- 1School of Food and Biological Engineering, Jiangsu University, Zhenjiang, China
- 2Jiangsu Key Laboratory for Food Quality and Safety-State Key Laboratory Cultivation Base of Ministry of Science and Technology, Institute of Food Safety and Nutrition, Jiangsu Academy of Agricultural Sciences, Nanjing, China
With the rapid emergence and dissemination of antimicrobial resistance (AMR) genes in bacteria from animal, animal-derived food and human clinic, it is of great significance to develop new approaches to combat the multidrug-resistant bacteria. This study presented a short linear antimicrobial peptide RW-BP100-4D, which was derived from RW-BP100 (RRLFRRILRWL-NH2) by transforming the N-terminal 4th amino acid from L- to D-enantiomer. This modification remarkably reduced the peptide cytotoxicity to mammalian cells, as indicated by hemolytic and cytotoxicity assays. Meanwhile, the antimicrobial activity of RW-BP100-4D was improved against a more variety of Gram-positive and Gram-negative bacteria (sensitive and resistant) as well as fungi. Also, RW-BP100-4D showed strong in vitro anti-biofilm activity in a concentration-dependent manner, including inhibition of the biofilm-formation and dispersion of the mature biofilms of Staphylococcus aureus. RW-BP100-4D could be efficiently uptaken by bovine mammary epithelial cells (MAC-T) cells to eliminate the intracellular S. aureus ATCC29213 and Salmonella enterica ATCC13076. Moreover, RW-BP100-4D was highly effective in food disinfection of multiple bacterial contamination (including S. aureus, Listeria monocytogenesis, Escherichia coli O157: H7, Campylobacter jejuni, S. enterica, and Shewanella putrefaction, 3.61 ± 0.063 log reduction) on chicken meat, and could kill 99.99% of the methicillin-resistant Staphylococcus aureus (MRSA) strain in the mouse skin infection model. In summary, RW-BP100-4D is a promising antimicrobial candidate for application on food disinfection and local infection treatment. However, the protease-sensitivity of RW-BP100-4D and toxic effect at higher doses reduced the therapeutic effect of the candidate peptide in vivo and should be improved in the future studies.
Introduction
Antimicrobial peptides (AMPs) are the vital components of innate immune systems found in a wide range of organisms including plants, insects, amphibians, and humans (Zasloff, 2002; Mangoni and Shai, 2011; Pasupuleti et al., 2012). There are many types of AMPs including small bacteriocins, fungal defensins, peptaibols, cyclopeptides, and pseudopeptide (Raaijmakers et al., 2006; Montesinos, 2007; Mangoni and Shai, 2009), which can be used to treat microbial infections such as bacteria, viruses and fungi. Nowadays, with the emergence and prevalence of various antimicrobial resistance (AMR) genes in bacteria, AMPs are considered to be one of the most promising alternatives to conventional antibiotics (Mangoni and Shai, 2011). In general, most AMPs have amphiphilic structures with net positive charges (Zamora-Carreras et al., 2016). Due to the negative charge of the microbial cell membrane, the cationic AMPs could be easily accumulated on the cell membrane by an electrostatic affinity, thus leading to membrane lysis and cell content release (Toke, 2005; Bechinger and Gorr, 2017). Besides, other evidences have suggested that other antimicrobial mechanisms may be involved, such as targeting the intracellular substances (DNA or protein) and related biological process or pathways (Toke, 2005). Considering the complexity of the antimicrobial mechanisms of AMPs, it is believed that resistance to AMPs could hardly be developed (Brogden, 2005). In addition, due to the differences of net charge of anionic lipids between bacteria and eukaryotic cells, AMPs can be rationally designed to selectively target microorganisms, with low toxicity toward mammalian cells (Yeaman and Yount, 2003). This fact further supports the application of AMP as a novel intervention for the treatment of clinical infections in vivo, either alone or act synergistically with conventional antibiotics (Li et al., 2021).
BP100 is a short cationic AMP of 11 amino acids (KKLFKKILKYL-NH2), which is a hybrid of cecropin A (an AMP from the moth Hyalophora cecropia) and melittin M (a membrane-permeabilizing component of bee venom) (Ferre et al., 2009). This peptide displayed good antimicrobial activity against Gram-negative bacteria, with the minimal inhibitory concentration (MIC) of 2.5-5 μM against Escherichia coli and Psudomnas aeruginosa isolates. However, the antimicrobial activity of BP100 against Gram-positive bacteria was poor compared to that of Gram-negative bacteria. Subsequently, several BP100 analogues were designed and one derivative designated RW-BP100 (RRLFRRILRWL-NH2) showed expanded antibacterial activity against both Gram-negative and Gram-positive bacteria, whose Tyr residue was replaced with a Trp and all the Lys residues were substituted with Arg (Torcato et al., 2013). However, the cytotoxicity of RW-BP100 was not desirable. For instance, the hemolytic rate of RW-BP100 (4.9 μM) toward human erythrocytes was 50%, and the cell death rate of HeLa was 73% at the peptide concentration of 11 μM. Therefore, RW-BP100 may not be appropriate for further therapeutical application (Torcato et al., 2013).
To reduce the toxicity of RW-BP100 and improve its antimicrobial activity, a series of RW-BP100 derivatives were designed in this study, including L- to D-enantiomer at each amino acid position from 1st to 11th and the head-to-tail cyclized RW-BP100. According to the initial evaluation by cytotoxicity and antimicrobial activity tests, RW-BP100-4D (RRL{D-F}RRILRWL-NH2) was identified as the ideal candidate. Subsequently, RW-BP100-4D was further comprehensively evaluated for its stress (thermal, pH, and protease) stability, intracellular antibacterial activity, biofilm inhibition ability, food disinfection capacity, and treatment efficacy in mouse local and system infections.
Materials and Methods
Peptide Synthesis
The sequences of RW-BP100 and its serial derivatives were listed in Table 1, including the single substitution of L-amino acid with corresponding D-isomer at each position (RW-BP100-1∼11D), all D-isomers (RW-BP100-All-D), and the N to C-terminal cyclic RW-BP100 (RW-BP100-cycle). All the peptides were synthesized by GenScript Biotechnology Company (Nanjing, China) using the solid-phase method. The cationic peptides were of >95% purity and their structures were verified by mass spectrometry. The peptide powders were stored at −20°C until further experiment. The structural model of RW-BP100-4D was predicted at https://heliquest.ipmc.cnrs.fr/cgi-bin/ComputParams.py, and illustrated in Figure 1.
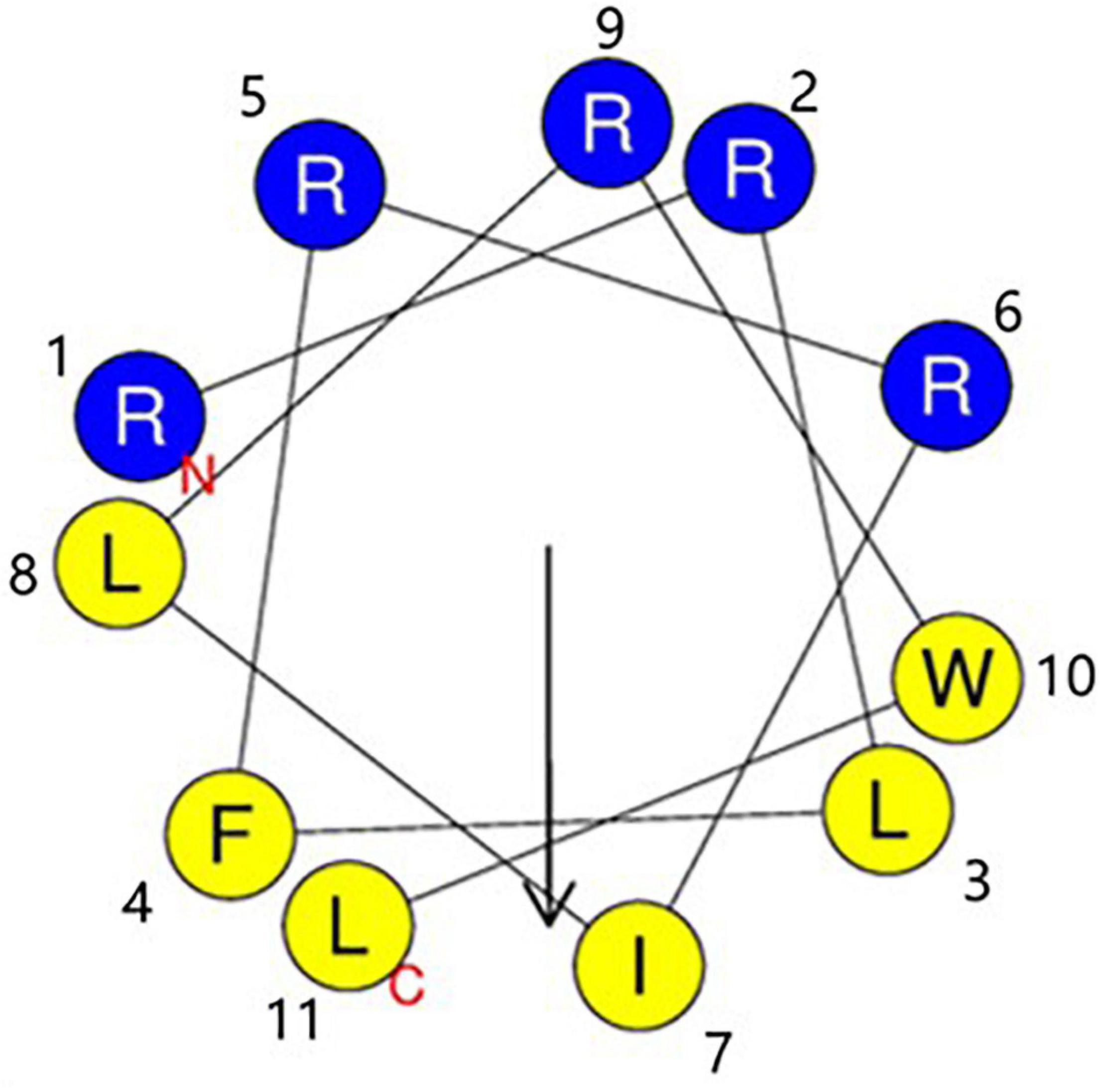
Figure 1. Edmunson wheel projection of the cationic peptide RW-BP100-4D. The structure was predicted at https://heliquest.ipmc.cnrs.fr/cgi-bin/ComputParams.py. RW-BP100-4D displays an amphiphilic structure with a net charge of 5. Blue background stands for hydrophilic amino acids, and yellow background for hydrophobic amino acids. N indicates the 1st amino acid (Arg) and T indicates the 11th amino acid (Leu).
