variable positions are highlighted. (C) Recombination masked whole genome phylogeny (left) with the repeat type usage per strain (right). The 60 bp arp repeats are colored by type.
To better visualize the relationships and pattern of repeat Type use between different arp variants, we plotted them in the context of the whole genome phylogeny (Figure 3C). In this context, several general patterns emerge. Most strikingly, out of 121 strains in the SS14-like clade with ARP sequence information, 115 include at least two Type II repeats, a penultimate Type II/III repeat, and a 3′ Type III repeat, in contrast to the Nichols-like clade, which instead contains four or more Type II repeats followed by one (Nichols subclade A) or two (Nichols subclades B–E) Type III repeats. Furthermore, with the exception of arp sequences in the Nichols B subclade, which start with a Type I repeat followed by Type II, arp variants in 99% of strains across both major clades start with two Type I repeats.
Within each subclade, we defined the dominant arp sequence as the one most commonly found. Out of the 226 T. pallidum subsp. pallidum strains with arp sequence information, we identified 170 strains with the dominant arp sequence in the subclade to which it belongs, 25 strains where the arp repeat sequence clearly did not match the dominant sequence in its subclade, and 26 strains that comprised three clusters of closely related strains all exhibiting the same arp variant but diverged from the predominant variant in the subclade to which they belonged. (“Dominant arp Variant in Subclade,” Supporting Information). The dominant strain in the SS14 Mexico or Nichols X subclades could not be determined, and Nichols D Lab Strain Chicago was excluded due to a known sequencing error. We did not find any SNVs enriched among strains with an arp sequence altered from the dominant strain (p > 0.05, Fisher’s exact test). We also examined the relationship between non-dominant arp sequence and branch length on the whole genome maximum likelihood tree. We found that the strains with the non-dominant arp sequence had terminal branch lengths (defined as the number of SNPs that separate a tip from its most recent ancestral node) that were on average 3.6 times longer than those with the dominant sequence [Supplementary Figure 4, mean (median) 4.11 (3) SNPs vs. 1.14 (0) SNPs, p = 0.0046, Welch’s t-test]. While these observations do not account for sources of selective pressure such as host immune response or anatomic site of infection, they do suggest that events that result in a novel arp sequence are likely stochastic and less frequent than SNP fixation, which occurs approximately once per genome every 5 years (Beale et al., 2019; Lieberman et al., 2021; Taouk et al., 2022).
In cases where a strain’s arp sequence did not match the dominant sequence in its subclade, we confirmed the sequence by examination of WGS short read linkage and/or Sanger sequencing. We then attempted to determine the simplest mechanism to generate the novel sequence. In most cases, a single intra-strain recombination event, resulting in insertion, deletion, or substitution of one or more arp modules, is most parsimonious (Supplementary Figure 5; most variants could theoretically be generated by addition or removal of modules from the dominant sequence in a different pattern than shown). For example, in the SS14 Omega’ and East Asia subclades, ARP14.A is most commonly modified presumptively through loss of repeat modules, resulting in sequence lengths between 7 and 12 modules. Notably, though, we do not know how long the recombination junctions must be, therefore sequences such as ARP11.B, which could have been formed by a recombination event between the 4th and 5th variable nucleotides of type III and type II modules as shown, or could also have been generated via deletion of the three tandem type II repeats in conjunction with mutation of the 5th and 6th variable positions in the type III repeat from A to G, to generate the novel Type IIIG module.
In addition to novel arp variants that can be generated via a single intrastrain recombination, there are other variants whose presumptive lineage is less clear. For example, the strain UAB46xei is very unusual, both starting and ending with type II modules, unlike any other arp variants. Interestingly, though, the UAB46xei arp sequence is 10 repeats, like the ARP10.A sequence that predominates the Nichols B subclade. Strains TPVMW082H and Dublin57B, belonging to Nichols X and SS14 Omega’ subclades, respectively, also contain unique sequences generated via complex mechanisms. However, it is plausible that several individual recombination events generated the 20-repeat variant found in TPVMW082H, which is only distantly related to any other strains in this dataset.
Other strains show clear evidence of inter-strain recombination. For example, ARP10.A is the dominant sequence in the Madagascar strains that comprise Nichols subclade B and found in no other strains except for the single Madagascar sample in the SS14 East Asia subclade. Similarly, although the dominant arp variant in the SS14 Mexico subclade cannot be determined since each strain has a unique sequence, the arp variant in SS14 Mexico strain MD06B is only shared with the SS14 Omega’ subclade strain MD51x, both of which were collected from Maryland, United States. Finally, strain Japan317x in Nichols subclade C harbors the same ARP14.D variant as is found in strains in Nichols subclades D and E, including in one Japan sample. However, deletion of five modules from the ARP19.A variant private to the Nichols C subclade is also a possible mechanism for generation of the 14.D variant in Japan317x. Together, these data suggest that both intra- and inter-strain recombination is employed by T. pallidum to generate diversity at the arp locus.
We also attempted to determine if tp0470 repeat length or arp repeat length and sequence were associated with syphilis stage. Among the 79 strains with stage information available, 49 were primary and 30 were secondary; although longer tp0470 variants were seen on average in secondary syphilis samples [Supplementary Figure 6A, mean (median) 23.6 (25) for secondary vs. 18.8 (15) for primary, p = 0.03624, Welch’s t-test], no significant differences in tp0470 repeat length by disease stage were observed when samples were further split by SS14- or Nichols-like clades, suggesting that sampling bias may be confounding interpretation of the association of tp0470 repeat length with disease stage. There was no association between non-dominant arp sequence and primary or secondary syphilis (p > 0.05, Fisher’s exact test), nor did we find a significant difference in the number of arp repeats among primary vs. secondary syphilis samples (Supplementary Figure 6B, p = 0.358, Welch’s t-test). However, secondary syphilis was overrepresented among strains with the ARP10.A sequence (Fisher’s Exact Test, p = 0.0171), while primary syphilis was overrepresented among strains with the ARP14.A sequence (Fisher’s Exact Test, p = 0.0188). No other arp sequence variants had enough samples with stage data to determine overrepresentation.
