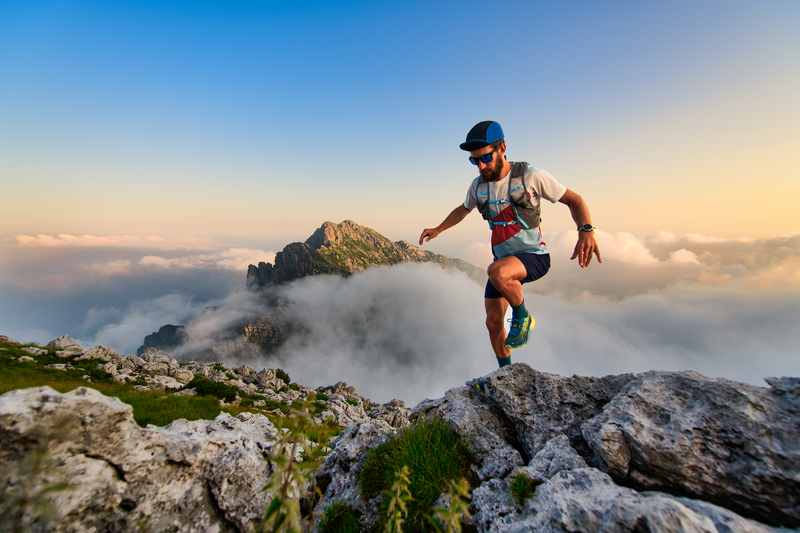
95% of researchers rate our articles as excellent or good
Learn more about the work of our research integrity team to safeguard the quality of each article we publish.
Find out more
REVIEW article
Front. Microbiol. , 14 January 2022
Sec. Microbial Symbioses
Volume 12 - 2021 | https://doi.org/10.3389/fmicb.2021.829099
This article is part of the Research Topic Plant Microbiome: Interactions, Mechanisms of Action, and Applications, Volume II View all 20 articles
Crop plants are more often exposed to abiotic stresses in the current age of fast-evolving climate change. This includes exposure to extreme and unpredictable changes in climatic conditions, phytosanitary hazards, and cultivation conditions, which results in drastic losses in worldwide agricultural productions. Plants coexist with microbial symbionts, some of which play key roles in the ecosystem and plant processes. The application of microbial biostimulants, which take advantage of symbiotic relationships, is a long-term strategy for improving plant productivity and performance, even in the face of climate change-associated stresses. Beneficial filamentous fungi, yeasts, and bacteria are examples of microbial biostimulants, which can boost the growth, yield, nutrition and stress tolerance in plants. This paper highlights recent information about the role of microbial biostimulants and their potential application in mitigating the abiotic stresses occurring on crop plants due to climate change. A critical evaluation for their efficient use under diverse climatic conditions is also made. Currently, accessible products generally improve cultural conditions, but their action mechanisms are mostly unknown, and their benefits are frequently inconsistent. Thus, further studies that could lead to the more precisely targeted products are discussed.
Global climate records in the last decades have revealed a rise in global temperature alongside changes in rainfalls, resulting in various serious implications on environmental and agricultural aspects (Füssel et al., 2012). Crop plants are more frequently exposed to abiotic stresses caused by climate change because, aside from direct implications of abiotic stresses on plants, climate change could increase the number of pests and diseases, as well as increase the severity and frequency of the outbreak of diseases (De Wolf and Isard, 2007; Garrett et al., 2016). According to recent estimates, abiotic stresses are anticipated to cause up to 50% losses, or higher, in worldwide agricultural productivity, depending on the region (Kumar and Verma, 2018). These losses, coupled with the continual rise in the human population, revealed that about 60% boosting of agricultural production is needed to meet the world food needs (Wild, 2003), with a concrete risk of dramatic deforestation increment and loss of natural ecosystems (Byerlee et al., 2014). Increased plant resilience to mitigate climate change-associated stresses is a sustainable method for ensuring food security with a restricted increase in agricultural surface, and the use of microbial biostimulants is one of the best options to achieve this goal (Calvo et al., 2014; Yakhin et al., 2017).
Plants are associated with a diverse group of microorganisms (the microbiome) in their endosphere (internal compartments), rhizosphere (attached soil to roots), and phyllosphere (aboveground parts), making microbial biostimulants particularly fascinating (Compant et al., 2019; Babalola et al., 2020). Crop plants coexist with microbial symbionts, which play key roles in plant production, performance, nutrition, and tolerance to abiotic stress (Vandenkoornhuyse et al., 2015; Enebe and Babalola, 2018; Ojuederie et al., 2019). For instance, geological evidence indicates that the relationship between microbes and plants predates the emergence from the water, suggesting that symbiosis involving arbuscular mycorrhizal was important in the process of terrestrialization (Selosse and Le Tacon, 1998). Moreover, microorganisms are involved in multiple biogeochemical cycles, such as nitrogen and carbon cycling, nitrogen fixation, soil formation and plant nutrition acquisition in the ecosystems (Wagg et al., 2014; Igiehon et al., 2019). As a result, many microbial symbionts can be used as a biofertilizer, releasing additional nutrients to the plant through synergistic mechanisms, which include nitrogen fixation (e.g., Mesorhizobium loti, Rhizobium etli, Azotobacter vinelandii, and Azospirillum brasilense), phosphate solubilization (e.g., Arbuscular mycorrhizal fungi, Azospirillum spp., Bacillus spp., and Pseudomonas spp.), cellulolytic activity (Aspergillus spp., Trichoderma spp., Bacillus spp., and Penicillium spp.), soil acidification (Bacillus spp. and Arthrobacter spp.), and production of siderophores (e.g., Pseudomonas spp.) (Bhattacharyya and Jha, 2012; Orozco-Mosqueda et al., 2021). Also, thanks to its abilities to boost plant development, defenses, antibacterial compounds, combat pathogen infections and feed on nematodes, Trichoderma spp. is a well-studied symbiotic fungal genus (Adnan et al., 2019; Szczałba et al., 2019). Despite the beneficial effects exhibited on their hosts, some of which include increased protection from abiotic stresses and nutritional efficiency, some weaknesses may limit the use of Trichoderma spp. as commercial biostimulant products, such as difficulties of in vitro cultivation and escalating of bioproduction, the lack of understanding on host specificity and population dynamics in the agroecosystem (Du Jardin, 2015). Other types of fungi can form part of the beneficial microbiome associated with plants, such as yeasts belonging to Brettanomyces naardensis, Candida oleophila, Aureobasidium pullulans, Metschnikowia fructicola, Cryptococcus albidus, and Saccharomyces cerevisiae (Freimoser et al., 2019; Nafady et al., 2019). Foliar infections can be controlled by yeasts that could colonize the leaf of a plant using direct antagonism (Preininger et al., 2018) or through the induction of systemic resistance (Lee et al., 2017). Likewise, yeast inhabiting the soil can enhance the growth of the plant through phosphate solubilization, digestion of organic materials, soil aggregation and stimulation of root development, and suppressing root infections (Sarabia et al., 2018). Plant growth-promoting bacteria (PGPB), which includes rhizobacteria or bacterial endophytes, are known to majorly populate the plant rhizosphere and the most studies genera are Azospirillum, Azotobacter, Arthrobacter, Burkholderia, Gluconacetobacter, Pseudomonas, Bacillus, Streptomyces, and Serratia (Kour et al., 2020a). Additional genera are more recently proposed as possible bioinoculants with biocontrol and/or plant growth-promoting activities, such as Rouxiella badensis and Rahnella spp. (Ulloa-Muñoz et al., 2020; Morales-Cedeńo et al., 2021). Physiological, molecular, and biochemical investigations of the interactions that exist between plant and beneficial microorganisms have shown that the presence of microbe-induced plant stress responses (Farrar et al., 2014; Igiehon et al., 2021; Igiehon and Babalola, 2021), which may trigger induced systemic tolerance (IST) against abiotic stressors (Yang et al., 2009; Vacheron et al., 2015).
Microbial biostimulants are a viable alternative for supporting plants exposed to abiotic stresses in the current context of fast-developing climate change (Santoyo et al., 2021b). While recent advancements and laboratory studies have revealed the positive activities of plant-associated microorganisms, the efficacy of microbial biostimulants is yet to be successfully validated in field experiments. As a result, microbial biostimulants are often used as supplemental therapies rather than being used to their full potential in crop management. The goal of this paper is to summarize current information about microbial biostimulants, especially the current commercially available products, examine their applications in enhancing plant tolerance to abiotic stresses caused by climate changes, and forecast the creation of novel products that may be used in adverse conditions. Also, limitations in the application of microbial biostimulants under field conditions alongside further studies required for the better development of targeted products are discussed.
Climate change is caused by a variety of factors, including variations in solar radiation, shifts in the Earth orbit, changes in the composition of the atmospheric gases, ocean oscillations, and changes in the surface characteristics of the soil (Meena et al., 2017; Cassia et al., 2018). The impacts of climate change, which often influence not only human existence but also ecological systems, elicit strong conflicts and emotions (Rising and Devineni, 2020). Agriculture is highly vulnerable to climate change because it is so reliant on soil quality, irrigation and weather conditions (Kumar et al., 2021). Floods, droughts, heat stress, rainfall unpredictability, and severe weather occurrences have a significant negative influence on global agricultural practices as a result of climate change (Myers et al., 2017; Rising and Devineni, 2020; Fiodor et al., 2021). It is clear that climate change may have a greater impact in some regions of the world, suggesting that existing farming systems and infrastructure will need to change and rapidly adapt to future changes (Müller et al., 2011). Plants, as stationary creatures, are compelled to adapt to their surrounding conditions to live and they can respond to diverse abiotic stressors with precise acclimations of physiological, developmental, and metabolic processes (Meena et al., 2017; Myers et al., 2017). Unfortunately, plant tolerance to one abiotic factor may be reduced as a result of exposure to another abiotic or biotic factor, increasing plant sensitivity (Laanisto and Niinemets, 2015). However, a multivariate acclimation in response to multiple abiotic factors is also possible (Laanisto and Niinemets, 2015).
The Intergovernmental Panel on Climate Change (IPCC) defines global warming as an “increase in both sea surface temperature and surface air averaged above 30 years” throughout the planet (Allen et al., 2018). It is also claimed that as the Earth moves from the relatively stable Holocene to the more dynamic Anthropocene, where human activities are now implicated as a major source of climate change and human-caused global warming has now surpassed pre-industrial levels by roughly 1°C (Allen et al., 2018). Subsequently, several parts of the world have experienced a far greater rise in temperature than the worldwide average, leading to a possible rise from 1.5 to 4.8°C by 2100, according to estimates (Fiodor et al., 2021; Jagadish et al., 2021). Furthermore, land-based warming is outpacing the global average and it was estimated 1.5°C in the years 2006–2015 compared to the years 1850–1900 (Fiodor et al., 2021).
Temperature is an important environmental factor that affects physiological processes and plant growth. Temperature increases promote quicker mass growth and shorter culture times (Zhao et al., 2017). However, a sudden increase or decrease in temperature can damage plant cells, resulting in reduced growth and yield overall (Zhao et al., 2017; Seguini et al., 2019). Also, for every 1°C increase in world average temperature, 3.1 to 7.4% yield losses were estimated in rice, maize, soybean and wheat for over 29 years of warming trends (Seguini et al., 2019). Another research revealed a 4 and 6% reduction in maize and wheat yields, respectively (Lobell and Gourdji, 2012). Heat stress is a limiting factor for photosynthesis in C3 crops, like rice and wheat, as well as C4 plants, like maize and sugarcane crops (Crafts-Brandner and Salvucci, 2002; Song et al., 2014; Zhao et al., 2017). Plants are also affected by temperature rise due to changes in humidity since reduced water vapor concentration in the air causes water loss from the plant, forcing stomata to be closed and lowering photosynthetic efficiency and leaf cooling by transpiration (Zhang et al., 2019; Díaz-Barradas et al., 2020). Moreover, water stress in plants is caused by prolonged high temperatures, which results in water shortages (Díaz-Barradas et al., 2020).
The hazard of global warming to crops is not limited to changes in global mean temperature, but also to sea-level rise, desertification, extreme weather conditions and changed precipitation (Russo et al., 2014). For example, due to water mixing, sea-level rise causes salinization of fresh water and land loss (Fiodor et al., 2021). Exacerbation of uncommon weather phenomena, such as floods, heatwaves, and severe precipitation, is being reported when the hydrological cycle accelerates with global warming (Hutchins et al., 2019; Perkins-Kirkpatrick and Lewis, 2020; Hawkings et al., 2021). Thus, global warming might have a slew of unanticipated consequences. For example, the melting of Greenland’s ice sheet is supposed to become a possible source of hazardous mercury contamination in the Atlantic seas (Hawkings et al., 2021).