Microbial Strains, Cell Lines, and Animals
Multiple bacterial species were used in this study. Standard strains included Staphylococcus aureus ATCC29213 and S. aureus ATCC33591 (mecA+), Enterococcus faecalis JH2-2, Streptococcus agalactiae ATCC13813, Staphylococcus epidermidis ATCC29887, Listeria monocytogenes ATCC19115, E. coli ATCC25922, Salmonella enterica ATCC13076, Campylobacter jejuni ATCC 33291, Shewanella putrefaction ATCC 49138, and Candida albicans ATCC10231. Clinical isolates were previously collected and identified in our lab, including E. faecalis 32 (optrA+), L. monocytogenes LM08 (tetM +), E. coli A3 (O157:H7), E. coli XG-E1 (blaNDM–5+ and mcr-1+), E. coli 47EC (tet(X4)+), Klebsiella pneumoniae XG-Kpn03 (blaNDM–5+), Acinetobacter baumannii 34AB (tet(X3)+), Pseudomonas aeruginosa 42 (blaOXA–10), Pseudomonas fluorescens AC04, and Fusarium oxysporum LD21. All bacterial strains were stored in 20% glycerol at −80°C. For the subsequent experiments, the bacteria were recovered and cultured in the corresponding enrichment broth at 37°C.
The MAC-T cell line and mouse macrophage leukemia cells RAW 264.7 were cultured in Dulbecco’s modified Eagle’s medium (DMEM), supplemented with 10% (v/v) of fetal bovine serum (Sigma-Aldrich, MO, United States), 100 U/mL of penicillin and 0.1 mg/mL of streptomycin, at 37°C in the presence of 5% CO2.
Female ICR mice (∼18 g, four weeks old) bought from Yangzhou University were used in this study. They were reared with ad libitum access to drinking water and antibiotic-free feed. The animal experiments were conducted in strict accordance with the Laboratory Animal Welfare and Animal Experimental Ethical Certificate, as issued by Committee on Animal Welfare and Experimental Ethics in Jiangsu Academy of Agricultural Sciences (approval no. JAAS20200516 and JAAS20211208).
Antimicrobial Susceptibility Testing
To compare the antibacterial activity of RW-BP100 and its serial derivatives, the MIC against S. aureus ATCC29213 and E. coli ATCC25922 were determined using broth microdilution method according to the guidelines of Clinical and Laboratory Standards Institute (CLSI) M100-S25 (CLSI, 2015). Briefly, peptides were dissolved in sterilized water and twofold diluted in Mueller-Hinton (MH) broth (Beijing Land Bridge Technology) at the concentration ranges of 0.098–100 μg/mL. Then the peptides were mixed with an equal volume (100 μL) of bacterial suspensions of 106 CFU/mL in a sterilized 96-well microtiter plate. After incubation at 37°C for 18 h, the MIC values were determined as the lowest concentrations of peptides with no visible growth of bacteria. To further evaluate the antimicrobial spectrum of RW-BP100-4D, MICs were determined against a more variety of Gram-positive and Gram-negative bacterial species, and the fungal strains (according to CLSI M38 guideline). At the end of incubation, aliquots (100 μL) of the bacterial solutions were placed on MH agar plates, and allowed growth at 37°C overnight for measurement of the minimal bactericidal concentration (MBC). The lowest peptide concentration that displayed no bacterial growth (zero colony) was defined as MBC. MIC and MBC testing were performed with three independent repeats.
Hemolytic and Cytotoxicity Assays
To screen the peptide with lower toxicity, the hemolytic activity of RW-BP100 and its derivatives was tested using fresh defibrinated sheep blood (Beijing Land Bridge Technology company) (Song et al., 2020). Firstly, the sheep blood was centrifuged at 6,000 g for 5 min, washed with phosphate-buffered saline (PBS, 0.01 M, pH 7.4) for three times, and then diluted to the 8% (v/v) red blood cell suspension in PBS. All the peptides were dissolved in PBS and diluted to the concentrations of 100, 200, 300, and 600 μg/mL, respectively. Aliquots (100 μL) of the peptides were mixed with an equal volume of the 8% red blood cell suspension and incubated at 37°C for 1 h. The mixture was then centrifuged at 3,500 g for 10 min and 100 μL of the supernatant was transferred to a 96-well microplate to detect the OD576nm using Infinite M200 Microplate reader (Tecan, Switzerland). Melittin and PBS were used as positive and negative controls. The percentage of hemolysis (H) was calculated as follows: H = 100% × [(Op-Ob)/(Om-Ob)], where Op, Ob, and Om represented the densities of a given peptide, PBS buffer and melittin, respectively. Each test was performed with three independent repeats.
The cytotoxicity of RW-BP100 and its derivatives toward MAC-T and RAW264.7 cells was determined using the water-soluble tetrazolium salt-1 (WST-1) viability assay (Kai et al., 2011). Briefly, the MAC-T and RAW264.7 cells (103 cells/mL) were seeded in a 96-well plate and then added with different peptide (150 μg/mL) for 24 h, respectively. Next, 10 μL of WST-1 (Beyotime, China) was added to each well to incubate for 1 h. The absorbance of the suspensions at 450 nm was measured as described above. Moreover, RW-BP100-4D was determined for its cytotoxicity toward MAC-T and RAW264.7 cells at the concentration ranges of 0–512 μg/mL. The cell survival rate was calculated as follows: (ODdrug – ODblank)/(ODcontrol – ODBlank) × 100%, where ODdrug, ODblank, and ODcontrol are the densities of cultured cells treated with different concentrations of peptide, the culture medium, and growing cells without any treatment, respectively. Each test was conducted with three independent repeats.
Stress Stability Testing
To evaluate the thermal and pH stability of RW-BP100-4D, 100 μL of the peptide (100 μg/mL, in PBS buffer) was incubated under different temperatures (40, 50, 60, 70, 80, 90, and 100°C, pH 7) or different pHs (2, 3, 4, 5, 6, 7, 8 and 9, 37°C) for 1 h (Song et al., 2020). After incubation, the pH of the peptide solutions was adjusted to 7.0 using NaOH or HCl. For protease and serum stability test, 100 μL of RW-BP100-4D (100 μg/mL) was incubated with proteinase K (1 mg/mL), trypsin (1 mg/mL), papain (1 mg/mL), or sterile calf serum at 37°C for 1 h, respectively (Song et al., 2020). After the treatments, the proteases and calf serum were inactivated by heating at 80°C for 30 min. The remnant activity of the treated RW-BP100-4D was evaluated by determining the MICs against E. coli ATCC25922, S. aureus ATCC29213, and C. albicans ATCC10231 using broth microdilution method. RW-BP100-4D without any treatment was used as the control and each test was conducted with three independent repeats. Moreover, degradation rate (%) was estimated as the percentage of degraded peptide calculated from the decrease of the high-performance liquid chromatography (HPLC) peak area of the native peptide. HPLC analysis was performed using the Agilent™ 1100 Series equipped with an Agilent analysis column (ZORBAX RX-C8, 2.1 mm × 150 mm, 5 μm) and an UV detector at 220 nm. Mobile phase was composed of A (H2O) and B (acetonitrile), which contained 0.065% trifluoroacetic (v/v) and 0.05% trifluoroacetic (v/v), respectively.