There is ample evidence the proteins encoded by tp0470 and arp genes are present during infection: Previous studies have shown that the tp0470 transcript is expressed (Smajs et al., 2005; De Lay et al., 2021), and sera from infected rabbits (McKevitt et al., 2005) and patients (Brinkman et al., 2006) are reactive to TP0470 protein. The arp transcript is expressed (Smajs et al., 2005; De Lay et al., 2021), and the ARP protein was found to be one of the top 10% most abundant proteins by mass spectrometry (Osbak et al., 2016). T. pallidum-infected rabbit sera are reactive to ARP (McKevitt et al., 2005; Liu et al., 2007), while sera from infected human patients are weakly reactive to ARP during primary infection (Brinkman et al., 2006). Therefore, we attempted to model select variants of the full-length proteins encoded by tp0470 and arp.
The tp0470 gene is identical in all strains included in this study outside of the repeat length variation, and TP0470 is confidently predicted by both SignalP 6.0 (Teufel et al., 2022) and PSORTb V3.0 (Yu et al., 2010) to contain a signal sequence with no lipid anchor, which suggests it resides in the periplasm. A conserved domain search (Lu et al., 2020) reveals the tetratricopeptide repeat protein domain (e-value: 1.52e–8) at the N-term of the protein, with no predicted conserved domains otherwise. This is consistent in structures predicted by both trRosetta (Yang et al., 2020; Du et al., 2021) and AlphaFold (Jumper et al., 2021; Varadi et al., 2022), which contain four pairs of antiparallel α-helices that comprise the conserved tetratricopeptide motif in the N terminus, followed by an extended α-helix largely composed of the highly charged eight amino acid repeat motif “EAEEARRK” (Figures 4A,B; tetratricopeptide repeat motifs in green, pre-repeat linker in gray, 15 repeats of eight amino acids in purple, post-repeat C terminus in gold). Confidence metrics for trRosetta are high for the overall structure (TM-score = 0.756), while for AlphaFold the local Difference Distance Test score is >80 (high) throughout the tetratricopeptide repeat domain, and drops throughout the length of the extended helix. Variants with longer tandem repeats are predicted to have a helix that folds back on itself by AlphaFold, while trRosetta predicts an elongated structure; although at low confidence (Supplementary Figures 7A,B). The length of the repeat portion of the helix ranges between 4 and 51 repeats, or a total of 32–408 residues in repeats, with a modal number of repeats of 15 (Supplementary Figure 7C). Assuming 0.54 nm in length per helical turn of 3.6 residues, the elongated length of the predicted helix may range between 11.5 and 68.1 nm including the non-repetitive 23 amino acids N terminal and 23 amino acids C terminal to the repeats, with 90% of lengths between 7 and 36 repeats (15.3–50.1 nm), and the helix of the 15 repeat variant measuring approximately 24.9 nm. Within the helix, a single eight-residue repeat makes just over two helical turns. Figure 4C zooms in on four repeats, comprising approximately nine helical turns. Although the orientation of amino acid residues in a structural model does not reflect the precise native conformation, the stick representation of sidechains (Figure 4C, top) and smoothed surface charge (Figure 4C, bottom) demonstrates the highly polar nature of the TP0470 repeats.
Figure 4. Structure predictions of TP0470. (A) trRosetta and (B) AlphaFold predictions of structure of 15 repeat TP0470 variant. N terminal tetratricopeptide repeat domain is shown in green, repeats are in purple, and C-terminal region is in gold. (C) APBS electrostatic surface potential (top) and stick model of sidechains (bottom) for portion of repeat helix.
We predicted domains and structures for select variants of the arp. By SignalP 6.0, it is predicted to have a signal sequence (probability = 0.67) and possibly lipidation site at cysteine-29 (probability = 0.33), however, PSORT predicts neither of these elements. A conserved domain search reveals a C-terminal SPOR domain (e-value: 7.5e–3), which in other proteins is a peptidoglycan binding domain (Yahashiri et al., 2017). Together, these results suggest that the arp is localized to the periplasm.
For model generation, we first examined the ARP14.A variant, by far the most common variant in our phylogeny, harbored by 103 strains. While both trRosetta and AlphaFold predicted the expected twisted β strand structure of the C-terminal SPOR domain (Figures 5A,B, magenta), AlphaFold’s low confidence prediction of the repeat regions (local Distance Difference Test ∼40) is entirely unstructured (Figure 5B), whereas the trRosetta prediction is for the acidic repeats to form a disordered linker comprising the first five repeats, followed by a parallel β-sheet structure that contains nine strands composed of the last nine acidic repeats (Figure 5A), although the confidence in the prediction is quite low (TM-score = 0.288). This structure would contain an extremely acidic face of the β-sheet (Figure 5C) with a periodicity of 20 residues, the same as the repeat. Interestingly, the repeat region in most other variants was not predicted by trRosetta to fold into a beta sheet, rather, they were highly disordered (Supplementary Figure 8); only variants ARP14.H and ARP15.A were also predicted to form a β-sheet from the repeats. Overall, despite a plausible structure for some variants, structural modeling of the arp remains challenging with only low confidence models returned by two methods and any attempt to infer function based on these results should be made cautiously.
Figure 5. Structure predictions ARP14A. (A) trRosetta and (B) AlphaFold predictions of structure of ARP14A. C-terminal SPOR domain is shown in magenta, repeats in cyan. (C) APBS electrostatic surface potential for trRosetta ARP structure from panel (A). Red denotes negative charge (acidic) and blue denotes positive charge (basic).
