- 1Host-Pathogen Interaction Laboratory, Microbiology and Mycology Program, ICBM, Faculty of Medicine, University of Chile, Santiago, Chile
- 2School of Medicine, Faculty of Medicine, University of Chile, Santiago, Chile
- 3Department of Pediatrics and Pediatric Surgery, Dr. Luis Calvo Mackenna Hospital, Faculty of Medicine, University of Chile, Santiago, Chile
- 4Department of Pediatrics and Pediatric Surgery, Dr. Roberto del Río Hospital, Faculty of Medicine, University of Chile, Santiago, Chile
- 5Microbiology and Mycology Program, ICBM, Faculty of Medicine, University of Chile, Santiago, Chile
- 6Millennium Institute on Immunology and Immunotherapy, Faculty of Medicine, University of Chile, Santiago, Chile
- 7ANID - Millennium Science Initiative Program – Millennium Nucleus in the Biology of Intestinal Microbiota, Santiago, Chile
Gut microbiota composition during the first years of life is variable, dynamic and influenced by both prenatal and postnatal factors, such as maternal antibiotics administered during labor, delivery mode, maternal diet, breastfeeding, and/or antibiotic consumption during infancy. Furthermore, the microbiota displays bidirectional interactions with infectious agents, either through direct microbiota-microorganism interactions or indirectly through various stimuli of the host immune system. Here we review these interactions during childhood until 5 years of life, focusing on bacterial microbiota, the most common gastrointestinal and respiratory infections and two well characterized gastrointestinal diseases related to dysbiosis (necrotizing enterocolitis and Clostridioides difficile infection). To date, most peer-reviewed studies on the bacterial microbiota in childhood have been cross-sectional and have reported patterns of gut dysbiosis during infections as compared to healthy controls; prospective studies suggest that most children progressively return to a “healthy microbiota status” following infection. Animal models and/or studies focusing on specific preventive and therapeutic interventions, such as probiotic administration and fecal transplantation, support the role of the bacterial gut microbiota in modulating both enteric and respiratory infections. A more in depth understanding of the mechanisms involved in the establishment and maintenance of the early bacterial microbiota, focusing on specific components of the microbiota-immunity-infectious agent axis is necessary in order to better define potential preventive or therapeutic tools against significant infections in children.
Introduction
Development in molecular microbiology techniques, sequencing platforms and bioinformatics during the past decade have allowed us to expand our knowledge on microbiota composition, dynamics, and impact on human health and disease. We now know that the most abundant and diverse human microbiota niche is harbored in the gastrointestinal tract. Moreover, during the first 5 years of childhood, the gut microbiota (GM) undergoes major changes as it progresses toward an “adult-like, more stable community.” This GM plays a role in the development of the enteric immune and nervous systems, nutrient metabolism, and interactions with infectious agents, among other functions. The GM can affect the occurrence and course of systemic diseases, not only during childhood, but also later in life, including autism spectrum disorders (Li et al., 2019), attention deficit hyperactivity disorder (Boonchooduang et al., 2020), asthma and allergies (Kim et al., 2019), obesity (Muscogiuri et al., 2019), and autoimmune disorders (Russell et al., 2019), among others. For better comprehension we include Table 1 with basic terms needed for a proper understanding of the topics discussed in this review.
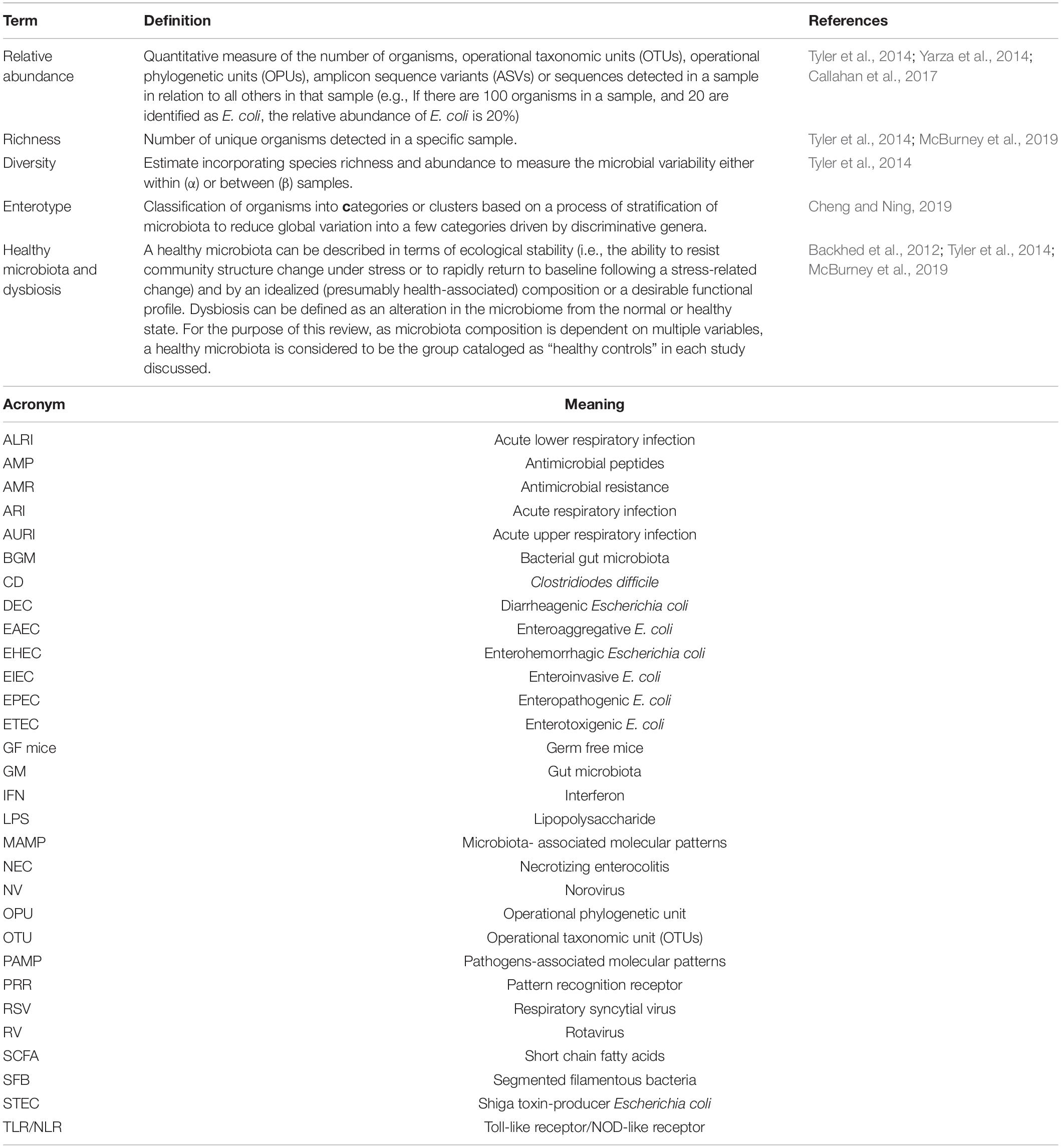
Table 1. Definition of relevant terms included in microbiota studies, and acronyms used in this review.
Though bacteria dominate gut microbial communities, GM is also composed of viruses, fungus, parasites and archaea, denominated Virome, Mycobiome, Parasitome, and Archaeome, respectively (Vemuri et al., 2020). Methodological difficulties associated with their study (including lack of updated bioinformatic tools and reference databases) have most likely downplayed our understanding of their true role in GM. Recent advances in next generation sequencing technologies have reflected on increasing literature, especially on gut virome development from childhood to adulthood and its role in gut homeostasis and immune-related diseases including childhood infectious diseases, as extensively reviewed (Tiew et al., 2020; Vemuri et al., 2020; Fulci et al., 2021; Townsend et al., 2021; Wu et al., 2021). Briefly, adult gut virome is mainly composed by bacteriophages, and in lower proportion by DNA and RNA eukaryotic viruses. Development of virome during childhood is dynamic and occurs in a stepwise manner, with initial colonization of bacteriophages during the first weeks of life (after pioneer bacteria colonization in the newborn gut) (Liang et al., 2020), a progressive increase of eukaryotic viruses during first 2 years of life (Lim et al., 2015) together with an expansion of bacterial abundance and richness, and a later predominance of bacteriophages toward an adult-like composition (Gregory et al., 2020). Gut mycobiome (Strati et al., 2016), and archaeome (van de Pol et al., 2017; Pausan et al., 2019) composition in children have been explored, but their trajectories during the first years of life are not deeply understood at the moment. For parasites, some studies have evaluated the presence of commensal parasites in children and their effects on bacterial GM (BGM) composition (Alzate et al., 2020), but an exhaustive “omic” approach to understand the parasitome relevance during childhood is still needed.
This review focuses on the dynamics of BGM during childhood, and their relationships with the most common gastrointestinal and respiratory infections, as well as with two well identified dysbiosis-related diseases: necrotizing enterocolitis (NEC) and Clostridioides difficile infection.
Bacterial Gut Microbiota Dynamics and Modulation During the First 5 Years of Life
Human gut bacterial communities in healthy adults are highly diverse, with each individual harboring over 100 trillion bacteria, belonging to over 150 different species. The BGM during adulthood is dominated by the phyla Firmicutes (e.g., Lactobacillus, Bacillus, and Clostridioides) and Bacteroidetes (e.g., Bacteroides), with lower abundances of Proteobacteria (e.g., Escherichia) and Actinobacteria (e.g., Bifidobacterium) (Arumugam et al., 2011; Rinninella et al., 2019). The establishment of this “mature microbiota” begins during early childhood and is influenced by prenatal and postnatal factors (Roswall et al., 2015; Chu et al., 2019).
Several studies have questioned whether the in-uterus environment is sterile. The presence of bacteria in amniotic fluid originating from both the maternal genitourinary tract and oral cavity was initially demonstrated in preterm infants, suggesting that ascending (from the genitourinary tract) and hematogenous (from the oral cavity) bacterial dissemination causes intra-amniotic colonization/infection (Romero et al., 2014). There is additional conflicting evidence, as some studies report the detection of bacteria in the placenta (Aagaard et al., 2014), amniotic fluid (Rautava et al., 2012) and the umbilical cord (Jimenez et al., 2005) in women with healthy term pregnancies, as well as in the meconium of term newborns (Su et al., 2018), while other studies report a sterile in-uterus environment in this context (Lim et al., 2018; Rehbinder et al., 2018). Thus, the role of “in utero” colonization is currently uncertain, this may in part be due to the lack of evidence of bacterial viability beyond detection of bacterial DNA (Perez-Munoz et al., 2017).
Delivery mode is a major determinant of BGM composition during early infancy. Vaginal delivery is followed by newborn gut colonization with the mother’s vaginal microbiota, mainly composed of Prevotella and Lactobacillus spp. (Dominguez-Bello et al., 2010), while the microbiota of infants delivered through cesarean section resembles that of the mother’s skin microbiota, and of the nosocomial environment (Dominguez-Bello et al., 2010; Shao et al., 2019). These differences are most distinct during the first 6 months of life (Rutayisire et al., 2016).