In vitro Anti-biofilm Activity
The activity of RW-BP100-4D against biofilm-formation and mature biofilms in S. aureus ATCC29213 was tested as described previously (Gakhar et al., 2010). For biofilm-formation inhibition assay, aliquots (100 μL) of the bacterial cultures (5 × 105CFU/mL) were added to a 96-well plate and incubated with 100 μL of different concentrations (0.78-100 μg/mL) of RW-BP100-4D or RW-BP100 for 24 h at 37°C, respectively. For the mature biofilms, 100 μL of the bacterial solutions (5 × 105CFU/mL) were cultured in a sterile 96-well plate for 24 h. RW-BP100-4D or RW-BP100 at different concentrations (0.78–200 μg/mL) were added, respectively and incubated at 37°C for 30 min. After different treatments, all the wells were washed with sterilized PBS for three times, fixed with 200 μL of anhydrous methanol for 15 min, and then stained with 150 μL crystal violet (0.1%) for 15 min. The plates were rinsed in water for five times and air dried in room temperature. Lastly, the stained biofilms were completely dissolved in 33% ethanoic acid (100 μL) and the sample absorbance was measured at OD590 nm. Each test was performed with three independent repeats.
Intracellular Antibacterial Activity
Confocal fluorescence assay was performed to investigate the uptaken ability of RW-BP100-4D by mammalian cells. MAC-T cells (104 cells/mL) were cultured in glass-based dishes (Thermo Fisher Scientific) for 24 h, then N-terminal fluorescein isothiocyanate (FITC)-labeled RW-BP100-4D was added at a final concentration of 50 μg/mL and incubated for 2 h. After three times washing with PBS, the cells were fixed with cold methanol for 30 min, and stained with phalloidin and 6-diamidino-2-phenylindole (DAPI) for 30 and 5 min, sequentially. Fluorescence was observed using a Zeiss confocal fluorescence microscope (LSM 800, Carl Zeiss, Oberkochen, Germany). Fluorescence intensity was analyzed with Image J version 1.8.0.
The intracellular antibacterial activity of RW-BP100-4D against S. aureus ATCC29213 and S. enterica ATCC13076 were determined as described previously (John et al., 2019). MAC-T cells were cultured in a 12-well plate and then infected with S. aureus ATCC29213 or S. enterica ATCC13076 (∼105CFU, MOI = 10) for 1 h. The spare bacteria were eliminated by treating with kanamycin (100 μg/mL) for 1 h. After that, kanamycin was removed by washing with PBS for 4 times. Then RW-BP100-4D or RW-BP100 were added to a final concentration of 50 μg/mL, respectively and incubated for 2 h. Meanwhile, kanamycin (50 μg/mL) and PBS were set as the antibiotic treatment group and treatment control group, respectively. Finally, MAC-T cells were treated with 0.1% TritonX-100. The lysates were serially diluted and spread on selective agar plates to enumerate the intracellular live bacteria. Each test was performed with three independent repeats.
Effect on Chicken Meat Disinfection
The raw chicken meat was cut to 1 cm3 square pieces (about 0.25 g), then disinfected by washing with sodium hypochlorite, 75% ethanol, sterilized PBS for three times, and UV radiation for 1 h, sequentially. Bacterial strains of S. aureus ATCC29213, L. monocytogenes ATCC19115, E. coli O157:H7 clinical strain A3, S. enterica ATCC13076, C. jejuni ATCC 33291, and S. putrefaction ATCC 49138 (∼5 × 103 CFU) were evenly spread on the surface of the chicken meat squares, individually or mixed. After bacterial contamination at 4°C for 1 h, the meat pieces were soaked in 1 mL of RW-BP100-4D (50 μg/mL) at 4°C for 24 h. PBS was used as the negative control. Lastly, the chicken pieces were homogenized and centrifuged, then the supernatants were diluted and spread on the corresponding selective medium to enumerate the residual live bacteria.
Treatment Efficacy in Animal Models
The mouse skin infection model and bacteremia model were established to evaluate the treatment efficacy of RW-BP100-4D on local and system infections, respectively. In each model, ICR female mice were equally allocated into different treatment groups and each group contained six mice. The mouse skin infection model and bacteremia model were constructed according to previously described methods (Natalia et al., 2019; Song et al., 2020). The mice were challenged with methicillin-resistant S. aureus ATCC33591 (mecA+, MRSA) via spreading on skin lesion (5 × 105 CFU/mL, 0.1 mL) or intraperitoneal injection (3.0 × 108CFU/mL, 0.5 mL). After infection for 2 h, RW-BP100-4D or RW-BP100 were administrated to the local skin lesion of each mouse at 50 μg (1 mg/mL, 50 μL) for skin infection model, respectively. Ampicillin (1 mg/mL, 100 μL) and PBS were used as the antibiotic treatment control and negative control, respectively. In bacteremia model, peptides (RW-BP100-4D or RW-BP100) were injected intraperitoneally after 2 h of infection at different dose for each mice group (25, 50, and 100 mg/kg, respectively). Ampicillin (100 mg/kg) and PBS were used as the antibiotic treatment control and negative control, respectively. After treatment for 24 h, all the mice were sacrificed to collect the infected wound skin and infected organs (heart, liver, and kidney) from each model. S. aureus isolates were counted by using the Chromagar selective agar (Chromagar, France) supplemented with 8 μg/mL oxacillin.
Statistical Analyses
Statistical analysis was performed using GraphPad Prism software (Version 8.2.1). All data were presented as the mean ± S.D. p values were calculated using an independent two sample t-test for the difference in CFU after different treatments. p-values <0.05 (*) were considered statistically significant.
Results
Antimicrobial Activity
Minimal inhibitory concentration was determined to evaluate the antimicrobial activity of RW-BP100 and its derivatives (Table 1). S. aureus ATCC29213 and E. coli ATCC25922 were chosen as the representative Gram-positive and Gram-negative bacteria, respectively. The results indicated that the MICs of the parental peptide RW-BP100 against S. aureus ATCC29213 and E. coli ATCC25922 were both 12 μg/mL, whereas the derivatives RW-BP100-4D, RW-BP100-5D, RW-BP100-6D, RW-BP100-8D, and RW-BP100-All-D displayed equal or better antibacterial activities (MIC = 3–12 μg/mL). In contrast, the transformation of N terminal (9th–11th) amino acids into corresponding D-enantiomers (RW-BP100-9D, RW-BP100-10D, and RW-BP100-11D) decreased the antimicrobial activity, especially that of RW-BP100-11D (MIC > 50 μg/mL). These results indicated that the 11th amino acid was crucial for the activity of RW-BP100 and could not be replaced.
Among these derivatives, RW-BP100-4D showed the best antimicrobial activity, with the MIC of 3 μg/mL against S. aureus ATCC29213 and 6 μg/mL against E. coli ATCC25922. Subsequently, the antibacterial spectrum of RW-BP100-4D was determined against a more variety of microorganisms compared to that of RW-BP100. As shown in Table 2, the MICs of RW-BP100-4D ranged from 3 to 12 μg/mL against the selected Gram-positive bacterial isolates (S. aureus, S. epidermidis, E. faecalis, S. agalactiae, and L. monocytogenes), and 3–25 μg/mL against the selected Gram-negative bacterial isolates (E. coli, Salmonella enterica, K. pneumoniae, C. jejuni, A. baumannii, Pseudomonas spp., and S. putrefaction), respectively. Besides, RW-BP100-4D was active toward fungal isolates and the MICs against C. albicans and F. oxysporum were both 12.5 μg/mL. Compared to RW-BP100, RW-BP100-4D retained the antibacterial activity against Gram-negative bacteria and fungi, and improved its activity against some Gram-positive bacteria, including both the sensitive and resistant strains. The MBC values of RW-BP100-4D were usually two fold higher than the corresponding MIC values, whereas the MBC values of RW-BP100 were 4–8 fold of the corresponding MIC values, indicating that RW-BP100-4D possessed better antimicrobial activity than its parental peptide RW-BP100, and may display a bactericidal action towards the target pathogens.
Hemolytic Activity and Cytotoxicity
As shown in Table 1, the hemolytic rates of RW-BP100 to sheep blood cells were 3.10 and 4.70% at the concentration of 50 and 100 μg/mL, respectively, which significantly increased to 41.82 and 56.67% at the peptide concentrations of 150 and 300 μg/mL, respectively. The derivatives exhibited low hemolytic rates (0.11–5.99%) at 50–100 μg/mL; however, the hemolytic rates increased substantially (16.17–41.82%) at 150 μg/mL and most of the peptides have hemolysis of >40% at 300 μg/mL. A comparison of hemolytic rates between RW-BP100 and its derivatives at 150–300 μg/mL revealed that both single D-amino acid substitution and All-D-isomers led to reduced hemolytic activity. Especially, single D-amino acid substitution at the 4th, 7th, 9th, 10th, or 11th positions deceased the hemolytic rate to <20% at 150 μg/mL. For cytotoxicity assay, the survival rates of MAC-T and RAW264.7 cells were 57.80 and 36.29%, respectively, when treated with RW-BP100 at 150 μg/mL (Table 1). In contrast, single D-amino acid substitution (4th, 7th, 9th or 10th positions) largely increased the survival rates to >90 and >70% for MAC-T and RAW264.7 cells, respectively (Table 1). Among these derivatives, RW-BP100-7D showed the lowest cytotoxicity, with the survival rates of MAC-T and RAW264.7 cells (at peptide concentration of 150 μg/mL) reaching 96.41 and 93.74%, respectively. However, the antimicrobial activity of RW-BP100-7D was much weaker than that of RW-BP100-4D, which also possessed relatively lower cytotoxicity among these derivatives (Tables 1, 2 and Supplementary Figure 1). Taken together, RW-BP100-4D was selected for further stability and activity evaluation.