Despite the relatively low rate of SNP fixation, with a mean rate of approximately 1–3 × 10–7 substitutions per site per year in putative non-recombinogenic loci (Beale et al., 2019; Lieberman et al., 2021; Taouk et al., 2022), T. pallidum uses additional mechanisms to increase its genetic diversity and antigenic repertoire. These include inter-strain and inter-species recombination in genes encoding the Tpr family of antigens (Gray et al., 2006; Kumar et al., 2018; Grillová et al., 2019 sne), gene conversion in the variable regions of tprK (Centurion-Lara et al., 2004; Giacani et al., 2010; Reid et al., 2014), and homopolymer expansion and contraction to alter promoter activity and hence expression level of putative outer membrane proteins (Giacani et al., 2015). Previous work has demonstrated that diversity in the tp0470 and arp repeat length, and repeat type usage in arp, is likely generated by recombination, although modification of the number of repeats in the tp0470 could also be possible via a polymerase slippage mechanism. Our current study extends these findings to a large cohort of clinical samples with near-complete genomes available, enabling examination of differences between subclades and correlation with genome features.
From our results, it is clear that a very wide distribution of tp0470 repeat lengths is possible but with no sequence variation within the repeat. Although T. pallidum has an extremely low rate of mutation and most genes are highly conserved, the absence of sequence variation within the tp0470 gene outside of repeat length variation suggests the protein may be under purifying selection. The arp gene has multiple sequence variants generated by using different repeat module types in a tandem arrangement, but is highly enriched for sequences with fourteen repeats. For both genes, some differences between Nichols- and SS14-like clades are observed: in tp0470, there is a slight increase in repeat length in the Nichols-like clade vs. SS14. In the arp gene, variants are generally limited to a single subclade, particularly in the Nichols-like clade, which has far greater genetic diversity than the SS14-like clade, with an average pairwise SNP distance of 42, as compared to an average pairwise SNP distance of 10 for the SS14-like clade. It is unclear whether differences between tp0470 lengths or repeat module pattern in arp between subclades have functional consequences and are being selected for, or whether the differences simply reflect random events during diversification. In the case of arp, we did not find any SNPs throughout the genome that correlated with an unexpected repeat sequence.
Until very recently (Romeis et al., 2021), no reverse genetics system for T. pallidum existed, therefore, traditional bacteriological genetic tools, such as mutants and knockout strains, to interrogate gene functions have not been available for the syphilis spirochete. Prior to the development of an epithelial cell co-culture system in 2018 (Edmondson et al., 2018), T. pallidum could only be passaged through rabbit testes, precluding forward genetics screens. While proteome-wide bioinformatic structural predictions have helped to shed light on the likely role of conserved structural domains (Houston et al., 2018), the structure and function T. pallidum proteins containing novel motifs, such as the repeat sequences found in TP0470 and ARP, remain unknown. To gain insight into their biological function and possible role in syphilis pathogenesis, we employed several in silico tools to predict the topology and structure of full-length ARP and TP0470 proteins.
The presence of a signal peptide on TP0470 is strong evidence that it is localized to the periplasm, where it likely binds other as-yet-undermined protein(s) via its N-terminal tetratricopeptide motif, which was predicted by both trRosetta and AlphaFold. Both algorithms predicted an extended α-helix with regions of alternating positive and negative surface electrostatic potential, regardless of the length of the repeats. Although TP0470 does not have any known interacting partners, it seems plausible that in addition to interactions formed by tetratricopeptide motif, the unusually long, very polar α-helix that comprises the repeats also serves to mediate protein-protein interactions.
Although a signal peptide was not confidently predicted by one tool (SignalP 6.0) and not predicted at all by a second (PSORT), the presence of a C-terminal SPOR domain, which binds denuded peptidoglycan, strongly suggests ARP must be present in the periplasm. However, it is less clear whether ARP is free in the periplasm or is acylated at cys-29 (weakly predicted by SignalP 6.0), tethering it to the inner membrane. Both topologies are consistent with other SPOR domain-containing proteins (Yahashiri et al., 2017); mass spectrometry, Edman degradation, or other biochemical techniques will be necessary to resolve this question.
In addition to the unclear localization of ARP, the structure formed by the ARP repeat modules remains murky. The most biologically plausible structure generated by the modeling software is of the modular repeats forming a parallel beta sheet, with periodicity of 20 residues, the same length as the repeats. The β-sheet and the loops that connect the strands form an extremely negatively charged surface; it seems likely that whatever the ARP repeat domain binds, it will be positively charged.
Figure 6 summarizes our current understanding of the structure and topology of TP0470 and ARP. Because TP0470 is predicted to be soluble, it may be able to traverse the peptidoglycan layer through pores. In contrast, ARP may be associated with the inner membrane, constraining its movement within the periplasm. However, these models do not resolve why there is tremendous diversity of tp0470 repeat lengths, and arp repeat lengths and repeat module usage. To answer these questions and determine the biological function of the repeat domains, extensive biochemical and biophysical studies of different variants will be necessary.
Figure 6. Model showing ARP and TP0470 cellular location and putative interactions. Both ARP and TP0470 are localized to the periplasm. The ARP N terminus may be acylated at cysteine 29. OM, outer membrane; IM, inner membrane; PG, peptidoglycan. Proteins and cellular structures have not been drawn to scale.
One of the primary limitations of our genomic dataset is that of bias introduced by unequal sampling. For example, although we and others have reported that 14-repeat arp variants predominate, particularly variant 14.A, this may reflect the increased sampling in geographical regions (Europe and United States) where SS14-like clade strains predominate. Furthermore, many of the strains included in our study were not collected with extensive clinical histories or patient characteristics, limiting our ability to infer functional differences from sequence variation. Finally, the use of PCR to interrogate genes containing repetitive sequences, particularly GC rich repeats like tp0470, is always challenging due to the generation of artifact products due to polymerase slippage. These can amplify preferentially over the “real” product, as we saw for tp0470 in some samples (Supplementary Figure 1B). Suspected artifact products were readily apparent in both the gel images and histograms of mapped reads; therefore the data remained interpretable, and were consistent in technical replicates (Supporting Information). However, we cannot rule out that these small bands represent true intrastrain heterogeneity, as has been previously observed (Marra et al., 2010).