Breastfeeding is another key factor in determining the microbiota composition during early childhood (Ho et al., 2018; Ossa et al., 2018). A recent study showed that receiving breast milk (either exclusive or partial) was the most significant factor associated with gut microbiota structure from months 3 to 14 of life, and was associated with the presence of Bifidobacterium species and the species Lactobacillus rhamnosus and Staphylococcus epidermidis (Stewart et al., 2018). Both Bifidobacterium and Lactobacillus spp. are present in human milk and S. epidermidis colonize maternal skin; and their metabolic functions in falta una palabra aqui are related to breastfeeding, as Bifidobacterium species metabolize human milk oligosaccharides (HMOs) (James et al., 2019) and Lactobacillus species including L. rhamnosus can metabolize lactose (Lubiech and Twaruzek, 2020). Cessation of breastfeeding seems to be the major determinant in the “maturation” of the gut microbiota, characterized by an increase in Firmicutes (Stewart et al., 2018), concordant with other studies showing an increase specifically in the Lachnospiraceae and Ruminococcaceae families (Laursen et al., 2016), which can metabolize plant-derived complex carbohydrates introduced with solid foods (Flint et al., 2012). After 6 months of age, the composition of complementary diet also determines the BGM evolution, e.g., the progression to a higher protein and fiber diet is associated with an increase in microbial α-diversity (Laursen et al., 2016).
Overall, a history of breastfeeding seems to be more influential than the mode of delivery in determining long-term BGM composition, e.g., the lack of breastfeeding during infancy is associated with higher degrees of the genus Bacteroides in stool during childhood and adolescence (Cioffi et al., 2020). However, the impact of these findings on a child’s clinical outcomes is not entirely elucidated.
Maternal diet may also influence the composition of infant gut-microbiota during the first 6 weeks of life, especially in the case of a high-fat maternal diet (Chu et al., 2016), fruit intake and dairy intake (Lundgren et al., 2018; Maher et al., 2020). Maternal diet is also associated with changes in mother’s milk microbiota, which may influence the BGM in the child (Babakobi et al., 2020). Overall, these changes in BGM composition reflect a gradual functional specialization in parallel to the nutritional changes over time, in order to deal with the substrates provided by changing diets, as extensively reviewed by other authors (Derrien et al., 2019).
Geographic differences in BGM composition during the first years of life have been described, most likely due in part to differences in diet and socioeconomic status; e.g., 6-month-old children from Malawi have increased abundance of Bifidobacterium group, Bacteroides-Prevotella group, and Clostridium histolyticum group compared to children from Finland (Grzeskowiak et al., 2012); while 1–6 years old children from Burkina Faso have enrichment in Bacteroidetes and depletion in Firmicutes compared to European children (De Filippo et al., 2010). African children have a diet based primarily on fiber and complex carbohydrates, which results in higher levels of fecal short-chain fatty acids (SCFA), likely reflecting the ability of their microbiota to degrade these complex carbohydrates. Conversely, European children have a characteristically “western diet” (high in animal protein, sugar, starch, fat, and low in fiber), associated with low fecal SCFA levels; thus they develop a BGM more suited to metabolize simple carbohydrates, animal fat and protein (De Filippo et al., 2017). These differences suggest a tight relationship among BGM evolution, diet and functionality.
Exposure to antibiotics can modulate BGM composition from gestation onwards. The use of intrapartum antibiotics for prophylaxis against group B Streptococcus infection was associated with lower bacterial diversity, lower abundance of Actinobacteria and Bacteroidetes phyla; and higher abundance of Proteobacteria phylum and Enterobacteriaceae or Streptococcaceae families in the BGM of newborns, compared to those delivered by mothers not receiving antibiotic prophylaxis (Aloisio et al., 2016). These changes seem to be more prominent, lasting up to day 30 of life in breastfed infants (Mazzola et al., 2016). When the delivery mode is concomitantly analyzed, intrapartum antibiotic use is associated with a lower abundance of the genus Bacteroides and Parabacteroides and higher abundance of Enterococcus and Clostridium levels at 3 months of life irrespective of delivery mode; changes are more prominent and persist up to 12 months of life in children born by emergency cesarean section, especially in those who did not receive exclusive breastfeeding during first 3 months of life (Azad et al., 2016).
The use of systemic antibiotics during the neonatal period has been associated with disruption of normal microbiota colonization in both preterm and term newborns, with lower rates of commensal bacteria such as the genus Bifidobacterium (Tanaka et al., 2009) and phylum Bacteroidetes (Eck et al., 2020) and increased rates of potentially pathogenic bacteria such as the genus Enterobacter from the first weeks of life (Greenwood et al., 2014). Changes in BGM diversity and composition related to the early use of narrow-spectrum antibiotics persists up to 6 months of life (Tapiainen et al., 2019). In addition, perinatal antibiotic use is associated with increased antimicrobial resistance (AMR) genes in stools of both preterm and term newborns (Gibson et al., 2016; Tapiainen et al., 2019).
Throughout the first 3 years of life, children with repeated short courses of oral antibiotics (prescribed mainly for “respiratory infections”) have decreased BGM in terms of both bacterial species and strains, and increased variability in composition compared to children not receiving antibiotics (Yassour et al., 2016). Expectedly, detection of AMR genes increases after antibiotic intake; chromosomal genes increase rapidly and decrease after antibiotics are ceased, while some episomally codified AMR genes do not decrease until several months after antibiotic consumption (Yassour et al., 2016). Moreover, macrolides but not penicillin consumption has been associated with a distinct microbiota composition at the phylum level, characterized by an increased abundance of the phyla Bacteroidota and Proteobacteria, and decreased abundance of Actinobacteria (Korpela et al., 2016) in children 2–7 years old. In addition, macrolide use was associated with a long-term (12–24 months) reduction in microbial richness, but a transitory effect on macrolide resistance genes and culture-based macrolide resistance in the gut which increased immediately after macrolide intake and returned to low levels at 6–12 months (Korpela et al., 2016).
Interestingly, mass azithromycin distribution in biannual courses in Nigerian preschool children (1–59 months) reduced all-causes of mortality by 13.5% compared to placebo (Keenan et al., 2018), and the effect on mortality was higher in children 1–5 months of age (24.9% lower mortality). Microbiota analysis of these children showed that the relative abundances of two Campylobacter species (which cause diarrhea and are susceptible to azithromycin) along with another 33 gut bacteria were significantly reduced at 24-months of follow-up. This suggests a potential short-term benefit of macrolides in the control of enteric infectious diseases; however, macrolide resistance genes increased during the following 6 months (Doan et al., 2019).
In summary, normal BGM colonization begins in utero or during birth, and evolves during the first years of life influenced by several environmental and host factors which finally determine a BGM’s individual pattern. As recently described in a large Swedish cohort, BGM progression during this period is characterized by increasing α-diversity as children get older, with major shifts in composition between 4 and 12 months, resembling a more adult microbiota as the children reach 3–5 years of age (Roswall et al., 2021). Based on this cohort of children, four characteristic trajectories were defined based on relative abundance of single genera at different time points: (1) a first peak at 4 months of Bifidobacterium, Enterococcus, Streptococcus, Lactobacillus and Enterobacteriaceae; (2) a second peak at 12 months, mainly in Ruminococcus abundance; (3) a rapid increase in Bacteroides between 4 and 12 months of age and relative stability after 3 years of age; and (4) an increase in Methanobrevibacter, Desulfovibrio, Bilophila, and some Clostridia after 12 months followed by additional increases after 3 years. Noticeably, despite the fact that these major and critical changes are observed during these first years of life, at 5 years of age the childhood BGM composition was still significantly different compared with their mothers and other unrelated adults (Roswall et al., 2021), suggesting that the BGM continues to evolve during childhood and future life. Whether these results are also observed in other geographical regions, remains to be seen.
Mechanisms Involved in Bacterial Gut Microbiota–Pathogen Interactions
The eubiotic BGM plays an important role in sustaining a “state of good health” as well as in modulating pathogenesis of several diseases, including susceptibility to infections and clinical outcomes after infection has occurred (Harris et al., 2017b; Gaufin et al., 2018; Libertucci and Young, 2019).
The pathophysiology behind BGM-infectious pathogen interactions is influenced by host factors. Two fundamental pathways are involved: one direct, mediated by microbiota interactions with non-commensal agents, and another indirect, via microbiota-mediated immune system modulation (Libertucci and Young, 2019).
Microbiota–Pathogen Interactions
Commensal bacteria limit (or enhance) pathogen colonization through direct bactericidal/bacteriostatic or stimulatory effects (by direct binding or mediated by metabolites), as well as through competition for nutrients and specific resources required for infection, in addition to modification of gut mucosa sugars and/or receptors. Key factors involved in microbiota–pathogen interactions are summarized in Table 2.
Microbiota-Mediated Immune System Modulation
Experiments in germ-free (GF) mice have provided significant information on the role of the BGM in the development and functionality of the immune system, as summarized in Table 3. The microbiota and key components of the innate and adaptive immune system interact in a bidirectional manner, e.g., the BGM composition determines the development of either effective or defective innate and adaptive immune system components, and, specific components of the immune system contribute to either maintain or break BGM homeostasis. Development of the BGM is related to immune system maturation, and the first weeks of life seem to be critical as evaluated in animal models. Based on the age-dependant effect of the BGM restoration in GF animals on several immune components (iNKT, Treg, IgE, and TLR signaling on epithelial cells), Gensollen et al. (2016) postulated the term “window of opportunity” related to the period during early infancy where BGM can still be modified, otherwise resulting in permanent immune alterations. In humans, this model could explain the association of BGM alterations during early childhood with future immune-mediated chronic diseases including allergy, asthma, undernutrition, or obesity and inflammatory bowel disease (Arrieta et al., 2014). In the following sections, we will analyze the association of BGM composition during this apparently critical period with common infections during childhood.
Gut Microbiota and Specific Gastrointestinal Infectious Diseases
Necrotizing Enterocolitis
Necrotizing enterocolitis is characterized by intestinal inflammation and necrosis that can progress to systemic infection, multiorgan failure, death (Tanner et al., 2015), and long-term neurological complications (Rees et al., 2007). Prematurity is the main risk factor, and additional factors include lack of human milk feeding (Cacho et al., 2017), meconium aspiration syndrome, postnatal asphyxia, congenital heart disease (Lu et al., 2017), and aberrant microbial colonization and infections (Neu and Pammi, 2018). Microorganisms that potentially play a role include: Gram-negative enteric bacteria (Escherichia coli, Klebsiella spp., and Pseudomonas aeruginosa), Gram-positive bacteria (Enterococcus, Staphylococcus aureus, and S. epidermidis), Viruses (rotavirus, norovirus, astrovirus, cytomegalovirus, and echovirus), and fungus (Candida spp.) (Coggins et al., 2015). Studies based on several different animal models have shown that key factors in NEC pathogenesis are immune response and the coexistence of multifactorial dysbiosis and an altered gut barrier. This alteration is characterized by an increased TLR4 response to gut bacteria, decreased AMPs and mucin production, and impaired production of certain growth factors and cytokines (epidermal growth factor, TGF-β and IL-10, among others). The result is intestinal bacterial translocation, a local and systemic pro-inflammatory response, and gut mucosal damage and necrosis (Tanner et al., 2015).