Thermal, pH, Protease, and Serum Stability
The stability of RW-BP100-4D was evaluated under different temperature, pH, and protease condition (Supplementary Table 1). After incubation at temperatures from 40 to 80°C, the MICs of RW-BP100-4D against E. coli ATCC25922, S. aureus ATCC29213, and C. albicans ATCC10231 remained unchanged (6, 3 and 12 μg/mL, respectively) in all three repeats. However, incubation at 90 and 100°C caused 2–8 fold increase of RW-BP100-4D MICs against the corresponding reference strains. HPLC determination revealed that 56 and 82% of the original peptide were disrupted at 90 and 100°C, respectively (Supplementary Table 1). The results indicated that RW-BP100-4D maintained its activity at the temperature below 80°C, which is consistent with the previous study showing that AMPs were more tolerant to high temperatures (Tanhaeian et al., 2020). With respect to pH sensitivity, the MICs of RW-BP100-4D against the three reference strains remained stable at the pH ranges of 2–7, but increased 2–4 fold at the pHs of 8 and 9. This result indicated that RW-BP100-4D was more tolerant to the acidic environment than alkaline conditions, which might be due to the disruption of peptide bonds at higher pHs (Jones et al., 2015). Treatment with proteinase K increased the MICs of RW-BP100-4D against the corresponding strains to 4–8 fold of the original level. Likewise, treatment with papain, trypsin, or calf serum significantly reduced the antimicrobial activity (MIC > 50 μg/mL and >95% degradation). These results indicated that RW-BP100-4D was vulnerable to proteases and may not be stable in the serum.
Anti-biofilm Activity in vitro
As shown in Figure 2A, RW-BP100-4D effectively inhibited the biofilm formation of S. aureus ATCC29213 and the inhibition ability was significantly enhanced as the concentration of RW-BP100-4D increased from 0.78 to 100 μg/mL compared to the PBS control (p < 0.05). In contrast, RW-BP100 also inhibited the biofilm formation of S. aureus ATCC29213 and the inhibition ability of RW-BP100 was weaker than that of RW-BP100-4D (Figure 2A). Compared with the PBS control, 82.3 and 45.8% of the biofilm in S. aureus was inhibited by RW-BP100-4D and RW-BP100 at 6.25 μg/mL, respectively. Although the anti-biofilm activity was concentration-dependent, the activity was slowly increased when RW-BP100-4D and RW-BP100 concentrations were above 12.5 and 50 μg/mL, respectively, which may be due to that the peptides at concentrations near the MBC of the target strain killed most of the bacteria. For mature biofilms, treatment with lower concentrations (≤4 × MIC) of RW-BP100-4D led to a slight loss in biomass (Figure 2B). However, higher concentrations (50–200 μg/mL) slightly improved the anti-biofilm activity and about 40.4% of the biofilm matrix was lost after treatment with 200 μg/mL of RW-BP100-4D compared to the PBS control (p < 0.01). In contrast, RW-BP100 showed a weaker anti-biofilm activity than that of RW-BP100-4D and 29.4% of the biofilm matrix was lost after treatment with 200 μg/mL of RW-BP100.
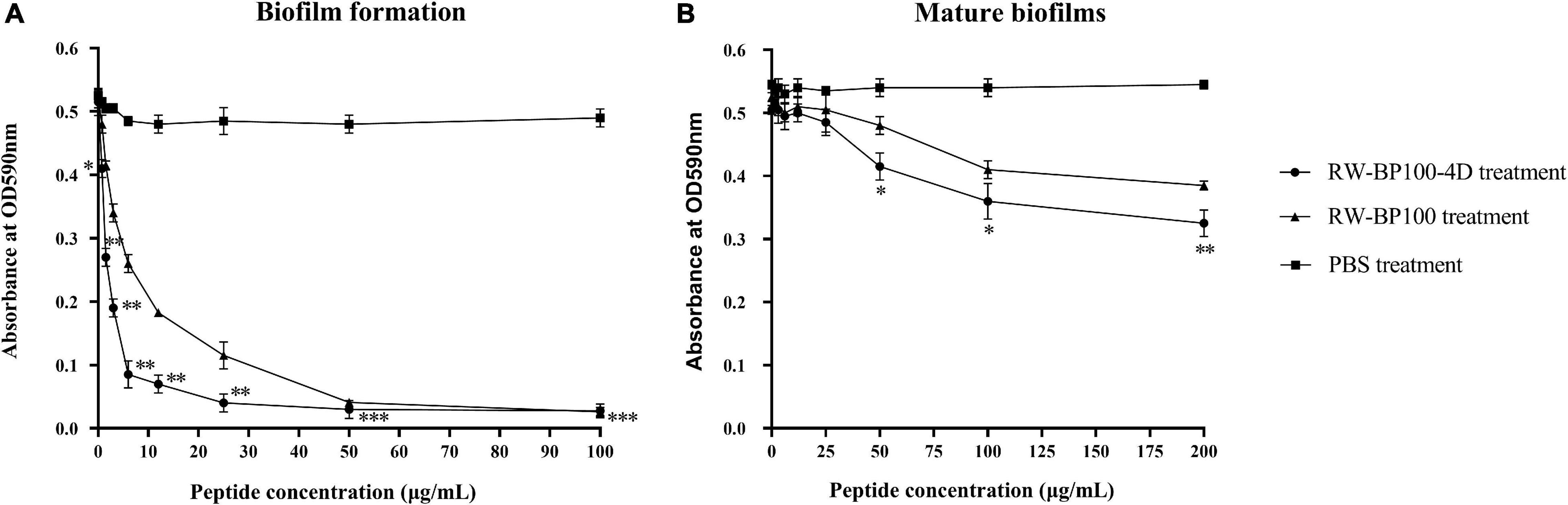
Figure 2. Anti-biofilm activity of RW-BP100-4D against S. aureus ATCC29213. (A) Activity of RW-BP100-4D against the biofilm-formation of S. aureus ATCC29213 (B) Activity of RW-BP100-4D against the mature biofilm of S. aureus ATCC29213. Data are the mean ± SD of triplicate wells from three independent experiments. p values were calculated using an independent two sample t-test for the difference in absorbance at OD590nm between RW-BP100-4D-treated group and the PBS control, *p < 0.5, **p < 0.01, ***p < 0.001.
Antibacterial Activity in the MAC-T Cells
The confocal fluorescence images in Figure 3 showed that the FITC-labeled RW-BP100-4D at 50 μg/mL was rapidly (≤2 h) and efficiently uptaken by the MAC-T cells and evenly distributed throughout the whole cytoplasm. To further investigate the intracellular antibacterial activity of RW-BP100-4D, S. aureus ATCC29213 and S. enterica ATCC13076 were used as the target pathogens. It showed that both S. aureus and S. enterica at an initial dose of 5 × 105 CFU could infect and colonize in the MAC-T cells at CFU of 3.55 × 103 and 6.92 × 103, respectively (Figure 4). Notably, RW-BP100-4D at 50 μg/mL could significantly eliminate the intracellular S. aureus ATCC29213 by 3.42 log and S. enterica ATCC13076 by 3.81 log, compared with that of kanamycin treatments (p < 0.0001). In contrast, RW-BP100 at 50 μg/mL killed the intracellular S. aureus ATCC29213 by 2.34 log and S. enterica ATCC13076 by 2.57 log. RW-BP100-4D showed better intracellular antibacterial activity than that of RW-BP100 (p < 0.01) and there was no significant difference between the CFU of kanamycin treatment group and the untreated group (Figure 4).