In addition to developing a novel method to examine repeat length and sequence in two challenging genomic loci in T. pallidum, our study has demonstrated extensive tp0470 and arp repeat diversity among more than 200 clinical strains with whole genome sequence, by far the largest study of these genes to date. Importantly, we found that more than 10% of strains contained an arp variant that had a different length and sequence than the dominant variant in the subclade, which builds on concerns about the utility of using the number of arp repeats as part of strain typing tools for epidemiology (Mikalová et al., 2013). Finally, we have proposed a possible mechanism by which each may interact with peptidoglycan and/or other periplasmic factors and influence morphogenesis. Although additional genetic, biophysical, and biochemical interaction studies will be necessary to characterize their function and elucidate their binding partners, our study of tp0470 and arp lays the foundation to directly link the genotype to function of two novel genes that may influence T. pallidum pathogenesis.
The datasets presented in this study can be found in online repositories. The names of the repository/repositories and accession number(s) can be found below: https://www.ncbi.nlm.nih.gov/, Bioprojects PRJNA723099 and PRJNA815321.
All human samples were collected and deidentified following protocols established at each institution. All IRB information from samples collected in Japan, Italy, Ireland, Maryland, United States, Madagascar, Peru, Papua New Guinea, and Nanjing, China has been previously published (Hopkins et al., 2004; Lukehart et al., 2004; Van Damme et al., 2009; Hook et al., 2010; Marra et al., 2010; Lieberman et al., 2021). Collection of additional samples was covered by the following IRBs: Malawi: National Health Sciences Research Committee Ministry of Health and Population (IRB Approval Number 2252); Colombia: Centro Internacional de Entrenamiento e Investigaciones Medicas (CIDEIM) Institutional Human Research Ethics Committee (CIEIH) (IRB protocol number 1289); Guangzhou, China: Dermatology Hospital of Southern Medical University (SMU) Medical Ethic Committee [IRB protocol number GDDHLS-20181202(R3)]; Chapel Hill, United States: University of North Carolina IRB Protocol Number 19-0311. Sequencing of deidentified strains was covered by the University of Washington Institutional Review Board (IRB) protocol number STUDY00000885 and University of North Carolina IRB protocol number 19-0311. The patients/participants provided their written informed consent to participate in this study.
NL, LG, and AG designed the study. NL wrote the bioinformatic pipelines, analyzed and interpreted the data, wrote the manuscript, and generated the figures. TA and BC developed the methods. TA, BC, DP, and CH generated and processed the raw data. CH, AH, and ER extracted DNA from clinical specimens. Q-QW, R-LZ, C-XK, GC, IC, MC, FD, S-IN, KL, MO, KK, SV, ME, CC, JK, OM, AR, FM, EH, IH, MM, HZ, BY, EL-M, and LR managed specimen procurement at clinical sites. KH generated the figures. JR, KH, SL, JP, LG, and AG offered expert interpretation and contextualization of results. JR, KH, JS, AS, JP, LG, and AG provided the funding. All authors edited the final manuscript.
This work was supported, in whole or in part, by the Bill and Melinda Gates Foundation INV-036560. Under the grant conditions of the Foundation, a Creative Commons Attribution 4.0 Generic License has already been assigned to the author accepted manuscript version that might arise from this submission (AG, LG, JP, AS, JR, and KH). This work was further supported by NIAID grant numbers U19AI144133 (LG and AG), U19AI144177 (JR, KH, JS, AS, and JP), and R01AI139265 (to JK), as well as grants from Ministry of Education, Culture, Sports, Science and Technology of Japan (number 21K09388) to S-IN and from Japan Agency for Medical Research and Development (number 21fk0108091j0303) to MO. Additional research funds were generously provided by Connecticut Children’s (JS, JR, and KH).
The authors declare that the research was conducted in the absence of any commercial or financial relationships that could be construed as a potential conflict of interest.
All claims expressed in this article are solely those of the authors and do not necessarily represent those of their affiliated organizations, or those of the publisher, the editors and the reviewers. Any product that may be evaluated in this article, or claim that may be made by its manufacturer, is not guaranteed or endorsed by the publisher.
The Supplementary Material for this article can be found online at: https://www.frontiersin.org/articles/10.3389/fmicb.2022.1007056/full#supplementary-material
Supplementary Figure 1 | tp0470 PCR band and nanopore pipeline call concordance of select samples. (A) PCR bands following barcoding. Bands should have a size of 24 bp times the number of repeats plus 273, including both flanking regions (225 bp) and two barcodes (48 bp total). The red box highlights non-specific amplification of low molecular weight fragments, while the red arrow shows the correct band size. (B) Histogram of the distribution of reads aligned to each length variant in the mapping reference file. (C) Distribution of the percent of all aligned reads in the top hit for all samples.
Supplementary Figure 2 | arp PCR band and nanopore pipeline call concordance of select samples. (A) PCR bands following barcoding. Bands should have a size of 60 bp times the number of repeats plus 874, including both flanking regions (826 bp) and two barcodes (48 bp total). (B) Histogram of the distribution of reads aligned to each length variant in the mapping reference file.
Supplementary Figure 3 | arp repeat length is not correlated with tp0470 repeats. No correlation was seen between number of arp and tp0470 repeats (Pearson coefficient = 0.008).
Supplementary Figure 4 | Terminal branch lengths are longer for strains with non-dominant arp sequences. Average branch lengths from tip to ancestral node were determined for strains with dominant and non-dominant arp variants. p = 0.0046, Welch’s t-test.
Supplementary Figure 5 | Possible single recombination events to generate arp variants from the dominant arp variant in each subclade. The dominant sequence from each subclade is shown in the top position for each pair, and the non-dominant (possibly nascent) variant below. Dotted lines show possible junctions. Only a single possibility per variant is shown.