Greenhouse gases (GHGs) are parts of the troposphere of the Earth, and they may sustain solar energy. Thanks to GHGs, the average temperature in most areas remains below 40°C, allowing plants to flourish freely (Fiodor et al., 2021). Forest fires, seas, earthquakes, permafrost, wetlands, and volcanoes are the natural environmental sources of GHG emissions that are generally absorbed by the environment (Yue and Gao, 2018). However, human activity causes an excess in GHG emission, which upsets the equilibrium (Yue and Gao, 2018). Methane, nitrous oxide and carbon dioxide are examples of the three most common greenhouse gases generated by human activity and more than 90% of human-derived global warming is thought to be caused by these gases (Wu and Mu, 2019). Along with the rise in greenhouse gases release, global warming processes have become more intense as a result of urbanization, globalization, and industrialization (Yusuf et al., 2020), while the ever-increasing human population has increased the need for energy production.
When comparing carbon dioxide levels in the atmosphere before and after the industrial revolution, it is apparent to state that human activities are the primary source of this gas (Meena et al., 2017; Hawkings et al., 2021). Carbon dioxide levels are quickly rising, from 280 ppm before the industrial revolution to 400 ppm now and it is estimated that 540 ppm will be achieved by 2100 (Myers et al., 2017). Deforestation-related increases in land use account for one-fifth of total carbon dioxide emissions (Szczałba et al., 2019). Over the decades, vast amounts of carbon dioxide are introduced into the atmosphere as fossil carbon that has accumulated in the Earth’s crust over millions of years (Anwar et al., 2020). Greenhouse gas emissions in the European Union (EU) and United States of America (USA) were 4.4 and 6.5 billion metric tons of carbon dioxide equivalent in 2018–2019, respectively (Fiodor et al., 2021; Gołasa et al., 2021). China topped the list of the biggest polluters in 2014, accounting for 30% of all carbon dioxide emissions, while Japan, Russia, India, European Union and the United States contributed 4, 5, 7, 9, and 15% of carbon dioxide emissions, respectively (Fiodor et al., 2021). Different major economic sectors are associated to GHG emission, such as (i) industry, (ii) heat and electricity production, (iii) forestry, agriculture, and other land use, (iv) building, and (v) transportation (EPA, 2021). In particular, forestry, agriculture, and other land uses were responsible for 24% of worldwide greenhouse gas emissions in the year 2010, while only agriculture showed a proportion that ranged from 1 to 14%, which is similar to the production from the industry (Fiodor et al., 2021). Since 1990, greenhouse gas emissions in agriculture have risen by 12%, due to a 9% increase in nitrous oxide emissions from soil management practices and a 60% rise in nitrous oxide and methane emissions from animal manure management systems (EPA, 2021; Fiodor et al., 2021), indicating the increasing application of emission-intensive liquid systems over the years. However, agricultural soil management strategies that increase nitrogen availability in the soil and reduce nitrous oxide emissions are available (IPCC, 2014). Nitrous oxide emissions from agricultural areas are caused by the application of organic and chemical fertilizers, the growth of nitrogen-fixing crops, irrigation methods and organic soil draining (EPA, 2021). In particular, excess nitrogen application can result in increased nitrous oxide emission without contributing to the growth and yield of the crop, indicating that more precise nitrogen fertilization for optimum crop production may help lower greenhouse gas emissions (Fiodor et al., 2021).
Changes in nutrient composition and photosynthetic rates in plants are two major effects of GHGs in agriculture (Myers et al., 2017). Reduced transpiration occurs as a result of increment in carbon dioxide concentrations, with a consequent increment in photosynthesis and water use efficiency. Rapid plant growth in high carbon dioxide environments changes the composition of essential elements for animals and humans. For example, legumes and cereals grown under carbon dioxide concentration of 550 ppm have iron and zinc levels that are 3–11% lower compared to a plant grown under normal atmospheric concentration (Gornall et al., 2010; Sangiorgio et al., 2020). Likewise, phosphorus, calcium, potassium, zinc, sulfur, iron, copper, and magnesium concentrations decrease by about 5–13% in various crops when the carbon dioxide concentration is 690 ppm (Loladze, 2014). Under elevated carbon dioxide, a decrease in nitrogen and protein content in edible parts of C3 plants have been widely reported (Jobe et al., 2020), indicating an increased risk of nutritional problems for people living in the underdeveloped parts of the world (Loladze, 2014).
High temperatures affect the physiology of the plant by raising the respiration rates and leaf transpiration and altering photosynthate allocation (Munns, 2002; Malhotra, 2017). Rubisco affinity for carbon dioxide decreases at high temperatures and its affinity for oxygen increases (Jordan and Ogren, 1984; Sangiorgio et al., 2020). Carbon dioxide solubility is reduced more than oxygen when the temperature rises, lowering carbon dioxide concentration in the chloroplast in comparison to oxygen (Ku and Edwards, 1977). Furthermore, plants shut their stomata to decrease evapotranspiration water losses when the temperature rises. When stomata closure occurs, carbon dioxide concentration declines rapidly, but oxygen concentration rises, limiting photosynthesis, increasing the photorespiration (Bhattacharya, 2019).
Higher temperatures in spring would allow many cycles per year for annual crops, such as tomato (Sangiorgio et al., 2020) and lettuce (Pearson et al., 1997), enhancing early flowering in horticultural crops (Wheeler et al., 1993; Bisbis et al., 2018). On the other hand, increasing temperatures may provide difficulty for floral differentiation in several horticultural crops. For example, high temperatures enhance male floral development but reduce feminine flower differentiation in cucumbers (Sangiorgio et al., 2020). In fruit crops, such as apple (Funes et al., 2016), peach, and plum (Hazarika, 2013), difficulty in meeting cold requirements for floral differentiation might reduce crop production (Luedeling, 2012). In the long run, climate change may move fruit cultivation areas to the North (Luedeling, 2012), but moderate winter temperatures are capable of stimulating early blooming, thereby exposing plants to late frost risks (Vitasse and Rebetez, 2018). Finally, increasing temperatures may affect agricultural practice in tropical environments which often result in the extinction of vulnerable crops (Gornall et al., 2010).
Heat stress causes complex molecular, biochemical, and physiological reactions in plants (Sangiorgio et al., 2020), which may result in the synthesis of heat shock proteins, enzymes that degrade reactive oxygen species (ROS), osmoprotectant chemicals, amino acids, sugars, and sulfur compounds (Shulaev et al., 2008). Thus, oxidative stress and ROS production are stimulated by heat stress, which is sensed by histidine kinases and Heat stress factors (Hsfs), then redox-sensitive transcription factors are downstream activated by the MAPK signal pathway, which subsequently activates other transcription factors (e.g., BF1c and Rboh) to turn on the expression of genes involved in the synthesis of antioxidant enzymes (Qu et al., 2013; Figure 1).
Figure 1. Plant cell response to exogenous and endogenous ROS production stimulated by heat stress (modified from Qu et al., 2013).
Hormonal signaling is in charge of heat stress responses. Ethylene is one among them (Byerlee et al., 2014), and it is involved not only in senescence, development, plant physiology, and development but also in plant responses to abiotic stressors, such as heat, salinity and drought (Dubois et al., 2018). Plant responses to heat stress can be boosted by microbial biostimulants. For example, the synthesis of ROS-degrading enzymes (e.g., superoxide, catalase, peroxidases, and dismutase) enhance heat stress tolerance and it can be boosted in plants colonized by beneficial bacteria, such as Pseudomonas and Bacillus, and mycorrhizal fungi, such as Septoglomus deserticola and Septoglomus constrictum (Duc et al., 2018). SoilPro® (Liventia, TX, United States) is a microbial biostimulant that helps in improving soil quality and heat stress tolerance and it contains P. fluorescens and P. aeruginosa, which are commercialized for bioremediation, phytostimulation, and improved capabilities of soil fertility (Sangiorgio et al., 2020). Likewise, Bacillus spp. have been developed not only as biopesticides (e.g., Bacillus amyloliquefaciens, Bacillus firmus, Bacillus pumilus, Bacillus thuringensis, Bacillus licheniformis, Bacillus sphaericus, and Bacillus subtilis), and some biostimulant products made up of Bacillus spp. are available, such as Endox® (Scam, Spa, Italy) and Activate® (Natural resources Group, Inc., United States). While these microorganisms are used in many commercial treatments, whether alone or as a mixture, mitigation from heat stress is not usually stated as one of their benefits.
The use of microorganisms that limit ethylene emissions has a lot of promise because lowering ethylene levels in stressful situations might help plants escape the harmful effects of heat stress (Glick, 2014). In particular, bacteria that exhibit 1-aminocyclopropane-1-carboxylic acid (ACC) deaminase activity, appear to be highly promising, such as Bacillus subtilis BERA 7, Leclercia adecarboxylata MO1, Pseudomonas fluorescens YsS6, and Pseudomonas migulae 8R6 (del Carmen Orozco-Mosqueda et al., 2020). For example, the ACC deaminase-producing Paraburkholderia phytofirmans PsJN allows normal tomato development under heat stress (Esmaeel et al., 2018). Despite its apparent beneficial properties, P. phytofirmans PsJN is yet to be used as a commercial product (Sangiorgio et al., 2020). The ability of microbial biostimulants able to mitigate heat stress in crops was associated to the reduction of ROS content, the increase in heat shock protein and osmoprotectant (e.g., sugar and proline) content and the modulation of hormonal levels (Figure 2), such as ethylene and abscisic acid (Table 1). In particular, Pseudomonas sp. AKMP6 and P. putida AKMP7, Glomus sp., B. aryabhatthai SRBO2, B. amyloliquefaciens, A. brasilense, and P. phytofirmans (Ali et al., 2009; Ali and Xie, 2020). However, further efforts on the understanding of the mechanism of action of microbial biostimulants against heat stress and on the development of efficient formulations are required to mitigate the impact of heat stress on agricultural and food production by sustainable approaches.
Figure 2. Protective mechanisms used by microbial biostimulants in mitigation of various stresses in plants (modified from Sangiorgio et al., 2020).
Frost events have been reported in several counties in recent years, such as in Italy, Switzerland, Germany, Belgium, USA, and France, resulting in serious crop damage and economic losses (Unterberger et al., 2018; Vitasse and Rebetez, 2018). Low temperatures decrease plant metabolism, leading to reduced photosynthetic activity, reduced foliar growth, and early senescence, when temperatures are above the freezing point (Huner et al., 1993). Bud development can be hampered by temperatures below freezing since rehydrated and growing buds might be destroyed by the cold stress (Mishra et al., 2012). Cold can dehydrate plant tissues, causing a rise in the concentration of osmolytes in the cytoplasm of the cell and leading to the breakdown of the plasma membrane (Mishra et al., 2012).
Microorganisms possessing ice nucleating activity (INA+), which can survive on fruits, leaves, and roots, can promote the development of ice cores in plants (Lindow, 1983). The extracellular polymeric substances (exopolysaccharides) produced by these microorganisms are made up of proteins that function as ice nucleation centers on their cell wall, promoting the production of ice crystals (Lee et al., 1995). These microbes are mostly bacteria, although ice-nucleating fungi have also been discovered (Pouleur et al., 1992), which may colonize the plant both epiphytically and endophytically. For example, P. syringae was the first bacterial strain reported to possess ice nucleating activity (Arny et al., 1976; Fadiji and Babalola, 2020a,b; Galambos et al., 2021). The presence of P. syringae strains with ice-nucleating activity enhanced the sensitivity of soybean and tomato to cold damage in experimental conditions (Anderson et al., 1982). Likewise, other species exhibited ice nucleating activity, such as Erwinia herbicola (syn: P. agglomerans) (Lindow et al., 1978), Xanthomonas campestris (Kim et al., 1987), and the Gram-positive bacteria Lysinibacillus sp. (Failor et al., 2017). On the other hand, frost damage was minimized using P. syringae A506 with an inactivated ice-nucleating gene (Skirvin et al., 2000) and the use of microbial biostimulants capable of competing INA+ bacteria has become a critical strategy for reducing frost damages (Figure 2; Selvakumar et al., 2012). For example, Blightban A506® (Nufarm Americas, Inc., TX, United States), which was produced using lyophilized P. fluorescens A506 (Lindow and Brandl, 2003), is a commercial product for the prevention of frost damage in USA (Table 1) and this formulation is also used to combat fire blight (E. amylovora) in pear and apple trees (Lindow and Brandl, 2003).
Microbial biostimulants can also help to reduce the effect of chilling temperatures. Plant growth suppression caused by low temperature can be countered by microbial symbionts that produce growth-related hormones (e.g., auxins or gibberellins) or that reduce ethylene concentration (Figure 2 and Table 1; Sangiorgio et al., 2020). Several bacteria produce indole-3-acetic acid (IAA), which represents an example of auxin (Amara et al., 2015). For instance, IAA is produced by P. dispersa 1A and S. marcescens SRM strains isolated from the Himalayan northwest (Amara et al., 2015). Thus, wheat seedlings inoculated with these strains and cultivated under cold condition exhibited considerably better nutrient and yield absorption ability competed to control seedlings (Selvakumar et al., 2008a). Likewise, cold tolerance is enhanced in wheat seedlings treated with Pseudomonas sp. NARs9 and PGERs (Mishra et al., 2008, 2012). Cold stress causes a rise in ethylene production, which reduces plant productivity (Glick, 2014) and P. phytofirmans PsJN, which exhibited ACC deaminase activity, improved cold tolerance in grapevine by decreasing the damage to the cell membrane (Theocharis et al., 2012). Bean plants cultivated in extreme cold conditions and inoculated with psychrophilic ACC deaminase-producing bacteria, such as P. fragi, P. fluorescens, P. proteolytica, B. frigoritolerans, and P. chlororaphis, revealed less frost damage through reduced ROS production and lipid peroxidation (Tiryaki et al., 2019). Out of all the bacterial species identified, P. fluorescens and P. chlororaphis are the examples of the few microbial biostimulants found in the market as biopesticides (Cedomon®, BioAgri AB, Uppsala, Sweden) while the other one is formulated in combination with similar PGPR products such as HyperGalaxy® (Holmes Enviro, Llc., Philomath, OR, United States), BFMS® (Tainio, Cheney, WA, United States), SoilBiotic® (SoilBiotics, Reddick, IL, United States) and BioStrain® (Monty’s Plant Food Company, Louisville, KY, United States). More studies toward the formulation of microbial biostimulant to combat cold stress in crop plant is advocated.