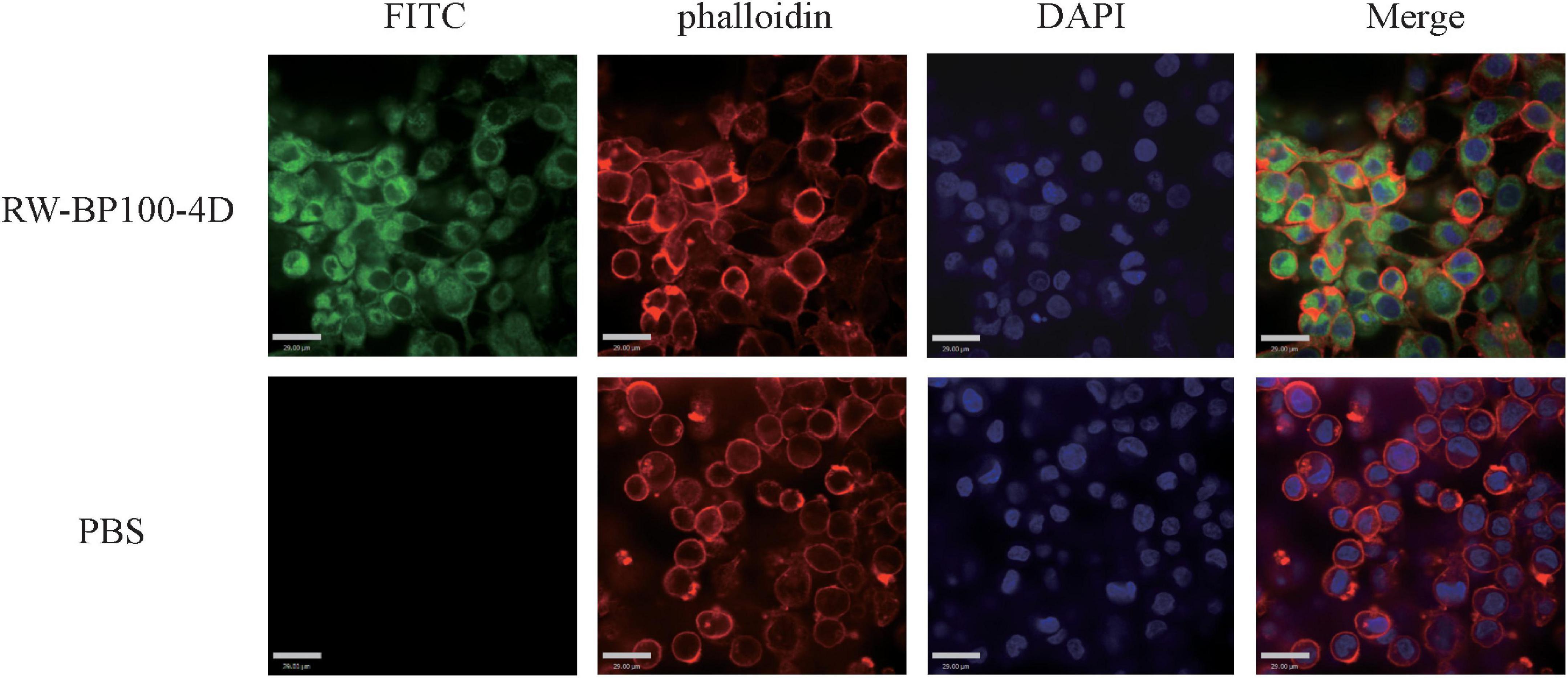
Figure 3. Confocal fluorescence images showing distribution of RW-BP100-4D in MAC-T cells. The up panel indicates the RW-BP100-4D treated group. The down panel indicates the PBS treated control group. Green color indicates FITC-labeled peptide RW-BP100-4D, red indicates the phalloidin-stained MAC-T cell membrane and blue indicates the DAPI-stained cell nucleus. The scale bar is 29.00 μm. FITC, Fluorescein isothiocyanate; DAPI, 6-diamidino-2-phenylindole.
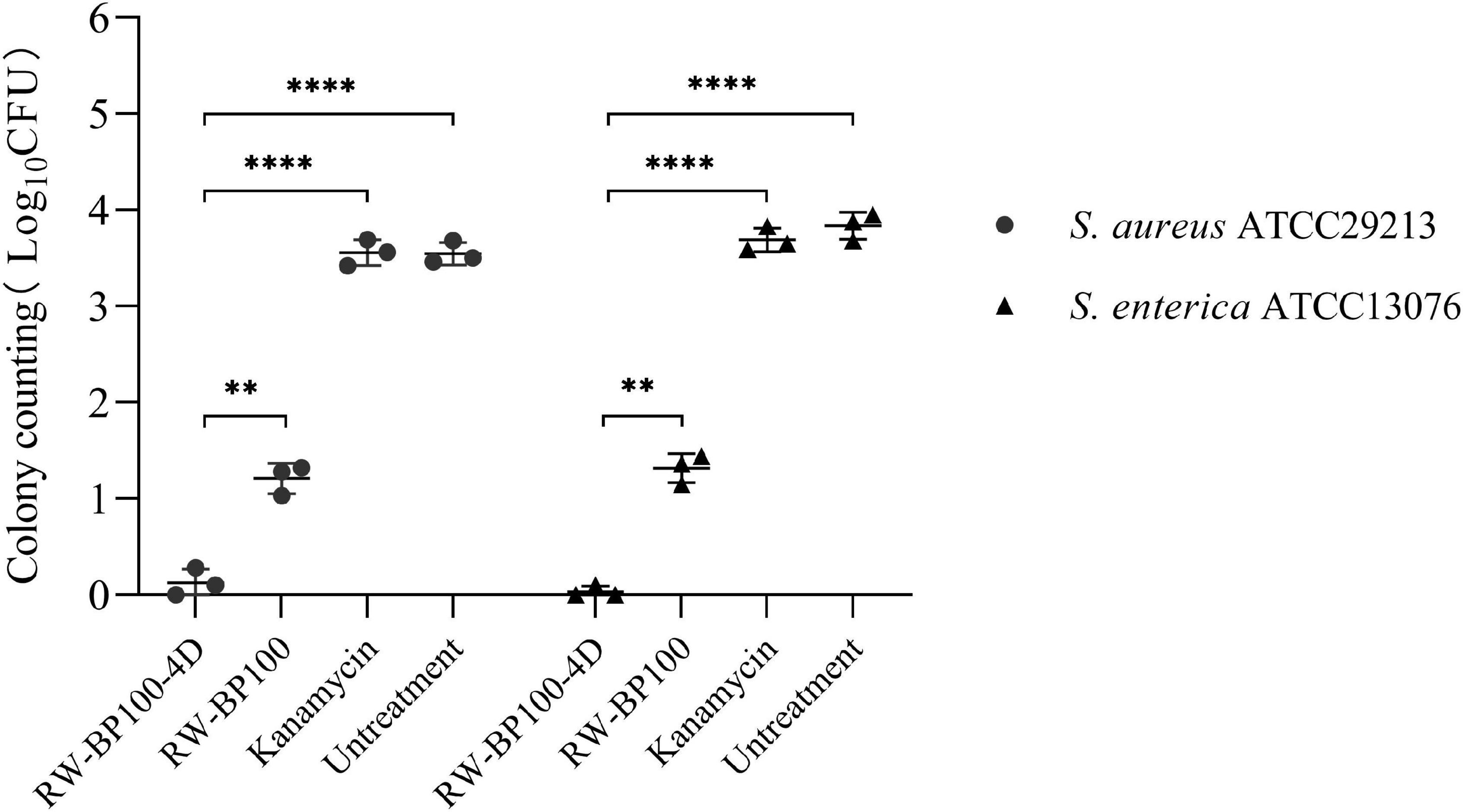
Figure 4. Intracellular antibacterial activities of RW-BP100-4D against S. aureus ATCC29213 and S. enterica ATCC13076 within the MAC-T cells. Kanamycin and PBS were used as the antibiotic-treatment and untreated controls, respectively. Data are the mean ± SD of triplicates from three independent experiments. p values were calculated using an independent two sample t-test for the difference in CFU after different treatments, **p < 0.01, ****p < 0.0001.
Disinfection Effect of RW-BP100-4D on Chicken Meat
The potential application of RW-BP100-4D to disinfect food was tested by using chicken meat manually contaminated by diverse bacteria. As shown in Figure 5, RW-BP100-4D effectively reduced the bacterial loads on chicken meat, including S. aureus by 3.09 ± 0.035 log (99.92% killing), L. monocytogenes by 2.96 ± 0.028 log (99.89% killing), E. coli O157: H7 by 3.43 ± 0.021 log (99.96% killing), C. jejuni by 1.24 ± 0.017 log (94.18% killing), S. enteritidis by 3.60 ± 0.021 log (99.97% killing) and S. putrefaciens by 2.95 ± 0.024 log (99.89% killing), respectively. There were significant differences between the CFU of RW-BP100-4D treatment group and the corresponding controls (p < 0.001) for each bacterial strain. Interestingly, RW-BP100-4D could also reduce the bacterial loads of multiple contamination (concurrently contaminated by S. aureus, L. monocytogenes, E. coli O157: H7, C. jejuni, S. enterica, and S. putrefaciens) by 3.61 ± 0.063 log in total, indicating the potential effectiveness of RW-BP100-4D in food disinfection.
