- 1Metabolomics Research Center for Functional Materials, Kyungsung University, Busan, South Korea
- 2Department of Bio-Safety, Kyungsung University, Busan, South Korea
Thanatin is an antimicrobial peptide (AMP) generated by insects for defense against bacterial infections. In the present study, we performed cDNA cloning of thanatin and found the presence of multiple precursor proteins from the bean bug, Riptortus pedestris. The cDNA sequences encoded 38 precursor proteins, generating 13 thanatin isoforms. In the phylogenetic analysis, thanatin isoforms were categorized into two groups based on the presence of the membrane attack complex/perforin (MACPF) domain. In insect-bacterial symbiosis, specific substances are produced by the immune system of the host insect and are known to modulate the symbiont’s population. Therefore, to determine the biological function of thanatin isoforms in symbiosis, the expression levels of three AMP genes were compared between aposymbiotic insects and symbiotic R. pedestris. The expression levels of the thanatin genes were significantly increased in the M4 crypt, a symbiotic organ, of symbiotic insects upon systemic bacterial injection. Further, synthetic thanatin isoforms exhibited antibacterial activity against gut-colonized Burkholderia symbionts rather than in vitro-cultured Burkholderia cells. Interestingly, the suppression of thanatin genes significantly increased the population of Burkholderia gut symbionts in the M4 crypt under systemic Escherichia coli K12 injection. Overgrown Burkholderia gut symbionts were observed in the hemolymph of host insects and exhibited insecticidal activity. Taken together, these results suggest that thanatin of R. pedestris is a host-derived symbiotic factor and an AMP that controls the population of gut-colonized Burkholderia symbionts.
Introduction
The bean bug, Riptortus pedestris (Hemiptera: Alydidae), possesses a specialized symbiotic organ in the posterior midgut region (named M4 crypt), where numerous crypts harbor specific gut symbionts, Burkholderia insecticola, recently reassigned to the genus Caballeronia (Kikuchi et al., 2005, 2007, 2011). These symbionts are orally acquired by R. pedestris nymphs from the environment of every generation, and are easily cultivated and genetically manipulated (Kim et al., 2013b,c, 2014, 2016b,2017; Lee et al., 2015). Based on these physiological characteristics, the Riptortus-Burkholderia symbiosis system has been recognized as a promising experimental model for studying insect-microbe interaction at the molecular and biochemical levels (Kim et al., 2016a). Notably, this model has several experimental advantages for studying insect symbiosis. By controlling the oral infection of Burkholderia cells, we can generate Burkholderia gut symbiont-colonized insects (Sym-insect) and gut symbiont-non-colonized insects (Apo-insect) in the laboratory (Kikuchi et al., 2007; Kim et al., 2015a; Lee J. B. et al., 2017). Additionally, as a large number of naïve Burkholderia gut symbionts can be collected from M4 crypts, it is feasible to study biochemical differences between in vitro-cultured Burkholderia cells and in vivo-colonized symbiotic cells (Kim et al., 2013c,2015b; Byeon et al., 2015). Accordingly, we recently reported unexpected observations and extended our understanding of how this symbiont interacts with host insects at the molecular and biochemical levels (Lee et al., 2019, 2020).
Insects have an immune system that comprises cellular and humoral immune responses (Hoffmann, 2003). When entomopathogenic microorganism infection occurs, the cellular immune response is activated, and the pathogen is immediately eliminated by phagocytosis, encapsulation, and nodulation (Lavine and Strand, 2002; Strand, 2008). At the same time, the humoral immune response of the insect is activated sequentially, and as a result, antimicrobial peptides (AMPs) are produced in the insect’s fat body tissue and secreted into the hemolymph to eradicate the infective pathogens (Lemaitre et al., 1997; Vallet-Gely et al., 2008). However, this type of immune response has been extensively studied in holometabolous insects (complete metamorphic), but not in hemimetabolous insects (incomplete metamorphic) (Kanost and Gorman, 2008; Hillyer, 2016; Yan and Hillyer, 2020).
For R. pedestris insect, we isolated and identified three types of AMPs, riptocin, defensin, and thanatin isoforms, from the hemolymph. These AMPs were found to exhibit effective antimicrobial activity against invasive pathogens (Kim et al., 2015b). In addition, we successfully purified a peptide trialysin from the salivary glands of R. pedestris to confirm the immune response in the oral infection route. Of note, this protein has been reported to play a crucial role in distinguishing gut commensal symbionts from pathogens (Lee D. J. et al., 2017).
Among these AMPs, thanatin isoforms have only been found in the hemipteran insect, Podisus maculiventris (spined soldier bug), which belongs to the same order as R. pedestris (Fehlbaum et al., 1996). Because the thanatin peptide has no sequence homology with other insect defense molecules, its exact role and significance in insects have not been elucidated. In previous study, we found that thanatin isoforms of R. pedestris were synthesized in the midgut region in which the gut symbiont resided (Park et al., 2018). However, we did not demonstrate why R. pedestris insects should control their gut bacterial populations with thanatin isoforms under infection. Therefore, we conducted a study to suggest the exact role of thanatin isoforms in the R. pedestris–Burkholderia symbiosis model. Based on these results, we hypothesized that thanatin isoforms can be unique AMPs that play an important role in hemimetabolous insects and symbiotic relationships. As the gut immune system of R. pedestris in a symbiotic condition has not been clearly elucidated, in this study, we investigated the gut immune response of host insects associated with thanatin isoforms and demonstrated the effect of this AMP on gut symbionts. Here, we found that the expression levels of only thanatin genes were increased in the M4 crypt of Sym-male insects compared to the two other AMPs tested upon systemic bacterial injection. Further, we revealed that thanatin isoforms not only function as AMPs, but also modulate the population of gut symbionts.
Materials and Methods
Bacterial Strains and Growth Conditions
The Burkholderia insecticola symbiont RPE75, a rifampicin-resistant mutant derived from the RPE64 strain (Kikuchi et al., 2011), was cultured until mid-log phase at 30°C in YG-RIF medium (0.5% yeast extract, 0.4% glucose, and 0.1% NaCl supplemented with 30 μg/ml rifampicin) (Lee J. B. et al., 2017). Escherichia coli K12, Serratia marcescens Db11, and Staphylococcus aureus RN4220 cells were cultured until mid-log phase at 37°C in Luria-Bertani medium (Gibco, United States) without any antibiotic substance (Kim et al., 2015a). All bacterial strains were incubated with vigorous shaking.
Insect Rearing and Burkholderia Symbiont Infection
The bean bugs, R. pedestris, were reared in our insect laboratory at 28°C under a photoperiod of 16 h light and 8 h dark, as previously described (Lee et al., 2020). Nymphal insects were reared in clean plastic containers containing soybean seeds and distilled water containing 0.05% ascorbic acid (DWA). Upon reaching adulthood, the insects were transferred to larger containers (35 cm long, 35 cm wide, and 40 cm high); soybean seeds were added to the containers as food, and cotton pads were attached to the walls for egg laying. Eggs were collected daily and transferred to new cages for hatching. A cotton dish was soaked in in vitro-cultured Burkholderia (107 cells/ml of DWA) was provided to the second instar nymphs for 12 h to generate Burkholderia-harboring Sym-insects (Lee et al., 2019). After the insects were fed the inoculum solution for 2 days, fresh DWA was provided instead of the inoculum solution.
cDNA Cloning of Thanatin Isoforms From Riptortus pedestris
To obtain cDNA sequences of thanatin AMPs in the fat body, we used a Gene Racer kit (Invitrogen, United States) (Lee D. J. et al., 2017). After mRNA isolation from the fat body of 3-day-old R. pedestris male adults, truncated and non-mRNAs were removed with calf intestinal phosphatase by dephosphorylation of their 5′ phosphates according to the manufacturer’s instructions. The mRNA was then decapped with tobacco acid phosphatase. The ligated mRNA was reverse-transcribed using a GeneRacer oligo dT primer and Superscript III reverse transcriptase (50°C, 1 h). To obtain the 5′ ends, cDNA was amplified using a GeneRacer 5′ forward primer and a gene-specific 5′ reverse primer or a GeneRacer 5′ nested forward primer and a gene-specific 5′ nested reverse primer with Phusion High-Fidelity DNA polymerase (NEB, United States). The PCR conditions were as follows: 95°C for 30 s, followed by 35 cycles of 95°C for 30 s, 65°C for 30 s, and 68°C for 2 min, and finally 68°C for 5 min. To obtain the 3′ ends, the cDNAs were amplified using a GeneRacer 3′ reverse primer and a gene-specific 3′ forward primer or a GeneRacer 3′ nested reverse primer and a gene-specific 3′ nested forward primer. The PCR conditions were as follows: 95°C for 30 s, followed by 35 cycles of 95°C for 30 s, 65°C for 30 s, and 68°C for 2 min, and finally 68°C for 5 min. The primer sets used for RACE-PCR are listed in Table 1. To determine the nucleotide sequence of the target PCR products, PCR amplification mixtures were cloned using a TOPO TA cloning kit (Invitrogen, United States) and sequenced.
Susceptibility Assay Against Thanatin Isoforms
Escherichia coli K12, S. marcescens Db11, S. aureus RN4220, and cultured-Burkholderia cells were cultured in LB and YG medium and washed with 10 mM phosphate buffer (PB; pH 7.0) to remove the medium components. Gut-colonized Burkholderia cells were collected from the fifth-instar nymphs of Sym-insects by dissection of the M4 crypt and washed twice with 10 mM PB to remove substances from R. pedestris (Byeon et al., 2015; Kim et al., 2015b). To test the antimicrobial activity of thanatin isoforms, the bacterial cells were suspended in 10 mM PB to 103 cells/50 μl for each sample and subsequently incubated with 50 μl of synthesized thanatin solution (Anygen, South Korea) at concentrations of 0.2, 0.4, 1, 2, or 4 μg/ml for 2 h at 30°C. After incubation, the reaction mixtures were spread on LB or YG agar plates containing selective antibiotics. The colonies grown after 48 h of incubation at 30°C were counted.
Thanatin Expression Upon Immune Challenging
qRT-PCR was performed as previously described (Kim et al., 2015a; Lee J. B. et al., 2017). Briefly, E. coli K12 and S. marcescens Db11 cells were adjusted to 1.0 × 107 cells/ml in 10 mM PB. Two microliters of the bacterial cells were systemically injected into 3-day-old R. pedestris male adults for immune induction. After incubation at 28°C for 3 h, immune-challenged fat body and all five midgut regions were collected from the insects by dissection. Total RNA was isolated using TRIzol reagent (Invitrogen, United States), according to the manufacturer’s recommendations. Thereafter, 500 ng of total RNA was converted to cDNA using TOPscript RT DryMix containing oligo-dT primers (Enzynomics, South Korea). The synthesized cDNAs were diluted 20-fold, and qRT-PCR was performed on a QuantStudio™3 Real-Time PCR System (Thermo Fisher Scientific Inc., United States). The PCR cycling condition was as follows: 95°C for 10 min, followed by 40 cycles of 95°C for 10 s, 60°C for 15 s, and 72°C for 20 s. The primer sets used for qRT-PCR are listed in Table 2. The comparative CT (ΔΔCT) method was used to calculate the relative gene expression levels based on the elongation factor 1α gene (EF1α) of R. pedestris (GenBank accession #AB591382) as an endogenous control gene (Lee J. B. et al., 2017). All analyses were performed using the QuantStudio™ Design & Analysis Software Ver 1.5.2 (Thermo Fisher Scientific Inc., United States).