Drought or excessive salinity can induce water stress, which happens when leaf transpiration losses or surpass root absorption, resulting in a decrease in water content and turgor in plant tissues (Sangiorgio et al., 2020). Drought stress has been increasingly severe and frequent, especially in semi-arid regions (Trenberth et al., 2014). Furthermore, when rainfall is light and irregular, salt buildup in the soil can worsen the effects of drought (Othman et al., 2006), since a rise in the solutes level in the saline soil lowers the osmotic potential of the liquid phase of the soil, limiting the absorption of water by plant roots (Sequi, 2006). For example, soil salinization affects around 30% of total arable land in the Mediterranean regions (Rasool et al., 2013). Drought stress and the resulting soil salinization are also major contributors to desertification in overexploited regions, they affect soil biodiversity and composition, contributing to plant deterioration, sparse soil covering and consequent soil erosion (Vicente-Serrano et al., 2020). Drylands now occupy 46% of the world’s land surface, impacting about 250 million persons in developing countries (Huang et al., 2020).
Drought stress influences plant physiology and morphology, causing harmful ROS buildup (Smirnoff, 1993), emission of ethylene (Ali et al., 2014), and decreased mineral nutrient availability, absorption, and transport (Rouphael et al., 2012). Plant tolerance to drought and salinity can be improved by microbial biostimulants through a variety of direct and plant-mediated processes (Figure 2 and Table 2). For example, microbial biostimulants can produce bacterial exopolysaccharides that enhance soil structure by forming micro and macroaggregates (Grover et al., 2011), improving plant development under water stress conditions (Sangiorgio et al., 2020). Furthermore, exopolysaccharides form hydrophilic biofilms and create a microenvironment that promotes the retention of water by shielding microbes from drought stress (Nwodo et al., 2012) and binds Na+ ions to reduce uptake by the plant (Tewari and Arora, 2014). For example, Pseudomonas spp. PF23 (Tewari and Arora, 2014) and Pseudomonas putida GAP-P45 (Tallon et al., 2003) exhibit protective potentials by the production of exopolysaccharides. Exploring root capacity can be strengthened by mycorrhizal fungi (Khan et al., 2015), allowing for increased root biomass, improved structure of the soil, increased water retention, and reduced leaching of the mineral nutrients (Cavagnaro et al., 2015; Santoyo et al., 2021a). In particular, Glomus sp. generates a glycoprotein called glomalin, which has an aggregating impact on soil structure and improves plant development and drought tolerance (Gong et al., 2013). Likewise, ascomycetes (P. glomerata LWL2, Exophiala sp. LHL08, P. formosus LHL10, and Penicillium sp. LWL3) colonize cucumber plants, enhancing leaf development and chlorophyll content under drought stress (Khan et al., 2015; Fadiji et al., 2020). Apart from promoting root development, mycorrhizal fungi can also improve water absorption through aquaporins (Aroca et al., 2007), which is one of the large families of integral membrane transporters that enable the flow of water through the phospholipid bilayer layer of the cell membrane (Bray, 2000). For example, Glomus intraradices mycorrhizes common bean plant and mitigate water stress affecting aquaporin activity and improving water conductivity in the roots (Aroca et al., 2007; Omomowo et al., 2018). Glomus intraradices, when cultivated in association with carrot, increased the expression of two fungal aquaporins (GintAQPF2 and GintAQPF1), which consequently enhanced water transport between the 2 symbionts and therefore gave the plant higher resilience to water shortage (Li T. et al., 2013). G. intraradices is commonly found in commercial products, often formulated in combination with many other beneficial bacterial and fungal strains, such as OroSoil® (Fomet, Spa, Verona, Italy) and MycoApply® All Purpose (Mycorrhizal Applications, Inc., OR, United States), but it also exists in single formulations, such as Agtiv® (PremierTech, Rivière-du-Loup, Canada) and Groundwork® (GroundWork BioAg, Ltd., Hashahar, Israel).
Microbial biostimulants have a variety of additional effects against drought stress on associated plants, such as the antioxidant defenses, the production of protective osmolytes (e.g., glycine betaine) or phytohormones, and the secretion of volatile organic compounds (VOCs) (Figure 2 and Table 2; Kaushal and Wani, 2016; Sharifi and Ryu, 2018). Since stress condition boosts ethylene synthesis, which limits plant development, microbial biostimulants (e.g., P. fluorescens) might subtract ACC, reduce ethylene synthesis and relieve inhibition mediated by ethylene (Glick, 2014). Other microbial species that produce ACC in reducing drought stress include Staphylococcus sp. Acb12, Staphylococcus sp. Acb13, Staphylococcus sp. Acb14, Bacillus, Achromobacter, Klebsiella, and Citrobacter spp. (Marasco et al., 2012; Jayakumar et al., 2020). Beneficial microorganisms that produce ACC deaminase might help to mitigate these side effects under drought and salt stresses, such as A. piechaudii ARV8 in pepper and tomato plants (Mayak et al., 2004) and P. fluorescens TDK1 in the peanut seedlings (Saravanakumar and Samiyappan, 2007). Plant growth promotion and soil improvement are attributed to Achromobacter spp. and Pseudomonas spp. and these beneficial microorganisms are developed in commercial products, such as SSB® and SOS® (Liventia, Inc., San Antonio, TX, United States) (Table 3).
Table 3. Summary of some currently available commercial products used in the mitigating abiotic stresses.
Meanwhile, microbial biostimulants can synthesize IAA, which stimulates plant growth and root branching of the plant under drought stress (Ouyang et al., 2017), for example, species belonging to Alcaligenes, Sinorhizobium, Serratia, Bacillus, and Arthrobacter (Ouyang et al., 2017; Table 2). In particular, when inoculated on salt-stressed cucumber and tomato plants, the bacterium P. chlororaphis TSAU13, an IAA producing strain, can enhance plant tolerance to drought and salinity (Egamberdieva, 2012). Likewise, the mycorrhizal fungus Funneliformis mosseae improves IAA level in the root, root hair development, and growth of orange plants under drought stress (Liu et al., 2018). The Wettable Mycorrhizae Blend® (BioLogic Crop Solutions, Inc., CA, United States) is a commercial product based on Funneliformis mosseae that improves the plant capacity to absorb nutrients and water (Sangiorgio et al., 2020). Similarly, microorganisms that produce cytokinins and gibberellins are effective in reducing water stress damage by promoting stomatal opening and shoot development in low-water circumstances (Liu et al., 2013). PGPR strains of Acinetobacter, Pseudomonas, and Burkholderia spp. have been characterized as being able to produce gibberellins and these bacteria can boost cucumber growth under salt and drought conditions (Kang et al., 2015). Despite their potentially helpful properties, no commercial products based on these species are available, suggesting that novel products will be probably launched in the future.
After exposure to drought stress, the synthesis of ABA increases to promote stomatal closure. When inoculated with P. putida H-2-3, ABA content decreases in soybean, reducing the impact of drought stress on plant productivity (Kang et al., 2014). P. putida is sold as the commercial product N-Texx® (CXI, Coppell, TX, United States) in conjunction with B. subtilis for their roles on soil fertility, but not particularly for drought stress alleviation. Likewise, G. intraradices inoculation lowers ABA concentration and reduces sensitivity to salt stress in lettuce (Jahromi et al., 2008). Plants produce more ethylene because of ABA and water shortage and plant development can be reduced by high ethylene levels.
Under water stress, the production of ROS and oxidative damage to lipids, nucleic acids, and proteins are common. Many microbes can mitigate the negative effects of increased ROS by producing antioxidant molecules or increasing the activity of antioxidant plant enzymes, such as peroxidases and catalase (Vurukonda et al., 2016). When inoculated with Pseudomonas sp., basil plants exhibited an increment in the activity of catalase under water stress (Vurukonda et al., 2016). Likewise, Pseudomonas sp., Bacillus lentus, and Azospirillum brasilense are an example of microbial stimulants that have been used individually or in the formulation of microbial consortia which can reduce drought stress in crop plants (Sangiorgio et al., 2020). Ascorbate, peroxidase and glutathione peroxidase activities of Pseudomonas sp., Bacillus lentus, and Azospirillum brasilense was used to enhance drought stress (Heidari and Golpayegani, 2012).
Osmocompatible solute accumulation is a type of stress response that allows the accumulation of inorganic and organic solutes in the vacuole and cytosol, respectively, to lower the osmotic potential of the cell and to maintain its turgor under water stress (Sangiorgio et al., 2020). Numerous bacteria produce osmolytes (Paul et al., 2008), which often work together with osmolytes produced by the plant (e.g., proline) to lower the osmotic potential and stabilize the cell wall components (Sanders and Arndt, 2012). The phosphate-solubilizing B. polymyxa strain produces proline when inoculated in tomato plants, reducing the effects of water stress (Shintu and Jayaram, 2015). Betaine produced by osmotolerant bacteria from the rhizosphere (e.g., Streptomyces tendae F4) often works in tandem with that produced by the host plant in rice, leading to an increased tolerance to water stress (Dimkpa et al., 2009). Even though the results were encouraging, B. polymixa is not yet available as a commercial product.
Some bacteria can interact with plants by VOCs, which can trigger stress adaption reactions mediated by mineral uptake, water conservation, and root growth (Bailly et al., 2014). While the importance of hormone signaling cascades has been demonstrated (Bhattacharyya et al., 2015; Tahir et al., 2017), the underlying VOC-mediated mechanisms involved in plant-microbe associations under extreme conditions remain mainly unknown. Beneficial effects of microbial VOCs are known on plants, such as 2,3-butanediol on plant fitness (Sangiorgio et al., 2020), synthesis of osmoprotectants and control of stomata closure (Zhang et al., 2010). Likewise, 1-heptanol, 3-methyl-butanol, and 2-undecanone produced by Parabulkholderia phytofirmans enhance salinity tolerance (Ledger et al., 2016), whereas butyrolactone and 1-butanol increase the root growth and the exchange of carbon in plant’s rhizosphere (Gutiérrez-Luna et al., 2010). The future of VOC-based plant promotion will almost certainly hinge on the identification of stress-induced signaling pathways. The production of VOCs by Bacillus thuringiensis AZP2 helped in the mitigation of drought stress in wheat plant (Khan et al., 2018).
Although there are a large number of microorganisms that are capable of protecting plants from water stress, a limited number of commercial products exist for this vital purpose. The majority of these market products Ryze® (L.Gobbi, Srl, Genova, Italy), Micosat F® (CCS, Srl, Aosta, Italy; Suma), Grolux® (RRR Supply Inc., Munger, MI, United States), HyperGalaxy® (Holmes Enviro, Llc., Philomath, OR, United States, BFMS® (Tainio, Cheney, WA, United States), Environoc® (Biodyne, Llc., Wayne, IN, United States), and SoilBiotics® (SoilBiotics, Reddick, IL, United States) are just a few of the successfully commercialized products based on consortia of Azospirillum brasilense, Bacillus altitudinis, Bacillus amyloliquefaciens, Bacillus licheniformis, Cellulomonas cellasea, P. fluorescens, Pseudomonas putida, Pseudomonas stutzeri, Streptomyces albidoflavus, Glomus spp., and Trichoderma spp., that exhibit water stress capacity, as well as enhancement of plant yield through the production of exopolysaccharides alongside the enrichment of nutrients and soil organic matter (Table 3). Efforts toward the formulation/production of more universally accepted commercial products, which will be active in most ecosystems will go a long way in the mitigation of water stress in crop plants.