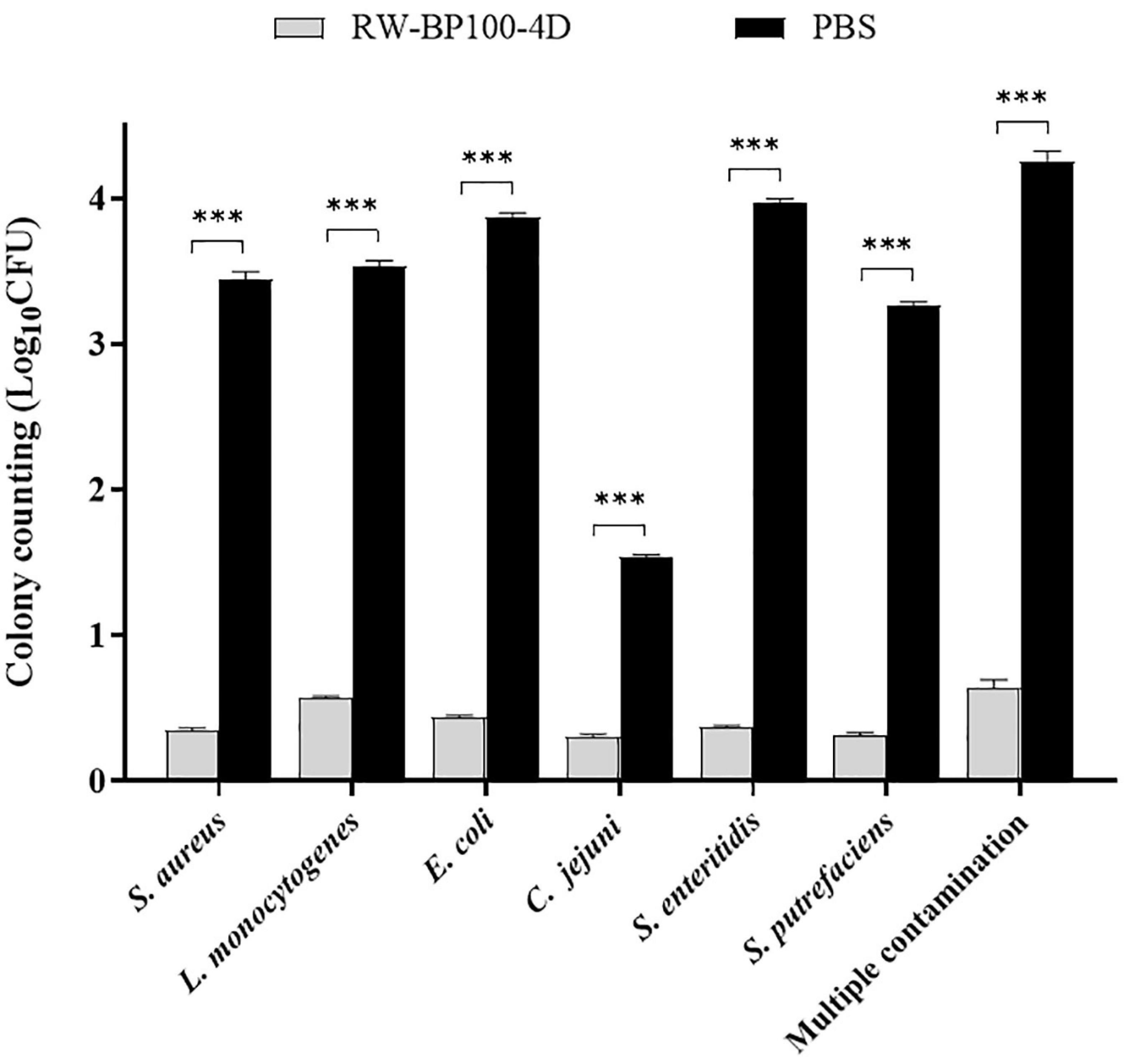
Figure 5. Effect of RW-BP100-4D on chicken meat disinfection contaminated with various bacterial species. Multiple contamination indicates the chicken meat concurrently contaminated by S. aureus, L. monocytogenes, E. coli O157: H7, C. jejuni, S. enterica, and S. putrefaciens. Data are the mean ± SD from three independent experiments. Independent two sample t-test was used to compare the difference of CFU between RW-BP100-4D-treatment group and PBS control group, ***p < 0.001.
Treatment Efficacy in Mouse Skin Infection and Bacteremia Models
The mecA-positive S. aureus (MRSA) ATCC33591 was used to establish the mouse skin infection model. In the control group, the bacterial load in the infected mice skin reached 4.12 × 107 CFU after 24 h (Figure 6A). RW-BP100-4D reduced S. aureus ATCC33591 by >4 log orders of magnitude (99.99% killing) over a 24 h period, while ampicillin did not decrease the bacteria load. Statistical analysis revealed that there was a significant difference of CFU in the infection sites between RW-BP100-4D treatment group and ampicillin treatment group (p < 0.0001), whereas no significant difference was found between the ampicillin treatment group and the untreatment controls. Although RW-BP100 also showed good efficacy in reducing bacterial load (3.13 log reduction) in the infected mice skin, there was a significant difference between CFU of RW-BP100-4D and RW-BP100 treatment group (p < 0.001, Figure 6A). For bacteremia model, the bacterial loads in the mouse’s heart, liver and kidney in the control group reached 3.55 × 102, 5.87 × 102 and 5.67 × 102 CFU, respectively. RW-BP100-4D administrated at 100 mg/kg and RW-BP100 at 50 or 100 mg/kg caused the death of >80% mice. Therefore, the bacterial loads were only counted in mice groups that were treated with the other concentrations. It revealed that RW-BP100 or RW-BP100-4D administrated at 25 mg/kg did not significantly reduce the bacterial loads in these organs. However, RW-BP100-4D administrated at 50 mg/kg significantly reduced the bacterial loads in the mouse’s heart and liver (p < 0.5), indicating a modest treatment efficacy of RW-BP100-4D in mouse systemic infections (Figure 6B).
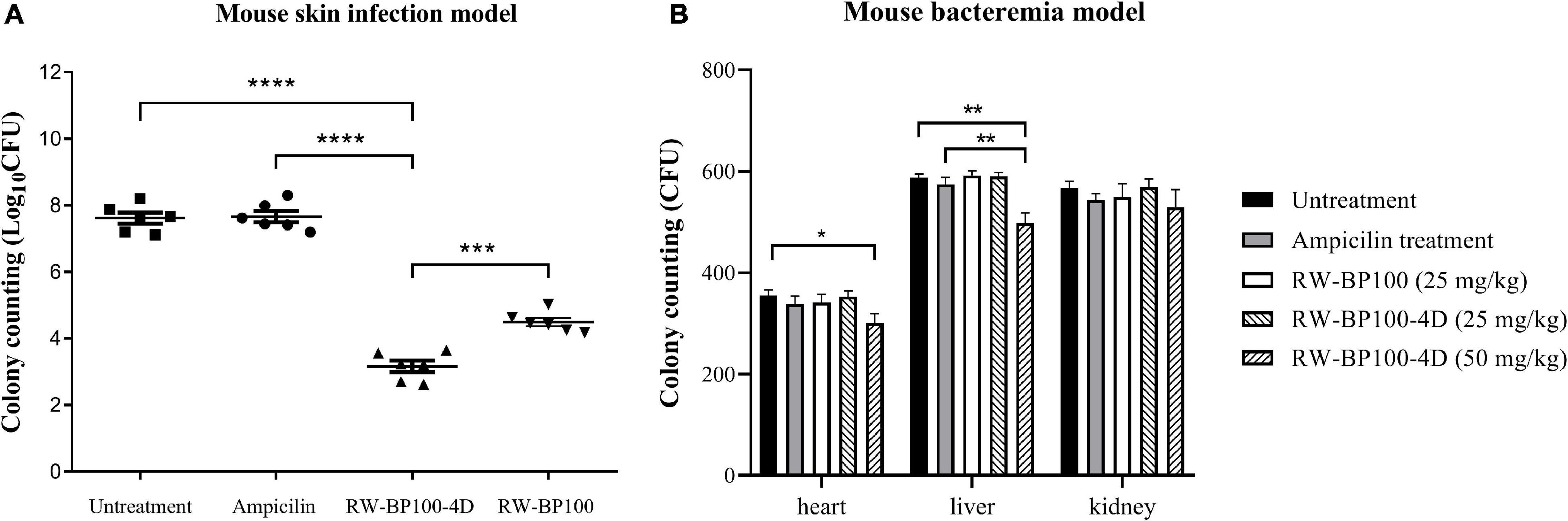
Figure 6. Treatment efficacy of RW-BP100-4D against S. aureus ATCC33591 in (A) mouse skin infection model and (B) mouse bacteremia model. Ampicilin and PBS were used as the antibiotic-treatment and untreatment controls, respectively. Data are the mean ± SD from three independent experiments. Independent two sample t-test was used to compare the difference of CFU between different treatment groups, *p < 0.5, **p < 0.01, ***p < 0.001, ****p < 0.0001.
Discussion
In this study, we designed a series of derivatives from RW-BP100 and screened out the ideal candidate RW-BP100-4D, which showed relatively low cytotoxicity and the best antimicrobial activity. Generally, the activity of AMP is infuenced by many factors, such as peptide length, net charge, hydrophobicity and secondary structure (Zhang et al., 2021). Since single D-amino acid transformation does not alter the length and net charge of the parental peptide, the enhanced antimicrobial activity of RW-BP100-4D in this study may be largely due to the variation of peptide hydrophobicity or secondary structure, thus leading to a stronger binding affinity with the anionic components on the microbial membrane, such as LPS of Gram-negative bacteria, lipoteichoic acid of Gram-positive bacteria and mannan of fungi (Zhang et al., 2021). Notably, the antimicrobial activity of RW-BP100-4D against antibiotic-susceptible and resistant strains were very similar, such as methicillin-sensitive S. aureus ATCC29213 versus methicillin-resistant S. aureus ATCC33591 (both MICs were 6 μg/mL), and antibiotic-sensitive E.coli ATCC25922 (MIC = 6 μg/mL) versus tigecycline-resistant E. coli 47EC (MIC = 3 μg/mL), indicating that the antimicrobial activity of RW-BP100-4D was indiscriminately and not affected by the resistance genes carried by the bacteria, which may be due to the special bactericidal mechanisms of the peptide (Toke, 2005).