In humans, prematurity itself is related to an altered BGM development pattern compared with term infants. This altered development pattern is also influenced by other prematurity-related factors, e.g., gastrointestinal and immune system immaturity, early antibiotics use, long-term hospitalization, mechanical ventilation and parenteral nutrition (Tirone et al., 2019). In comparison to term infants, the preterm BGM displays an evolutionary pattern characterized by an initial predominance of the class Bacilli, followed by predominance of the class Gammaproteobacteria, and later on by Clostridia (La Rosa et al., 2014); in a minor taxonomic level, a progression in four phases characterized by dominance of Staphylococcus, Enterococcus, Enterobacter (Korpela et al., 2018) and finally Bifidobacterium genera, respectively (Korpela et al., 2018; Tauchi et al., 2019).
In preterm infants, patterns of BGM development differ between infants who develop NEC compared to healthy controls: with a higher abundance of Clostridia (mainly Clostridioides sensu stricto) among infants developing early-onset NEC (NEC onset <23 days of life), and of Gammaproteobacteria (E. coli and Shigella) in those developing late-onset NEC; in both groups, changes were observed beginning 6 days prior to NEC onset (Zhou et al., 2015). These changes reflect the absence of one common gut bacterial pattern associated with NEC, which varies according to the age of infants developing NEC. In another study, BGM in preterm infants developing NEC tended to be less diverse from days 17–22 postpartum, with a higher abundance of E. coli; while metagenomic analysis showed that detection of uropathogenic E. coli was a risk factor for both NEC and mortality (Ward et al., 2016). In cohorts of low birth weight infants (<1,500 g) followed by Warner et al. (2016) differences in BGM composition between children with NEC and controls were observed after 30 days postpartum, with increased proportions of Gammaproteobacteria and lower proportions of Negativicutes and the class Clostridia. Yet another study, reported similar overall diversity between preterm infants over 28 weeks of gestational age with NEC and preterm controls, albeit specific differences were observed with increased abundance of Propionibacterium among infants with NEC, while Lactobacillus, Phascolarctobacterium, and Streptococcus salivarius were more abundant in controls; in addition, functionality inference analysis showed that NEC cases had lower xenobiotic biodegradation and metabolic activity compared to controls, suggesting not only an altered composition pattern but also a functionally altered microbiota (Feng J. et al., 2019). In these infants, reduced xenobiotic detoxification by BGM may be related to an inflammatory response in the gut, as described in inflammatory bowel disease (Langmann et al., 2004). Modifications of the microbiota composition are observed not only in advanced NEC stages (Bell’s grades II–III), as in the above studies, but also in grade I stage with changes in the microbiota composition varying according to days of life: e.g., increased abundance of Streptococcus during the second 10-days of life, and Staphylococcus during the third 10-days of life compared to controls, and of Raoultella in NEC stage I cases during the second month of life (Brehin et al., 2020).
Altogether, current evidence suggests that the BGM composition is altered in preterm infants developing NEC compared to preterm controls, characterized largely by a less diverse microbiota, enrichment in certain components, especially Gammaproteobacteria, with a potential influence of specific bacterial components (uropathogenic E. coli) in disease outcome. Gram-negative bacteria in mouse models of hypoxia-induced NEC are associated with early histological damage (Carlisle et al., 2011), while members of Clostridia and Bifidobacteriales are producers of SCFA, which in physiological amounts are protective against enterocytes injury (Zheng et al., 2020). Thus, the described disbalance of these BGM components in preterm infants could partially explain NEC development. BGM composition in environments such as NICUs is affected by various factors, e.g., by antibiotic use more than by disease states such as NEC or sepsis itself (Wandro et al., 2018). Large, prospective studies are required to clarify the specific timing at which BGM dysbiosis is initiated, in order to potentially modulate the BGM in order to increase protective bacteria or limit expansion of pathogenic bacteria before NEC onset.
Rotavirus and Norovirus Infections
Diarrheal disease is an important cause of mortality in children under 5 years of age in low-income countries, and an important cause of medical resource utilization in middle-high income countries (Florez et al., 2020). The leading causes of diarrhea in children are viruses (70–90%). Historically RV was the leading cause of viral diarrhea in children, however, following the introduction of RV vaccines, norovirus (NV) has become the primary cause of moderate-severe diarrhea in several of those countries (Hemming et al., 2013; Bucardo et al., 2014; McAtee et al., 2016). Viruses produce diarrhea through several mechanisms, including damage or death of epithelial cells, altered epithelial absorption, intestinal hypermotility by effects on the enteric nervous system (Crawford et al., 2017), and secretion mediated by viral factors, such as the rotavirus non-structural protein NSP4 (Beau et al., 2007). Immune response to enteric viral infections is mediated by both the innate immune system (including PAMPs-PRR interaction, antiviral IFN-I and II responses) (Villena et al., 2016) and adaptive immunity, including humoral responses (both local IgA and systemic IgM/IgG responses) (Blutt et al., 2012) and cellular responses (LTCD8, LTh1, LTh2, and LTreg) (Malm et al., 2019; Schorer et al., 2020); although the role of cellular response has been less characterized. Studies in animal models have provided insights on the interaction of enteric pathogens and the BGM. RV infection altered the BGM in neonatal-mice, with a time-dependent decrease of Lactobacillus and an increase in the mucin-degrading bacteria Bacteroides and Akkermansia associated with an increased glycan availability in the ileum; yet no changes were found in other intestinal segments (Engevik et al., 2020). Glycan degradation by these bacteria decreases RV decoy-binding to mucins, suggesting that changes in BGM promote RV infection by affecting the protective role of mucus (Engevik et al., 2020). A recent study in neonatal germ-free piglets transplanted with a human child’s microbiota reported bidirectional interactions between the BGM and RV infection, e.g., colonized piglets had reduced RV-induced diarrhea and viral shedding compared to germ-free controls, and RV infection caused alterations in the BGM (Kumar et al., 2018). In addition, a recent study showed that spontaneous resistance to RV infection in mouse colonies was determined by specific BGM composition, specifically by segmented filamentous bacteria, which increase epithelial turnover, protecting against infection (Shi et al., 2019). However, a previous study showed that BGM ablation through antibiotic treatment in mice delayed RV infection and reduced infectivity in mice, enhancing specific antiRV humoral immunity (Uchiyama et al., 2014). NV infection can also alter the BGM in mice, increasing the Bacteroidetes/Firmicutes ratio (Hickman et al., 2014), although this finding has not been consistent (Nelson et al., 2013). On the other hand, antibiotic-induced gut dysbiosis prevents persistent NV infection in mice (Baldridge et al., 2015), which suggests a crucial role of the BGM in promoting NV infection.
The above findings lead to the conclusion that the BGM has bidirectional interactions with RV and NV infections, where BGM composition can either protect against or predispose the host to infection; an infection which in turn can alter the BGM.
While several studies have evaluated BGM composition during acute diarrhea in children (Supplementary Tables 1, 2), there is limited evidence for BGM during diarrheal disease caused specifically by RV and NV in children. A reduction of BGM α-diversity, considered broadly as an “unhealthy microbiota,” was observed in children suffering from RV and NV diarrhea episodes compared to healthy controls (Chen et al., 2017; Xiong et al., 2021); this reduction was more prominent in RV-caused diarrheas (Chen et al., 2017). However, an increase in bacterial abundance (Chao1 index) was found in NV-caused diarrheas compared to healthy controls (Xiong et al., 2021). This is concordant with studies in animal models, where NV requires the presence of determined components of BGM to produce infection, while dysbiosis (e.g., loss of diversity) predisposes the host to RV infection in most studies. Cohort studies in children are needed to assess if these differences in the BGM under stable conditions, differentially predispose the host to either RV or NV infection, and whether these BGM variations persist during diarrhea episodes as observed in cross-sectional studies. RV diarrhea episodes tend to be more severe than those caused by NV (Chen et al., 2017; Xiong et al., 2021), which has also been correlated with decreased α -diversity compared to mild diarrhea episodes (Chen et al., 2017). Beyond diversity, BGM composition is consistently altered during episodes of RV and NV diarrhea compared to healthy controls, but which specific taxa are increased or decreased varies among studies (details in Tables 4, 5). RV-caused diarrhea episodes tend to have a predominance of Bifidobacterium, Streptococcus, Enterococcus, and Lactobacillus at the genus level compared to healthy controls, and NV-caused diarrhea episodes have a predominance of Streptococcus and Enterococcus compared to healthy controls (Xiong et al., 2021). Enterococcus can bind to NV in vitro and predispose the host to infection (Almand et al., 2017); thus the predominance of this taxa during NV-caused diarrhea may indicate a previous predisposition to infection. Interestingly, Bifidobacterium and Lactobacillus are widely used as probiotics to restore BGM composition, reduce the duration of diarrhea episodes, and to promote an antiviral immune response (Lee et al., 2015); an increase in these “beneficial” bacteria may facilitate restoration of gut homeostasis in RV-induced diarrhea. In linear discriminant effect-size analysis [which aims to determine those taxa that explain the differences between microbial communities (Segata et al., 2011)] – Bacillus spp. was the most characteristic taxa in both RV and NV-caused diarrheas compared to healthy controls (Xiong et al., 2021). Bacillus spp. are spore-forming bacteria with probiotic properties which reduce duration of diarrhea in children (Ianiro et al., 2018). In a cellular model of RV infection, a mix of Bacillus clausii strains prevented RV-induced epithelial cell barrier disruption and inhibited the expression of proinflammatory cytokines including IFN-Beta (Paparo et al., 2020). In murine NV infection, the use of Poly-gamma-glutamic acid (γ-PGA) – an extracellular polypeptide produced by Bacillus species- induced a systemic and intestinal IFN-beta response without inducing other proinflammatory cytokines, which was associated with later prevention of NV infection (Lee et al., 2018). Thus, the enrichment of this genus during diarrhea episodes in children may reflect a protective self-regulatory response, if this impacts on immune response and clinical outcome, or if there are different effects on RV and NV- caused diarrheas remains unknown.