Interannual rainfall and seasonal variability patterns of rain are two of the most significant problems of the current climate change (Foster and Rahmstorf, 2011; Stevenson et al., 2012). Flooding affects 13% of the Earth’s surface, and severe rainfall intensity and frequency are expected to rise worldwide in the future (Sangiorgio et al., 2020). Water stagnation, anoxia and hypoxia of the root are caused by heavy floods and rains. The enzyme ACC synthase, noted in the production of ethylene, is produced in significant amounts by roots when they are flooded (Sangiorgio et al., 2020). The enzyme ACC oxidase, requires oxygen to catalyze the final stage of biosynthesis of ethylene and ACC is transported to the aerial portion of the plant via the xylem (Else and Jackson, 1998), where it is transformed to ethylene, resulting in wilting, chlorosis, leaf necrosis, fruit and flower loss, and limited yield (Glick, 2014). Thanks to ACC deaminase activity, which lowers the level of endogenous ethylene, microbial biostimulants can help to alleviate stress related to water stagnation (Glick, 2014; Ali and Kim, 2018). For example, tomato seedlings inoculated with ACC deaminase-producing strains of Pseudomonas spp. and Enterobacter spp. show a reduction of anoxia stress and an improvement of germination (Grichko and Glick, 2001; Sangiorgio et al., 2020). The commercial product SumaGrow® (RRR Supply Inc., Munger, MI, United States), which contains Pseudomonas and Enterobacter spp., increases yield and improves stress tolerance, but it does not promise to give particular protection from waterlogging stress. Chlorophyll content, plant elongation, biomass, formation of the adventitious root, and leaf area were promoted, while ethylene levels were reduced, using Pseudomonas sp. on cucumber seeds (Li J. et al., 2013) and Streptomyces sp. GMKU 336 strain on Vigna radiata (Jaemsaeng et al., 2018). The active ingredients of Mycostop® (Verdera Oy, Espoo, Finland) and Actinovate® (Mycorrhizal Applications, Inc., OR, United States) are S. lydicus WYEC 108, and Streptomyces K61, respectively. Streptomyces spp. is beneficial bacteria frequently utilized against biotic stresses and their application against abiotic stresses is currently understudied. Future studies into the production of more microbial stimulant and the full exploration of the roles of all currently available commercial products for the mitigation of water stress is needed.
The development of a novel microbial biostimulant presents some unique challenges. To begin with, the business registration procedure is often complicated, and there is still a lack of unified international regulation (Backer et al., 2018). Secondly, it should be noted that product effectiveness highly relies on the type of agricultural crop used, its phenological stage and environmental conditions (Khan et al., 2018). The relationship between microorganisms and host plants must be evaluated while developing microbial biostimulants. To establish the efficacy of microbial biostimulant, favorable and long-lasting colonization of the plant is required, and it could be affected by the indigenous plant-associated microbial communities (Sangiorgio et al., 2020).
Finally, to reduce the effects of cultural and environmental factors on product conservation and efficacy, the optimum formulation should be developed (Bashan et al., 2014). Due to spore formation by bacteria, biostimulants that are formulated using Gram-positive bacteria allow powder formulations to have drying tolerance and long-term stability. Even though there are several types of the effectiveness of microbe utilization in stimulating plant development under adverse conditions, there are relatively few biostimulant products available that particularly address the challenges exacerbated by climate change. Moreover, the high costs associated with microbial biostimulant registration and manufacturing (Nadeem et al., 2014) are key roadblocks to product development, resulting in a small number of products that are commercialized and limited adoption in horticulture. Thus, several factors must be taken into consideration for microbial biostimulant development and use, such as crop and soil characteristics, type of application and microbial strain characteristics. About the first aspect, no microbes can be said to be universally fit for all ecosystems (Adesemoye et al., 2009) or on any plant host (Finkel et al., 2017), indicating that selection of a strain for the production of microbial biostimulant requires consideration of the plant and the soil properties to maximize efficacy under specific conditions (Ipek et al., 2014). Biostimulant efficiency can be reduced when there is competition for space and nutrients between the selected microbial strains and the indigenous microbiota (Ruzzi and Aroca, 2015). Thus, isolation and application of endemic microbial strains, possibly with multiple plant growth promotion traits would be an important issue to observe better results in field trials (Etesami and Maheshwari, 2018). For example, it was well demonstrated that microorganisms extracted from the host plant microbiome are more effective than non-indigenous microbial inoculants (Mazzola and Freilich, 2017). Moreover, it is also important to optimize the mode of microbial biostimulant application (e.g., seed or root inoculation, spore spray, etc.), which should minimize microbe dispersion or mortality caused by abiotic variables, such as temperature and UV light effect (Vejan et al., 2016).
Crop breeding strategies in the future should include the plant ability to form permanent symbiotic partnerships with beneficial microbes as a very important feature, strongly connected to stress tolerance, resilience and production (Msimbira and Smith, 2020). Simultaneously, a better understanding of microbial activities and interaction processes may allow for the selection of appropriate microbial biostimulants for a certain cultivar or crop under a specific cultural situation (Clúa et al., 2018). Likewise, other approaches are required to fully realize the potential of microbial biostimulants, such as a depth characterization of the plant microbial biocoenosis using real-time investigation microbial functional dynamics, next-generation sequencing such as RNA/metagenome/amplicon sequencing optimization and development of the synthetic community of microbes (Fadiji and Babalola, 2020c). As a result, a critical step in the effective selection of microbial biostimulants is the characterization of the indigenous microbiome using high-throughput next-generation sequencing approaches in combination with meta-analysis of the associated population, to identify microorganisms that can persist on plants in harsh conditions (Purahong et al., 2018; Fadiji and Babalola, 2020c). Furthermore, understanding and exploiting plant-microbe interactions requires detailed research of the microbiome using functional markers rather than taxonomic markers (Lemanceau et al., 2017). The use of real-time monitoring tools for important microbial activities might be used to optimize the effectiveness of microbial inoculants. For example, the use of real-time PCR techniques enables for broad-range assessment of the functional genes available in the microbes and might be applied for future monitoring of the agroecological function (Bouffaud et al., 2018).
Finally, the creation of synthetic communities of microbes, i.e., the combination of numerous microbes with specific plant growth-promoting functions, offers an opportunity to improve the effectiveness and reliability of microbial biostimulants (Ahkami et al., 2017). However, to ensure the sustainability of the beneficial community, the intricacy of ecological interactions between microorganisms should be thoroughly explored, such as competition and commensalism.
Microbial biostimulants have the potential to be a long-term and successful method for reducing the abiotic stressors that climate change has exacerbated. Furthermore, the application of microbial biostimulants may help to maintain the ecological balance of agro-ecosystems, reducing the usage of pesticides and/or heavy metals for agricultural practices. Nonetheless, several concerns should be considered both at the regulatory level and throughout the development and research, to achieve greater efficacy of the product and wider adoption.
Plant biostimulant has a claim-based definition, which means that the function is used to establish the product. Many potent ingredients with various activities and objectives can be found in a single product. As a result, the inherent heterogeneity of microbial biostimulants could elude regulatory categorization (e.g., fungicide, fertilizer, and amendment). Products may be subjected to lengthy and costly trial procedures depending on the country of registration. The lack of a consistent worldwide regulatory framework creates a barrier to product marketing and may deter the development of novel products. In agroecological and biological research, the adoption of microbial biostimulants still has certain drawbacks, mostly due to their lesser efficacy and greater environmental sensitivity when compared to synthetic products (e.g., pesticides, fertilizers, and growth regulators). Furthermore, the efficacy of microbial biostimulants varied widely according to the crop and environmental conditions. Future research should focus on developing better-targeted products, such as delving deeper into interactions of the microbial biostimulant with indigenous plant-associated microbiomes.
The application of microbial biostimulants might provide a long-term and cost-effective solution to plant productivity losses caused by changing climatic factors, as well as aid in the optimization of human inputs in agro-ecosystem. Results from preliminary experiments involving microbial biostimulants should be disseminated by all relevant policymakers and stakeholders, such as extension services and growers, to ensure that this methodology can be largely applied to a variety of crops, regions, and under different environmental conditions.
AF and OB conceived the ideas, collected the data, and developed the manuscript. GS and MP provided professional input and critiqued the work. All authors have carefully read the final manuscript and have agreed that the manuscript be published.
This study was funded by the National Research Foundation, South Africa (UID123634 and UID132595).
The authors declare that the research was conducted in the absence of any commercial or financial relationships that could be construed as a potential conflict of interest.
All claims expressed in this article are solely those of the authors and do not necessarily represent those of their affiliated organizations, or those of the publisher, the editors and the reviewers. Any product that may be evaluated in this article, or claim that may be made by its manufacturer, is not guaranteed or endorsed by the publisher.
AF gratefully acknowledged the National Research Foundation, South Africa/The World Academy of Science African Renaissance Ph.D. Scholarship (Ref: UID116107), which supported his studies. OB appreciates the National Research Foundation, South Africa, for the grant (UID123634 and UID132595) that have supported this research.
Abd El-Daim, I. A., Bejai, S., and Meijer, J. (2014). Improved heat stress tolerance of wheat seedlings by bacterial seed treatment. Plant Soil 379, 337–350. doi: 10.1007/s11104-014-2063-3
Adesemoye, A., Torbert, H., and Kloepper, J. (2009). Plant growth-promoting rhizobacteria allow reduced application rates of chemical fertilizers. Microb. Ecol. 58, 921–929. doi: 10.1007/s00248-009-9531-y
Adnan, M., Islam, W., Shabbir, A., Khan, K. A., Ghramh, H. A., Huang, Z., et al. (2019). Plant defense against fungal pathogens by antagonistic fungi with Trichoderma in focus. Microb. Pathog. 129, 7–18. doi: 10.1016/j.micpath.2019.01.042
Ahkami, A. H., White Iii, R. A., Handakumbura, P. P., and Jansson, C. (2017). Rhizosphere engineering: enhancing sustainable plant ecosystem productivity. Rhizosphere 3, 233–243.
Ait Barka, E., Nowak, J., and Clément, C. (2006). Enhancement of chilling resistance of inoculated grapevine plantlets with a plant growth-promoting rhizobacterium, Burkholderia phytofirmans strain PsJN. Appl. Environ. Microbiol. 72, 7246–7252. doi: 10.1128/AEM.01047-06
Akhgar, A., Arzanlou, M., Bakker, P., and Hamidpour, M. (2014). Characterization of 1-aminocyclopropane-1-carboxylate (ACC) deaminase-containing Pseudomonas spp. in the rhizosphere of salt-stressed canola. Pedosphere 24, 461–468.
Ali, S. Z., Sandhya, V., Grover, M., Kishore, N., Rao, L. V., and Venkateswarlu, B. (2009). Pseudomonas sp. strain AKM-P6 enhances tolerance of sorghum seedlings to elevated temperatures. Biol. Fertil. Soils 46, 45–55. doi: 10.1007/s00374-009-0404-9
Ali, S. Z., Sandhya, V., Grover, M., Linga, V. R., and Bandi, V. (2011). Effect of inoculation with a thermotolerant plant growth promoting Pseudomonas putida strain AKMP7 on growth of wheat (Triticum spp.) under heat stress. J. Plant Interact. 6, 239–246.
Ali, S., and Kim, W.-C. (2018). Plant growth promotion under water: decrease of waterlogging-induced ACC and ethylene levels by ACC deaminase-producing bacteria. Front. Microbiol. 9:1096. doi: 10.3389/fmicb.2018.01096
Ali, S., and Xie, L. (2020). Plant growth promoting and stress mitigating abilities of soil born microorganisms. Recent Pat. Food Nutr. Agric. 11, 96–104. doi: 10.2174/2212798410666190515115548
Ali, S., Charles, T. C., and Glick, B. R. (2014). Amelioration of high salinity stress damage by plant growth-promoting bacterial endophytes that contain ACC deaminase. Plant Physiol. Biochem. 80, 160–167. doi: 10.1016/j.plaphy.2014.04.003
Allen, M. R., Dube, O. P., Solecki, W., Aragón-Durand, F., Cramer, W., Humphreys, S., et al. (2018). “Framing and context,” in Global Warming of 1.5 C: An IPCC Special Report on the Impacts of Global Warming of 1.5 C Above pre-Industrial Levels and Related Global Greenhouse Gas Emission Pathways, in the Context of Strengthening the Global Response to the Threat Of Climate Change, Sustainable Development, and Efforts to eradicate poverty. Sustainable development, and Efforts to Eradicate Poverty’, eds V. Masson-Delmotte, P. Zhai, H. O. Pörtner, D. Roberts, J. Skea, P. R. Shukla, et al. (Geneva: IPCC), 41–91.
Amara, U., Khalid, R., and Hayat, R. (2015). “Soil bacteria and phytohormones for sustainable crop production,” in Bacterial Metabolites in Sustainable Agroecosystem, ed. D. K. Maheshwari (Cham: Springer), 87–103.
Anderson, J., Buchanan, D., Stall, R., and Hall, C. (1982). Frost injury of tender plants increased by Pseudomonas syringae van Hall. J. Am. Soc. Hortic. Sci. 107, 123–125.
Anwar, A., Younis, M., and Ullah, I. (2020). Impact of urbanization and economic growth on CO2 emission: a case of far east Asian countries. Int. J. Environ. Res. Public Health 17:2531. doi: 10.3390/ijerph17072531
Arkhipova, T., Prinsen, E., Veselov, S., Martinenko, E., Melentiev, A., and Kudoyarova, G. (2007). Cytokinin producing bacteria enhance plant growth in drying soil. Plant Soil Biol. 292, 305–315. doi: 10.1007/s11104-007-9233-5
Arny, D., Lindow, S., and Upper, C. (1976). Frost sensitivity of Zea mays increased by application of Pseudomonas syringae. Nature 262, 282–284.