Supplementary Figure 6 | Longer tp0470 repeats are associated with secondary syphilis. Out of 79 samples with stage information, samples from secondary syphilis had on average approximately five more repeats than primary p = 0.03624, Welch’s t-test.
Supplementary Figure 7 | TP0470 predictions: (A) trRosetta and (B) AlphaFold predictions of structure of 51 repeat TP0470 variant. Structures are colored from blue to red N term to C term. (C) Distribution of tp0470 variants in phylogeny.
Supplementary Figure 8 | trRosetta predictions for additional ARP variants. Structures are colored from blue to red N term to C term.
Arora, N., Schuenemann, V. J., Jäger, G., Peltzer, A., Seitz, A., Herbig, A., et al. (2017). Origin of modern syphilis and emergence of a pandemic Treponema pallidum cluster. Nat. Microbiol. 2:16245. doi: 10.1038/nmicrobiol.2016.245
Beale, M. A., Marks, M., Cole, M. J., Lee, M.-K., Pitt, R., Ruis, C., et al. (2021). Global phylogeny of Treponema pallidum lineages reveals recent expansion and spread of contemporary syphilis. Nat. Microbiol. 6, 1549–1560. doi: 10.1038/s41564-021-01000-z
Beale, M. A., Marks, M., Sahi, S. K., Tantalo, L. C., Nori, A. V., French, P., et al. (2019). Genomic epidemiology of syphilis reveals independent emergence of macrolide resistance across multiple circulating lineages. Nat. Commun. 10:3255. doi: 10.1038/s41467-019-11216-7
Brinkman, M. B., McKevitt, M., McLoughlin, M., Perez, C., Howell, J., Weinstock, G. M., et al. (2006). Reactivity of antibodies from syphilis patients to a protein array representing the Treponema pallidum proteome. J. Clin. Microbiol. 44, 888–891. doi: 10.1128/JCM.44.3.888-891.2006
Bushnell, B. (2014). BBMap Short Read Aligner, and Other Bioinformatic Tools. Berkeley, CA: Ernest Orlando Lawrence Berkeley National Laboratory.
Cejková, D., Zobaníková, M., Chen, L., Pospíšilová, P., Strouhal, M., Qin, X., et al. (2012). Whole genome sequences of three Treponema pallidum ssp. pertenue strains: Yaws and syphilis treponemes differ in less than 0.2% of the genome sequence. PLoS Negl. Trop. Dis. 6:e1471. doi: 10.1371/journal.pntd.0001471
Centurion-Lara, A., LaFond, R. E., Hevner, K., Godornes, C., Molini, B. J., Van Voorhis, W. C., et al. (2004). Gene conversion: A mechanism for generation of heterogeneity in the tprK gene of Treponema pallidum during infection. Mol. Microbiol. 52, 1579–1596. doi: 10.1111/j.1365-2958.2004.04086.x
Cerveny, L., Straskova, A., Dankova, V., Hartlova, A., Ceckova, M., Staud, F., et al. (2013). Tetratricopeptide repeat motifs in the world of bacterial pathogens: Role in virulence mechanisms. Infect. Immun. 81, 629–635. doi: 10.1128/IAI.01035-12
Chen, W., Šmajs, D., Hu, Y., Ke, W., Pospíšilová, P., Hawley, K. L., et al. (2021). Analysis of Treponema pallidum Strains From China Using Improved Methods for Whole-Genome Sequencing From Primary Syphilis Chancres. J. Infect. Dis. 223, 848–853. doi: 10.1093/infdis/jiaa449
Currin, A., Swainston, N., Dunstan, M. S., Jervis, A. J., Mulherin, P., Robinson, C. J., et al. (2019). Highly multiplexed, fast and accurate nanopore sequencing for verification of synthetic DNA constructs and sequence libraries. Synth. Biol. 4:ysz025. doi: 10.1093/synbio/ysz025
De Lay, B. D., Cameron, T. A., De Lay, N. R., Norris, S. J., and Edmondson, D. G. (2021). Comparison of transcriptional profiles of Treponema pallidum during experimental infection of rabbits and in vitro culture: Highly similar, yet different. PLoS Pathog. 17:e1009949. doi: 10.1371/journal.ppat.1009949
Du, Z., Su, H., Wang, W., Ye, L., Wei, H., Peng, Z., et al. (2021). The trRosetta server for fast and accurate protein structure prediction. Nat. Protoc. 16, 5634–5651. doi: 10.1038/s41596-021-00628-9
Edmondson, D. G., Hu, B., and Norris, S. J. (2018). Long-Term In Vitro Culture of the Syphilis Spirochete Treponema pallidum subsp. pallidum. mBio 9:e01153–18. doi: 10.1128/mBio.01153-18
Flasarová, M., Pospíšilová, P., Mikalová, L., Vališová, Z., Dastychová, E., Strnadel, R., et al. (2012). Sequencing-based molecular typing of treponema pallidum strains in the Czech Republic: All identified genotypes are related to the sequence of the SS14 strain. Acta Derm. Venereol. 92, 669–674. doi: 10.2340/00015555-1335
Giacani, L., Brandt, S. L., Ke, W., Reid, T. B., Molini, B. J., Iverson-Cabral, S., et al. (2015). Transcription of TP0126, Treponema pallidum putative OmpW homolog, is regulated by the length of a homopolymeric guanosine repeat. Infect. Immun. 83, 2275–2289. doi: 10.1128/IAI.00360-15
Giacani, L., Molini, B. J., Kim, E. Y., Godornes, B. C., Leader, B. T., Tantalo, L. C., et al. (2010). Antigenic variation in Treponema pallidum: TprK sequence diversity accumulates in response to immune pressure during experimental syphilis. J. Immunol. 184, 3822–3829. doi: 10.4049/jimmunol.0902788
Gray, R. R., Mulligan, C. J., Molini, B. J., Sun, E. S., Giacani, L., Godornes, C., et al. (2006). Molecular evolution of the tprC, D, I, K, G, and J genes in the pathogenic genus Treponema. Mol. Biol. Evol. 23, 2220–2233. doi: 10.1093/molbev/msl092