RNA Interference
RNA interference (RNAi) was performed as described previously (Lee J. B. et al., 2017). Double-stranded RNA (dsRNA) for silencing the thanatin genes (target) and the ampicillin-resistant gene (mock control) were synthesized using the primers listed in Table 3. Each recombinant plasmid was constructed by cloning the PCR products that were amplified from R. pedestris fat body cDNA with sense and antisense primers into the pT7Blue T-vector (Novagen, United States). Each T-cloned plasmid was amplified by PCR using a specific primer set containing the T7 promoter region. The cycling conditions were as follows: 95°C for 10 min, followed by 35 cycles of 95°C for 30 s, 65°C for 30 s, and 72°C for 1 min, and finally 72°C for 10 min. After purification of the product with a PCR purification kit (Enzynomics, South Korea), both sense and antisense strands of the transcript were simultaneously synthesized using the MEGA-script RNAi kit (Ambion, United States) with 500 ng of template DNA at 37°C for 2 h, according to the manufacturer’s instructions. The synthesized RNA product was treated with DNase I and RNase to remove the template DNA and ssRNA at 37°C for 30 min. The dsRNAs were purified using the MEGAclear kit (Ambion, United States) and quantified using a Nanodrop 2000 (Thermo Fisher Scientific, United States). The Sym-male insects were treated with dsRNA 200 ng/μl in DNase/RNase-free water using a glass capillary and an air-pressure injector (Picospritzer, Parker, United States). Briefly, two microliters of thanatins dsRNA or DNase/RNase-free water (for mock control) were systemically injected into the joint of the hind leg that connects to the thorax of the insect. The dsRNA-treated insects were reared in clean plastic containers, and colony-forming units (CFUs) in M4 crypts and hemolymph were measured on 4 days after dsRNA injection and 3 days after E. coli cells injection.
Measurement of Symbiont Titers in the M4 Region
Bacterial cells were washed with 10 mM PB and suspended in Grace’s Insect Medium (Gibco, United States) to 1.0 × 107 cells/ml bacterial solutions (Kim et al., 2013a; Lee et al., 2020). Briefly, two microliters of thanatins dsRNA were systemically injected into 3-day-old R. pedestris male adults for immune suppression. In addition, two microliters of the bacterial cell suspensions were injected into the joint of the hind leg that connects to the thorax of the insect. E. coli K12 cells were injected 24 h after dsRNA injection. Individual M4 crypts dissected from R. pedestris 3 days after inoculation were collected in 100 μl of PB, homogenized using a plastic pestle, and serially diluted with PB. The diluted samples were spread onto YG-RIF agar plates and colonies grown at 30°C were counted 2 days later. The symbiont titers for each insect were evaluated. The survival rate was evaluated 7 days later after the bacterial septic injections.
Identification of Symbiotic Burkholderia Cells in Host Hemolymph
To detect gut-colonized symbionts penetrating the M4 crypt into the host hemolymph, each R. pedestris insect with its legs removed was immersed in 200 μl of 10 mM PB (Kim et al., 2015b). After homogenization via vortexing, the insects were removed, and the buffer was serially diluted to measure CFUs. The diluted samples were spread onto YG-RIF agar plates. Colonies grown at 30°C were counted 2 days later.
Tree Analysis
The protein sequences of the thanatin isoforms from R. pedestris were compared. The protein sequences were aligned with ClustalW using MegAlign (ver. 7.1.0.). Phylogenetic trees for the alignment were calculated via the neighbor-joining method and the Jones–Taylor–Thornton model. The bootstrap tree was obtained from the protein sequence using the Molecular Evolutionary Genetic Analysis-X software.
Statistical Analysis
The statistical significance of data was determined by unpaired Student’s t-test or one-way ANOVA with Tukey’s correction using SPSS 23 and GraphPad Prism 8 software.
Results
Thanatin Isoforms With Repeated Specific Regions
First, to identify thanatin isoforms in R. pedestris, total RNA was collected from insect fat body and cDNA was synthesized by RACE-PCR. We cloned putative target DNA fragments encoding precursor proteins of thanatin isoforms, ranging from 125 to 148 residues in length. The InterPro protein families database1 was used to identify the functional domain of the thanatin isoforms. Thanatin precursor proteins contain a signal peptide at the N-terminus. Four repetitive sequences cleaved by trypsin, which did not contain any conserved motifs, were identified (Figure 1).
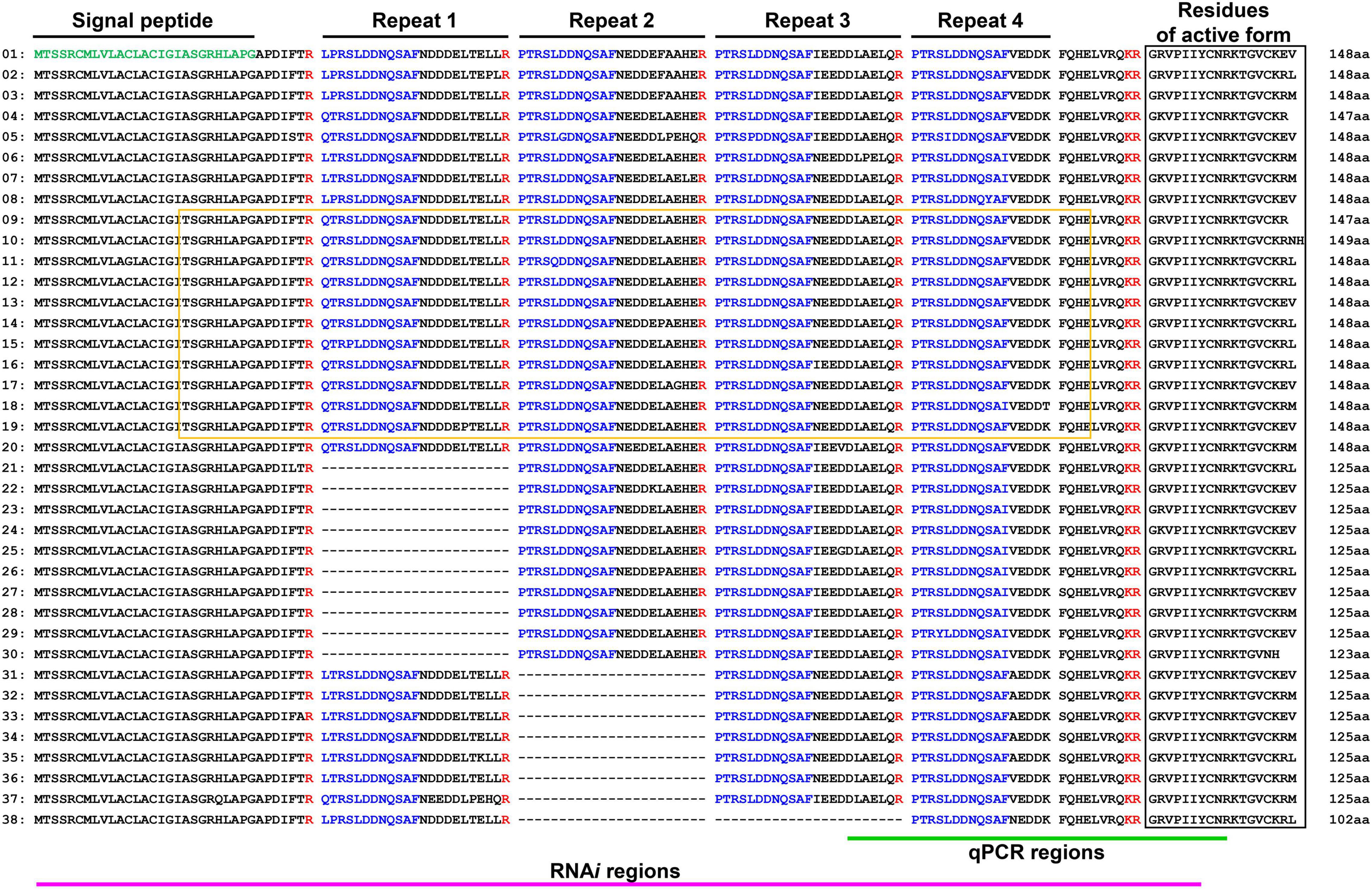
Figure 1. Multiple precursor proteins of thanatin isoforms in R. pedestris. Structure analysis of precursor molecules (green: signal peptide region; blue: repetitive sequence). Black and yellow boxes indicate the region of antimicrobial activity and MACPF domain.
Three Active Forms of Thanatin Isoforms
Structurally active thanatin isoforms derived from 38 precursor proteins were aligned, and their frequencies were investigated. The C-terminus region had three main active forms: KEV, KRL, and KRM (Figure 2A). When thanatin isoforms in R. pedestris were compared with those of P. maculiventris, their active sequences showed similarities and harbored a conserved cysteine disulfide bridge (Figure 2B). The phylogenetic analysis showed that thanatin isoforms can be categorized into two groups: with (upper clade, O) or without (bottom clade, X) the membrane attack complex/perforin (MACPF) domain (Figure 2C).