Aroca, R., Porcel, R., and Ruiz-Lozano, J. M. (2007). How does arbuscular mycorrhizal symbiosis regulate root hydraulic properties and plasma membrane aquaporins in Phaseolus vulgaris under drought, cold or salinity stresses? New Phytol. 173, 808–816. doi: 10.1111/j.1469-8137.2006.01961.x
Aroca, R., Vernieri, P., and Ruiz-Lozano, J. M. (2008). Mycorrhizal and non-mycorrhizal Lactuca sativa plants exhibit contrasting responses to exogenous ABA during drought stress and recovery. J. Exp. Bot. 59, 2029–2041. doi: 10.1093/jxb/ern057
Babalola, O. O., Fadiji, A. E., Enagbonma, B. J., Alori, E. T., Ayilara, M. S., and Ayangbenro, A. S. (2020). The nexus between plant and plant microbiome: revelation of the networking strategies. Front. Microbiol. 11:2128. doi: 10.3389/fmicb.2020.548037
Backer, R., Rokem, J. S., Ilangumaran, G., Lamont, J., Praslickova, D., Ricci, E., et al. (2018). Plant growth-promoting rhizobacteria: context, mechanisms of action, and roadmap to commercialization of biostimulants for sustainable agriculture. Front. Plant Sci. 9:1473. doi: 10.3389/fpls.2018.01473
Bailly, A., Groenhagen, U., Schulz, S., Geisler, M., Eberl, L., and Weisskopf, L. (2014). The inter-kingdom volatile signal indole promotes root development by interfering with auxin signalling. Plant J. 80, 758–771. doi: 10.1111/tpj.12666
Bashan, Y., De-Bashan, L. E., Prabhu, S., and Hernandez, J.-P. (2014). Advances in plant growth-promoting bacterial inoculant technology: formulations and practical perspectives (1998–2013). Plant Soil 378, 1–33. doi: 10.1007/s11104-013-1956-x
Bhattacharya, A. (2019). “Effect of high temperature on carbohydratemetabolism in plants,” in Effect of High Temperature on Crop Productivity and Metabolism of Macro Molecules, ed. A. Bhattacharya (Cambridge, MA: Academic Press), 115–216.
Bhattacharyya, D., Garladinne, M., and Lee, Y. H. (2015). Volatile indole produced by rhizobacterium Proteus vulgaris JBLS202 stimulates growth of Arabidopsis thaliana through auxin, cytokinin, and brassinosteroid pathways. J. Plant Growth Regul. 34, 158–168. doi: 10.1007/s00344-014-9453-x
Bhattacharyya, P. N., and Jha, D. K. (2012). Plant growth-promoting rhizobacteria (PGPR): emergence in agriculture. World J. Microbiol. Biotechnol. 28, 1327–1350. doi: 10.1007/s11274-011-0979-9
Bisbis, M. B., Gruda, N., and Blanke, M. (2018). Potential impacts of climate change on vegetable production and product quality–A review. J. Clean. Product. 170, 1602–1620. doi: 10.1016/j.jclepro.2017.09.224
Bouffaud, M.-L., Renoud, S., Dubost, A., Moënne-Loccoz, Y., and Muller, D. (2018). 1-Aminocyclopropane-1-carboxylate deaminase producers associated to maize and other Poaceae species. Microbiome 6:114. doi: 10.1186/s40168-018-0503-7
Bray, E. A. (2000). “Response to abiotic stress,” in Biochemistry and Molecular Biology of Plants, eds B. B. Buchanan, W. Gruissem, and R. L. Jones (Rockville, MD: ASPP), 1158–1203.
Byerlee, D., Stevenson, J., and Villoria, N. (2014). Does intensification slow crop land expansion or encourage deforestation? Glob. Food Secur. 3, 92–98.
Calvo, P., Nelson, L., and Kloepper, J. W. (2014). Agricultural uses of plant biostimulants. Plant Soil 383, 3–41.
Cassia, R., Nocioni, M., Correa-Aragunde, N., and Lamattina, L. (2018). Climate change and the impact of greenhouse gasses: CO2 and NO, friends and foes of plant oxidative stress. Front. Plant Sci. 9:273. doi: 10.3389/fpls.2018.00273
Cavagnaro, T. R., Bender, S. F., Asghari, H. R., and Van Der Heijden, M. G. (2015). The role of arbuscular mycorrhizas in reducing soil nutrient loss. Trends Plant Sci. 20, 283–290. doi: 10.1016/j.tplants.2015.03.004
Clúa, J., Roda, C., Zanetti, M. E., and Blanco, F. A. (2018). Compatibility between legumes and rhizobia for the establishment of a successful nitrogen-fixing symbiosis. Genes 9:125. doi: 10.3390/genes9030125
Compant, S., Samad, A., Faist, H., and Sessitsch, A. (2019). A review on the plant microbiome: ecology, functions and emerging trends in microbial application. J. Adv. Res. 19, 29–37. doi: 10.1016/j.jare.2019.03.004
Crafts-Brandner, S. J., and Salvucci, M. E. (2002). Sensitivity of photosynthesis in a C4 plant, maize, to heat stress. Plant Physiol. 129, 1773–1780. doi: 10.1104/pp.002170
De Wolf, E. D., and Isard, S. A. (2007). Disease cycle approach to plant disease prediction. Annu. Rev. Phytopathol. 45, 203–220. doi: 10.1146/annurev.phyto.44.070505.143329
del Carmen Orozco-Mosqueda, M., Glick, B. R., and Santoyo, G. (2020). ACC deaminase in plant growth-promoting bacteria (PGPB): an efficient mechanism to counter salt stress in crops. Microbiol. Res. 235:126439. doi: 10.1016/j.micres.2020.126439
Díaz-Barradas, M., Gallego-Fernández, J., and Zunzunegui, M. (2020). Plant response to water stress of native and non-native Oenothera drummondii populations. Plant Physiol. Biochem. 154, 219–228. doi: 10.1016/j.plaphy.2020.06.001
Dimkpa, C., Merten, D., Svatoš, A., Büchel, G., and Kothe, E. (2009). Siderophores mediate reduced and increased uptake of cadmium by Streptomyces tendae F4 and sunflower (Helianthus annuus), respectively. J. Appl. Microbiol. 107, 1687–1696. doi: 10.1111/j.1365-2672.2009.04355.x
Du Jardin, P. (2015). Plant biostimulants: definition, concept, main categories and regulation. Sci. Hortic. 196, 3–14. doi: 10.1016/j.scienta.2015.09.021
Dubois, M., Van Den Broeck, L., and Inzé, D. (2018). The pivotal role of ethylene in plant growth. Trends Plant Sci. 23, 311–323. doi: 10.1016/j.tplants.2018.01.003
Duc, N. H., Csintalan, Z., and Posta, K. (2018). Arbuscular mycorrhizal fungi mitigate negative effects of combined drought and heat stress on tomato plants. Plant Physiol. Biochem. 132, 297–307. doi: 10.1016/j.plaphy.2018.09.011
Egamberdieva, D. (2012). Pseudomonas chlororaphis: a salt-tolerant bacterial inoculant for plant growth stimulation under saline soil conditions. Acta Physiol. Plant. 34, 751–756. doi: 10.1007/s11738-011-0875-9
Else, M. A., and Jackson, M. B. (1998). Transport of 1-aminocyclopropane-1-carboxylic acid (ACC) in the transpiration stream of tomato (Lycopersicon esculentum) in relation to foliar ethylene production and petiole epinasty. Funct. Plant Biol. 25, 453–458. doi: 10.1071/pp97105
Enebe, M. C., and Babalola, O. O. (2018). The influence of plant growth-promoting rhizobacteria in plant tolerance to abiotic stress: a survival strategy. Appl. Microbiol. Biotechnol. 102, 7821–7835. doi: 10.1007/s00253-018-9214-z
EPA (2021). Greenhouse Gas (GHG) Emissions. Sources of Greenhouse Gas Emissions. Available online at: https://www.epa.gov/ghgemissions/sources-greenhouse-gas-emissions#agriculture (accessed June 8, 2021).
Esmaeel, Q., Miotto, L., Rondeau, M., Leclère, V., Clément, C., Jacquard, C., et al. (2018). Paraburkholderia phytofirmans PsJN-plants interaction: from perception to the induced mechanisms. Front. Microbiol. 9:2093. doi: 10.3389/fmicb.2018.02093
Etesami, H., and Maheshwari, D. K. (2018). Use of plant growth promoting rhizobacteria (PGPRs) with multiple plant growth promoting traits in stress agriculture: action mechanisms and future prospects. Ecotoxicol. Environ. Saf 156, 225–246. doi: 10.1016/j.ecoenv.2018.03.013
Fadiji, A. E., and Babalola, O. O. (2020b). Exploring the potentialities of beneficial endophytes for improved plant growth. Saudi J. Biol. Sci. 27, 3622–3633. doi: 10.1016/j.sjbs.2020.08.002
Fadiji, A. E., and Babalola, O. O. (2020a). Elucidating mechanisms of endophytes used in plant protection and other bioactivities with multifunctional prospects. Front. Bioeng. Biotechnol. 8:467. doi: 10.3389/fbioe.2020.00467
Fadiji, A. E., and Babalola, O. O. (2020c). Metagenomics methods for the study of plant-associated microbial communities: a review. J. Microbiol. Methods 170:105860. doi: 10.1016/j.mimet.2020.105860
Fadiji, A. E., Ayangbenro, A. S., and Babalola, O. O. (2020). Organic Farming enhances the diversity and community structure of endophytic archaea and fungi in maize plant: a shotgun approach. J. Soil Sci. Plant Nutr. 20, 2587–2599. doi: 10.1007/s42729-020-00324-9
Failor, K., Schmale, D. G., Vinatzer, B. A., and Monteil, C. L. (2017). Ice nucleation active bacteria in precipitation are genetically diverse and nucleate ice by employing different mechanisms. ISME J. 11, 2740–2753. doi: 10.1038/ismej.2017.124
Farrar, K., Bryant, D., and Cope-Selby, N. (2014). Understanding and engineering beneficial plant–microbe interactions: plant growth promotion in energy crops. Plant Biotechnol. J. 12, 1193–1206. doi: 10.1111/pbi.12279
Finkel, O. M., Castrillo, G., Paredes, S. H., González, I. S., and Dangl, J. L. (2017). Understanding and exploiting plant beneficial microbes. Curr. Opin. Plant Biol. 38, 155–163. doi: 10.1016/j.pbi.2017.04.018
Fiodor, A., Singh, S., and Pranaw, K. (2021). The contrivance of plant growth promoting microbes to mitigate climate change impact in agriculture. Microorganisms 9:1841. doi: 10.3390/microorganisms9091841
Foster, G., and Rahmstorf, S. (2011). Global temperature evolution 1979–2010. Environ. Res. Lett. 6:044022. doi: 10.1088/1748-9326/6/4/044022
Freimoser, F. M., Rueda-Mejia, M. P., Tilocca, B., and Migheli, Q. (2019). Biocontrol yeasts: mechanisms and applications. World J. Microbiol. Biotechnol. 35:154. doi: 10.1007/s11274-019-2728-4
Funes, I., Aranda, X., Biel, C., Carbó, J., Camps, F., Molina, A. J., et al. (2016). Future climate change impacts on apple flowering date in a Mediterranean subbasin. Agric. Water Manage. 164, 19–27. doi: 10.1016/j.agwat.2015.06.013
Füssel, H.-M., Heinke, J., Popp, A., and Gerten, D. (2012). “Food security in a changing climate,” in Climate Change, Justice and Sustainability: Linking Climate and Development Policy, eds O. Edenhofer, J. Wallacher, H. Lotze-Campen, M. Reder, B. Knopf, and J. Müller (Dordrecht: Springer), 33–43.