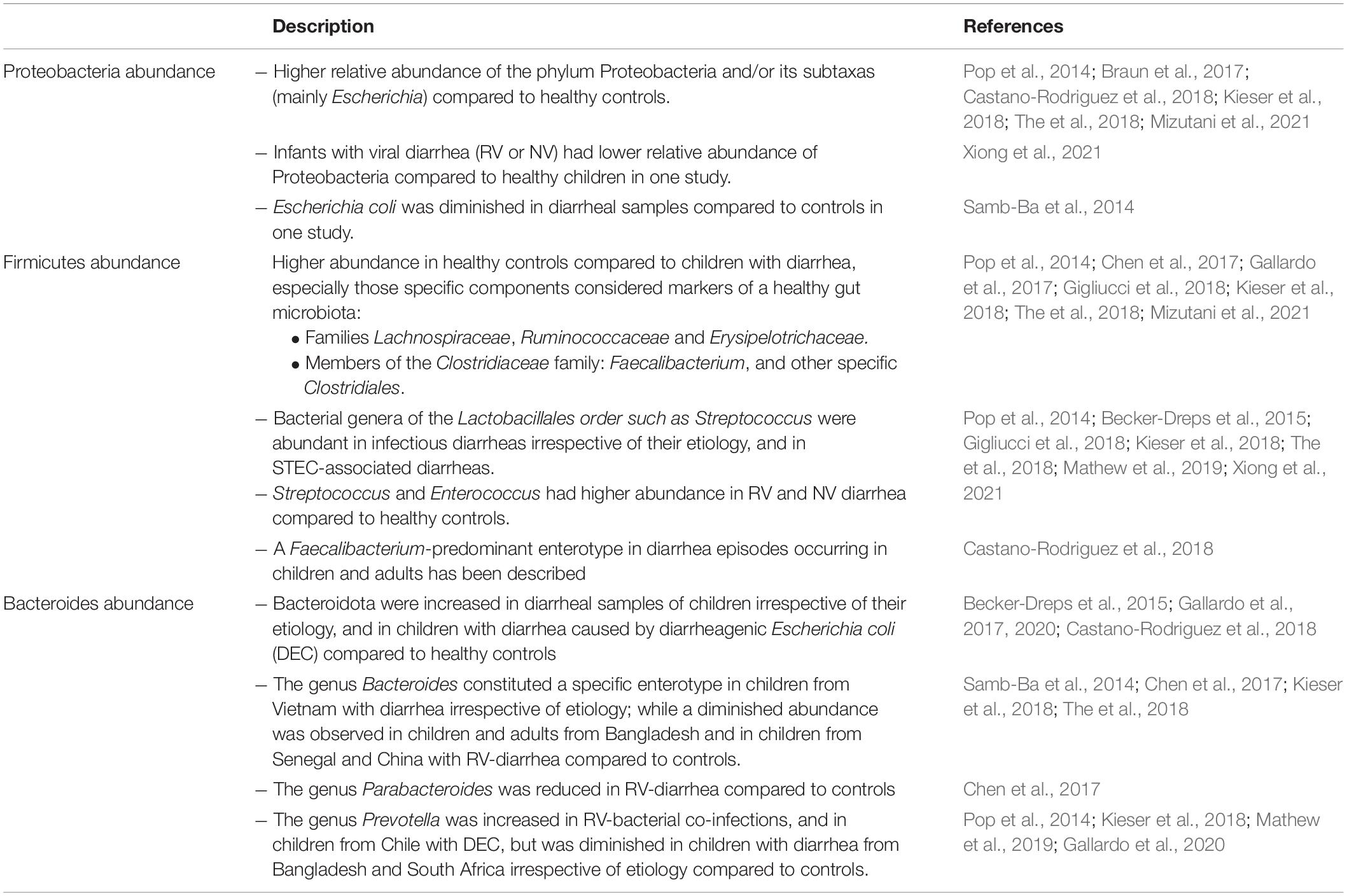
Table 4. Abundance of the gut microbiota’s main phyla and their components during diarrhea episodes compared to healthy controls.
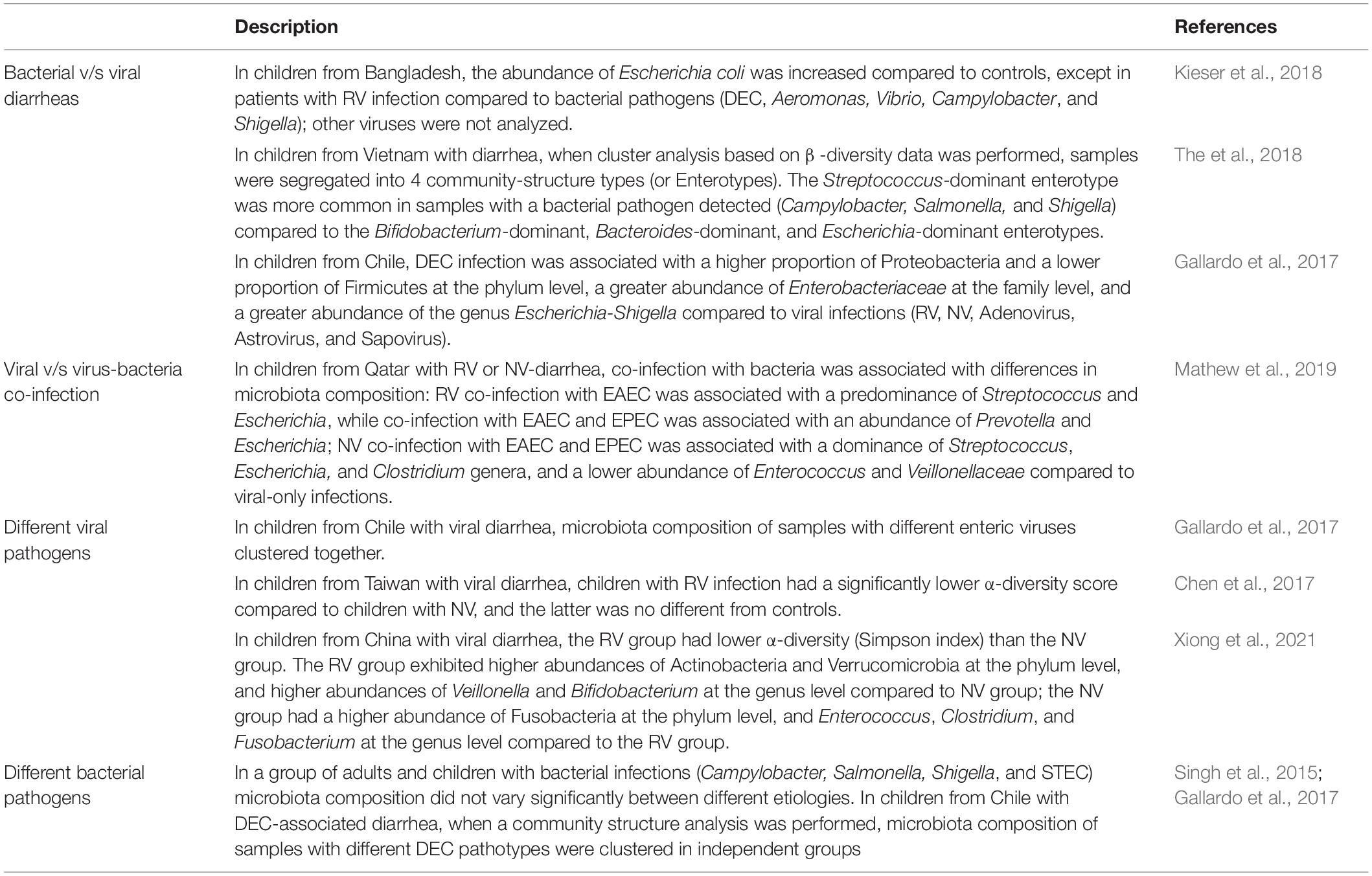
Table 5. Differences in the gut microbiota composition during childhood infectious diarrhea according to etiology.
Clinical manifestations related to viral diarrhea are also associated with specific BGM taxa, e.g., abdominal pain was related to the abundance of Prevotella (Chen et al., 2017), which is associated with a proinflammatory response in several chronic diseases (Larsen, 2017), but also to a lack of effective response to the RV vaccine (Harris et al., 2017a); convulsions were related to a substantial decrease in Faecalibacterium (Chen et al., 2017), a SCFA producer important in the maintenance of regulatory intestinal immunity (Alameddine et al., 2019). Whether specific BGM components influence clinical outcomes in viral diarrhea episodes through immune mechanisms, as postulated here, remains to be clarified. Functional predictions of the BGM in RV and NV diarrhea episodes showed an increase in chloroplast and photosynthesis pathways compared to healthy controls, which may be explained by incomplete BGM-dependant digestion of plant food in the gut during diarrhea (Xiong et al., 2021).
Enteric Bacterial Infections With a Focus on Diarrheagenic E. coli
Bacteria cause approximately 10–20% of diarrhea episodes in children, of which Shigella, Salmonella, Campylobacter, and enterotoxigenic E. coli (ETEC) predominate; less common causes include enteroinvasive E. coli (EIEC) (Florez et al., 2020). Shiga-toxin producing E. coli (STEC) is particularly important in children, as it is the most common cause of hemolytic uremic syndrome (HUS) (Exeni et al., 2018). Bacteria produce diarrhea through both common and specific mechanisms: inflammation occurs during infections with cytotoxin-producing bacteria (Shigella and STEC) and in the case of bacteria that invade and disrupt intestinal mucosa (Salmonella, Campylobacter). This leads to inflammation and necrosis of the epithelium and sub epithelium microabscesses (Florez et al., 2020). Secretory diarrhea occurs when bacteria producing toxins increase intracellular cAMP or cGMP levels (e.g., Vibrio cholerae and ETEC) (Thiagarajah et al., 2015). Interaction between the BGM and bacterial enteric pathogens has been evaluated in animal models, with Citrobacter rodentium infection widely used to mimic human diarrheagenic E. coli in mice. C. rodentium induces time-dependant changes in BGM with a rapid decrease in colonic Mucispirillum during the early phases, and increases in members of the Clostridiales and Lactobacillales families followed by successful resolution of colitis (Belzer et al., 2014). On the other hand, the BGM (butyrate-producing bacteria) appear to be essential in protecting against C. rodentium infection (Wlodarska et al., 2011; Osbelt et al., 2020), again demonstrating the bidirectional interaction between enteric bacterial pathogens and the BGM.
In children with DEC-caused diarrhea, changes in BGM composition during bacterial pathogen infections are characterized by an increase in Proteobacteria and a decrease in Firmicutes (Gallardo et al., 2017), also observed in other bacterial pathogens in children and adults along with a decrease in α-diversity (Singh et al., 2015). Increases in Proteobacteria can be partially explained by an increase in Escherichia/Shigella species as the cause of diarrhea, but also by other members of Enterobacteriaceae, such as Citrobacter and Enterobacter (Gallardo et al., 2017). These changes in BGM composition are associated with specific metabolic functions, as DEC samples display enrichment in pathways involved in histidine degradation, while healthy controls were enriched in L-ornithine and L-histidine biosynthesis pathways, correlating with higher levels of histamine (an histidine-degradation product) and lower levels of ornithine, explained mainly by the presence of Enterobacter hormaechei, Citrobacter werkmanii/freundii, Shigella spp., and Bifidobacterium stercoris (Gallardo et al., 2020). Histamine production is induced by proinflammatory environments and associated with E. coli adherence, while ornithine is associated with a healthy microbiota and the maintenance of an unscathed intestinal barrier; these findings suggest that microbiota metabolites may be related to DEC pathogenesis (Gallardo et al., 2020). Whether this determines the clinical impact of infection needs to be explored further. The decrease in Firmicutes during STEC-caused diarrhea episodes is primarily explained by decreases in Clostridiales (including the genera Lachnospiraceae and Ruminococcaceae) compared to healthy controls (Gigliucci et al., 2018). This occurs alongside a decrease in Bifidobacteriales, all recognized as SCFA producers, metabolites which can modulate the expression of flagellin, chemotaxis proteins and adhesins either promoting or limiting pathogenicity depending on SCFA concentrations in the intestinal lumen (Tobe et al., 2011; Navarro-Garcia, 2014; Lackraj et al., 2016). Whether the reduction of these bacteria during STEC-diarrhea is accompanied by changes in SCFA levels, and how this may influence the immune response and clinical outcomes in children remains undetermined.
In comparison to viral only infections, co-infection with DEC is associated with higher clinical severity scores (Mathew et al., 2019) and with changes in BGM composition, which is dependant on the DEC pathotype, e.g., RV + EAEC co-infection is associated with higher Streptococcus abundance, which is not observed in RV + EPEC co-infections. Interestingly, an increase of the potentially beneficial Bifidobacterium, previously described in viral diarrheas, is more marked in virus-DEC co-infections (Mathew et al., 2019) which have higher clinical severity scores. This indicates that BGM changes during diarrhea episodes are variable between studies, and it is important to assess BGM composition separately in different diarrheal etiologies, as the role of the BGM during acute diarrhea seems to be pathogen-dependent.