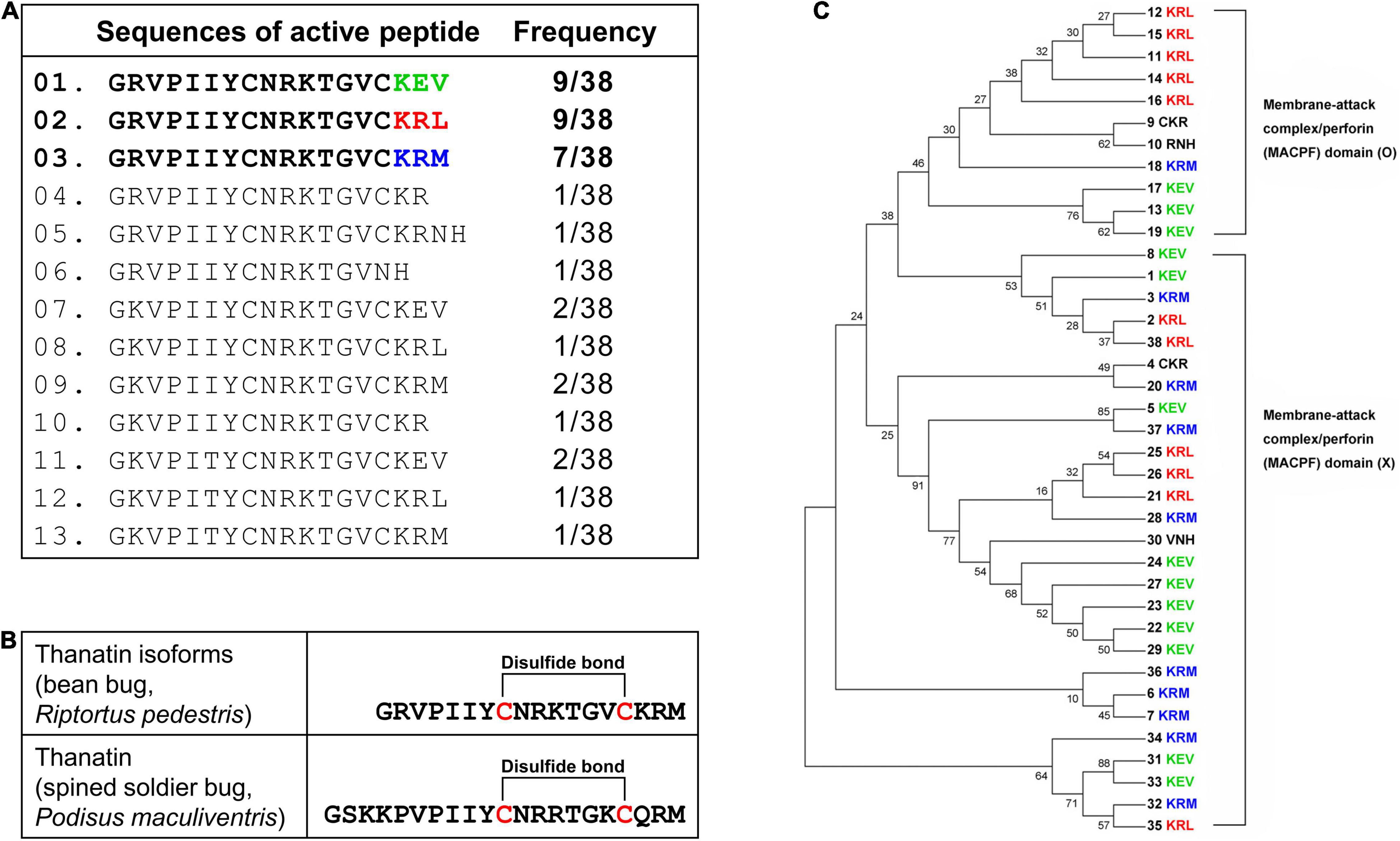
Figure 2. Alignment of active thanatin isoforms. (A) The alignment of amino acid sequences and frequency of thanatin isoforms. (B) Comparison of amino acid sequences and structure between thanatin KRM isoform from R. pedestris and thanatin of P. maculiventris. The line between cysteine residues represents a disulfide bond. (C) Phylogenetic tree using precursor sequences of thanatin isoforms. Thanatin precursor proteins with (upper clade, O) or without (bottom clade, X) the membrane attack complex/perforin (MACPF) domain.
Antimicrobial Activity of Thanatin KEV Isoform Against Gut Colonized Symbionts
To evaluate the antimicrobial activity of the thanatin KEV isoform as an AMP in R. pedestris, the antibacterial activities against E. coli K12, S. marcescens Db11, S. aureus RN4220, cultured Burkholderia, and symbiotic Burkholderia were measured. The Staphylococcus strain, a gram-positive bacterium, had high resistance to the thanatin KEV isoform (Figure 3A). When the KEV isoform was incubated with gram-negative bacterial cells, the growth of the E. coli cells was markedly reduced, but S. marcescens was not killed by the thanatin KEV isoform (Figure 3B). In contrast to in vitro-cultured Burkholderia cells, in vivo-colonized symbionts living in the M4 crypt of R. pedestris were highly susceptible to the KEV isoform (Figure 3C). Other main isoforms showed similar antibacterial activity to the KEV isoform (Supplementary Figure S1).
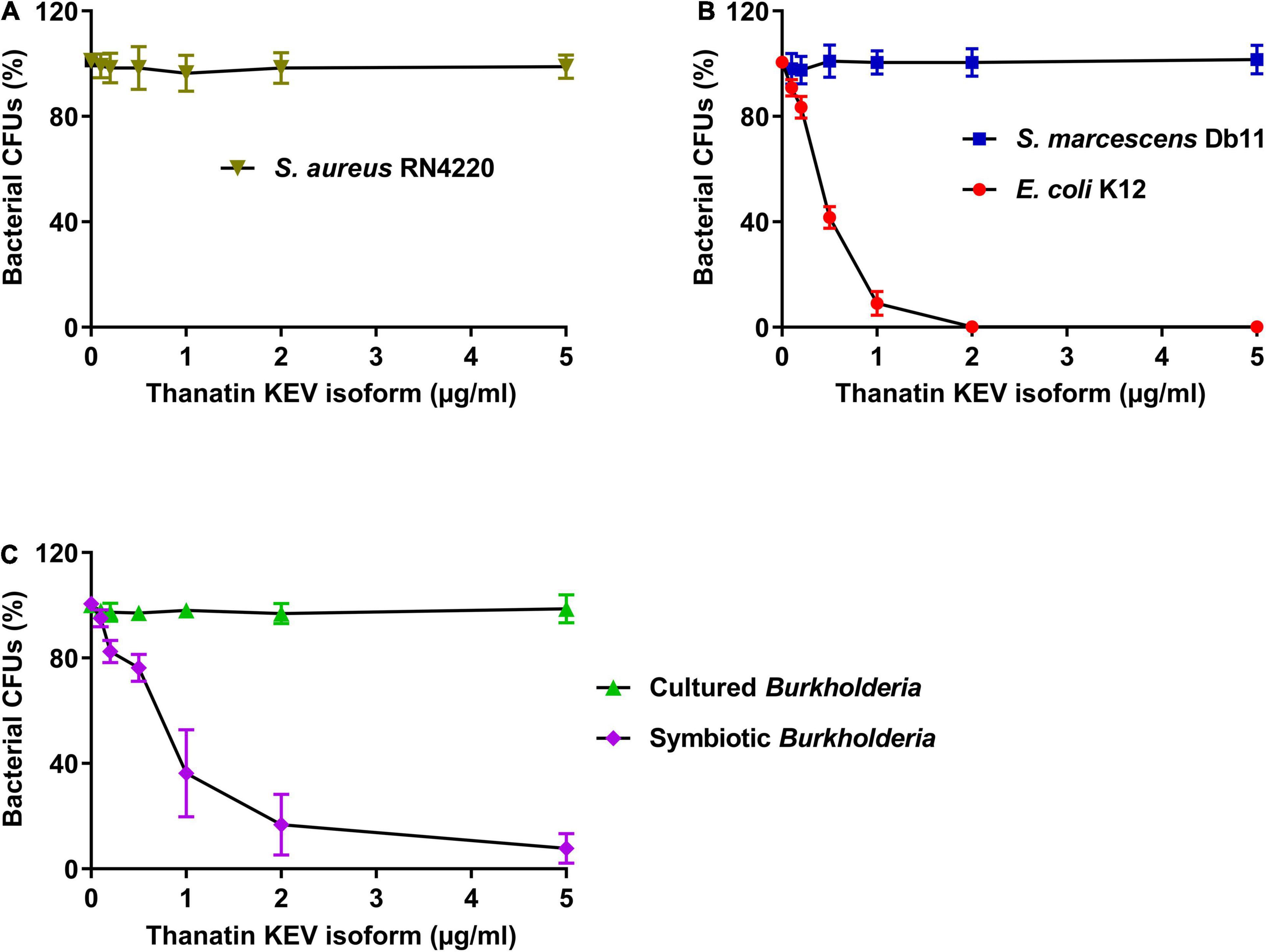
Figure 3. Measurement of the antibacterial activity of thanatin isoforms. The antibacterial activity of thanatin isoforms against (A) S. aureus RN4220, (B) E. coli K12 and S. marcescens Db11, (C) cultured Burkholderia and symbiotic Burkholderia cells. The CFUs were calculated and normalized using AMP-untreated CFUs set to 100%. Data are expressed as mean ± SD (n = 3) and are representative of three independent experiments.
Modulation of Thanatin Isoforms by Burkholderia Gut Symbiont
Based on the above results, thanatin isoforms could be closely involved in the control of symbiotic bacteria colonization as well as the defense response of insects against invasive pathogens. To investigate the expression of thanatin isoforms against systemic bacterial injection in insect midgut regions, total RNA was extracted from Sym-male insect, and the target DNA fragments were amplified with gene-specific primers (Table 2). Interestingly, the expression levels of thanatin genes were comparatively high in the M4 crypt, where the symbiotic bacteria reside, whereas it was not expressed in the other midgut regions of M1 to M4B (Figure 4A). Further, the expression of thanatin genes was not detected without immune-challenge despite the presence of Burkholderia gut symbionts in the M4 crypt (mock-injected and non-injected; Figure 4A). To determine whether riptocin and defensin genes, which are other AMPs of R. pedestris, were also expressed in the symbiotic organ M4 crypt, their expression was evaluated. The expression of thanatin genes after a bacterial challenge with E. coli or S. marcescens cells was higher than that of the riptocin and defensin genes (Figure 4B). We also measured the mRNA levels of the thanatin genes in the M4 crypt of Apo-R. pedestris without symbionts. Expression of thanatin isoforms in Apo-R. pedestris was not detected despite induction of immune response by systemic E. coli or S. marcescens injection (Figure 4C). Taken together, these results demonstrate that the Burkholderia gut symbiont modulates the expression of thanatin isoforms.