From the hemolytic activity and cytotoxicity assays, we could see that the derivatives with single D-amino acid substitution at the 4th, 7th, 9th, 10th or 11th positions showed decreased cytotoxicity compared to the parental peptide RW-BP100. These results were consistent with the previous study, which showed that subtle changes in a peptide influenced not only the antimicrobial activity but also the hemolytic activity (Imma et al., 2011). Other studies have reported excellent in vitro antimicrobial activity profiles of several D-enantiomer peptides. However, the high production costs imposed severe limitations to their application as antimicrobial products (McGrath et al., 2013; Chen et al., 2016). Considering the short length of only 11 amino acids and just one D-amino acid replacement, which could largely reduce the cost of peptide synthesis, RW-BP100-4D can be taken as a promising candidate for further development in antimicrobial application.
As more than 95% of the bacteria in nature are existing in biofilms, which could help them resistant to antibiotics and the host immune system, and played an important role in the persistence of chronic infections (Imma et al., 2011; Kharidia and Liang, 2011), it is of great significance to eradicate the bacterial biofilms in the clinical treatment. Findings in this study indicated that RW-BP100-4D showed better anti-biofilm activity in vitro than its parental peptide RW-BP100. RW-BP100-4D showed a dose-dependent anti-biofilm activity and the biofilm-formation in S. aureus was completely inhibited when peptide concentrations were kept at a lower concentration (≥1 × MIC). It is well known that mature biofilms are more common and complicated in human clinical treatment, especially the implants associated infections (Costerton et al., 2007). Interestingly, RW-BP100-4D also showed modest dispersal activity against mature biofilms of S. aureus when applied with a higher dose (>8 × MIC). Despite RW-BP100-4D displayed good biofilm-inhibition ability in vitro against S. aureus, further studies are still needed to prove its in vivo anti-biofilm activity as some AMPs may have their activity nullified in the presence of tryptone, proteins, or high concentration of salts in the body fluid (Beckloff et al., 2007).
Previous studies have shown that some short cationic peptides could be used as cell-penetrating carrier to transport functional cargoes (such as DNA, proteins, and chemical agents) into the target cells (Mazel et al., 2001; Perea et al., 2004). As RW-BP100-4D has an amphiphilic structure that may easily penetrate through the hydrophobic lipid bilayer, this short peptide is tested for its cell-penetrating ability. The results revealed that RW-BP100-4D could efficiently penetrate into the MAC-T cells and concurrently kill the S. aureus and S. enterica isolates colonized within the cells. Intracellular bacteria, including opportunistic pathogens (such as S. aureus and Salmonella spp.) and obligate parasites (e.g., mycobacterium tuberculosis and Brucella spp.), posed a great challenge to clinical treatment since these microorganisms could colonize within the cells and thus evade the killing of some clinically-important antibiotics such as β-lactams and aminoglycosides (Paul and AndrÉ, 1978; Petra and Matthias, 2005). Although RW-BP100 also showed good intracellular bacterial-killing ability, the cytotoxic concentration of RW-BP100 was much higher than that of RW-BP100-4D. Therefore, the modified peptide has a higher therapeutic index and may be more appropriate to be developed in treating the intracellular bacterial infections or as a cell-penetrating tool to deliver the functional molecules in the future.
Staphylococcus aureus, L. monocytogenes, E. coli O157: H7, C. jejuni, and Salmonella spp. were the main foodborne pathogens commonly identified in food poisoning incidences (Kirk et al., 2015), and S. putrefaciens was one of the dominated pathogens that led to food spoilage of chilled meat (Lan et al., 2019). The present study showed that RW-BP100-4D was active against the above-mentioned pathogens contaminated on chicken meat, either by single microorganism or by multiple pathogens. Previous study has reported that Nisin, which is a natural antimicrobial peptide and has been commercialized as a food preservative, is active against many foodborne pathogens such as L. monocytogenes and Clostridium botulinumon (Gharsallaoui et al., 2016). However, the antimicrobial spectrum of Nisin is limited to the Gram-positive bacteria, and Gram-negative bacteria are usually resistant to nisin mainly due to their impermeable outer membranes (Gharsallaoui et al., 2016). Therefore, RW-BP100-4D may have the advantages in food disinfection as it could reduce the transmission risk of foodborne pathogens, including both Gram-postitive and Gram-negative bacteria. In addition to food disinfection, RW-BP100-4D also displayed good efficacy in treating MRSA infection in mouse local skin. However, the in vivo treatment efficacy of RW-BP100-4D toward MRSA was modest in mouse bacteremia model, which may be due to its degradation by proteolytic enzymes in the animal serum or tissues. Moreover, a higher dose (100 mg/kg) of RW-BP100-4D led to the death of most treated mice, indicating that the candidate peptide is toxic to the animals when applied in vivo with higher doses. Development of AMP-based application has encountered many challenges including stability, efficacy and toxicity (Zhang et al., 2021). As indicated in this study, RW-BP100-4D showed generally good bactericidal effect in vitro, including the removal of food bacteria and treatment of local skin infection, which has a potential to be applied as a food bactericide and local external use. However, the toxicity of RW-BP100-4D should not be ignored (hemolysis at higher concentrations) when the candidate peptide is to be developed on these applications. Moreover, both toxicity and protease-sensitivity of RW-BP100-4D are unavoidable problems when used in vivo, which will limit the development of the peptide in clinical therapy, including the biofilm removal and systemic infection treatment. Therefore, future research works are still needed to further reduce the toxicity and improve the therapeutic effect of RW-BP100-4D in vivo.
Conclusion
This study presented a broad-spectrum AMP designated RW-BP100-4D, which was derived from RW-BP100 by transforming the N-terminal-4th amino acid from L- to D-enantiomer. Compared to its parent analogue, RW-BP100-4D showed remarkably reduced cytotoxicity and enhanced antimicrobial activity, including both Gram-positive and Gram-negative bacteria as well as the fungi. Moreover, RW-BP100-4D displayed good bactericidal effect in the removal of food bacteria and local skin infection. However, the protease-sensitivity of RW-BP100-4D and toxic effect at higher doses reduced the therapeutic effect of the candidate peptide in vivo and should be managed in the future studies.
Data Availability Statement
The original contributions presented in the study are included in the article/Supplementary Material, further inquiries can be directed to the corresponding authors.
Ethics Statement
The animal study was reviewed and approved by Committee on Animal Welfare and Experimental Ethics in Jiangsu Academy of Agricultural Sciences (approval no. JAAS20200516 and JAAS20211208).
Author Contributions
RW and TH designed the study. TH, XT, JL, RCW, and LG conducted all the experiments. TH, JL, and XJ analyzed and interpreted the data. TH, XT, and JL wrote the manuscript. All authors reviewed, revised, and approved the final manuscript.
Funding
This study was supported by grants from Natural Science Foundation of Jiangsu Province (BK20200056 and BK20190261), National Natural Science Foundation of China (31930110 and 32102728), Jiangsu Agricultural Science and Technology Foundation [CX(20)3088], and Exploration and Disruptive Innovation Projects of Jiangsu Academy of Agricultural Sciences [ZX(21)1224].
Conflict of Interest
The authors declare that the research was conducted in the absence of any commercial or financial relationships that could be construed as a potential conflict of interest.
Publisher’s Note
All claims expressed in this article are solely those of the authors and do not necessarily represent those of their affiliated organizations, or those of the publisher, the editors and the reviewers. Any product that may be evaluated in this article, or claim that may be made by its manufacturer, is not guaranteed or endorsed by the publisher.
Supplementary Material
The Supplementary Material for this article can be found online at: https://www.frontiersin.org/articles/10.3389/fmicb.2021.815980/full#supplementary-material
References
Bechinger, B., and Gorr, S. U. (2017). Antimicrobial peptides: mechanisms of action and resistance. J. Dent. Res. 96, 254–260. doi: 10.1177/0022034516679973
Beckloff, N., Laube, D., Castro, T., Furgang, D., Park, S., Perlin, D., et al. (2007). Activity of an antimicrobial peptide mimetic against planktonic and biofilm cultures of oral pathogens. Antimicrob. Agents Chemother. 51, 4125–4132. doi: 10.1128/AAC.00208-07
Brogden, K. A. (2005). Antimicrobial peptides: pore formers or metabolic inhibitors in bacteria? Nat. Rev. Microbiol. 3, 238–250. doi: 10.1038/nrmicro1098
Chen, H. L., Su, P. Y., and Shih, C. (2016). Improvement of in vivo antimicrobial activity of HBcARD peptides by D-arginine replacement. Appl. Microbiol. Biotechnol. 100, 9125–9132. doi: 10.1007/s00253-016-7621-6
CLSI (2015). Performance standards for antimicrobial susceptibility testing. CLSI document M100-S25. Wayne, PA: CLSI.