Galambos, N., Compant, S., Wäckers, F., Sessitsch, A., Anfora, G., Mazzoni, V., et al. (2021). Beneficial insects deliver plant growth-promoting bacterial endophytes between tomato plants. Microorganisms 9:1294. doi: 10.3390/microorganisms9061294
Garrett, K. A., Nita, M., De Wolf, E., Esker, P. D., Gomez-Montano, L., and Sparks, A. H. (2016). “Plant pathogens as indicators of climate change,” in Climate Change, 2nd Edn, ed. T. M. Letcher (Amsterdam: Elsevier), 325–338. doi: 10.1016/b978-0-444-63524-2.00021-x
Glick, B. R. (2014). Bacteria with ACC deaminase can promote plant growth and help to feed the world. Microbiol. Res. 169, 30–39. doi: 10.1016/j.micres.2013.09.009
Gołasa, P., Wysokiński, M., Bieńkowska-Gołasa, W., Gradziuk, P., Golonko, M., Gradziuk, B., et al. (2021). Sources of greenhouse gas emissions in agriculture, with particular emphasis on emissions from energy used. Energies 14:3784. doi: 10.3390/en14133784
Gong, M., Tang, M., Chen, H., Zhang, Q., and Feng, X. (2013). Effects of two Glomus species on the growth and physiological performance of Sophora davidii seedlings under water stress. New For. 44, 399–408. doi: 10.1007/s11056-012-9349-1
Gornall, J., Betts, R., Burke, E., Clark, R., Camp, J., Willett, K., et al. (2010). Implications of climate change for agricultural productivity in the early twenty-first century. Philos. Trans. R. Soc. Lond. B Biol. Sci. 365, 2973–2989. doi: 10.1098/rstb.2010.0158
Grichko, V. P., and Glick, B. R. (2001). Amelioration of flooding stress by ACC deaminase-containingplant growth-promoting bacteria. Plant Physiol. Biochem. 39, 11–17. doi: 10.1016/s0981-9428(00)01212-2
Grover, M., Ali, S. Z., Sandhya, V., Rasul, A., and Venkateswarlu, B. (2011). Role of microorganisms in adaptation of agriculture crops to abiotic stresses. World J. Microbiol. Biotechnol. 27, 1231–1240. doi: 10.1007/s11274-010-0572-7
Gupta, S., and Pandey, S. (2019). ACC deaminase producing bacteria with multifarious plant growth promoting traits alleviates salinity stress in French bean (Phaseolus vulgaris) plants. Front. Microbiol. 10:1506. doi: 10.3389/fmicb.2019.01506
Gutiérrez-Luna, F. M., López-Bucio, J., Altamirano-Hernández, J., Valencia-Cantero, E., De La Cruz, H. R., and Macías-Rodríguez, L. (2010). Plant growth-promoting rhizobacteria modulate root-system architecture in Arabidopsis thaliana through volatile organic compound emission. Symbiosis 51, 75–83. doi: 10.1007/s13199-010-0066-2
Hawkings, J. R., Linhoff, B. S., Wadham, J. L., Stibal, M., Lamborg, C. H., Carling, G. T., et al. (2021). Large subglacial source of mercury from the southwestern margin of the Greenland Ice Sheet. Nat. Geosci. 14, 496–502. doi: 10.1038/s41561-021-00753-w
Hazarika, T. (2013). Climate change and Indian horticulture: opportunities, challenges and mitigation strategies. Int. J. Environ. Eng. Manage. 4, 629–630.
Heidari, M., and Golpayegani, A. (2012). Effects of water stress and inoculation with plant growth promoting rhizobacteria (PGPR) on antioxidant status and photosynthetic pigments in basil (Ocimum basilicum L.). J. Saudi Soc. Agric. Sci. 11, 57–61. doi: 10.1016/j.jssas.2011.09.001
Hernández-León, R., Rojas-Solís, D., Contreras-Pérez, M., Del Carmen Orozco-Mosqueda, M., Macías-Rodríguez, L. I., and Reyes-De, et al. (2015). Characterization of the antifungal and plant growth-promoting effects of diffusible and volatile organic compounds produced by Pseudomonas fluorescens strains. Biol. Control 81, 83–92. doi: 10.1016/j.biocontrol.2014.11.011
Huang, J., Zhang, G., Zhang, Y., Guan, X., Wei, Y., and Guo, R. (2020). Global desertification vulnerability to climate change and human activities. Land Degrad. Dev. 31, 1380–1391. doi: 10.1002/ldr.3556
Huner, N. P., Öquist, G., Hurry, V. M., Krol, M., Falk, S., and Griffith, M. (1993). Photosynthesis, photoinhibition and low temperature acclimation in cold tolerant plants. Photosynth. Res. 37, 19–39. doi: 10.1007/BF02185436
Hutchins, D. A., Jansson, J. K., Remais, J. V., Rich, V. I., Singh, B. K., and Trivedi, P. (2019). Climate change microbiology—problems and perspectives. Nat. Rev. Microbiol. 17, 391–396. doi: 10.1038/s41579-019-0178-5
Igiehon, N. O., Babalola, O. O., and Aremu, B. R. (2019). Genomic insights into plant growth promoting rhizobia capable of enhancing soybean germination under drought stress. BMC Microbiol. 19:159. doi: 10.1186/s12866-019-1536-1
Igiehon, N. O., Babalola, O. O., Cheseto, X., and Torto, B. (2021). Effects of rhizobia and arbuscular mycorrhizal fungi on yield, size distribution and fatty acid of soybean seeds grown under drought stress. Microbiol. Res. 242:126640. doi: 10.1016/j.micres.2020.126640
Igiehon, O. N., and Babalola, O. O. (2021). Rhizobium and mycorrhizal fungal species improved soybean yield under drought stress conditions. Curr. Microbiol. 78, 1615–1627. doi: 10.1007/s00284-021-02432-w
IPCC (2014). “Mitigation of climate change,” in Contribution of Working Group III to the Fifth Assessment Report of the Intergovernmental Panel on Climate Change, eds O. Edenhofer, R. Pichs-Madruga, Y. Sokona, E. Farahani, S. Kadner, K. Seyboth, et al. (Cambridge: Cambridge University Press).
Ipek, M., Pirlak, L., Esitken, A., Figen Dönmez, M., Turan, M., and Sahin, F. (2014). Plant growth-promoting rhizobacteria (PGPR) increase yield, growth and nutrition of strawberry under high-calcareous soil conditions. J. Plant Nutr. 37, 990–1001. doi: 10.1080/01904167.2014.881857
Jaemsaeng, R., Jantasuriyarat, C., and Thamchaipenet, A. (2018). Positive role of 1-aminocyclopropane-1-carboxylate deaminase-producing endophytic Streptomyces sp. GMKU 336 on flooding resistance of mung bean. Agric. Nat. Resour. 52, 330–334. doi: 10.1016/j.anres.2018.09.008
Jagadish, S. K., Way, D. A., and Sharkey, T. D. (2021). Plant heat stress: concepts directing future research. Plant Cell Environ. 44, 1992–2005. doi: 10.1111/pce.14050
Jahromi, F., Aroca, R., Porcel, R., and Ruiz-Lozano, J. M. (2008). Influence of salinity on the in vitro development of Glomus intraradices and on the in vivo physiological and molecular responses of mycorrhizal lettuce plants. Microb. Ecol. 55, 45–53. doi: 10.1007/s00248-007-9249-7
Jayakumar, A., Padmakumar, P., Nair, I. C., and Radhakrishnan, E. (2020). Drought tolerant bacterial endophytes with potential plant probiotic effects from Ananas comosus. Biologia 75, 1769–1778. doi: 10.2478/s11756-020-00483-1
Jobe, T. O., Rahimzadeh Karvansara, P., Zenzen, I., and Kopriva, S. (2020). Ensuring nutritious food under elevated CO2 conditions: a case for improved C4 crops. Front. Plant Sci. 11:1267. doi: 10.3389/fpls.2020.01267
Jordan, D. B., and Ogren, W. L. (1984). The CO2/O2 specificity of ribulose 1, 5-bisphosphate carboxylase/oxygenase. Planta 161, 308–313. doi: 10.1007/bf00398720
Kang, S.-M., Radhakrishnan, R., Khan, A. L., Kim, M.-J., Park, J.-M., Kim, B.-R., et al. (2014). Gibberellin secreting rhizobacterium, Pseudomonas putida H-2-3 modulates the hormonal and stress physiology of soybean to improve the plant growth under saline and drought conditions. Plant Physiol. Biochem. 84, 115–124. doi: 10.1016/j.plaphy.2014.09.001
Kang, S.-M., Radhakrishnan, R., You, Y.-H., Khan, A. L., Park, J.-M., Lee, S.-M., et al. (2015). Cucumber performance is improved by inoculation with plant growth-promoting microorganisms. Acta Agric. Scand. Sect. B Soil Plant 65, 36–44. doi: 10.1080/09064710.2014.960889
Kaushal, M., and Wani, S. P. (2016). Plant-growth-promoting rhizobacteria: drought stress alleviators to ameliorate crop production in drylands. Ann. Microbiol. 66, 35–42. doi: 10.1007/s13213-015-1112-3
Khan, A. L., Hussain, J., Al-Harrasi, A., Al-Rawahi, A., and Lee, I.-J. (2015). Endophytic fungi: resource for gibberellins and crop abiotic stress resistance. Crit. Rev. Biotechnol. 35, 62–74. doi: 10.3109/07388551.2013.800018
Khan, M. A., Asaf, S., Khan, A. L., Ullah, I., Ali, S., Kang, S.-M., et al. (2019). Alleviation of salt stress response in soybean plants with the endophytic bacterial isolate Curtobacterium sp. SAK1. Ann. Microbiol. 69, 797–800. doi: 10.1007/s13213-019-01470-x
Khan, N., Bano, A., and Zandi, P. (2018). Effects of exogenously applied plant growth regulators in combination with PGPR on the physiology and root growth of chickpea (Cicer arietinum) and their role in drought tolerance. J. Plant Interact. 13, 239–247. doi: 10.1080/17429145.2018.1471527
Kim, H., Orser, C., Lindow, S., and Sands, D. (1987). Xanthomonas campestris pv. translucens strains active in ice nucleation. Plant Dis. 71, 994–997.
Kour, D., Kaur, T., Devi, R., Rana, K. L., Yadav, N., Rastegari, A. A., et al. (2020a). “Kour, Biotechnological applications of beneficial microbiomes for evergreen agriculture and human health,” in New and Future Developments in Microbial Biotechnology and Bioengineering, eds A. A. Rastegari, A. N. Yadav, and N. Yadav (Elsevier), 255–279. doi: 10.1016/b978-0-12-820528-0.00019-3
Kour, D., Rana, K. L., Sheikh, I., Kumar, V., Yadav, A. N., Dhaliwal, H. S., et al. (2020c). Alleviation of drought stress and plant growth promotion by Pseudomonas libanensis EU-LWNA-33, a drought-adaptive phosphorus-solubilizing bacterium. Proc. Natl. Acad. Sci. India B Biol. Sci. 90, 785–795. doi: 10.1007/s40011-019-01151-4
Kour, D., Rana, K. L., Yadav, A. N., Sheikh, I., Kumar, V., Dhaliwal, H. S., et al. (2020d). Amelioration of drought stress in Foxtail millet (Setaria italica L.) by P-solubilizing drought-tolerant microbes with multifarious plant growth promoting attributes. Environ. Sustain. 3, 23–34. doi: 10.1007/s42398-020-00094-1
Kour, D., Rana, K. L., Kaur, T., Sheikh, I., Yadav, A. N., Kumar, V., et al. (2020b). Microbe-mediated alleviation of drought stress and acquisition of phosphorus in great millet (Sorghum bicolour L.) by drought-adaptive and phosphorus-solubilizing microbes. Biocatal. Agric. Biotechnol. 23:101501.
Ku, S.-B., and Edwards, G. E. (1977). Oxygen inhibition of photosynthesis: I. Temperature dependence and relation to O2/CO2 solubility ratio. Plant Physiol. 59, 986–990. doi: 10.1007/BF00389372
Kumar, A., and Verma, J. P. (2018). Does plant—microbe interaction confer stress tolerance in plants: a review? Microbiol. Res. 207, 41–52. doi: 10.1016/j.micres.2017.11.004
Kumar, N., Khamzina, A., Knöfel, P., Lamers, J., and Tischbein, B. (2021). Afforestation of degraded croplands as a water-saving option in irrigated region of the aral sea basin. Water 13:1433. doi: 10.3390/w13101433
Laanisto, L., and Niinemets, Ü. (2015). Polytolerance to abiotic stresses: How universal is the shade–drought tolerance trade-off in woody species? Glob. Ecol. Biogeogr. 24, 571–580. doi: 10.1111/geb.12288
Ledger, T., Rojas, S., Timmermann, T., Pinedo, I., Poupin, M. J., Garrido, T., et al. (2016). Volatile-mediated effects predominate in Paraburkholderia phytofirmans growth promotion and salt stress tolerance of Arabidopsis thaliana. Front. Microbiol. 7:1838. doi: 10.3389/fmicb.2016.01838
Lee, G., Lee, S.-H., Kim, K. M., and Ryu, C.-M. (2017). Foliar application of the leaf-colonizing yeast Pseudozyma churashimaensis elicits systemic defense of pepper against bacterial and viral pathogens. Sci. Rep. 7:39432. doi: 10.1038/srep39432
Lee, M. R., Lee, R. E. Jr., Strong-Gunderson, J. M., and Minges, S. R. (1995). Isolation of ice-nucleating active bacteria from the freeze-tolerant frog, Rana sylvatica. Cryobiology 32, 358–365. doi: 10.1006/cryo.1995.1036
Lemanceau, P., Blouin, M., Muller, D., and Moënne-Loccoz, Y. (2017). Let the core microbiota be functional. Trends Plant Sci. 22, 583–595. doi: 10.1016/j.tplants.2017.04.008
Li, J., Mcconkey, B. J., Cheng, Z., Guo, S., and Glick, B. R. (2013). Identification of plant growth-promoting bacteria-responsive proteins in cucumber roots under hypoxic stress using a proteomic approach. J. Proteomics 84, 119–131. doi: 10.1016/j.jprot.2013.03.011
Li, T., Hu, Y.-J., Hao, Z.-P., Li, H., and Chen, B.-D. (2013). Aquaporin genes GintAQPF1 and GintAQPF2 from Glomus intraradices contribute to plant drought tolerance. Plant Signal. Behav. 8:e24030. doi: 10.4161/psb.24030
Lim, J.-H., and Kim, S.-D. (2013). Induction of drought stress resistance by multi-functional PGPR Bacillus licheniformis K11 in pepper. Plant Pathol. J. 29, 201–208. doi: 10.5423/PPJ.SI.02.2013.0021
Lindow, S. (1983). The role of bacterial ice nucleation in frost injury to plants. Annu. Rev. Phytopathol. 21, 363–384. doi: 10.1146/annurev.py.21.090183.002051
Lindow, S. E., and Brandl, M. T. (2003). Microbiology of the phyllosphere. Appl. Environ. Microbiol. 69, 1875–1883. doi: 10.1128/aem.69.4.1875-1883.2003
Lindow, S., Arny, D., and Upper, C. (1978). Erwinia herbicola: a bacterial ice nucleus active in increasing frost injury to corn. Phytopathology 68, 523–527.