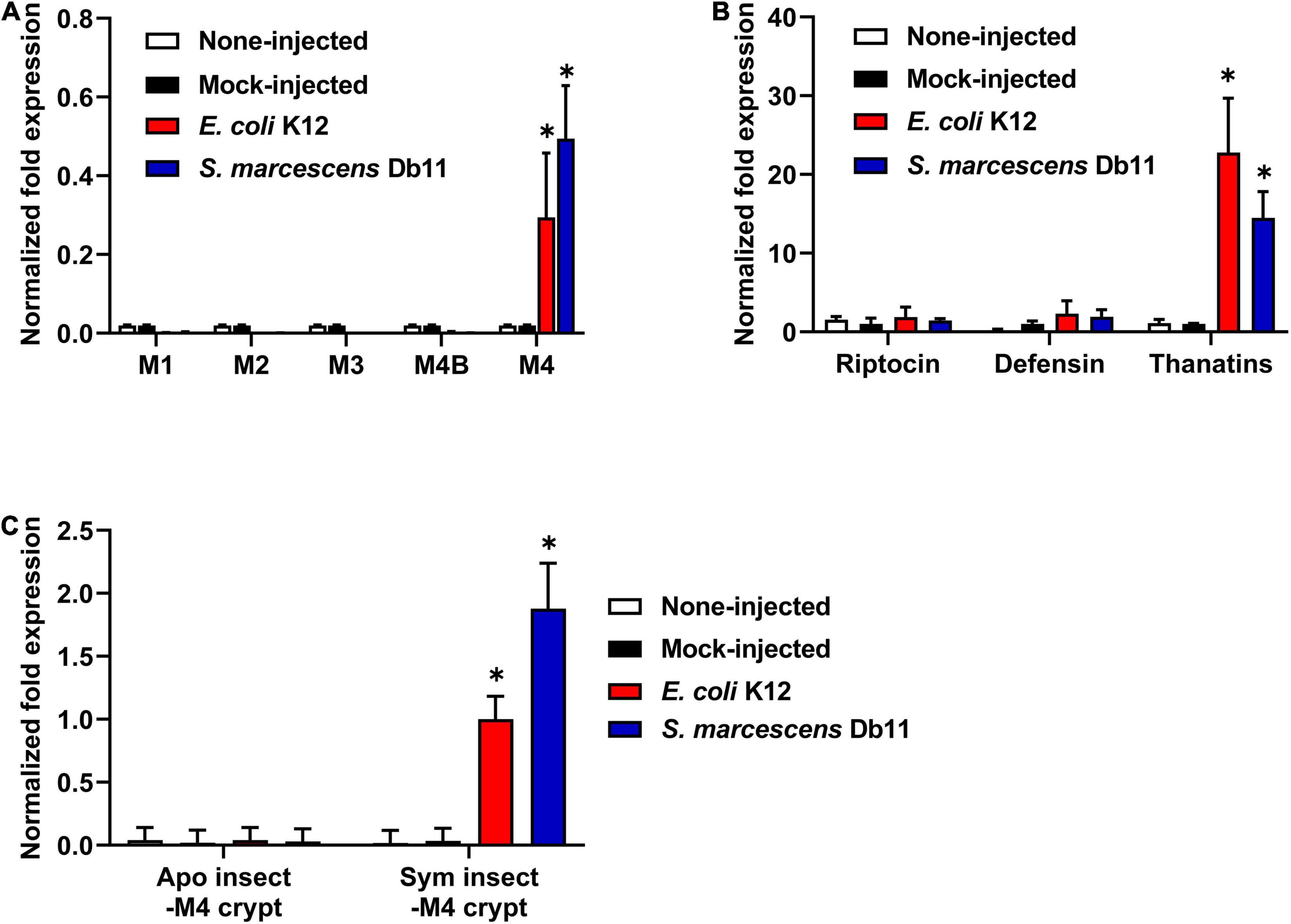
Figure 4. The expression levels of thanatin genes in R. pedestris. (A) mRNA expression levels of thanatin genes in each midgut region (M1, M2, M3, M4B, and M4) of the Sym-male insect upon gram-negative E. coli K12 and S. marcescens Db11 injection. (B) mRNA expression levels of three AMP genes in the M4 crypt of the Sym-male insect upon gram-negative E. coli K12 and S. marcescens Db11 injection. (C) mRNA expression levels of thanatin genes in the M4 crypt of both the Apo-insect and Sym-male insect upon gram-negative E. coli K12 and S. marcescens Db11 injection. The ampicillin resistance gene (amp) was used as a mock-control in the RNAi experiment. The expression levels of the thanatin genes were normalized to the expression level of (A,C) E. coli K12-injected insect or (B) mock-control, which was set as 1. Error bars indicate the standard deviation of the mean (n = 6). Asterisk indicates a significant difference between each group (*p < 0.01; unpaired t-test). (A; M1, M2, M3, and M4B vs. M4), (B; Riptocin or Defensin vs. Thanatins), and (C; Apo insect-M4 crypt vs. Sym insect-M4 crypt).
Control of Gut Symbiont Population by Thanatin Isoforms During Systemic Bacterial Injection
Based on the unique expression of the thanatin isoforms in Sym-R. pedestris, we hypothesized that thanatin isoforms may play another role in the M4 crypt. To elucidate the interaction between the Burkholderia gut symbiont and thanatin isoforms in the M4 crypt, the expression of thanatin genes was suppressed by RNAi. The expression level of the thanatin genes was completely reduced from 1 day after injection of thanatin dsRNA in both fat body and M4 crypts (Figure 5A). Changes in symbiotic bacterial growth were monitored in the M4 crypt following systemic E. coli K12 injection. Interestingly, the suppression of thanatin genes significantly increased the population of Burkholderia gut symbionts (Figure 5B).
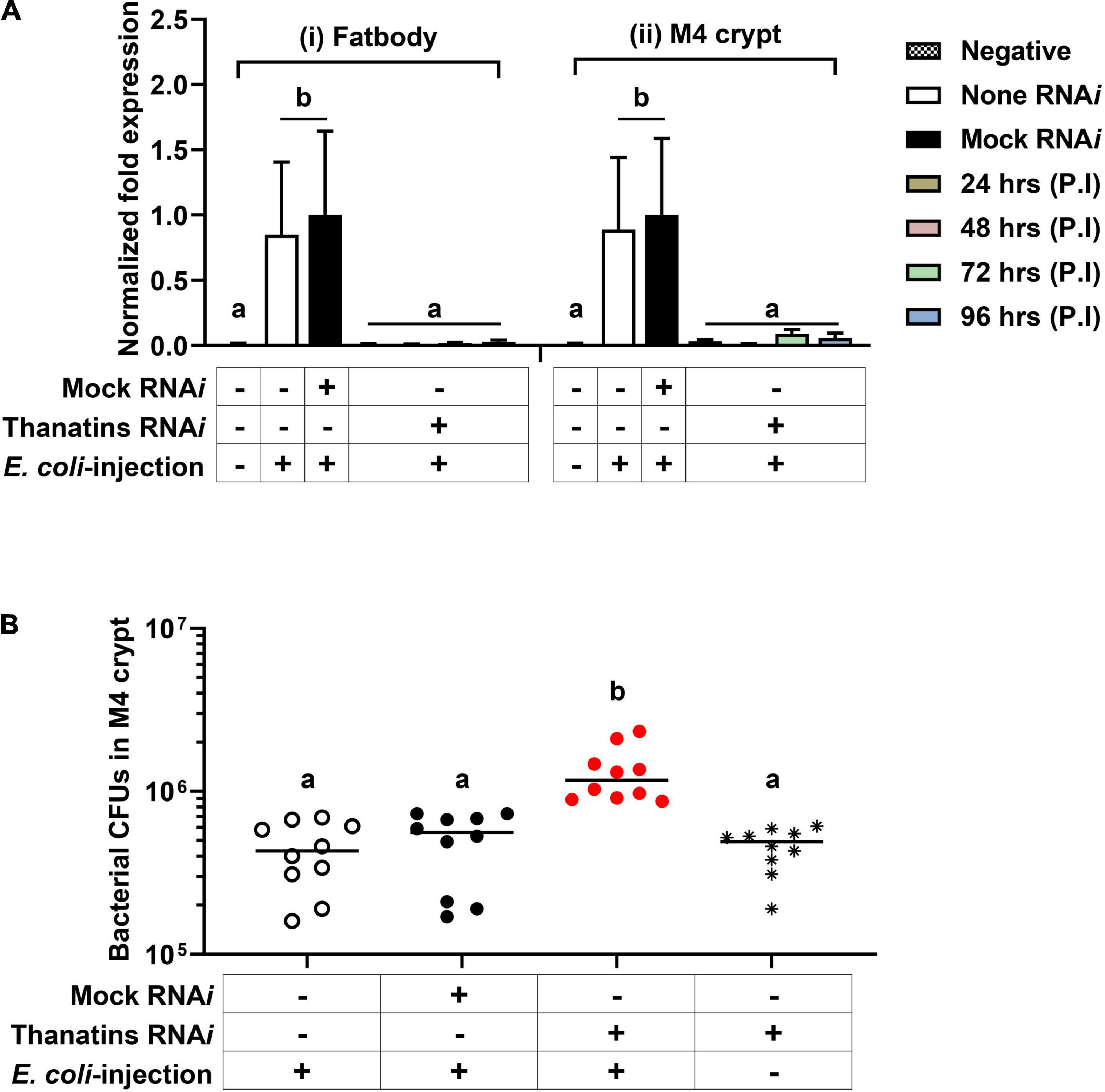
Figure 5. The population of Burkholderia gut symbiont after RNAi of the thanatin isoforms. (A) mRNA expression levels of thanatin genes in (i) the fat body and (ii) M4 crypt after RNAi of the target gene upon systemic E. coli K12 injection. The expression levels of the thanatin genes were normalized to the expression level of each mock-control, which was set as 1. Data are expressed as mean ± SD (n = 6). Different letters (a and b) on the top of the columns indicate statistically significant differences (P < 0.05; one-way ANOVA with Tukey’s correction). (B) The population of M4 crypt-colonized Burkholderia cells in the thanatin genes silenced R. pedestris upon systemic E. coli K12 injection. Data are expressed as mean ± SD (n = 10). Different letters (a and b) on the top of the columns indicate statistically significant differences (P < 0.05; one-way ANOVA with Tukey’s correction). The CFUs in M4 crypts were measured on 4 days after dsRNA injection or 3 days after E. coli K12 cells injection.
Insecticidal Activity and Growth of Gut Symbiont in the Hemolymph of Host Insect
We evaluated the health parameters of host insect to determine the impacts on R. pedestris that can be caused by an increase in the symbiont population in the M4 crypts. Briefly, the survival rate of thanatins knock-downs Sym-R. pedestris was examined after systemic injection with E. coli cells. In addition, to prevent epithelial damage of M4 crypts by systemically injected E. coli cells, an appropriate 104 CFUs was used in the experiment to induce an insect immune response but not cause any harm to the insects. The survival rate of thanatins-suppressed Sym-R. pedestris was found to be markedly lower than that in the control group under the context of systemic injection with E. coli cells (Figure 6A). In contrast, the survival rate of thanatins-suppressed Apo-R. pedestris did not show any statistically significant differences (Figure 7). These results suggest that overgrown symbiont in the M4 crypts by co-injection with RNAi and E. coli cells adversely affect the survival of host insects. Since systemically injected E. coli cells would have been cleared by riptocin AMPs in the hemolymph of insects, it can be expected that overgrown Burkholderia cells are directly linked to insect survival. Based on these results, we investigated whether overgrown symbiont cells were observed in the hemolymph. Burkholderia gut symbiont cells were found to grow rapidly in the hemolymph 3 days post-injection (Figure 6B). Taken together, when the gut symbiont grows out of control in the host, survival of the host decreases, while at the same time gut symbionts are observed in the host’s hemolymph.