Thus far, we have summarized studies in children focused on specific pathogens. In Supplementary Table 2 we describe studies that do not determine diarrhea etiology, and/or do not clearly separate their analysis according to etiology or age groups. Considering the global data, we can conclude that the BGM composition is altered during episodes of infectious diarrhea in children, characterized by decreased α-diversity and modifications in predominant bacteria, with an increase in Proteobacteria (mainly Escherichia) and a decrease in Firmicutes, while changes in Bacteroides and other groups are variable (Figure 1 and Table 4). In addition, the etiologic agent is associated with differences in BGM composition, e.g., bacterial only vs. virus-bacteria co-infections tend to display different microbiota patterns compared to those caused by viruses in the absence of bacteria (Table 5). Overall microbiota recovery toward a “healthy-status microbiota” begins several weeks after diarrhea resolution (Becker-Dreps et al., 2015; Singh et al., 2015), which is influenced by the etiologic agent and other potential but less explored factors, such as diarrhea severity, social context, and age.
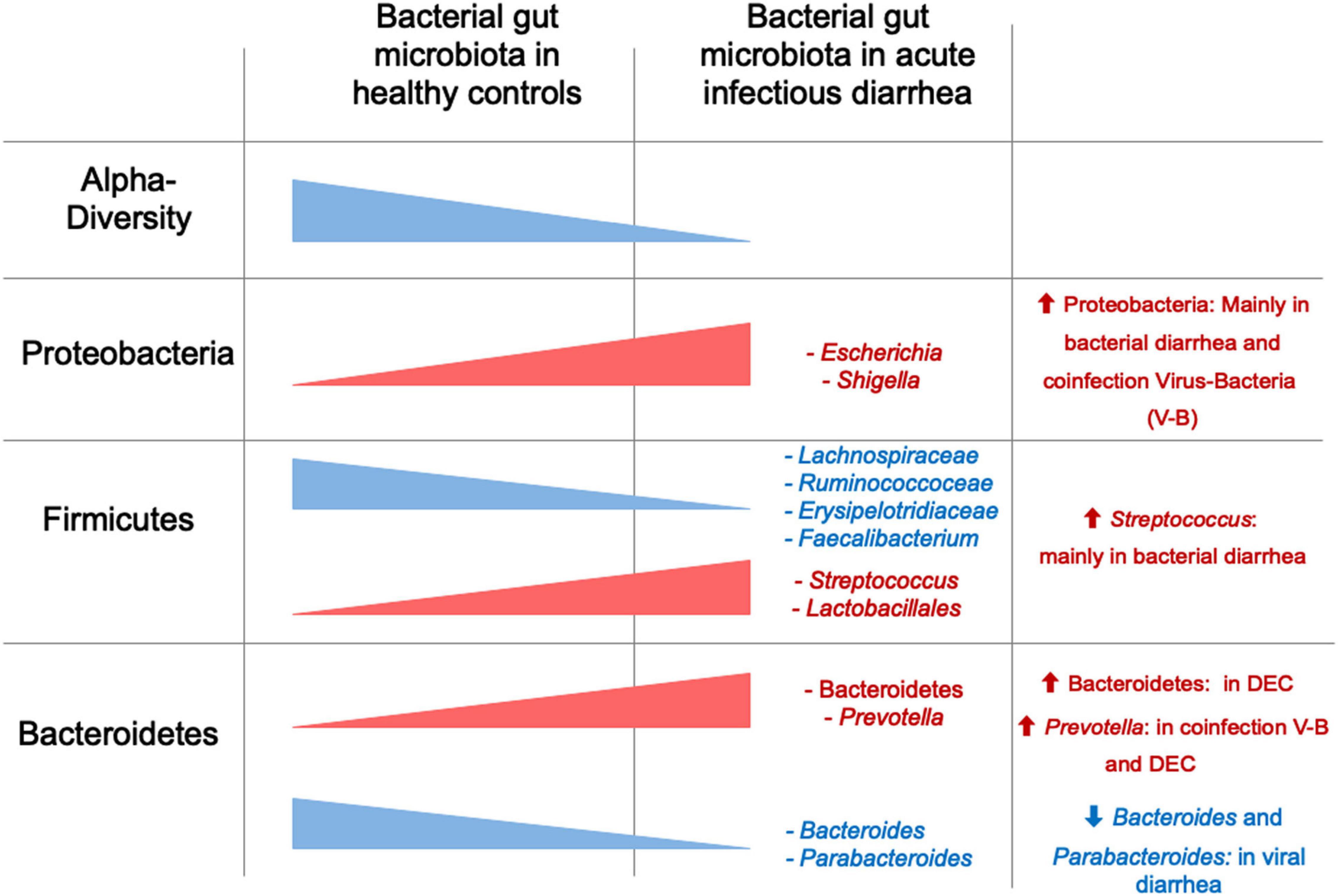
Figure 1. α-diversity and relative abundance of main taxa in children with acute diarrhea compared to healthy controls. Red represents higher diversity or abundance in children with diarrhea, and blue represents higher values in healthy controls.
Clostridioides difficile Infection
Clostridioides difficile is present in the gut microbiota of around 30% of healthy infants <1 month of age; this decreases to approximately 10% at 12 months and 0–3% at 3 years of age (Jangi and Lamont, 2010). Why infants with CD in their BGM do not develop disease is unknown. Studies in newborn rabbits suggest that the lack of toxin receptors on the surface of epithelial cells may play a role (Eglow et al., 1992). In certain situations CD can cause mild to severe disease, especially in children suffering a malignancy, recent surgery, antibiotic exposure, or solid organ transplantation (Nicholson et al., 2015; Anjewierden et al., 2019). These situations may lead to characteristic disruptions in the BGM and the absence of an efficient immune response to CD-infection (Buonomo and Petri, 2016). The pathogenicity of CD is attributed to its production of the toxins A (enterotoxin) and B (cytotoxin), which inactivate GTP-binding proteins, causing cytoskeleton disruption and apoptosis of colonic cells (Abt et al., 2016). Disruption of the epithelial barrier causes bacterial translocation from the gut and access to other tissues. This disruption and translocation activates an inflammatory response (pro-inflammatory cytokines, chemokines, recruitment of innate and adaptive immune cells, reactive oxygen species production) that can limit the systemic spread of CD (Abt et al., 2016). However, an exaggerated inflammatory response can be detrimental in exacerbating epithelial damage and increasing the severity and duration of disease, as shown in mouse models (Buonomo et al., 2013). Animal models of CD infection have been constructed dominantly of mice treated with antibiotics, which causes gut dysbiosis characterized by a decrease in richness, a decrease in Bacteroidetes and an increase in Proteobacteria or Enterobacteriaceae (Theriot and Young, 2015), with specific differences between studies possibly related to the administration of different antibiotics. These changes in the BGM are related to susceptibility to CD-caused disease and the restoration of this microbiota using specific strains e.g., a mix of Staphylococcus warneri, Enterococcus hirae, Lactobacillus reuteri, Anaerostipes sp. nov., Bacteroidetes sp. and Enterorhabdus sp. resolves CD-recurrent disease in mice (Lawley et al., 2012).
CD-associated diarrhea (CDAD) is uncommon in children, especially during the first years of life, and only a few studies have analyzed BGM composition during CD infection in this age group. In children from China with CDAD, α-diversity was decreased compared to healthy controls. In these children, differences in microbiota composition at the phylum level (higher abundance of Firmicutes, Actinobacteria, Proteobacteria and Acidobacteria, and lower abundance of Bacteroidetes), as well as the genera level (increase in Enterococcus, Streptococcus, Escherichia/Shigella, Klebsiella, Stenotrophomonas and Haemophilus, and decrease in Bacteroides, Faecalibacterium, Parabacteroides, Lachnospiracea incertae sedis, Dialister, and Alistipes) were observed when compared to healthy controls (Ling et al., 2014). In hospitalized children from the United Kingdom with acute diarrhea, CD carriage (detected by culture and lateral flow test) was associated with a higher α-diversity index, and a higher abundance of the families Lachnospiraceae and Ruminococcaceae compared to culture-negative children (Lees et al., 2020). These findings are contrary to those described by Ling et al. (2014) in Chinese children; in this study detection of CD was associated with a decrease in the abundance of butyrate-producing bacteria from the Ruminococcaceae and Lachnospiraceae families. This coincides with results from adults with CD-associated diarrhea (Antharam et al., 2013). A protective role of butyrate in preventing CD disease has been described in animal models, given that antibiotics that predispose the host to CD-infection deplete butyrate levels (Theriot et al., 2014), and butyrate reduces intestinal epithelial permeability, inflammation and bacterial translocation in infected mice (Fachi et al., 2019). The potential role of differences in the abundance of BGM butyrate-producing bacteria in relation to different clinical outcomes has not been characterized; metabolomic studies in children with CD-associated diarrhea would be useful in determining the validity of this hypothesis.
The toxigenic status of CD also determines differences in microbiota composition. In children from China with CD-associated diarrhea, α-diversity was lower in those with CD producing both toxins A and B (A+B+) compared to those producing only toxin B (A−B+); CD A+B+ was associated with increased abundance of Firmicutes and Acidobacteria at phylum level, increased abundance of Lactobacillales at order level and Enterococcaceae at the family level compared to CD A−B+ (Ling et al., 2014). In the cohort from the United Kingdom, the presence of toxigenic CD was associated with an increased abundance of Proteobacteria and a decreased abundance of Firmicutes and Bacteroidota at the phylum level, and an increased abundance of Klebsiella at the genus level compared to non-toxigenic CD carriers and culture-negative children (Lees et al., 2020). Differences in both studies may be partially explained by the age of children studied, as Chinese children ranged from 2 to 4 years of age while children from United Kingdom ranged from 0 to 16 years of age.
In animal models, metabolism of bile acids driven by the BGM has a protective role by inhibiting spore germination and overgrowth of CD-vegetative cells (Theriot et al., 2014); whether this (or other) mechanisms explain why some children are asymptomatic carriers of inactive forms of CD, while others are toxin-producers and can develop CD-associated disease is still unknown.
The fact that fecal transplantation, which aims to restore a “healthy gut microbiota,” has proven effective in the treatment of recurrent CD in adults and children, supports that gut microbiota disruption is a key factor in the development of CD-disease. In children, fecal transplantation causes an increase in α-diversity (which was particularly reduced in children requiring multiple transplants to achieve clinical success), a decrease in Proteobacteria and an increase in Bacteroidota, evolving toward a “healthy microbiota” in parallel with clinical improvement (Fareed et al., 2018; Li X. et al., 2018). Metabolite-mediated mechanisms related to BGM restoration have been described in FMT (Martinez-Gili et al., 2020). Bile salt hydrolases (BSH)-producing bacteria present in adult donor samples have been associated with a decrease of taurocholic acid (TCA) in recipient stools, a conjugated bile acid that triggers CD germination (Mullish et al., 2019). Also, a restoration of SCFA levels is seen in FMT adult recipients (Seekatz et al., 2018), which might be partially explained by changes in diet after FMT or antibiotic cessation post resolution of recurrent CD-disease as discussed by other authors (Martinez-Gili et al., 2020). In an in vitro model of CD infection, the SCFAs succinate, butyrate, acetate, and isobutyrate, decrease when antibiotics are discontinued, and are not affected by a later FMT. However, recovery of valerate levels is only seen after FMT, which is related to inhibition of CD growth (McDonald et al., 2018). As we previously described, SCFA have anti-inflammatory effects which can participate in CD-recovery after FMT by other mechanisms beyond inhibition of CD growth. It is important to consider that FMT is associated with changes in gut virome and mycobiome (Fujimoto, Zhang), which is not intended to be discussed in this review, but highlights the fact that even when BGM disruption seems to be a key factor in CD-disease, the FMT effects are multifactorial and most likely only partially mediated by the restoration of BGM and bacterial metabolites.