Liu, C.-Y., Zhang, F., Zhang, D.-J., Srivastava, A., Wu, Q.-S., and Zou, Y.-N. (2018). Mycorrhiza stimulates root-hair growth and IAA synthesis and transport in trifoliate orange under drought stress. Sci. Rep. 8:1978. doi: 10.1038/s41598-018-20456-4
Liu, F., Xing, S., Ma, H., Du, Z., and Ma, B. (2013). Cytokinin-producing, plant growth-promoting rhizobacteria that confer resistance to drought stress in Platycladus orientalis container seedlings. Appl. Microbiol. Biotechnol. 97, 9155–9164. doi: 10.1007/s00253-013-5193-2
Lobell, D. B., and Gourdji, S. M. (2012). The influence of climate change on global crop productivity. Plant Physiol. 160, 1686–1697. doi: 10.1104/pp.112.208298
Loladze, I. (2014). Hidden shift of the ionome of plants exposed to elevated CO2 depletes minerals at the base of human nutrition. eLife 3:e02245. doi: 10.7554/eLife.02245
Luedeling, E. (2012). Climate change impacts on winter chill for temperate fruit and nut production: a review. Sci. Hortic. 144, 218–229.
Malhotra, S. (2017). Horticultural crops and climate change: a review. Indian J. Agric. Sci. 87, 12–22.
Marasco, R., Rolli, E., Ettoumi, B., Vigani, G., Mapelli, F., Borin, S., et al. (2012). A drought resistance-promoting microbiome is selected by root system under desert farming. PLoS One 7:e48479. doi: 10.1371/journal.pone.0048479
Mayak, S., Tirosh, T., and Glick, B. R. (2004). Plant growth-promoting bacteria that confer resistance to water stress in tomatoes and peppers. Plant Sci. 166, 525–530. doi: 10.1016/j.plantsci.2003.10.025
Mazzola, M., and Freilich, S. (2017). Prospects for biological soilborne disease control: application of indigenous versus synthetic microbiomes. Phytopathology 107, 256–263. doi: 10.1094/PHYTO-09-16-0330-RVW
Meena, K. K., Sorty, A. M., Bitla, U. M., Choudhary, K., Gupta, P., Pareek, A., et al. (2017). Abiotic stress responses and microbe-mediated mitigation in plants: the omics strategies. Front. Plant Sci. 8:172. doi: 10.3389/fpls.2017.00172
Mishra, P. K., Bisht, S. C., Bisht, J. K., and Bhatt, J. C. (2012). “Cold-tolerant PGPRs as bioinoculants for stress management,” in Bacteria in Agrobiology: Stress Management, ed. D. K. Maheshwari (Berlin: Springer), 95–118.
Mishra, P. K., Mishra, S., Selvakumar, G., Bisht, S. C., Bisht, J. K., Kundu, S., et al. (2008). Characterisation of a psychrotolerant plant growth promoting Pseudomonas sp. strain PGERs17 (MTCC 9000) isolated from North Western Indian Himalayas. Ann. Microbiol. 58, 561–568.
Morales-Cedeńo, L. R., De Los Santos-Villalobos, S., and Santoyo, G. (2021). Functional and genomic analysis of Rouxiella badensis SER3 as a novel biocontrol agent of fungal pathogens. Front. Microbiol. 12:709855. doi: 10.3389/fmicb.2021.709855
Msimbira, L. A., and Smith, D. L. (2020). The roles of plant growth promoting microbes in enhancing plant tolerance to acidity and alkalinity stresses. Front. Sustain. Food Syst. 4:106. doi: 10.3389/fsufs.2020.00106
Müller, C., Cramer, W., Hare, W. L., and Lotze-Campen, H. (2011). Climate change risks for African agriculture. Proc. Natl. Acad. Sci. U.S.A. 108, 4313–4315.
Munns, R. (2002). Comparative physiology of salt and water stress. Plant Cell Environ. 25, 239–250. doi: 10.1046/j.0016-8025.2001.00808.x
Myers, S. S., Smith, M. R., Guth, S., Golden, C. D., Vaitla, B., Mueller, N. D., et al. (2017). Climate change and global food systems: potential impacts on food security and undernutrition. Annu. Rev. Public Health 38, 259–277. doi: 10.1146/annurev-publhealth-031816-044356
Nadeem, S. M., Ahmad, M., Zahir, Z. A., Javaid, A., and Ashraf, M. (2014). The role of mycorrhizae and plant growth promoting rhizobacteria (PGPR) in improving crop productivity under stressful environments. Biotechnol. Adv. 32, 429–448. doi: 10.1016/j.biotechadv.2013.12.005
Nafady, N. A., Hashem, M., Hassan, E. A., Ahmed, H. A., and Alamri, S. A. (2019). The combined effect of arbuscular mycorrhizae and plant-growth-promoting yeast improves sunflower defense against Macrophomina phaseolina diseases. Biol. Control 138:104049. doi: 10.1016/j.biocontrol.2019.104049
Naveed, M., Mitter, B., Reichenauer, T. G., Wieczorek, K., and Sessitsch, A. (2014). Increased drought stress resilience of maize through endophytic colonization by Burkholderia phytofirmans PsJN and Enterobacter sp. FD17. Environ. Exp. Bot. 97, 30–39. doi: 10.1016/j.envexpbot.2013.09.014
Nwodo, U. U., Green, E., and Okoh, A. I. (2012). Bacterial exopolysaccharides: functionality and prospects. Int. J. Mol. Sci. 13, 14002–14015. doi: 10.3390/ijms131114002
Ojuederie, O. B., Olanrewaju, O. S., and Babalola, O. O. (2019). Plant growth promoting rhizobacterial mitigation of drought stress in crop plants: implications for sustainable agriculture. Agronomy 9:712. doi: 10.3390/agronomy9110712
Omomowo, I. O., Fadiji, A. E., and Omomowo, O. I. (2018). Assessment of bio-efficacy of Glomus versiforme and Trichoderma harzianum in inhibiting powdery mildew disease and enhancing the growth of cowpea. Ann. Agric. Sci. 63, 9–17. doi: 10.1016/j.aoas.2018.03.001
Orozco-Mosqueda, M., Flores, A., Rojas-Sánchez, B., Urtis-Flores, C. A., Morales-Cedeño, L. R., Valencia-Marin, M. F., et al. (2021). Plant growth-promoting bacteria as bioinoculants: attributes and challenges for sustainable crop improvement. Agronomy 11:1167. doi: 10.1002/jobm.202000370
Othman, Y., Al-Karaki, G., Al-Tawaha, A., and Al-Horani, A. (2006). Variation in germination and ion uptake in barley genotypes under salinity conditions. World J. Agric. Sci. 2, 11–15.
Ouyang, L., Pei, H., and Xu, Z. (2017). Low nitrogen stress stimulating the indole-3-acetic acid biosynthesis of Serratia sp. ZM is vital for the survival of the bacterium and its plant growth-promoting characteristic. Arch. Microbiol. 199, 425–432. doi: 10.1007/s00203-016-1312-7
Park, Y.-G., Mun, B.-G., Kang, S.-M., Hussain, A., Shahzad, R., Seo, C.-W., et al. (2017). Bacillus aryabhattai SRB02 tolerates oxidative and nitrosative stress and promotes the growth of soybean by modulating the production of phytohormones. PLoS One 12:e0173203. doi: 10.1371/journal.pone.0173203
Paul, M. J., Primavesi, L. F., Jhurreea, D., and Zhang, Y. (2008). Trehalose metabolism and signaling. Annu. Rev. Plant Biol. 59, 417–441. doi: 10.1146/annurev.arplant.59.032607.092945
Pearson, S., Wheeler, T., Hadley, P., and Wheldon, A. (1997). A validated model to predict the effects of environment on the growth of lettuce (Lactuca sativa L.): implications for climate change. J. Hortic. Sci. 72, 503–517. doi: 10.1080/14620316.1997.11515538
Perkins-Kirkpatrick, S., and Lewis, S. (2020). Increasing trends in regional heatwaves. Nat. Commun. 11:3357. doi: 10.1038/s41467-020-16970-7
Pouleur, S., Richard, C., Martin, J.-G., and Antoun, H. (1992). Ice nucleation activity in Fusarium acuminatum and Fusarium avenaceum. Appl. Environ. Microbiol. 58, 2960–2964. doi: 10.1128/aem.58.9.2960-2964.1992
Preininger, C., Sauer, U., Bejarano, A., and Berninger, T. (2018). Concepts and applications of foliar spray for microbial inoculants. Appl. Microbiol. Biotechnol. 102, 7265–7282. doi: 10.1007/s00253-018-9173-4
Purahong, W., Orrù, L., Donati, I., Perpetuini, G., Cellini, A., Lamontanara, A., et al. (2018). Plant microbiome and its link to plant health: host species, organs and Pseudomonas syringae pv. actinidiae infection shaping bacterial phyllosphere communities of kiwifruit plants. Front. Plant Sci. 9:1563. doi: 10.3389/fpls.2018.01563
Qu, A.-L., Ding, Y.-F., Jiang, Q., and Zhu, C. (2013). Molecular mechanisms of the plant heat stress response. Biochem. Biophys. Res. Commun. 432, 203–207. doi: 10.1016/j.bbrc.2013.01.104
Rasool, S., Hameed, A., Azooz, M., Siddiqi, T., and Ahmad, P. (2013). “Salt stress: Causes, types and responses of plants,” in Ecophysiology and Responses of Plants Under Salt Stress, eds M. N. V. Prasad and P. Ahmad (New York, NY: Springer), 1–24. doi: 10.1007/978-1-4614-4747-4_1
Rising, J., and Devineni, N. (2020). Crop switching reduces agricultural losses from climate change in the United States by half under RCP 8.5. Nat. Commun. 11:4991. doi: 10.1038/s41467-020-18725-w
Rouphael, Y., Cardarelli, M., Schwarz, D., Franken, P., and Colla, G. (2012). “Effects of drought on nutrient uptake and assimilation in vegetable crops,” in Plant Responses to Drought Stress, ed. R. Aroca (Springer: Berlin), 171–195. doi: 10.1111/plb.13304
Russo, S., Dosio, A., Graversen, R. G., Sillmann, J., Carrao, H., Dunbar, M. B., et al. (2014). Magnitude of extreme heat waves in present climate and their projection in a warming world. J. Geophys. Res. Atmos. 119, 12500–12512.