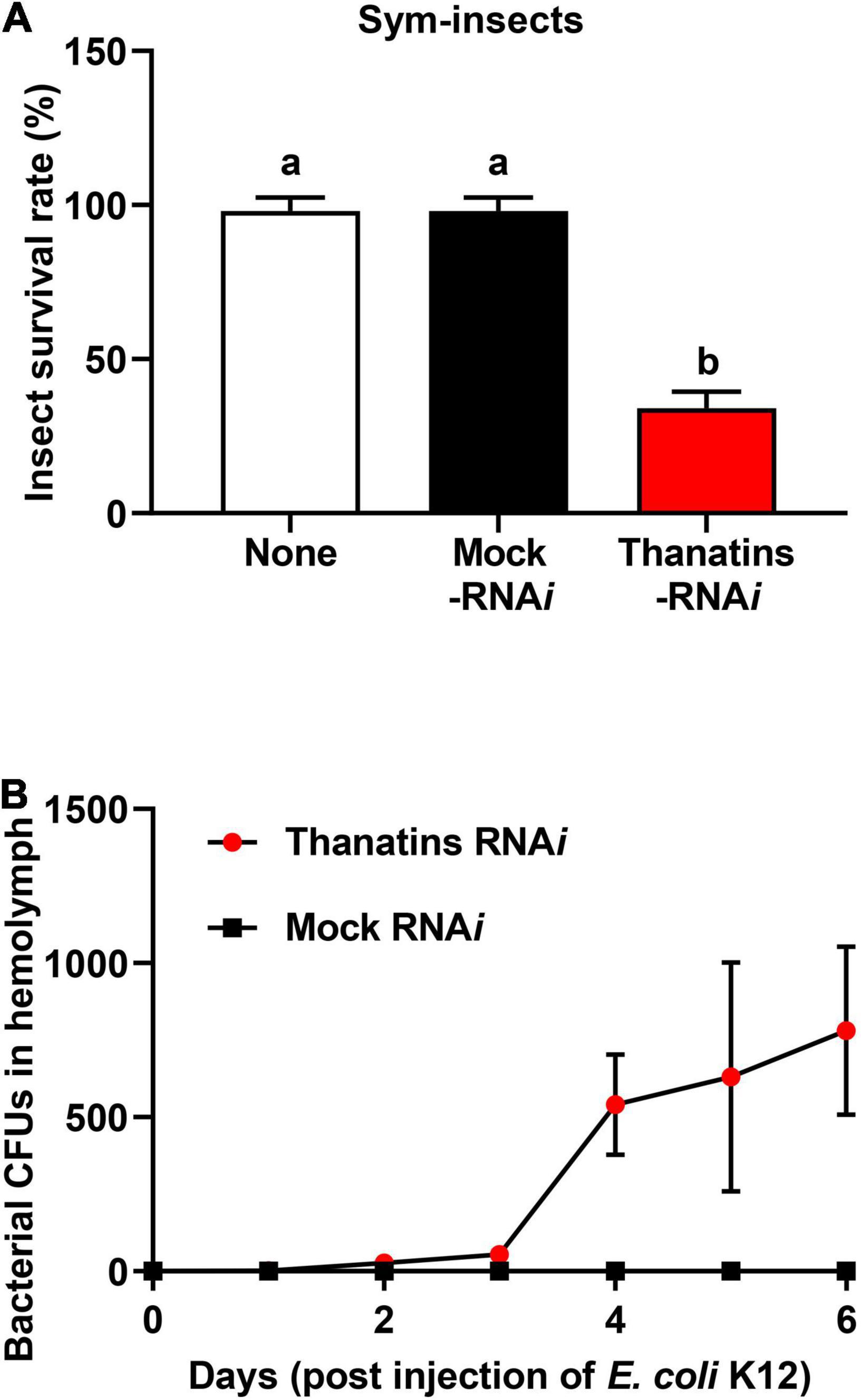
Figure 6. Insecticidal effect of Burkholderia gut symbionts in the hemolymph during systemic E. coli K12 injection. (A) Survival rates of the thanatin gene-silenced Sym-male insects upon systemic E. coli K12 injection. Data are expressed as mean ± SD (n = 10). Different letters (a and b) on the top of the columns indicate statistically significant differences (P < 0.05; one-way ANOVA with Tukey’s correction). (B) Comparison of the symbiont cells detected in insect’s hemolymph by RNAi and systemic E. coli K12 injection. Data are expressed as mean ± SD (n = 3). All data are representative of three independent experiments.
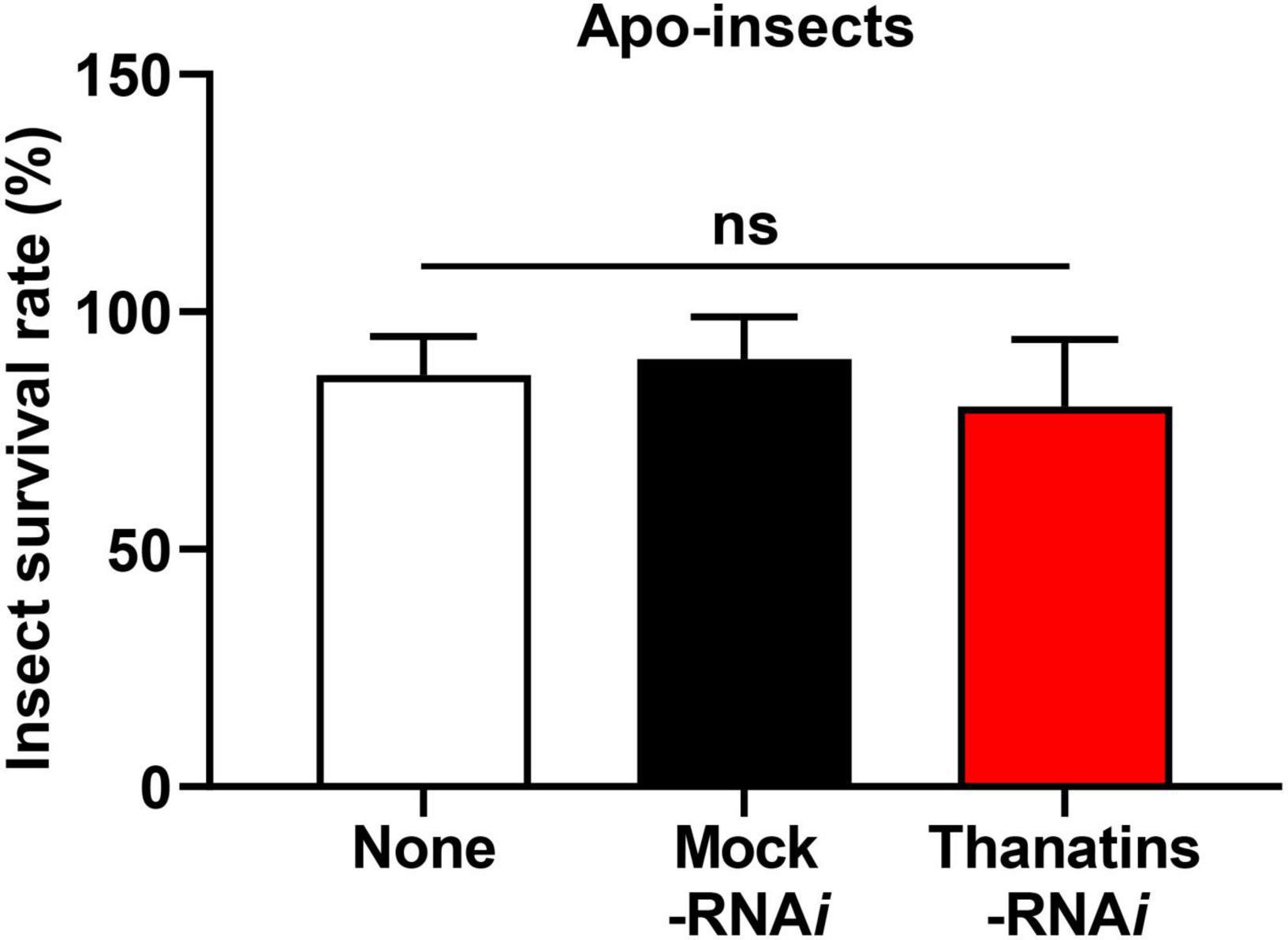
Figure 7. Survival rates of the thanatin gene-silenced Apo-insect upon systemic E. coli K12 injection. Data are expressed as mean ± SD (n = 10). Data are representative of three independent experiments (ns = non-significant) (P < 0.05; one-way ANOVA with Tukey’s correction).
Discussion
Here, we revealed that 38 thanatin precursor proteins consisted of three major active forms. In addition, expression levels of thanatin genes were significantly increased in the M4 crypt of symbiotic insects upon systemic bacterial injection. Moreover, synthetic thanatin isoforms exhibited antibacterial activity against gut-colonized Burkholderia symbionts rather than in vitro-cultured Burkholderia cells. Interestingly, the suppression of thanatin genes significantly increased the population of Burkholderia gut symbionts in the M4 crypt under systemic Escherichia coli K12 injection. Finally, overgrown Burkholderia gut symbionts were observed in the hemolymph of host insects and exhibited insecticidal activity.
As thanatin peptide was first identified in P. maculiventris insects, research has mainly focused on the differences in antimicrobial activity based on the structure of thanatin (Taguchi et al., 2000; Sinha et al., 2017; Vetterli et al., 2018). Thanatin peptides contain a disulfide bond between Cys11 and Cys18 at their C-terminus (Bulet et al., 1999). The core structure of thanatin is an anti-parallel β-sheet structure from Ile8 to the C-terminus and is maintained by a single disulfide bond; the seven amino acids at the N-terminus form the arm structure (Fehlbaum et al., 1996). Three residues (QRM) at the C-terminus are important for its antibacterial activity, and three residues (GSK) at the N-terminal region are required for its antifungal activity (Powers and Hancock, 2003; Bulet and Stocklin, 2005). The number of amino acid residues within a single disulfide bond affects antibacterial activity (Lee et al., 2002). Collectively, the C-terminal residues of thanatin are more critical for antimicrobial activity than the N-terminal residues (Dash and Bhattacharjya, 2021). Despite studies on the structure and antimicrobial activity of thanatin, the precursor molecules of this peptide have not been identified. Therefore, we report for the first time the amino acid sequences of the thanatin precursor proteins and demonstrate the novel role of the peptide thanatin in the insect–symbiont interaction.