Gut Microbiota and Respiratory Infections During Early Childhood
Acute respiratory infections (ARIs) are a major source of morbidity and mortality in children worldwide (United Nations Inter-agency Group for Child Mortality Estimation, 2019), representing the second leading cause of years of life lost due to premature mortality and one of the most frequent causes of hospitalization (Nair et al., 2013). These infections can range from mild upper ARIs (AURIs), such as the common cold, to life-threatening conditions including lower ARIs (ALRIs), such as pneumonia and bronchiolitis. ARIs are the most frequent type of infection during the first 3 years of life, with a median of 10 episodes per child (with up to 90% corresponding to AURIs) (Vissing et al., 2018). Viruses are the primary cause of both AURIs and ALRIs in children, with rhinovirus the most common cause of AURI (Kwiyolecha et al., 2020) and respiratory syncytial virus the most common cause of ALRI (O’Brien et al., 2019; Li et al., 2021). Influenza virus causes seasonal global epidemics, representing an important cause of ALRIs associated with hospitalization and severe outcomes in children aged <5 years as compared to older children and adults (Ruf and Knuf, 2014; Wang et al., 2020). Adenovirus, parainfluenza, metapneumovirus, and enterovirus (among others) also cause ARIs in children, however, they are less common (Benet et al., 2017; Chen J. et al., 2018). Bacteria are also a major cause of ALRIs, with Streptococcus pneumoniae causing the majority of infections despite global vaccination efforts, resulting in significant morbi-mortality in children <5 years of age (Wahl et al., 2018).
The RSV pathogenesis has been studied thoroughly. The virus replicates in the airway epithelium, which can result in lower airway inflammation, alveolar epithelial cell apoptosis, bronchial epithelial necrosis, multifocal acute alveolitis, intra-alveolar edema, and hemorrhage. An intense neutrophil response during this early phase is positively correlated with tissue damage and disease severity (Russell et al., 2017; Sebina and Phipps, 2020). Activation of adaptive immunity characterized by cytotoxic cells and Th1 cells are protective, mediating viral clearance; Th2-skewed responses on the other hand appear to be deleterious and are associated with severe disease outcome (Caballero et al., 2015) and may be related to a future risk of asthma development (Restori et al., 2018).
Linking the Gut Microbiota and Respiratory Infections: The Gut-Lung Axis
There is extensive evidence that the BGM may influence immunity at the respiratory tract level, resulting in protection, predisposition or modification of respiratory infection/disease. The mesenteric lymphatic system can translocate intact bacteria, fragments, or metabolites from the intestinal lumen to systemic circulation, reaching the respiratory tract and modulating the immune response at this level (Enaud et al., 2020). The gut-lung axis has been extensively reviewed by other authors (Budden et al., 2017; Dumas et al., 2018; Enaud et al., 2020; Sencio et al., 2021). We summarize the main components mediating BGM modulation over lung immunity in Table 6.
Gut Microbiota During Acute Respiratory Infections in Children and Mouse Models
Lung infections can induce changes in the gut microbiota, establishing a bidirectional axis between the respiratory tract and the gut microbiota.
Four microbiota profiles were identified among children hospitalized with bronchiolitis (65% with RSV and 23% with Rhinovirus) and healthy controls, e.g., Escherichia-dominant, Bifidobacterium-dominant, Enterobacter/Veillonella-dominant, and Bacteroides-dominant. The proportion of children with bronchiolitis was significantly higher in the Bacteroides-dominant profile; these children also had increased bacterial richness and α-diversity. The Enterobacter/Veillonella-dominant profile had the lowest proportion of children with bronchiolitis (Hasegawa et al., 2017). In addition, functional predictions based on taxonomy indicated that the BGM of children with bronchiolitis had increased abundance of gene functions related to sphingolipid metabolic pathways compared to controls, which may be associated with an immunomodulatory response (Hasegawa et al., 2017). In hospitalized children with RSV bronchiolitis, diminished α-diversity was observed in the BGM compared to healthy controls; additionally, alterations in composition were observed, characterized by enrichment in Proteobacteria and Bacteroidota at the phylum level and enrichment in Bacteroides and Streptococcus at the genus level, as compared to healthy children. Conversely, the oropharyngeal microbiota in children with bronchiolitis was enriched in Firmicutes and depleted in Bacteroidota and Proteobacteria compared to healthy controls. Both the fecal and oropharyngeal microbiota remained relatively unchanged 7–10 days after admission (Hu et al., 2017). The quantity of Bifidobacterium was decreased in fecal samples of wheezing children hospitalized with bronchiolitis or asthma of unknown etiology, which was in turn associated with lower serum levels of Th1 cytokines and higher levels of serum Th2 and Th17 cytokines, as compared to controls (Liwen et al., 2018).
In summary, relatively limited data suggests that the BGM is altered in children with bronchiolitis or wheezing episodes when compared to healthy age-matched controls, with a reduction in α-diversity, an increase in the phylum Bacteroidota (possibly associated with specific sphingolipid metabolic pathways) and a reduction in Bifidobacterium (possibly associated with serum Th2/Th1 imbalance). As these studies are cross-sectional, the question as to whether these alterations in the BGM are the result of or the cause of the host’s predisposition to ARIs is unclear. The latter theory is supported by a recent birth-cohort study from The Netherlands aimed at assessing the impact of delivery mode on the gut microbiota and health during the first year of life. This study concluded that there is an association between microbiota composition during the first week of life and the number of ARIs later in life; specifically the presence of Bifidobacterium was associated with fewer ARIs and the presence of Klebsiella and Enterococcus with more ARIs over the first year (Reyman et al., 2019). The fact that a “healthy” BGM has protective effects in the case of ARIs is also supported by studies in mouse models, e.g., the transfer of a “healthy” BGM from wild mice to laboratory mice resulted in reduced inflammation and increased survival following influenza infection (Rosshart et al., 2017); furthermore, gut microbiota-derived SCFAs (acetate) protected mice against RSV infection, through the promotion of type I IFN in pulmonary epithelial cells (Antunes et al., 2019). Conversely, experimental RSV and influenza infections in mice caused an alteration in their gut microbiota, with an increase in Bacteroidota (mainly due to Bacteroidaceae) and a decrease in Firmicutes (mainly Lachnospiraceae and Lactobacillaceae families) (Groves et al., 2018). Also, RSV infection altered lipid metabolism, increasing fecal abundance of sphingolipids, polyunsaturated fatty acids, and the SCFA valerate (Groves et al., 2020), supporting the gut microbiota metabolic functional prediction in children cited before (Hasegawa et al., 2017). Nevertheless, the theoretical impact of these changes on anti-inflammatory responses remains unclear.
For the purpose of this review, we were not able to find publications on the relationship between the BGM and other ARIs such as influenza or pneumococcal pneumonia, but there is rising evidence coming from animal models. Studies in mice focusing exclusively on the effect of viral influenza on the BGM describe an association with dysbiosis, primarily characterized by an increase in Proteobacteria or Enterobacteriaceae (Wang et al., 2014; Deriu et al., 2016; Bartley et al., 2017) and a decrease in Bacteroidetes (Yildiz et al., 2018) and/or Firmicutes (Wang et al., 2014). BGM alterations seem to be mediated by the inflammatory response initiated in the respiratory tract and through a systemic release of cytokines, type I-IFN (Deriu et al., 2016) and IFN-gamma (Wang et al., 2014), leading to intestinal imbalances that predispose the host to invasion by bacterial pathogens (Deriu et al., 2016; Yildiz et al., 2018).
A healthy BGM seems protective against Streptococcus pneumoniae infection in mice, as antibiotic-induced depletion of the gut microbiota was associated with worse outcomes compared to controls, partially mediated by decreased lung macrophage phagocytosis capacity (Schuijt et al., 2016). Segmented filamentous bacteria protected against pneumococcal pneumonia in immunocompromised mice Rag -/- by promoting neutrophil resolution following lung infection (Felix et al., 2018). Whether these findings have potential correlations in children, remains unclear.
Discussion and Therapeutic Projections
The development and the dynamics of BGM composition are closely related to the development of the immune system during first years of life, modulating protection or predisposition to infections. Studies in animal models and humans, mainly children, allow us to conclude that bidirectional interactions between infectious microorganisms and the gut BGM are common (Figure 2). One example is the process of gut commensal bacteria development and metabolite production, which interact directly with the offending bacteria or indirectly through immune-stimulated interactions. In the opposite direction is the effect of infection on modifications of the pre-existing BGM, resulting in dysbiosis, which progressively returns to a “healthy” BGM after the pathogen is cleared. Although there are direct interactions between components of the BGM and gastrointestinal pathogens (Table 3), host immune system stimulation seems to be the key mediator as exemplified by the “gut-lung” axis model. This immune modulatory role is further supported by several studies on immune response to vaccines, not reviewed here (Lynn et al., 2021), where the microbiota component is associated with differential immune responses to several vaccines in children in various populations, including the RV, the BCG, and the influenza vaccines.