Ruzzi, M., and Aroca, R. (2015). Plant growth-promoting rhizobacteria act as biostimulants in horticulture. Sci. Hortic. 196, 124–134. doi: 10.1016/j.scienta.2015.08.042
Sanders, G. J., and Arndt, S. K. (2012). “Osmotic adjustment under drought conditions,” in Plant Responses to Drought Stress, ed. R. A. Hernandez-Ros (Berlin: Springer), 199–229. doi: 10.1007/978-3-642-32653-0_8
Sangiorgio, D., Cellini, A., Donati, I., Pastore, C., Onofrietti, C., and Spinelli, F. (2020). Facing climate change: application of microbial biostimulants to mitigate stress in horticultural crops. Agronomy 10:794. doi: 10.3390/agronomy10060794
Santoyo, G., Guzmán-Guzmán, P., Parra-Cota, F. I., Santos-Villalobos, S. D. L., Orozco-Mosqueda, M., and Glick, B. R. (2021b). Plant growth stimulation by microbial consortia. Agronomy 11:219. doi: 10.3390/agronomy11020219
Santoyo, G., Gamalero, E., and Glick, B. R. (2021a). Mycorrhizal-bacterial amelioration of plant abiotic and biotic stress. Front. Sustain. Food Syst. 5:139. doi: 10.3389/fsufs.2021.672881
Sarabia, M., Cazares, S., González-Rodríguez, A., Mora, F., Carreón-Abud, Y., and Larsen, J. (2018). Plant growth promotion traits of rhizosphere yeasts and their response to soil characteristics and crop cycle in maize agroecosystems. Rhizosphere 6, 67–73. doi: 10.1016/j.rhisph.2018.04.002
Saravanakumar, D., and Samiyappan, R. (2007). ACC deaminase from Pseudomonas fluorescens mediated saline resistance in groundnut (Arachis hypogea) plants. J. Appl. Microbiol. 102, 1283–1292. doi: 10.1111/j.1365-2672.2006.03179.x
Seguini, L., Bussay, A., and Baruth, B. (2019). From extreme weather to impacts: the role of the areas of concern maps in the JRC MARS bulletin. Agric. Syst. 168, 213–223. doi: 10.1016/j.agsy.2018.07.003
Selosse, M.-A., and Le Tacon, F. (1998). The land flora: A phototroph-fungus partnership? Trends Ecol. Evol. 13, 15–20. doi: 10.1016/s0169-5347(97)01230-5
Selvakumar, G., Kundu, S., Joshi, P., Nazim, S., Gupta, A., Mishra, P., et al. (2008a). Characterization of a cold-tolerant plant growth-promoting bacterium Pantoea dispersa 1A isolated from a sub-alpine soil in the North Western Indian Himalayas. World J. Microbiol. Biotechnol. 24, 955–960. doi: 10.1007/s11274-007-9558-5
Selvakumar, G., Mohan, M., Kundu, S., Gupta, A., Joshi, P., Nazim, S., et al. (2008b). Cold tolerance and plant growth promotion potential of Serratia marcescens strain SRM (MTCC 8708) isolated from flowers of summer squash (Cucurbita pepo). Lett. Appl. Microbiol. 46, 171–175. doi: 10.1111/j.1472-765X.2007.02282.x
Selvakumar, G., Panneerselvam, P., and Ganeshamurthy, A. N. (2012). “Bacterial mediated alleviation of abiotic stress in crops,” in Bacteria in Agrobiology: Stress Management, ed. D. K. Maheshwari (Berlin: Springer), 205–224. doi: 10.1007/978-3-662-45795-5_10
Sharifi, R., and Ryu, C.-M. (2018). Sniffing bacterial volatile compounds for healthier plants. Curr. Opin. Plant Biol. 44, 88–97. doi: 10.1016/j.pbi.2018.03.004
Shintu, P., and Jayaram, K. (2015). Phosphate solubilising bacteria (Bacillus polymyxa)-An effective approach to mitigate drought in tomato (Lycopersicon esculentum Mill.). Trop. Plant Res. 2, 17–22.
Shulaev, V., Cortes, D., Miller, G., and Mittler, R. (2008). Metabolomics for plant stress response. Physiol. Plant. 132, 199–208. doi: 10.1111/j.1399-3054.2007.01025.x
Skirvin, R., Kohler, E., Steiner, H., Ayers, D., Laughnan, A., Norton, M., et al. (2000). The use of genetically engineered bacteria to control frost on strawberries and potatoes. Whatever happened to all of that research? Sci. Hortic. 84, 179–189. doi: 10.1016/s0304-4238(99)00097-7
Smirnoff, N. (1993). The role of active oxygen in the response of plants to water deficit and desiccation. New Phytol. 125, 27–58. doi: 10.1111/j.1469-8137.1993.tb03863.x
Song, Y., Chen, Q., Ci, D., Shao, X., and Zhang, D. (2014). Effects of high temperature on photosynthesis and related gene expression in poplar. BMC Plant Biol. 14:111. doi: 10.1186/1471-2229-14-111
Stevenson, S., Fox-Kemper, B., Jochum, M., Neale, R., Deser, C., and Meehl, G. (2012). Will there be a significant change to El Niño in the twenty-first century? J. Clim. 25, 2129–2145.
Szczałba, M., Kopta, T., Gąstoł, M., and Sêkara, A. (2019). Comprehensive insight into arbuscular mycorrhizal fungi, Trichoderma spp. and plant multilevel interactions with emphasis on biostimulation of horticultural crops. J. Appl. Microbiol. 127, 630–647. doi: 10.1111/jam.14247
Tahir, H. A., Gu, Q., Wu, H., Raza, W., Hanif, A., Wu, L., et al. (2017). Plant growth promotion by volatile organic compounds produced by Bacillus subtilis SYST2. Front. Microbiol. 8:171. doi: 10.3389/fmicb.2017.00171
Tahir, M., Ahmad, I., Shahid, M., Shah, G. M., Farooq, A. B. U., Akram, M., et al. (2019). Regulation of antioxidant production, ion uptake and productivity in potato (Solanum tuberosum L.) plant inoculated with growth promoting salt tolerant Bacillus strains. Ecotoxicol. Environ. Saf. 178, 33–42. doi: 10.1016/j.ecoenv.2019.04.027
Tallon, R., Bressollier, P., and Urdaci, M. C. (2003). Isolation and characterization of two exopolysaccharides produced by Lactobacillus plantarum EP56. Res. Microbiol. 154, 705–712. doi: 10.1016/j.resmic.2003.09.006
Tewari, S., and Arora, N. K. (2014). Multifunctional exopolysaccharides from Pseudomonas aeruginosa PF23 involved in plant growth stimulation, biocontrol and stress amelioration in sunflower under saline conditions. Curr. Microbiol. 69, 484–494. doi: 10.1007/s00284-014-0612-x
Theocharis, A., Bordiec, S., Fernandez, O., Paquis, S., Dhondt-Cordelier, S., Baillieul, F., et al. (2012). Burkholderia phytofirmans PsJN primes Vitis vinifera L. and confers a better tolerance to low nonfreezing temperatures. Mol. Plant Microbe Interact. 25, 241–249. doi: 10.1094/MPMI-05-11-0124
Tiryaki, D., Aydın, İ., and Atıcı, Ö. (2019). Psychrotolerant bacteria isolated from the leaf apoplast of cold-adapted wild plants improve the cold resistance of bean (Phaseolus vulgaris L.) under low temperature. Cryobiology 86, 111–119. doi: 10.1016/j.cryobiol.2018.11.001
Trenberth, K. E., Dai, A., Van Der Schrier, G., Jones, P. D., Barichivich, J., Briffa, K. R., et al. (2014). Global warming and changes in drought. Nat. Clim. Change 4, 17–22.
Ulloa-Muñoz, R., Olivera-Gonzales, P., Castañeda-Barreto, A., Villena, G. K., and Tamariz-Angeles, C. (2020). Diversity of endophytic plant-growth microorganisms from Gentianella weberbaueri and Valeriana pycnantha, highland Peruvian medicinal plants. Microbiol. Res. 233:126413. doi: 10.1016/j.micres.2020.126413
Unterberger, C., Brunner, L., Nabernegg, S., Steininger, K. W., Steiner, A. K., Stabentheiner, E., et al. (2018). Spring frost risk for regional apple production under a warmer climate. PLoS One 13:e0200201. doi: 10.1371/journal.pone.0200201
Vacheron, J., Renoud, S., Muller, D., Babalola, O. O., and Prigent-Combaret, C. (2015). “Handbook for Azospirillum. Technical Issues and Protocols. Everything practical you always wanted to know about Azospirillum sp. but were afraid to ask,” in Alleviation of Abiotic and Biotic Stresses in Plants by Azospirillum, eds F. D. Cassan, Y. Okon, M. Cecilia, and M. C. Creus (Cham: Springer), 333–365.
Vaishnav, A., Kumari, S., Jain, S., Varma, A., Tuteja, N., and Choudhary, D. K. (2016). PGPR-mediated expression of salt tolerance gene in soybean through volatiles under sodium nitroprusside. J. Basic Microbiol. 56, 1274–1288. doi: 10.1002/jobm.201600188
Vandenkoornhuyse, P., Quaiser, A., Duhamel, M., Le Van, A., and Dufresne, A. (2015). The importance of the microbiome of the plant holobiont. New Phytol. 206, 1196–1206. doi: 10.1111/nph.13312
Vejan, P., Abdullah, R., Khadiran, T., Ismail, S., and Nasrulhaq Boyce, A. (2016). Role of plant growth promoting rhizobacteria in agricultural sustainability—a review. Molecules 21:573. doi: 10.3390/molecules21050573
Vicente-Serrano, S. M., Quiring, S. M., Pena-Gallardo, M., Yuan, S., and Dominguez-Castro, F. (2020). A review of environmental droughts: Increased risk under global warming? Earth Sci. Rev. 201:102953. doi: 10.1080/15287390490492313
Vitasse, Y., and Rebetez, M. (2018). Unprecedented risk of spring frost damage in Switzerland and Germany in 2017. Clim. Change 149, 233–246. doi: 10.1007/s10584-018-2234-y
Vurukonda, S. S. K. P., Vardharajula, S., Shrivastava, M., and Skz, A. (2016). Enhancement of drought stress tolerance in crops by plant growth promoting rhizobacteria. Microbiol. Res. 184, 13–24. doi: 10.1016/j.micres.2015.12.003
Wagg, C., Bender, S. F., Widmer, F., and Van Der Heijden, M. G. A. (2014). Soil biodiversity and soil community composition determine ecosystem multifunctionality. Proc. Natl. Acad. Sci. U.S.A. 111, 5266–5270. doi: 10.1073/pnas.1320054111
Wheeler, T., Hadley, P., Ellis, R., and Morison, J. (1993). Changes in growth and radiation use by lettuce crops in relation to temperature and ontogeny. Agric. For. Meteorol. 66, 173–186. doi: 10.1016/0168-1923(93)90069-t
Wild, A. (2003). Soils, Land and Food: Managing the Land During the Twenty-First Century. Cambridge: Cambridge University Press.
Wu, B., and Mu, C. (2019). Effects on greenhouse gas (CH4, CO2, N2O) emissions of conversion from over-mature forest to secondary forest and Korean pine plantation in northeast China. Forests 10:788. doi: 10.3390/f10090788
Yakhin, O. I., Lubyanov, A. A., Yakhin, I. A., and Brown, P. H. (2017). Biostimulants in plant science: a global perspective. Front. Plant Sci. 7:2049. doi: 10.3389/fpls.2016.02049
Yandigeri, M. S., Meena, K. K., Singh, D., Malviya, N., Singh, D. P., Solanki, M. K., et al. (2012). Drought-tolerant endophytic actinobacteria promote growth of wheat (Triticum aestivum) under water stress conditions. Plant Growth Regul. 68, 411–420. doi: 10.1007/s10725-012-9730-2
Yang, J., Kloepper, J. W., and Ryu, C.-M. (2009). Rhizosphere bacteria help plants tolerate abiotic stress. Trends Plant Sci. 14, 1–4. doi: 10.1016/j.tplants.2008.10.004
Yue, X., and Gao, Q. (2018). Contributions of natural systems and human activity to greenhouse gas emissions. Adv. Clim. Change Res. 9, 243–252. doi: 10.1016/j.accre.2018.12.003
Yusuf, A. M., Abubakar, A. B., and Mamman, S. O. (2020). Relationship between greenhouse gas emission, energy consumption, and economic growth: evidence from some selected oil-producing African countries. Environ. Sci. Pollut. Res. Int. 27, 15815–15823. doi: 10.1007/s11356-020-08065-z
Zhang, H., Murzello, C., Sun, Y., Kim, M.-S., Xie, X., Jeter, R. M., et al. (2010). Choline and osmotic-stress tolerance induced in Arabidopsis by the soil microbe Bacillus subtilis (GB03). Mol. Plant Microbe Interact. 23, 1097–1104. doi: 10.1094/MPMI-23-8-1097
Zhang, H., Pan, C., Gu, S., Ma, Q., Zhang, Y., Li, X., et al. (2019). Stomatal movements are involved in elevated CO2-mitigated high temperature stress in tomato. Physiol. Plant. 165, 569–583. doi: 10.1111/ppl.12752
Keywords: arbuscular mycorrhizal fungi, plant-microbe interaction, PGPR, sustainable agriculture, climate change
Citation: Fadiji AE, Babalola OO, Santoyo G and Perazzolli M (2022) The Potential Role of Microbial Biostimulants in the Amelioration of Climate Change-Associated Abiotic Stresses on Crops. Front. Microbiol. 12:829099. doi: 10.3389/fmicb.2021.829099
Received: 04 December 2021; Accepted: 29 December 2021;
Published: 14 January 2022.
Edited by:
Mahaveer P. Sharma, ICAR Indian Institute of Soybean Research, IndiaReviewed by:
Puneet Singh Chauhan, National Botanical Research Institute (CSIR), IndiaCopyright © 2022 Fadiji, Babalola, Santoyo and Perazzolli. This is an open-access article distributed under the terms of the Creative Commons Attribution License (CC BY). The use, distribution or reproduction in other forums is permitted, provided the original author(s) and the copyright owner(s) are credited and that the original publication in this journal is cited, in accordance with accepted academic practice. No use, distribution or reproduction is permitted which does not comply with these terms.
*Correspondence: Olubukola Oluranti Babalola, b2x1YnVrb2xhLmJhYmFsb2xhQG53dS5hYy56YQ==
Disclaimer: All claims expressed in this article are solely those of the authors and do not necessarily represent those of their affiliated organizations, or those of the publisher, the editors and the reviewers. Any product that may be evaluated in this article or claim that may be made by its manufacturer is not guaranteed or endorsed by the publisher.
Research integrity at Frontiers
Learn more about the work of our research integrity team to safeguard the quality of each article we publish.