The innate immune mechanisms in the hemimetabolous insect, R. pedestris, have been studied markedly less than those in holometabolous insects. However, recent research on the immune response has been steadily progressing owing to its advantages as a symbiotic model (Kim et al., 2015a; Jang et al., 2017; Lee D. J. et al., 2017). Although the three AMPs, riptocin (pyrrhocoricin-like), defensin, and thanatin, are well known in insects (Kragol et al., 2001; Oppenheim et al., 2003; Dash and Bhattacharjya, 2021), their molecular mechanisms and physiological roles in R. pedestris–B. insecticola symbiosis are still unclear. The peptide riptocin is derived from a precursor protein consisting of 678 amino acid residues with 14 tandem repeats (Miura et al., 1996). Each repeat contains a proline-rich region, which is also found in other insects (Levashina et al., 1995; Li et al., 2014; Taniguchi et al., 2016). While riptocin is produced from a single multipeptide precursor, we revealed that R. pedestris insects have 38 genes that can produce 13 different types of thanatin isoforms (Figure 1). The multiple precursor structures of thanatin isoforms are unique in R. pedestris and were categorized into two groups depending on the existence of the MACPF domain (Figure 2C). The MACPF domain is found in the complement system of mammalian immunity, and molecules with the MACPF domain are known to play important roles in defense against bacterial and viral infections (Rosado et al., 2008; Reboul et al., 2016; Liu and Lieberman, 2020). In the insect model, the MACPF domain was identified only in the Drosophila Torso-like protein, which is involved in the control of development and cellular immune response (Johnson et al., 2013; Forbes-Beadle et al., 2016).
In this study, thanatin isoforms showed antibacterial activities against E. coli K12 and gut colonized symbiont, but not against S. marcescens Db11 and cultured Burkholderia cells (Figure 3). This difference in antibacterial activity could be due to the molecular interaction between the thanatin isoforms and the lipopolysaccharide (LPS) pattern of each bacterium. Thanatin causes charge neutralization of the outer membrane, displacing the stabilizing Ca2+ ions from the LPS molecules (Ma et al., 2019). The charge-neutralized outer membrane efficiently induces cell aggregation, eventually leading to bacterial cell death (Wu et al., 2010; Sinha et al., 2017). In addition, thanatin binds to the LPS transport protein complex (Lpt proteins) located between the inner and outer membranes and inactivates metallo-β-lactamase in the periplasm (Vetterli et al., 2018; Moura et al., 2020). S. marcescens Db11 and cultured Burkholderia have a smooth type of LPS with lipid A plus core oligosaccharide (OS) and O-antigen, whereas E. coli K12 and gut colonized symbionts have a rough type of LPS lacking the O-antigen (Washizaki et al., 2016; Ebbensgaard et al., 2018). Of note, the main isoforms of thanatin exhibit selective antibacterial activity depending on growth conditions (Figure 3C) or the existence of O-antigen in the LPS layer (Figure 3B). Therefore, the structural differences in the LPS layers may affect the antibacterial activity of thanatin isoforms.
In many insects, mutualistic bacteria can inhabit symbiotic organs, or bacteriomes, providing nutrients to the host or enhancing the immune response, among other potential functions (Moran et al., 2003; Douglas, 2014; Flórez et al., 2015). Interestingly, the overgrown Burkholderia gut symbionts are eliminated by the host immune response (Kim et al., 2013a; Byeon et al., 2015), but can move to the hemolymph from the M4 crypt by immune suppression, such as the inhibition of thanatin isoforms with E. coli K12 injection (Figure 6B). The regulation of symbiont populations by host insects has been shown to influence their migration. A previous report revealed that the coleoptericin-A AMP of weevil insects selectively targets endosymbionts within the bacteriocytes and regulates their growth by inhibiting cell division (Anselme et al., 2008; Login et al., 2011). Therefore, similar to the AMP, coleoptericin-A, the thanatin isoforms of R. pedestris may also play an important role in regulating and maintaining colonization of the Burkholderia gut symbiont.
Recently, we reported that the cell wall components of Burkholderia gut symbionts are important factors for successful symbiosis (Kim et al., 2013b,2016b,2017). As mentioned above, the symbiotic Burkholderia colonized in the M4 crypt of R. pedestris lacks an O-antigen residue (Kim et al., 2015b). However, it has not yet been elucidated what additional changes occurred in the symbiotic Burkholderia cells other than the O-antigen deficiency. A further study revealed that the colonization of Burkholderia gut symbionts with truncated core OSs impairs host health and reduces insect survival during systemic bacterial injection (Kim et al., 2017). Based on previously reported studies that LPS composed of lipid A and core OS residues and O-antigens are associated with bacterial endotoxin (Raetz and Whitfield, 2002; Nikaido, 2003), these results suggest that Burkholderia gut symbionts that migrate to the hemolymph owing to overpopulation can kill insects. When Sym-R. pedestris was injected with Burkholderia LPS mutant strains, the insect survival rate was significantly decreased (Supplementary Figure S2). In addition, Burkholderia gut symbionts, which moved from the M4 crypt, showed insecticidal activity when co-injected with E. coli and impaired thanatin production in the insect (Figure 6A). However, insecticidal activity was not observed in thanatins-suppressed Apo-R. pedestris (Figure 7). This result suggests that systematically injected E. coli cells did not cause any harm to Apo-R. pedestris insects without Burkholderia gut symbionts. Therefore, the hypothesis that Burkholderia symbionts came out into hemolymph due to damage to midgut epithelial cells caused by systemically injected E. coli cells can be excluded. Taken together, the Burkholderia gut symbionts, when maintained up to a suitable number of populations in the symbiotic organ, confer positive effects, such as immunity enhancement on the host insect, but may exhibit an insecticidal effect in the hemolymph of R. pedestris, when the host cannot control them and under the context of a systemic injection with E. coli cells.
The Burkholderia gut symbionts accumulate polyhydroxyalkanoate (PHA) granules, a bacterial endocellular storage polymer, to adapt to the harsh environment in the midgut region of R. pedestris (Kim et al., 2013c). PHA granules allow the Burkholderia gut symbiont to resist nutrient depletion and environmental stress. Given the various resistance capabilities provided by PHA granules to bacterial cells (Anderson and Dawes, 1990; Kadouri et al., 2003; Ratcliff et al., 2008), the gut symbionts may also become resistant to insect immune cells due to the PHA granules. Therefore, we carefully suggest the following hypothetical mechanisms (Figure 8): in the natural state, R. pedestris synthesize thanatin isoforms during systemic injection of foreign bacteria to properly regulate the growth of Burkholderia gut symbionts. When thanatin genes were suppressed, the Burkholderia population in the M4 crypt was increased and subsequently migrated to the hemolymph through the intestinal barrier. Although migrating Burkholderia gut symbionts are engulfed by immune cells, such as phagocytic hemocytes in the hemolymph, these gut bacterial cells may become resistant to cellular immune responses by their own PHA granules. Eventually, Burkholderia gut symbionts with truncated core OSs present in the hemolymph can impair host health and reduces insect survival.
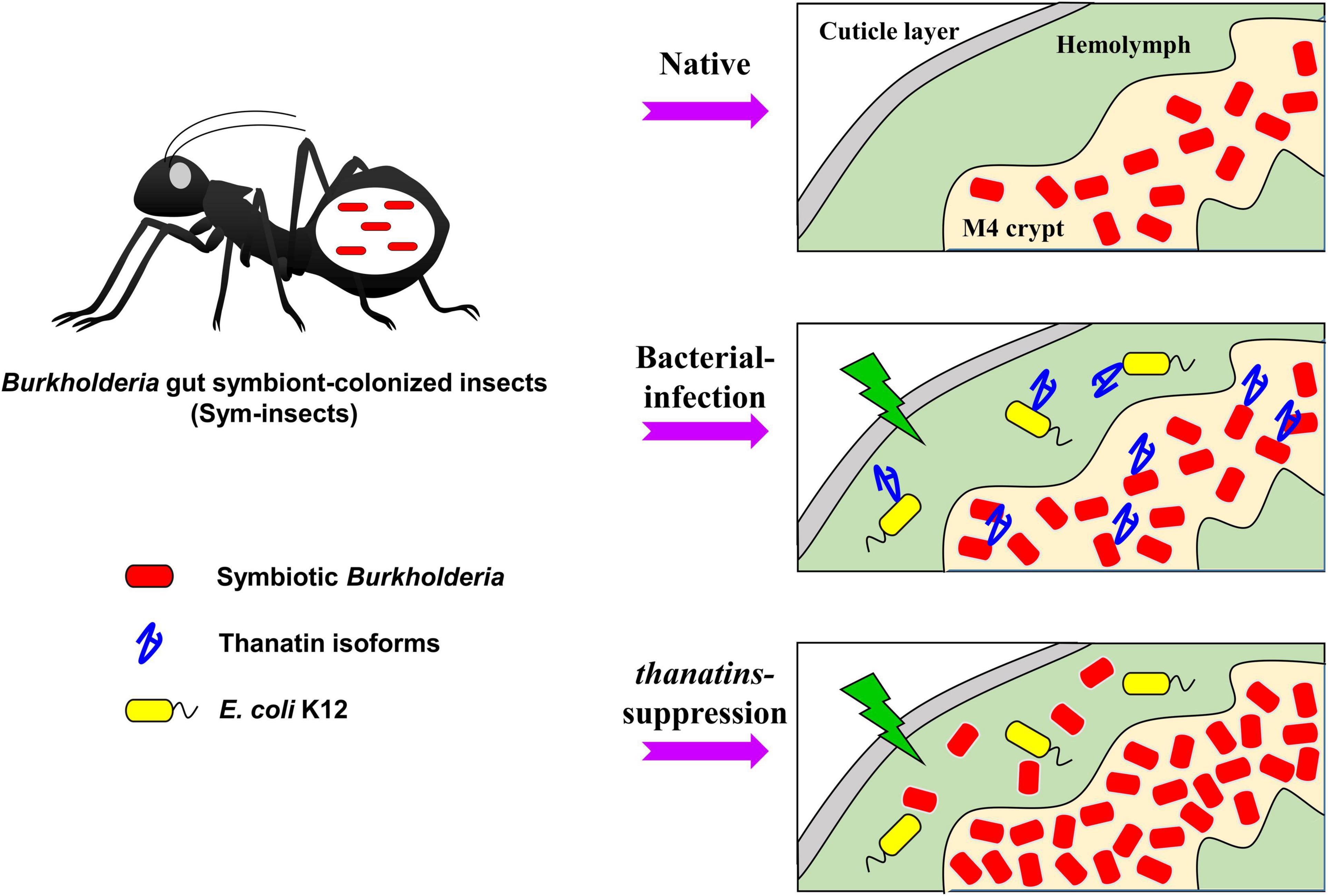
Figure 8. Putative mechanism of action of the thanatin isoforms against Burkholderia gut symbionts during systemic bacterial injection. Thanatin isoforms maintain the gut bacterial population in the M4 crypt during systemic bacterial injection. In contrast, in absence of thanatin (thanatins RNAi) and after systemic E. coli K12 injection, overgrown Burkholderia gut symbionts were observed in the hemolymph of the R. pedestris host and exhibited insecticidal activity.