Grillová, L., Oppelt, J., Mikalová, L., Nováková, M., Giacani, L., Niesnerová, A., et al. (2019). Directly Sequenced Genomes of Contemporary Strains of Syphilis Reveal Recombination-Driven Diversity in Genes Encoding Predicted Surface-Exposed Antigens. Front. Microbiol. 10:1691. doi: 10.3389/fmicb.2019.01691
Grimes, M., Sahi, S. K., Godornes, B. C., Tantalo, L. C., Roberts, N., Bostick, D., et al. (2012). Two mutations associated with macrolide resistance in Treponema pallidum: Increasing prevalence and correlation with molecular strain type in Seattle, Washington. Sex. Transm. Dis. 39, 954–958. doi: 10.1097/OLQ.0b013e31826ae7a8
Harper, K. N., Liu, H., Ocampo, P. S., Steiner, B. M., Martin, A., Levert, K., et al. (2008). The sequence of the acidic repeat protein (arp) gene differentiates venereal from nonvenereal Treponema pallidum subspecies, and the gene has evolved under strong positive selection in the subspecies that causes syphilis. FEMS Immunol. Med. Microbiol. 53, 322–332. doi: 10.1111/j.1574-695X.2008.00427.x
Hook, E. W., Behets, F., Van Damme, K., Ravelomanana, N., Leone, P., Sena, A. C., et al. (2010). A Phase III Equivalence Trial of Azithromycin versus Benzathine Penicillin for Treatment of Early Syphilis. J. Infect. Dis. 201, 1729–1735. doi: 10.1086/652239
Hopkins, S., Lyons, F., Coleman, C., Courtney, G., Bergin, C., and Mulcahy, F. (2004). Resurgence in Infectious Syphilis in Ireland: An Epidemiological Study. Sex. Transm. Dis. 31, 317–321. doi: 10.1097/01.OLQ.0000123653.84940.59
Houston, S., Lithgow, K. V., Osbak, K. K., Kenyon, C. R., and Cameron, C. E. (2018). Functional insights from proteome-wide structural modeling of Treponema pallidum subspecies pallidum, the causative agent of syphilis. BMC Struct. Biol. 18:7. doi: 10.1186/s12900-018-0086-3
Jumper, J., Evans, R., Pritzel, A., Green, T., Figurnov, M., Ronneberger, O., et al. (2021). Highly accurate protein structure prediction with AlphaFold. Nature 596, 583–589. doi: 10.1038/s41586-021-03819-2
Kumar, S., Caimano, M. J., Anand, A., Dey, A., Hawley, K. L., LeDoyt, M. E., et al. (2018). Sequence Variation of Rare Outer Membrane Protein β-Barrel Domains in Clinical Strains Provides Insights into the Evolution of Treponema pallidum subsp. pallidum, the Syphilis Spirochete. mBio 9:e01006–18. doi: 10.1128/mBio.01006-18
Li, H., and Durbin, R. (2009). Fast and accurate short read alignment with Burrows-Wheeler transform. Bioinformatics 25, 1754–1760. doi: 10.1093/bioinformatics/btp324
Lieberman, N. A. P., Lin, M. J., Xie, H., Shrestha, L., Nguyen, T., Huang, M.-L., et al. (2021). Treponema pallidum genome sequencing from six continents reveals variability in vaccine candidate genes and dominance of Nichols clade strains in Madagascar. PLoS Negl. Trop. Dis. 15:e0010063. doi: 10.1371/journal.pntd.0010063
Liu, D., He, S.-M., Zhu, X.-Z., Liu, L.-L., Lin, L.-R., Niu, J.-J., et al. (2020). Molecular Characterization Based on MLST and ECDC Typing Schemes and Antibiotic Resistance Analyses of Treponema pallidum subsp. pallidum in Xiamen, China. Front. Cell. Infect. Microbiol. 10:618747. doi: 10.3389/fcimb.2020.618747
Liu, H., Rodes, B., George, R., and Steiner, B. (2007). Molecular characterization and analysis of a gene encoding the acidic repeat protein (Arp) of Treponema pallidum. J. Med. Microbiol. 56, 715–721. doi: 10.1099/jmm.0.46943-0
Lu, S., Wang, J., Chitsaz, F., Derbyshire, M. K., Geer, R. C., Gonzales, N. R., et al. (2020). CDD/SPARCLE: The conserved domain database in 2020. Nucl. Acids Res. 48:D265–D268. doi: 10.1093/nar/gkz991
Lukehart, S. A., Godornes, C., Molini, B. J., Sonnett, P., Hopkins, S., Mulcahy, F., et al. (2004). Macrolide resistance in Treponema pallidum in the United States and Ireland. N. Engl. J. Med. 351, 154–158. doi: 10.1056/NEJMoa040216
Marra, C. M., Sahi, S. K., Tantalo, L. C., Godornes, C., Reid, T., Behets, F., et al. (2010). Enhanced molecular typing of treponema pallidum: Geographical distribution of strain types and association with neurosyphilis. J. Infect. Dis. 202, 1380–1388. doi: 10.1086/656533
McKevitt, M., Brinkman, M. B., McLoughlin, M., Perez, C., Howell, J. K., Weinstock, G. M., et al. (2005). Genome scale identification of Treponema pallidum antigens. Infect. Immun. 73, 4445–4450. doi: 10.1128/IAI.73.7.4445-4450.2005
Mikalová, L., Pospíšilová, P., Woznicová, V., Kuklová, I., Zákoucká, H., and Smajs, D. (2013). Comparison of CDC and sequence-based molecular typing of syphilis treponemes: Tpr and arp loci are variable in multiple samples from the same patient. BMC Microbiol. 13:178. doi: 10.1186/1471-2180-13-178
Molepo, J., Pillay, A., Weber, B., Morse, S. A., and Hoosen, A. A. (2007). Molecular typing of Treponema pallidum strains from patients with neurosyphilis in Pretoria, South Africa. Sex. Transm. Infect. 83, 189–192. doi: 10.1136/sti.2006.023895
Noda, A. A., Méndez, M., Rodríguez, I., and Šmajs, D. (2022). Genetic Recombination in Treponema pallidum: Implications for Diagnosis, Epidemiology, and Vaccine Development. Sex. Transm. Dis. 49:e7–e10.