Costerton, J. W., Montanaro, L., and Arciola, C. R. (2007). Bacterial communications in implant infections: A target for an intelligence war. Int. J. Artif. Organs. 30, 757–763. doi: 10.1177/039139880703000903
Ferre, R., Melo, M. N., Correia, A. D., Feliu, L., Bardaji, E., Planas, M., et al. (2009). Synergistic effects of the membrane actions of cecropin-melittin antimicrobial hybrid peptide BP100. Biophys. J. 96, 1815–1827. doi: 10.1016/j.bpj.2008.11.053
Gakhar, L., Bartlett, J. A., Penterman, J., Mizrachi, D., Singh, P. K., Mallampalli, R. K., et al. (2010). PLUNC is a novel airway surfactant protein with anti-biofilm activity. PLoS One 5:e9098. doi: 10.1371/journal.pone.0009098
Gharsallaoui, A., Oulahal, N., Joly, C., and Degraeve, P. (2016). Nisin as a food preservative: Part 1: physicochemical properties, antimicrobial activity, and main uses. Crit. Rev. Food Sci. Nutr. 56, 1262–1274. doi: 10.1080/10408398.2013.763765
Imma, G., Jordi, C., Esther, B., Rafael, F., Montserrat, T., Eduard, B., et al. (2011). Improvement of the efficacy of linear undecapeptides against plant-pathogenic bacteria by incorporation of D-amino acids. Appl. Environ. Microbiol. 77, 2667–2675. doi: 10.1128/AEM.02759-10
John, C. M., Li, M., Feng, D., and Jarvis, G. A. (2019). Cationic cell-penetrating peptide is bactericidal against Neisseria gonorrhoeae. J. Antimicrob. Chemother. 74, 3245–3251. doi: 10.1093/jac/dkz339
Jones, E. M., Cochrane, C. A., and Percival, S. L. (2015). The effect of pH on the extracellular matrix and biofilms. Adv. Wound Care 4, 431–439. doi: 10.1089/wound.2014.0538
Kai, W., Xu, X., Pu, X., Hou, Z., and Zhang, Q. (2011). Cytotoxic effects and the mechanism of three types of magnetic nanoparticles on human hepatoma BEL-7402 cells. Nanoscale Res. Lett. 6:480. doi: 10.1186/1556-276X-6-480
Kharidia, R., and Liang, J. F. (2011). The activity of a small lytic peptide PTP-7 on Staphylococcus aureus biofilms. J. Microbiol. 49, 663–668. doi: 10.1007/s12275-011-1013-5
Kirk, M. D., Pires, S. M., Black, R. E., Caipo, M., Crump, J. A., Devleesschauwer, B., et al. (2015). World Health Organization estimates of the global and regional disease burden of 22 foodborne bacterial, protozoal, and viral diseases, 2010: A data synthesis. PLoS Med. 12:e1001921. doi: 10.1371/journal.pmed.1001921
Lan, W., Zhang, N., Liu, S., Chen, M., and Xie, J. (2019). epsilon-Polylysine inhibits Shewanella putrefaciens with membrane disruption and cell damage. Molecules 24:3727. doi: 10.3390/molecules24203727
Li, Q., Cebrian, R., Montalban-Lopez, M., Ren, H., Wu, W., and Kuipers, O. P. (2021). Outer-membrane-acting peptides and lipid II-targeting antibiotics cooperatively kill Gram-negative pathogens. Commun. Biol. 4:31. doi: 10.1038/s42003-020-01511-1
Mangoni, M. L., and Shai, Y. (2009). Temporins and their synergism against Gram-negative bacteria and in lipopolysaccharide detoxification. Biochim. Biophys. Acta. 1788, 1610–1619. doi: 10.1016/j.bbamem.2009.04.021
Mangoni, M. L., and Shai, Y. (2011). Short native antimicrobial peptides and engineered ultrashort lipopeptides: similarities and differences in cell specificities and modes of action. Cell Mol. Life Sci. 68, 2267–2280. doi: 10.1007/s00018-011-0718-2
Mazel, M., Clair, P., Rousselle, C., Vidal, P., Scherrmann, J.-M., Mathieu, D., et al. (2001). Doxorubicin-peptide conjugates overcome multidrug resistance. Anti Cancer Drugs 12, 107–116. doi: 10.1097/00001813-200102000-00003
McGrath, D. M., Barbu, E. M., Driessen, W. H., Lasco, T. M., Tarrand, J. J., Okhuysen, P. C., et al. (2013). Mechanism of action and initial evaluation of a membrane active all-D-enantiomer antimicrobial peptidomimetic. Proc. Natl. Acad. Sci. U S A. 110, 3477–3482. doi: 10.1073/pnas.1221924110
Montesinos, E. (2007). Antimicrobial peptides and plant disease control. FEMS Microbiol. Lett. 270, 1–11. doi: 10.1111/j.1574-6968.2007.00683.x
Natalia, M., Kobayashi, S. D., Jamie, L., and DeLeo, F. R. (2019). Mouse model of Staphylococcus aureus skin infection. Methods Mol. Biol. 1960, 139–147. doi: 10.1007/978-1-4939-9167-9_12
Pasupuleti, M., Schmidtchen, A., and Malmsten, M. (2012). Antimicrobial peptides: key components of the innate immune system. Crit. Rev. Biotechnol. 32, 143–171. doi: 10.3109/07388551.2011.594423
Paul, T., and AndrÉ, T. (1978). The uptake and intracellular accumulation of aminoglycoside antibiotics in lysosomes of cultured rat fibroblasts. Biochem. Pharmacol. 27, 415–424. doi: 10.1016/0006-2952(78)90370-2
Perea, S. E., Reyes, O., Puchades, Y., Mendoza, O., Vispo, N. S., Torrens, I., et al. (2004). Antitumor effect of a novel proapoptotic peptide that impairs the phosphorylation by the protein kinase 2 (casein kinase 2). Cancer Res. 64, 7127–7129. doi: 10.1158/0008-5472.CAN-04-2086
Petra, L., and Matthias, B. (2005). Interaction of 31 beta-lactam antibiotics with the H+/peptide symporter PEPT2: analysis of affinity constants and comparison with PEPT1. Eur. J. Pharm. Biopharm. 59, 17–24. doi: 10.1016/j.ejpb.2004.07.008
Raaijmakers, J. M., de Bruijn, I., and de Kock, M. J. (2006). Cyclic lipopeptide production by plant-associated Pseudomonas spp.: diversity, activity, biosynthesis, and regulation. Mol. Plant Microbe Interact. 19, 699–710. doi: 10.1094/MPMI-19-0699
Song, M., Liu, Y., Huang, X., Ding, S., Wang, Y., Shen, J., et al. (2020). A broad-spectrum antibiotic adjuvant reverses multidrug-resistant Gram-negative pathogens. Nat. Microbiol. 5, 1040–1050. doi: 10.1038/s41564-020-0723-z
Tanhaeian, A., Mohammadi, E., Mansury, D., and Zeinali, T. (2020). Assessment of a novel antimicrobial peptide against clinically isolated animal pathogens and prediction of its thermal-stability. Microb. Drug Resist. 26, 412–419. doi: 10.1089/mdr.2019.0062
Toke, O. (2005). Antimicrobial peptides: new candidates in the fight against bacterial infections. Biopolymers 80, 717–735. doi: 10.1002/bip.20286
Torcato, I. M., Huang, Y.-H., Franquelim, H. G., Gaspar, D., Craik, D. J., Castanho, M. A. R. B., et al. (2013). Design and characterization of novel antimicrobial peptides, R-BP100 and RW-BP100, with activity against Gram-negative and Gram-positive bacteria. Biochim. Biophys. Acta 1828, 944–955. doi: 10.1016/j.bbamem.2012.12.002
Yeaman, M. R., and Yount, N. Y. (2003). Mechanisms of antimicrobial peptide action and resistance. Pharmacol. Rev. 55, 27–55. doi: 10.1124/pr.55.1.2
Zamora-Carreras, H., Strandberg, E., Mühlhäuser, P., Bürck, J., Wadhwani, P., Jiménez, M. Á, et al. (2016). Alanine scan and (2)H NMR analysis of the membrane-active peptide BP100 point to a distinct carpet mechanism of action. Biochim. Biophys. Acta 1858, 1328–1338. doi: 10.1016/j.bbamem.2016.03.014
Zasloff, M. (2002). Antimicrobial peptides of multicellular organisms. Nature 415, 389–395. doi: 10.1038/415389a
Keywords: antimicrobial peptide, anti-biofilm, intracellular antibacterial activity, food disinfection, treatment efficacy
Citation: Tong X, Li J, Wei R, Gong L, Ji X, He T and Wang R (2022) RW-BP100-4D, a Promising Antimicrobial Candidate With Broad-Spectrum Bactericidal Activity. Front. Microbiol. 12:815980. doi: 10.3389/fmicb.2021.815980
Received: 16 November 2021; Accepted: 31 December 2021;
Published: 25 January 2022.
Edited by:
Josep M. Sierra, University of Barcelona, SpainReviewed by:
Joanna Wessely-Szponder, University of Life Sciences of Lublin, PolandJianhua Wang, Gene Engineering Laboratory, Feed Research Institute, Chinese Academy of Agricultural Sciences (CAAS), China
Copyright © 2022 Tong, Li, Wei, Gong, Ji, He and Wang. This is an open-access article distributed under the terms of the Creative Commons Attribution License (CC BY). The use, distribution or reproduction in other forums is permitted, provided the original author(s) and the copyright owner(s) are credited and that the original publication in this journal is cited, in accordance with accepted academic practice. No use, distribution or reproduction is permitted which does not comply with these terms.
*Correspondence: Tao He, dmV0aGV0YW9AMTYzLmNvbQ==; Ran Wang, cmFud2FuZ0BqYWFzLmFjLmNu
†These authors have contributed equally to this work and share first authorship