In this study, scientific evidence is needed as to whether symbiotic bacteria are resistant to hemocytes by PHA granules and what endotoxin substances they secrete. Further studies could clearly demonstrate that the Burkholderia gut symbionts outside the M4 crypt reduces the immunity of the R. pedestris host insect.
Conclusion
Thanatin isoforms are derived from multiple precursor proteins and function as AMPs that modulate the population of gut symbionts. This study provides novel information on the role of thanatin isoforms in the symbiotic organs of R. pedestris and the identification of novel symbiotic factors.
In addition, this study reveals very interesting findings about the indirect interaction between resident symbionts and foreign infecting bacteria that are modulated by the host immune system. Finally, this study can be considered as basic data on how stability can be maintained after establishment of symbiosis.
Data Availability Statement
The original contributions presented in the study are included in the article/Supplementary Material, further inquiries can be directed to the corresponding author/s.
Ethics Statement
The animal study was reviewed and approved by all animal treatment guidelines applicable to invertebrate animals under international and South Korean law have been followed.
Author Contributions
JL and D-WL conceived and designed the study and wrote the manuscript. JL performed the experiments. JL and WHC analyzed the data. All the authors read and approved the final manuscript.
Funding
This research was supported by the Basic Science Research Program through the National Research Foundation of Korea (NRF) funded by the Ministry of Education (NRF-2020R1I1A1A0106704312). This research was also supported by the Korea Basic Science Institute (National Research Facilities and Equipment Center) grant funded by the Ministry of Education (2019R1A6C1010044).
Conflict of Interest
The authors declare that the research was conducted in the absence of any commercial or financial relationships that could be construed as a potential conflict of interest.
Publisher’s Note
All claims expressed in this article are solely those of the authors and do not necessarily represent those of their affiliated organizations, or those of the publisher, the editors and the reviewers. Any product that may be evaluated in this article, or claim that may be made by its manufacturer, is not guaranteed or endorsed by the publisher.
Acknowledgments
We thank Bok Luel Lee and Chan-Hee Kim (Pusan National University) for providing information on thanatin AMP, Doo-Hyung Lee (Gachon University) for providing R. pedestris, and Jiyeun Kate Kim (Kosin University) for providing B. insecticola mutant strains.
Supplementary Material
The Supplementary Material for this article can be found online at: https://www.frontiersin.org/articles/10.3389/fmicb.2021.796548/full#supplementary-material
Supplementary Figure S1 | The antibacterial activity of (i) thanatin KRL isoform and (ii) thanatin KRM isoform against (A) E. coli K12 and S. marcescens Db11, (B) Cultured Burkholderia and symbiotic Burkholderia cells. CFUs were normalized by AMP-untreated CFUs set to 100%. Data are expressed as mean ± SD (n = 3). Data are representative of three independent experiments.
Supplementary Figure S2 | Survival rates of R. pedestris upon systemic injection with the LPS mutant strains. Data are expressed as mean ± SD (n = 10). Data are representative of three independent experiments.
Footnotes
References
Anderson, A. J., and Dawes, E. A. (1990). Occurrence, metabolism, metabolic role, and industrial uses of bacterial polyhydroxyalkanoates. Microbiol. Rev. 54, 450–472. doi: 10.1128/mr.54.4.450-472.1990
Anselme, C., Pérez-Brocal, V., Vallier, A., Vincent-Monegat, C., Charif, D., Latorre, A., et al. (2008). Identification of the Weevil immune genes and their expression in the bacteriome tissue. BMC Biol. 6:43. doi: 10.1186/1741-7007-6-43
Bulet, P., and Stocklin, R. (2005). Insect antimicrobial peptides: structures, properties and gene regulation. Protein Pept. Lett. 12, 3–11.
Bulet, P., Hetru, C., Dimarcq, J.-L., and Hoffmann, D. (1999). Antimicrobial peptides in insects; structure and function. Dev. Comp. Immunol. 23, 329–344. doi: 10.1016/s0145-305x(99)00015-4
Byeon, J. H., Seo, E. S., Lee, J., Lee, M. J., Kim, J. K., Yoo, J. W., et al. (2015). A specific cathepsin-L-like protease purified from an insect midgut shows antibacterial activity against gut symbiotic bacteria. Dev. Comp. Immunol. 53, 79–84. doi: 10.1016/j.dci.2015.06.003
Dash, R., and Bhattacharjya, S. (2021). Thanatin: an emerging host defense antimicrobial peptide with multiple modes of action. Int. J. Mol. Sci. 22:1522. doi: 10.3390/ijms22041522
Douglas, A. E. (2014). The molecular basis of bacterial-insect symbiosis. J. Mol. Biol. 426, 3830–3837. doi: 10.1016/j.jmb.2014.04.005
Ebbensgaard, A., Mordhorst, H., Aarestrup, F. M., and Hansen, E. B. (2018). The role of outer membrane proteins and lipopolysaccharides for the sensitivity of Escherichia coli to antimicrobial peptides. Front. Microbiol. 9:2153. doi: 10.3389/fmicb.2018.02153
Fehlbaum, P., Bulet, P., Chernysh, S., Briand, J.-P., Roussel, J.-P., Letellier, L., et al. (1996). Structure-activity analysis of thanatin, a 21-residue inducible insect defense peptide with sequence homology to frog skin antimicrobial peptides. Proc. Natl. Acad. Sci. U.S.A. 93, 1221–1225. doi: 10.1073/pnas.93.3.1221
Flórez, L. V., Biedermann, P. H., Engl, T., and Kaltenpoth, M. (2015). Defensive symbioses of animals with prokaryotic and eukaryotic microorganisms. Nat. Prod. Rep. 32, 904–936. doi: 10.1039/c5np00010f
Forbes-Beadle, L., Crossman, T., Johnson, T. K., Burke, R., Warr, C. G., and Whisstock, J. C. (2016). Development of the cellular immune system of Drosophila requires the membrane attack complex/perforin-like protein torso-like. Genetics 204, 675–681. doi: 10.1534/genetics.115.185462
Hillyer, J. F. (2016). Insect immunology and hematopoiesis. Dev. Comp. Immunol. 58, 102–118. doi: 10.1016/j.dci.2015.12.006
Jang, H. A., Seo, E. S., Seong, M. Y., and Lee, B. L. (2017). A midgut lysate of the Riptortus pedestris has antibacterial activity against LPS O-antigen-deficient Burkholderia mutants. Dev. Comp. Immunol. 67, 97–106. doi: 10.1016/j.dci.2016.11.006
Johnson, T. K., Crossman, T., Foote, K. A., Henstridge, M. A., Saligari, M. J., Beadle, L. F., et al. (2013). Torso-like functions independently of torso to regulate Drosophila growth and developmental timing. Proc. Natl. Acad. Sci. U.S.A. 110, 14688–14692. doi: 10.1073/pnas.1309780110
Kadouri, D., Jurkevitch, E., and Okon, Y. (2003). Involvement of the reserve material poly-β-hydroxybutyrate in Azospirillum brasilense stress endurance and root colonization. Appl. Environ. Microbiol. 69, 3244–3250. doi: 10.1128/AEM.69.6.3244-3250.2003
Kanost, M. R., and Gorman, M. J. (2008). Phenoloxidases in insect immunity. Insect Immunology, 1, 69–96. doi: 10.1016/B978-012373976-6.50006-9
Kikuchi, Y., Hosokawa, T., and Fukatsu, T. (2007). Insect-microbe mutualism without vertical transmission: a stinkbug acquires a beneficial gut symbiont from the environment every generation. Appl. Environ. Microbiol. 73, 4308–4316. doi: 10.1128/AEM.00067-07
Kikuchi, Y., Hosokawa, T., and Fukatsu, T. (2011). An ancient but promiscuous host-symbiont association between Burkholderia gut symbionts and their heteropteran hosts. ISME J. 5:446. doi: 10.1038/ismej.2010.150
Kikuchi, Y., Meng, X.-Y., and Fukatsu, T. (2005). Gut symbiotic bacteria of the genus Burkholderia in the broad-headed bugs Riptortus clavatus and Leptocorisa chinensis (Heteroptera: Alydidae). Appl. Environ. Microbiol. 71, 4035–4043. doi: 10.1128/AEM.71.7.4035-4043.2005
Kim, J. K., Jang, H. A., Kim, M. S., Cho, J. H., Lee, J., Lorenzo, F. D., et al. (2017). The lipopolysaccharide core oligosaccharide of Burkholderia plays a critical role in maintaining a proper gut symbiosis with the bean bug Riptortus pedestris. J. Biol. Chem. 292, 19226–19237. doi: 10.1074/jbc.M117.813832
Kim, J. K., Jang, H. A., Won, Y. J., Kikuchi, Y., Han, S. H., Kim, C.-H., et al. (2014). Purine biosynthesis-deficient Burkholderia mutants are incapable of symbiotic accommodation in the stinkbug. ISME J. 8:552. doi: 10.1038/ismej.2013.168
Kim, J. K., Lee, H. J., Kikuchi, Y., Kitagawa, W., Nikoh, N., Fukatsu, T., et al. (2013b). Bacterial cell wall synthesis gene uppP is required for Burkholderia colonization of the stinkbug gut. Appl. Environ. Microbiol. 79, 4879–4886.