Osbak, K. K., Houston, S., Lithgow, K. V., Meehan, C. J., Strouhal, M., Šmajs, D., et al. (2016). Characterizing the Syphilis-Causing Treponema pallidum ssp. pallidum Proteome Using Complementary Mass Spectrometry. PLoS Negl. Trop. Dis. 10:e0004988. doi: 10.1371/journal.pntd.0004988
Parr, J. B. (2021). IDEELResearch/Tpallidum_Genomics: Tpallidum_Genomics. Honolulu: Zenodo, doi: 10.5281/ZENODO.5773174
Pětrošová, H., Zobaníková, M., Čejková, D., Mikalová, L., Pospíšilová, P., Strouhal, M., et al. (2012). Whole genome sequence of Treponema pallidum ssp. pallidum, strain Mexico A, suggests recombination between yaws and syphilis strains. PLoS Negl. Trop. Dis. 6:e1832. doi: 10.1371/journal.pntd.0001832
Pillay, A., Liu, H., Chen, C. Y., Holloway, B., Sturm, A. W., Steiner, B., et al. (1998). Molecular subtyping of Treponema pallidum subspecies pallidum. Sex. Transm. Dis. 25, 408–414. doi: 10.1097/00007435-199809000-00004
Pillay, A., Liu, H., Ebrahim, S., Chen, C. Y., Lai, W., Fehler, G., et al. (2002). Molecular typing of Treponema pallidum in South Africa: Cross-sectional studies. J. Clin. Microbiol. 40, 256–258. doi: 10.1128/JCM.40.1.256-258.2002
Pinto, M., Borges, V., Antelo, M., Pinheiro, M., Nunes, A., Azevedo, J., et al. (2016). Genome-scale analysis of the non-cultivable Treponema pallidum reveals extensive within-patient genetic variation. Nat. Microbiol. 2:16190. doi: 10.1038/nmicrobiol.2016.190
Pope, V., Fox, K., Liu, H., Marfin, A. A., Leone, P., Seña, A. C., et al. (2005). Molecular subtyping of Treponema pallidum from North and South Carolina. J. Clin. Microbiol. 43, 3743–3746. doi: 10.1128/JCM.43.8.3743-3746.2005
Reid, T. B., Molini, B. J., Fernandez, M. C., and Lukehart, S. A. (2014). Antigenic variation of TprK facilitates development of secondary syphilis. Infect. Immun. 82, 4959–4967. doi: 10.1128/IAI.02236-14
Romeis, E., Tantalo, L., Lieberman, N., Phung, Q., Greninger, A., and Giacani, L. (2021). Genetic engineering of Treponema pallidum subsp. pallidum, the Syphilis Spirochete. PLoS Pathog. 17:e1009612. doi: 10.1371/journal.ppat.1009612
Smajs, D., McKevitt, M., Howell, J. K., Norris, S. J., Cai, W.-W., Palzkill, T., et al. (2005). Transcriptome of Treponema pallidum: Gene expression profile during experimental rabbit infection. J. Bacteriol. 187, 1866–1874. doi: 10.1128/JB.187.5.1866-1874.2005
Šmajs, D., Strouhal, M., and Knauf, S. (2018). Genetics of human and animal uncultivable treponemal pathogens. Infect. Genet. Evol. 61, 92–107. doi: 10.1016/j.meegid.2018.03.015
Staudová, B., Strouhal, M., Zobaníková, M., Cejková, D., Fulton, L. L., Chen, L., et al. (2014). Whole genome sequence of the Treponema pallidum subsp. endemicum strain Bosnia A: The genome is related to yaws treponemes but contains few loci similar to syphilis treponemes. PLoS Negl. Trop. Dis. 8:e3261. doi: 10.1371/journal.pntd.0003261
Sutton, M. Y., Liu, H., Steiner, B., Pillay, A., Mickey, T., Finelli, L., et al. (2001). Molecular subtyping of Treponema pallidum in an Arizona County with increasing syphilis morbidity: Use of specimens from ulcers and blood. J. Infect. Dis. 183, 1601–1606. doi: 10.1086/320698
Taouk, M. L., Taiaroa, G., Pasricha, S., Herman, S., Chow, E. P. F., Azzatto, F., et al. (2022). Characterisation of Treponema pallidum lineages within the contemporary syphilis outbreak in Australia: A genomic epidemiological analysis. Lancet Microbe 3:e417–e426. doi: 10.1016/S2666-5247(22)00035-0
Teufel, F., Almagro Armenteros, J. J., Johansen, A. R., Gíslason, M. H., Pihl, S. I., Tsirigos, K. D., et al. (2022). SignalP 6.0 predicts all five types of signal peptides using protein language models. Nat. Biotechnol. 40, 1023–1025. doi: 10.1038/s41587-021-01156-3
Thurlow, C. M., Joseph, S. J., Ganova-Raeva, L., Katz, S. S., Pereira, L., Chen, C., et al. (2022). Selective Whole-Genome Amplification as a Tool to Enrich Specimens with Low Treponema pallidum Genomic DNA Copies for Whole-Genome Sequencing. mSphere 7:e0000922. doi: 10.1128/msphere.00009-22
Van Damme, K., Behets, F., Ravelomanana, N., Godornes, C., Khan, M., Randrianasolo, B., et al. (2009). Evaluation of Azithromycin Resistance in Treponema pallidum Specimens From Madagascar. Sex. Transm. Dis. 36, 775–776. doi: 10.1097/OLQ.0b013e3181bd11dd
Varadi, M., Anyango, S., Deshpande, M., Nair, S., Natassia, C., Yordanova, G., et al. (2022). AlphaFold Protein Structure Database: Massively expanding the structural coverage of protein-sequence space with high-accuracy models. Nucl. Acids Res. 50:D439–D444. doi: 10.1093/nar/gkab1061