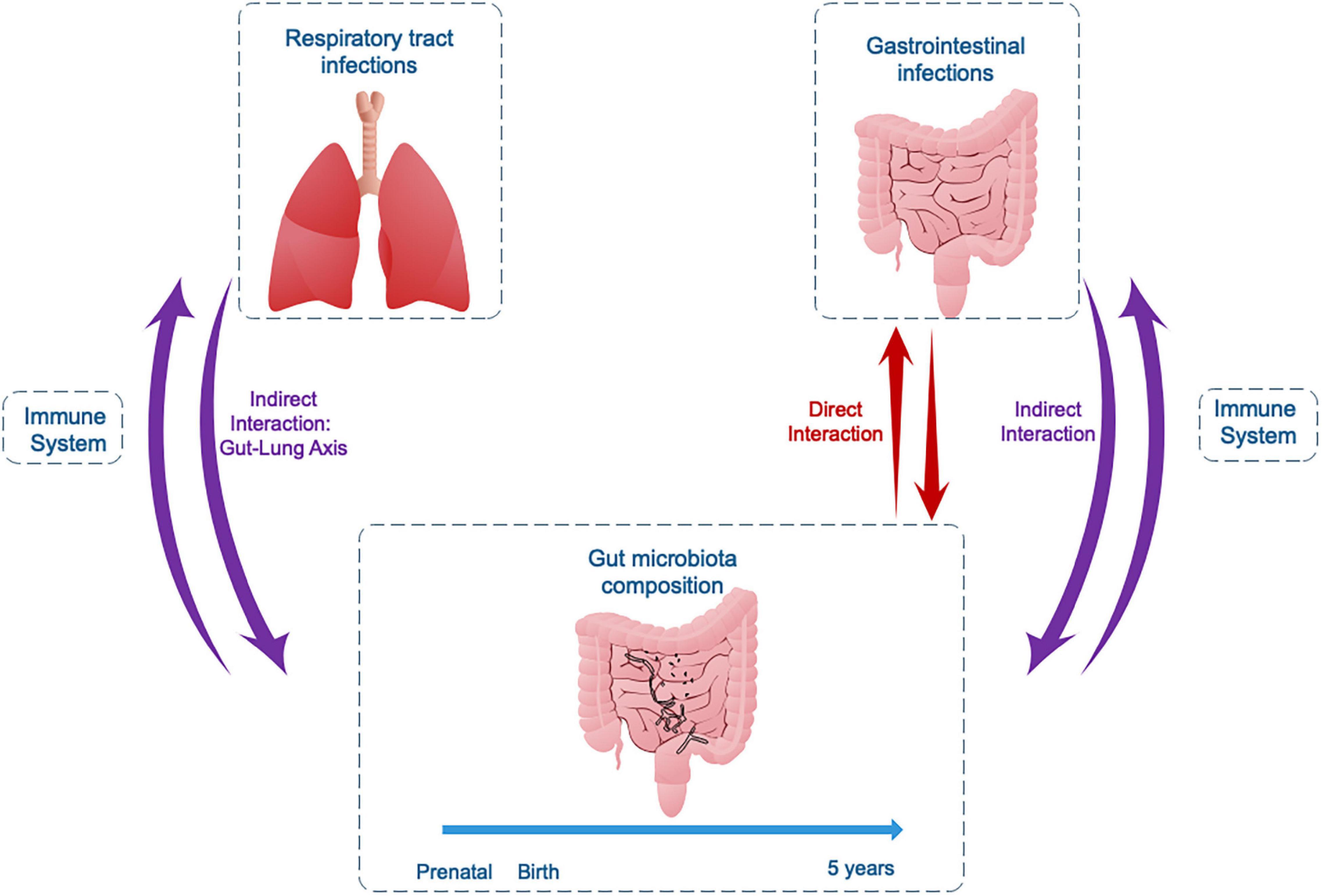
Figure 2. Bidirectional interactions between the gut microbiota and gastrointestinal or respiratory tract infections in childhood.
The specific components of the microbiota, immune system, and infectious agents that play a role in these interactions remains a matter of active research. Studies in children reviewed here have primarily been performed during acute infections, hindering assessment as to whether any differences could be explained by the infection itself, or rather pre-existing dysbiosis. Large cohort and longitudinal studies in healthy children are needed to better understand the intra-individual evolution from a developing healthy gut microbiota to an altered gut microbiota during an infectious episode, including possible convalescence.
Several attempts to modulate or modify the BGM in order to protect against infectious diseases have been explored, of which probiotics have been the most deeply studied. Clinical efficacy of this strategy in reducing the duration and intensity of gastroenteritis symptoms is weak (Szajewska et al., 2020), partially because studies have used diverse probiotic strains. In children with non-specific acute diarrhea, treatment with Lacticaseibacillus rhamnosus was associated with modulation of the gut microbiota, characterized by the attenuation of dysbiosis, higher fecal IgA levels, lower intestinal inflammatory markers (lactoferrin and calprotectin), and improved clinical outcomes compared to non-treated children (Lai et al., 2019). In children with acute RV-diarrhea, administration of S. boulardii was associated with reduced α-diversity and altered composition (an increase in Proteobacteria and a decrease in Firmicutes) during first 5 days of the diarrhea episode, but not at days 10–30, as compared to healthy controls; although a non-treated group was not evaluated in this study to corroborate if probiotics had a benefit in the recovery of the gut-microbiota (Dinleyici et al., 2018). In children with infectious diarrhea of any cause, administration of S. boulardii was associated with the recovery of α- and β-diversity over the 15 days following treatment to levels comparable to healthy controls, which was not observed in non-treated children with diarrhea (Toro Monjaraz et al., 2021). It is noteworthy that the effect of probiotics on BGM composition during diarrhea has been assessed using S. boulardii, a fungus closely related to Saccharomyces cerevisiae, which is detected in stools of healthy children (Strati et al., 2016). The mechanisms related to this specific probiotic include direct binding to pathogens, induction of antimicrobial peptides and immunomodulatory effects, among others (Pais et al., 2020), similar to mechanisms of interaction between bacterial BGM and pathogens previously discussed in this review. Though mycobiome was not assessed in this article, these findings highlight the complex equilibrium and interactions between different components of the microbiota and the host. For NEC prevention in preterm infants, clinical guidelines recommend specific probiotic strains with little evidence of efficacy (van den Akker et al., 2020). The clinical benefit of certain strains in preterm infants seems to be dependent on their effects on the BGM, e.g., Bifidobacterium breve strain BBG-001 did not affect NEC incidence in a multicenter, randomized, controlled phase 3 trial including preterm infants (PiPS trial). There was no difference in microbial richness and diversity of the gut microbiota in the probiotic and placebo groups (Millar et al., 2017). Conversely, a combination of 3 probiotic strains (Bifidobacterium longum subsp. infantis BB-02, Streptococcus thermophilus TH-4, and Bifidobacterium animalis subsp. lactis BB-12) was associated with a 54% reduction in Bell stage 2 or greater NEC, with significant changes in the gut microbiota compared to placebo (increased in Bifidobacterium and decreased in Enterococcus) (Plummer et al., 2018). Probiotics have been associated with some protective effect in preventing and reducing the duration of ARIs in children (Wang et al., 2016; Laursen and Hojsak, 2018); but to our knowledge there are no studies simultaneously evaluating their effect on the BGM.
Prebiotics, defined as “substrates selectively utilized by host microorganisms conferring a health benefit” (Gibson et al., 2017), synbiotics, defined as “mixture comprising live microorganisms and substrates selectively utilized by host microorganisms that confers a health benefit” (Swanson et al., 2020), and more recently postbiotics, defined as “preparation of inanimate microorganisms and/or their components that confers a health benefit” (Salminen et al., 2021) are additional potential therapeutic tools that may influence infections by action on the microbiota-immune system axis. A deeper understanding of the associated mechanisms and their clinical relevance in pediatric infectious diseases will be required before advancing these and other preventive or therapeutic interventions. Finally, the use of fecal transplantation to restore a “healthy BGM” beyond CD infection, e.g., for prevention of multi-drug resistant infections in colonized patients has been proposed but unexplored in children (Gurram and Sue, 2019). Whether the use of these therapeutic or preventive BGM-related tools during certain periods of early childhood within a “window of opportunity” can significantly affect the predisposition to, or clinical outcomes of infections during adolescence and adulthood is still not completely understood and requires large cohort studies.
This review has focused on the role of the bacterial gut microbiota on the most common infections in childhood (acute diarrhea and acute respiratory infections) and characteristics of infection-related gastrointestinal entities (necrotizing enterocolitis and C. difficile infection). However, several aspects of the interaction between the BGM and infections have not been explored in depth, including the influence of the BGM on vaccine response (Lynn et al., 2021) and its role in antimicrobial efficacy, acting as reservoirs for AMR genes (Anthony et al., 2021) or even modulating the metabolism and pharmacokinetics of antimicrobials (Zimmermann et al., 2019; Klünemann et al., 2021). Finally, there is increasing evidence about the importance of viruses, fungus, archaea and parasites in maintaining the gut homeostasis which was not included in this review. The role of these microorganisms in dysbiosis during common childhood infections, and how variations in these components affect the bacterial GM composition are matters to be assessed.
Author Contributions
SG and MO’R conceived the original idea. SG and XA performed literature search and study selection. SG and MO’R wrote the manuscript with substantial support from XA, YL, JT, PG, MF, and RV. All authors approved the final manuscript.
Conflict of Interest
The authors declare that the research was conducted in the absence of any commercial or financial relationships that could be construed as a potential conflict of interest.
Publisher’s Note
All claims expressed in this article are solely those of the authors and do not necessarily represent those of their affiliated organizations, or those of the publisher, the editors and the reviewers. Any product that may be evaluated in this article, or claim that may be made by its manufacturer, is not guaranteed or endorsed by the publisher.