Kim, J. K., Won, Y. J., Nikoh, N., Nakayama, H., Han, S. H., Kikuchi, Y., et al. (2013c). Polyester synthesis genes associated with stress resistance are involved in an insect-bacterium symbiosis. Proc. Natl. Acad. Sci. U.S.A. 110, E2381–E2389. doi: 10.1073/pnas.1303228110
Kim, J. K., Kim, N. H., Jang, H. A., Kikuchi, Y., Kim, C.-H., Fukatsu, T., et al. (2013a). Specific midgut region controlling the symbiont population in an insect-microbe gut symbiotic association. Appl. Environ. Microbiol. 79, 7229–7233. doi: 10.1128/AEM.02152-13
Kim, J. K., Lee, J., Huh, Y. R., Jang, H. A., Kim, C.-H., Yoo, J. W., et al. (2015a). Burkholderia gut symbionts enhance the innate immunity of host Riptortus pedestris. Dev. Comp. Immunol. 53, 265–269. doi: 10.1016/j.dci.2015.07.006
Kim, J. K., Son, D. W., Kim, C.-H., Cho, J. H., Marchetti, R., Silipo, A., et al. (2015b). Insect gut symbiont susceptibility to host antimicrobial peptides caused by alteration of the bacterial cell envelope. J. Biol. Chem. 290, 21042–21053. doi: 10.1074/jbc.M115.651158
Kim, J. K., Park, H. Y., and Lee, B. L. (2016b). The symbiotic role of O-antigen of Burkholderia symbiont in association with host Riptortus pedestris. Dev. Comp. Immunol. 60, 202–208. doi: 10.1016/j.dci.2016.02.009
Kim, J. K., Lee, J., Jang, H. A., Han, Y. S., Fukatsu, T., and Lee, B. L. (2016a). Understanding regulation of the host-mediated gut symbiont population and the symbiont-mediated host immunity in the Riptortus-Burkholderia symbiosis system. Dev. Comp. Immunol. 64, 75–81. doi: 10.1016/j.dci.2016.01.005
Kragol, G., Lovas, S., Varadi, G., Condie, B. A., Hoffmann, R., and Otvos, L. (2001). The antibacterial peptide pyrrhocoricin inhibits the ATPase actions of DnaK and prevents chaperone-assisted protein folding. Biochemistry 40, 3016–3026. doi: 10.1021/bi002656a
Lavine, M., and Strand, M. (2002). Insect hemocytes and their role in immunity. Insect Biochem. Mol. Biol. 32, 1295–1309. doi: 10.1016/s0965-1748(02)00092-9
Lee, D. J., Lee, J., Jang, H. A., Ferrandon, D., and Lee, B. L. (2017). An antimicrobial protein of the Riptortus pedestris salivary gland was cleaved by a virulence factor of Serratia marcescens. Dev. Comp. Immunol. 67, 427–433. doi: 10.1016/j.dci.2016.08.009
Lee, J. B., Park, K.-E., Lee, S. A., Jang, S. H., Eo, H. J., Jang, H. A., et al. (2017). Gut symbiotic bacteria stimulate insect growth and egg production by modulating hexamerin and vitellogenin gene expression. Dev. Comp. Immunol. 69, 12–22. doi: 10.1016/j.dci.2016.11.019
Lee, J., Byeon, J. H., Jang, H. A., Kim, J. K., Yoo, J. W., Kikuchi, Y., et al. (2015). Bacterial cell motility of Burkholderia gut symbiont is required to colonize the insect gut. FEBS Lett. 589, 2784–2790. doi: 10.1016/j.febslet.2015.08.022
Lee, J., Kim, C.-H., Jang, H. A., Kim, J. K., Kotaki, T., Shinoda, T., et al. (2019). Burkholderia gut symbiont modulates titer of specific juvenile hormone in the bean bug Riptortus pedestris. Dev. Comp. Immunol. 99:103399. doi: 10.1016/j.dci.2019.103399
Lee, J., Mao, X., Lee, Y. S., Lee, D. J., Kim, J., Kim, J. K., et al. (2020). Putative host-derived growth factors inducing colonization of Burkholderia gut symbiont in Riptortus pedestris insect. Dev. Comp. Immunol. 104:103570. doi: 10.1016/j.dci.2019.103570
Lee, M. K., Cha, L., Lee, S. H., and Hahm, K.-S. (2002). Role of amino acid residues within the disulfide loop of thanatin, a potent antibiotic peptide. BMB Rep. 35, 291–296. doi: 10.5483/bmbrep.2002.35.3.291
Lemaitre, B., Reichhart, J.-M., and Hoffmann, J. A. (1997). Drosophila host defense: differential induction of antimicrobial peptide genes after infection by various classes of microorganisms. Proc. Natl. Acad. Sci. U.S.A. 94, 14614–14619. doi: 10.1073/pnas.94.26.14614
Levashina, E. A., Ohresser, S., Bulet, P., Reichhart, J. M., Hetru, C., and Hoffmann, J. A. (1995). Metchnikowin, a novel immune-inducible proline-rich peptide from Drosophila with antibacterial and antifungal properties. Eur. J. Biochem. 233, 694–700. doi: 10.1111/j.1432-1033.1995.694_2.x
Li, W., Tailhades, J., O’brien-Simpson, N. M., Separovic, F., Otvos, L., Hossain, M. A., et al. (2014). Proline-rich antimicrobial peptides: potential therapeutics against antibiotic-resistant bacteria. Amino Acids 46, 2287–2294. doi: 10.1007/s00726-014-1820-1
Liu, X., and Lieberman, J. (2020). Knocking’em dead: pore-forming proteins in immune defense. Annu. Rev. Immunol. 38, 455–485. doi: 10.1146/annurev-immunol-111319-023800
Login, F. H., Balmand, S., Vallier, A., Vincent-Monégat, C., Vigneron, A., Weiss-Gayet, M., et al. (2011). Antimicrobial peptides keep insect endosymbionts under control. Science 334, 362–365. doi: 10.1126/science.1209728
Ma, B., Fang, C., Lu, L., Wang, M., Xue, X., Zhou, Y., et al. (2019). The antimicrobial peptide thanatin disrupts the bacterial outer membrane and inactivates the NDM-1 metallo-β-lactamase. Nat. Commun. 10:3517. doi: 10.1038/s41467-019-11503-3
Miura, K., Ueno, S., Kamiya, K., Kobayashi, J., Matsuoka, H., Ando, K., et al. (1996). Cloning of mRNA sequences for two antibacterial peptides in a hemipteran insect, Riptortus clavatus. Zool. Sci. 13, 111–117. doi: 10.2108/zsj.13.111
Moran, N. A., Plague, G. R., Sandström, J. P., and Wilcox, J. L. (2003). A genomic perspective on nutrient provisioning by bacterial symbionts of insects. Proc. Natl. Acad. Sci. U.S.A. 100, 14543–14548. doi: 10.1073/pnas.2135345100
Moura, E. C., Baeta, T., Romanelli, A., Laguri, C., Martorana, A. M., Erba, E., et al. (2020). Thanatin impairs lipopolysaccharide transport complex assembly by targeting LptC–LptA interaction and decreasing LptA stability. Front. Microbiol. 11:909. doi: 10.3389/fmicb.2020.00909
Nikaido, H. (2003). Molecular basis of bacterial outer membrane permeability revisited. Microbiol. Mol. Biol. Rev. 67, 593–656. doi: 10.1128/MMBR.67.4.593-656.2003
Oppenheim, J., Biragyn, A., Kwak, L., and Yang, D. (2003). Roles of antimicrobial peptides such as defensins in innate and adaptive immunity. Ann. Rheum. Dis. 62, (Suppl. 2) ii17–ii21. doi: 10.1136/ard.62.suppl_2.ii17
Park, K.-E., Jang, S. H., Lee, J., Lee, S. A., Kikuchi, Y., Seo, Y.-S., et al. (2018). The roles of antimicrobial peptide, rip-thanatin, in the midgut of Riptortus pedestris. Dev. Comp. Immunol. 78, 83–90. doi: 10.1016/j.dci.2017.09.009
Powers, J.-P. S., and Hancock, R. E. (2003). The relationship between peptide structure and antibacterial activity. Peptides 24, 1681–1691.
Raetz, C. R., and Whitfield, C. (2002). Lipopolysaccharide endotoxins. Annu. Rev. Biochem. 71, 635–700.
Ratcliff, W. C., Kadam, S. V., and Denison, R. F. (2008). Poly-3-hydroxybutyrate (PHB) supports survival and reproduction in starving rhizobia. FEMS Microbiol. Ecol. 65, 391–399. doi: 10.1111/j.1574-6941.2008.00544.x
Reboul, C. F., Whisstock, J. C., and Dunstone, M. A. (2016). Giant MACPF/CDC pore forming toxins: a class of their own. Biochim. Biophys. Acta Biomembr. 1858, 475–486. doi: 10.1016/j.bbamem.2015.11.017
Rosado, C. J., Kondos, S., Bull, T. E., Kuiper, M. J., Law, R. H., Buckle, A. M., et al. (2008). The MACPF/CDC family of pore-forming toxins. Cell. Microbiol. 10, 1765–1774.
Sinha, S., Zheng, L., Mu, Y., Ng, W. J., and Bhattacharjya, S. (2017). Structure and interactions of a host defense antimicrobial peptide thanatin in lipopolysaccharide micelles reveal mechanism of bacterial cell agglutination. Sci. Rep. 7:17795. doi: 10.1038/s41598-017-18102-6
Taguchi, S., Kuwasako, K., Suenaga, A., Okada, M., and Momose, H. (2000). Functional mapping against Escherichia coli for the broad-spectrum antimicrobial peptide, thanatin, based on an In Vivo monitoring assay system. J. Biochem. 128, 745–754. doi: 10.1093/oxfordjournals.jbchem.a022811
Taniguchi, M., Ochiai, A., Kondo, H., Fukuda, S., Ishiyama, Y., Saitoh, E., et al. (2016). Pyrrhocoricin, a proline-rich antimicrobial peptide derived from insect, inhibits the translation process in the cell-free Escherichia coli protein synthesis system. J. Biosci. Bioeng. 121, 591–598. doi: 10.1016/j.jbiosc.2015.09.002
Vallet-Gely, I., Lemaitre, B., and Boccard, F. (2008). Bacterial strategies to overcome insect defences. Nat. Rev. Microbiol. 6, 302–313. doi: 10.1038/nrmicro1870
Vetterli, S. U., Zerbe, K., Müller, M., Urfer, M., Mondal, M., Wang, S.-Y., et al. (2018). Thanatin targets the intermembrane protein complex required for lipopolysaccharide transport in Escherichia coli. Sci. Adv. 4:eaau2634. doi: 10.1126/sciadv.aau2634
Washizaki, A., Yonesaki, T., and Otsuka, Y. (2016). Characterization of the interactions between Escherichia coli receptors, LPS and OmpC, and bacteriophage T4 long tail fibers. MicrobiologyOpen 5, 1003–1015. doi: 10.1002/mbo3.384
Wu, G., Wu, H., Li, L., Fan, X., Ding, J., Li, X., et al. (2010). Membrane aggregation and perturbation induced by antimicrobial peptide of S-thanatin. Biochem. Biophys. Res. Commun. 395, 31–35. doi: 10.1016/j.bbrc.2010.03.107
Keywords: Riptortus pedestris, Burkholderia insecticola, symbiosis, thanatin isoforms, multiple precursor proteins
Citation: Lee J, Cha WH and Lee D-W (2022) Multiple Precursor Proteins of Thanatin Isoforms, an Antimicrobial Peptide Associated With the Gut Symbiont of Riptortus pedestris. Front. Microbiol. 12:796548. doi: 10.3389/fmicb.2021.796548
Received: 17 October 2021; Accepted: 10 December 2021;
Published: 05 January 2022.
Edited by:
Chih-Horng Kuo, Institute of Plant and Microbial Biology, Academia Sinica, TaiwanReviewed by:
Rosario Gil, University of Valencia, SpainLaura V. Flórez, University of Copenhagen, Denmark
Copyright © 2022 Lee, Cha and Lee. This is an open-access article distributed under the terms of the Creative Commons Attribution License (CC BY). The use, distribution or reproduction in other forums is permitted, provided the original author(s) and the copyright owner(s) are credited and that the original publication in this journal is cited, in accordance with accepted academic practice. No use, distribution or reproduction is permitted which does not comply with these terms.
*Correspondence: Junbeom Lee, d2luZDIwMDBuZXRAa3MuYWMua3I=; Dae-Weon Lee, ZGFld2VvbmxlZUBrcy5hYy5rcg==