Wang, L.-G., Lam, T. T.-Y., Xu, S., Dai, Z., Zhou, L., Feng, T., et al. (2020). Treeio: An R Package for Phylogenetic Tree Input and Output with Richly Annotated and Associated Data. Mol. Biol. Evol. 37, 599–603. doi: 10.1093/molbev/msz240
Wick, R. R., Judd, L. M., and Holt, K. E. (2019). Performance of neural network basecalling tools for Oxford Nanopore sequencing. Genome Biol. 20:129. doi: 10.1186/s13059-019-1727-y
Wick, R. R., Judd, L. M., Gorrie, C. L., and Holt, K. E. (2017). Completing bacterial genome assemblies with multiplex MinION sequencing. Microb. Genom. 3:e000132. doi: 10.1099/mgen.0.000132
Wickham, H. (2016). ggplot2: Elegant Graphics for Data Analysis, 2nd Edn. Berlin: Springer, doi: 10.1007/978-3-319-24277-4
Yahashiri, A., Jorgenson, M. A., and Weiss, D. S. (2017). The SPOR Domain, a Widely Conserved Peptidoglycan Binding Domain That Targets Proteins to the Site of Cell Division. J. Bacteriol. 199:e00118–17. doi: 10.1128/JB.00118-17
Yang, J., Anishchenko, I., Park, H., Peng, Z., Ovchinnikov, S., and Baker, D. (2020). Improved protein structure prediction using predicted interresidue orientations. Proc. Natl. Acad. Sci. U.S.A. 117, 1496–1503. doi: 10.1073/pnas.1914677117
Yu, G., Smith, D. K., Zhu, H., Guan, Y., and Lam, T. T. (2017). ggtree: An R package for visualization and annotation of phylogenetic trees with their covariates and other associated data. Methods Ecol. Evol. 8, 28–36. doi: 10.1111/2041-210X.12628
Yu, N. Y., Wagner, J. R., Laird, M. R., Melli, G., Rey, S., Lo, R., et al. (2010). PSORTb 3.0: Improved protein subcellular localization prediction with refined localization subcategories and predictive capabilities for all prokaryotes. Bioinformatics 26, 1608–1615. doi: 10.1093/bioinformatics/btq249
Zhou, L., Feng, T., Xu, S., Gao, F., Lam, T. T., Wang, Q., et al. (2022). ggmsa: A visual exploration tool for multiple sequence alignment and associated data. Brief. Bioinform. 22:bbac222. doi: 10.1093/bib/bbac222
Keywords: Treponema pallidum, syphilis, nanopore, genomics, AlphaFold, trRosetta, next generation sequencing (NGS), SPOR domain
Citation: Lieberman NAP, Armstrong TD, Chung B, Pfalmer D, Hennelly CM, Haynes A, Romeis E, Wang Q-Q, Zhang R-L, Kou C-X, Ciccarese G, Conte ID, Cusini M, Drago F, Nakayama S-i, Lee K, Ohnishi M, Konda KA, Vargas SK, Eguiluz M, Caceres CF, Klausner JD, Mitja O, Rompalo A, Mulcahy F, Hook EW III, Hoffman IF, Matoga MM, Zheng H, Yang B, Lopez-Medina E, Ramirez LG, Radolf JD, Hawley KL, Salazar JC, Lukehart SA, Seña AC, Parr JB, Giacani L and Greninger AL (2022) High-throughput nanopore sequencing of Treponema pallidum tandem repeat genes arp and tp0470 reveals clade-specific patterns and recapitulates global whole genome phylogeny. Front. Microbiol. 13:1007056. doi: 10.3389/fmicb.2022.1007056
Received: 29 July 2022; Accepted: 22 August 2022;
Published: 20 September 2022.
Edited by:
Humberto J. Debat, Instituto Nacional de Tecnología Agropecuaria, ArgentinaReviewed by:
Helena Petrosova, University of Victoria, CanadaCopyright © 2022 Lieberman, Armstrong, Chung, Pfalmer, Hennelly, Haynes, Romeis, Wang, Zhang, Kou, Ciccarese, Conte, Cusini, Drago, Nakayama, Lee, Ohnishi, Konda, Vargas, Eguiluz, Caceres, Klausner, Mitja, Rompalo, Mulcahy, Hook, Hoffman, Matoga, Zheng, Yang, Lopez-Medina, Ramirez, Radolf, Hawley, Salazar, Lukehart, Seña, Parr, Giacani and Greninger. This is an open-access article distributed under the terms of the Creative Commons Attribution License (CC BY). The use, distribution or reproduction in other forums is permitted, provided the original author(s) and the copyright owner(s) are credited and that the original publication in this journal is cited, in accordance with accepted academic practice. No use, distribution or reproduction is permitted which does not comply with these terms.
*Correspondence: Alexander L. Greninger, YWdyZW5pbmdAdXcuZWR1
†These authors have contributed equally to this work
Disclaimer: All claims expressed in this article are solely those of the authors and do not necessarily represent those of their affiliated organizations, or those of the publisher, the editors and the reviewers. Any product that may be evaluated in this article or claim that may be made by its manufacturer is not guaranteed or endorsed by the publisher.
Research integrity at Frontiers
Learn more about the work of our research integrity team to safeguard the quality of each article we publish.