Supplementary Material
The Supplementary Material for this article can be found online at: https://www.frontiersin.org/articles/10.3389/fmicb.2021.793050/full#supplementary-material
References
Aagaard, K., Ma, J., Antony, K. M., Ganu, R., Petrosino, J., and Versalovic, J. (2014). The placenta harbors a unique microbiome. Sci. Transl. Med. 6: 237ra65. doi: 10.1126/scitranslmed.3008599
Abt, M. C., McKenney, P. T., and Pamer, E. G. (2016). Clostridium difficile colitis: pathogenesis and host defence. Nat. Rev. Microbiol. 14, 609–620. doi: 10.1038/nrmicro.2016.108
Ahmad, R., Rah, B., Bastola, D., Dhawan, P., and Singh, A. B. (2017). Obesity-induces organ and tissue specific tight junction restructuring and barrier deregulation by claudin switching. Sci. Rep. 7:5125. doi: 10.1038/s41598-017-04989-8
Alameddine, J., Godefroy, E., Papargyris, L., Sarrabayrouse, G., Tabiasco, J., Bridonneau, C., et al. (2019). Faecalibacterium prausnitzii skews human DC to prime IL10-producing T Cells through TLR2/6/JNK signaling and IL-10, IL-27, CD39, and IDO-1 induction. Front. Immunol. 10:143. doi: 10.3389/fimmu.2019.00143
Almand, E. A., Moore, M. D., Outlaw, J., and Jaykus, L. A. (2017). Human norovirus binding to select bacteria representative of the human gut microbiota. PLoS One 12:e0173124. doi: 10.1371/journal.pone.0173124
Aloisio, I., Quagliariello, A., De Fanti, S., Luiselli, D., De Filippo, C., Albanese, D., et al. (2016). Evaluation of the effects of intrapartum antibiotic prophylaxis on newborn intestinal microbiota using a sequencing approach targeted to multi hypervariable 16S rDNA regions. Appl. Microbiol. Biotechnol. 100, 5537–5546. doi: 10.1007/s00253-016-7410-2
Alzate, J. F., Toro-Londono, M., Cabarcas, F., Garcia-Montoya, G., and Galvan-Diaz, A. (2020). Contrasting microbiota profiles observed in children carrying either Blastocystis spp. or the commensal amoebas Entamoeba coli or Endolimax nana. Sci. Rep. 10:15354. doi: 10.1038/s41598-020-72286-y
An, D., Oh, S. F., Olszak, T., Neves, J. F., Avci, F. Y., Erturk-Hasdemir, D., et al. (2014). Sphingolipids from a symbiotic microbe regulate homeostasis of host intestinal natural killer T cells. Cell 156, 123–133. doi: 10.1016/j.cell.2013.11.042
Anjewierden, S., Han, Z., Foster, C. B., Pant, C., and Deshpande, A. (2019). Risk factors for Clostridium difficile infection in pediatric inpatients: a meta-analysis and systematic review. Infect. Control. Hosp. Epidemiol. 40, 420–426. doi: 10.1017/ice.2019.23
Antharam, V. C., Li, E. C., Ishmael, A., Sharma, A., Mai, V., Rand, K. H., et al. (2013). Intestinal dysbiosis and depletion of butyrogenic bacteria in Clostridium difficile infection and nosocomial diarrhea. J. Clin. Microbiol. 51, 2884–2892. doi: 10.1128/JCM.00845-13
Anthony, W. E., Burnham, C. D., Dantas, G., and Kwon, J. H. (2021). The gut microbiome as a reservoir for antimicrobial resistance. J. Infect. Dis. 223, S209–S213. doi: 10.1093/infdis/jiaa497
Antunes, K. H., Fachi, J. L., de Paula, R., da Silva, E. F., Pral, L. P., Dos Santos, A. A., et al. (2019). Microbiota-derived acetate protects against respiratory syncytial virus infection through a GPR43-type 1 interferon response. Nat. Commun. 10:3273. doi: 10.1038/s41467-019-11152-6
Arpaia, N., Campbell, C., Fan, X., Dikiy, S., van der Veeken, J., deRoos, P., et al. (2013). Metabolites produced by commensal bacteria promote peripheral regulatory T-cell generation. Nature 504, 451–455. doi: 10.1038/nature12726
Arrieta, M. C., Stiemsma, L. T., Amenyogbe, N., Brown, E. M., and Finlay, B. (2014). The intestinal microbiome in early life: health and disease. Front. Immunol. 5:427. doi: 10.3389/fimmu.2014.00427
Arumugam, M., Raes, J., Pelletier, E., Le Paslier, D., Yamada, T., Mende, D. R., et al. (2011). Enterotypes of the human gut microbiome. Nature 473, 174–180. doi: 10.1038/nature09944
Azad, M. B., Konya, T., Persaud, R. R., Guttman, D. S., Chari, R. S., Field, C. J., et al. (2016). Impact of maternal intrapartum antibiotics, method of birth and breastfeeding on gut microbiota during the first year of life: a prospective cohort study. BJOG 123, 983–993. doi: 10.1111/1471-0528.13601
Babakobi, M. D., Reshef, L., Gihaz, S., Belgorodsky, B., Fishman, A., Bujanover, Y., et al. (2020). Effect of maternal diet and milk lipid composition on the infant gut and maternal milk microbiomes. Nutrients 12:2539. doi: 10.3390/nu12092539
Backhed, F., Fraser, C. M., Ringel, Y., Sanders, M. E., Sartor, R. B., Sherman, P. M., et al. (2012). Defining a healthy human gut microbiome: current concepts, future directions, and clinical applications. Cell Host Microbe 12, 611–622. doi: 10.1016/j.chom.2012.10.012
Baldridge, M. T., Nice, T. J., McCune, B. T., Yokoyama, C. C., Kambal, A., Wheadon, M., et al. (2015). Commensal microbes and interferon-lambda determine persistence of enteric murine norovirus infection. Science 347, 266–269. doi: 10.1126/science.1258025
Bartley, J. M., Zhou, X., Kuchel, G. A., Weinstock, G. M., and Haynes, L. (2017). Impact of age, caloric restriction, and influenza infection on mouse gut microbiome: an exploratory study of the role of age-related microbiome changes on influenza responses. Front. Immunol. 8:1164. doi: 10.3389/fimmu.2017.01164
Baumler, A. J., and Sperandio, V. (2016). Interactions between the microbiota and pathogenic bacteria in the gut. Nature 535, 85–93. doi: 10.1038/nature18849
Beau, I., Cotte-Laffitte, J., Geniteau-Legendre, M., Estes, M. K., and Servin, A. L. (2007). An NSP4-dependant mechanism by which rotavirus impairs lactase enzymatic activity in brush border of human enterocyte-like Caco-2 cells. Cell Microbiol. 9, 2254–2266. doi: 10.1111/j.1462-5822.2007.00956.x
Becker-Dreps, S., Allali, I., Monteagudo, A., Vilchez, S., Hudgens, M. G., Rogawski, E. T. I, et al. (2015). Gut microbiome composition in young nicaraguan children during diarrhea episodes and recovery. Am. J. Trop. Med. Hyg. 93, 1187–1193. doi: 10.4269/ajtmh.15-0322
Belkaid, Y. (2007). Regulatory T cells and infection: a dangerous necessity. Nat. Rev. Immunol. 7, 875–888. doi: 10.1038/nri2189
Belzer, C., Gerber, G. K., Roeselers, G., Delaney, M., DuBois, A., Liu, Q., et al. (2014). Dynamics of the microbiota in response to host infection. PLoS One 9:e95534. doi: 10.1371/journal.pone.0095534
Benet, T., Sanchez Picot, V., Messaoudi, M., Chou, M., Eap, T., Wang, J., et al. (2017). Microorganisms associated with pneumonia in children <5 years of age in developing and emerging countries: the gabriel pneumonia multicenter, prospective, case-control study. Clin Infect. Dis. 65, 604–612. doi: 10.1093/cid/cix378
Blutt, S. E., Miller, A. D., Salmon, S. L., Metzger, D. W., and Conner, M. E. (2012). IgA is important for clearance and critical for protection from rotavirus infection. Mucos. Immunol. 5, 712–719. doi: 10.1038/mi.2012.51
Boonchooduang, N., Louthrenoo, O., Chattipakorn, N., and Chattipakorn, S. C. (2020). Possible links between gut-microbiota and attention-deficit/hyperactivity disorders in children and adolescents. Eur. J. Nutr. 59, 3391–3403. doi: 10.1007/s00394-020-02383-1
Bouskra, D., Brezillon, C., Berard, M., Werts, C., Varona, R. I, Boneca, G., et al. (2008). Lymphoid tissue genesis induced by commensals through NOD1 regulates intestinal homeostasis. Nature 456, 507–510. doi: 10.1038/nature07450
Braun, T., Di Segni, A., BenShoshan, M., Asaf, R., Squires, J. E., Farage Barhom, S., et al. (2017). Fecal microbial characterization of hospitalized patients with suspected infectious diarrhea shows significant dysbiosis. Sci. Rep. 7:1088. doi: 10.1038/s41598-017-01217-1
Brehin, C., Dubois, D., Dicky, O., Breinig, S., Oswald, E., and Serino, M. (2020). Evolution of gut microbiome and metabolome in suspected necrotizing enterocolitis: a case-control study. J. Clin. Med. 9:2278. doi: 10.3390/jcm9072278
Bucardo, F., Reyes, Y., Svensson, L., and Nordgren, J. (2014). Predominance of norovirus and sapovirus in nicaragua after implementation of universal rotavirus vaccination. PLoS One 9:e98201. doi: 10.1371/journal.pone.0098201
Budden, K. F., Gellatly, S. L., Wood, D. L., Cooper, M. A., Morrison, M., Hugenholtz, P., et al. (2017). Emerging pathogenic links between microbiota and the gut-lung axis. Nat. Rev. Microbiol. 15, 55–63. doi: 10.1038/nrmicro.2016.142
Buonomo, E. L., Madan, R., Pramoonjago, P., Li, L., Okusa, M. D., and Petri, W. A. Jr. (2013). Role of interleukin 23 signaling in clostridium Difficile colitis. J. Infect. Dis. 208, 917–920. doi: 10.1093/infdis/jit277
Buonomo, E. L., and Petri, W. A. Jr. (2016). The microbiota and immune response during Clostridium difficile infection. Anaerobe 41, 79–84. doi: 10.1016/j.anaerobe.2016.05.009
Caballero, M. T., Serra, M. E., Acosta, P. L., Marzec, J., Gibbons, L., Salim, M., et al. (2015). TLR4 genotype and environmental LPS mediate RSV bronchiolitis through Th2 polarization. J. Clin. Invest. 125, 571–582. doi: 10.1172/JCI75183
Cacho, N. T., Parker, L. A., and Neu, J. (2017). Necrotizing enterocolitis and human milk feeding: a systematic review. Clin. Perinatol. 44, 49–67. doi: 10.1016/j.clp.2016.11.009
Cahenzli, J., Koller, Y., Wyss, M., Geuking, M. B., and McCoy, K. D. (2013). Intestinal microbial diversity during early-life colonization shapes long-term IgE levels. Cell Host Microbe 14, 559–570. doi: 10.1016/j.chom.2013.10.004
Callahan, B. J., McMurdie, P. J., and Holmes, S. P. (2017). Exact sequence variants should replace operational taxonomic units in marker-gene data analysis. ISME J. 11, 2639–2643. doi: 10.1038/ismej.2017.119
Cani, P. D., Possemiers, S., Van de Wiele, T., Guiot, Y., Everard, A., Rottier, O., et al. (2009). Changes in gut microbiota control inflammation in obese mice through a mechanism involving GLP-2-driven improvement of gut permeability. Gut 58, 1091–1103. doi: 10.1136/gut.2008.165886
Carlisle, E. M., Poroyko, V., Caplan, M. S., Alverdy, J. A., and Liu, D. (2011). Gram negative bacteria are associated with the early stages of necrotizing enterocolitis. PLoS One 6:e18084. doi: 10.1371/journal.pone.0018084
Carvalho, F. A., Aitken, J. D., Vijay-Kumar, M., and Gewirtz, A. T. (2012). Toll-like receptor-gut microbiota interactions: perturb at your own risk! Annu. Rev. Physiol. 74, 177–198. doi: 10.1146/annurev-physiol-020911-153330
Cash, H. L., Whitham, C. V., Behrendt, C. L., and Hooper, L. V. (2006). Symbiotic bacteria direct expression of an intestinal bactericidal lectin. Science 313, 1126–1130. doi: 10.1126/science.1127119
Castano-Rodriguez, N., Underwood, A. P., Merif, J., Riordan, S. M., Rawlinson, W. D., Mitchell, H. M., et al. (2018). Gut microbiome analysis identifies potential etiological factors in acute gastroenteritis. Infect. Immun. 86, e60–e18. e00060-18, doi: 10.1128/IAI.00060-18
Chassin, C., Kocur, M., Pott, J., Duerr, C. U., Gutle, D., Lotz, M., et al. (2010). miR-146a mediates protective innate immune tolerance in the neonate intestine. Cell Host Microbe 8, 358–368. doi: 10.1016/j.chom.2010.09.005
Chen, B., Chen, H., Shu, X., Yin, Y., Li, J., Qin, J., et al. (2018). Presence of segmented filamentous bacteria in human children and its potential role in the modulation of human gut immunity. Front. Microbiol. 9:1403. doi: 10.3389/fmicb.2018.01403
Chen, J., Hu, P., Zhou, T., Zheng, T., Zhou, L., Jiang, C., et al. (2018). Epidemiology and clinical characteristics of acute respiratory tract infections among hospitalized infants and young children in chengdu. West China, 2009-2014. BMC Pediatr. 18:216. doi: 10.1186/s12887-018-1203-y
Chen, L. W., Chen, P. H., and Hsu, C. M. (2011). Commensal microflora contribute to host defense against Escherichia coli pneumonia through Toll-like receptors. Shock 36, 67–75. doi: 10.1097/SHK.0b013e3182184ee7
Chen, S. Y., Tsai, C. N., Lee, Y. S., Lin, C. Y., Huang, K. Y., Chao, H. C., et al. (2017). Intestinal microbiome in children with severe and complicated acute viral gastroenteritis. Sci. Rep. 7:46130. doi: 10.1038/srep46130
Cheng, M., and Ning, K. (2019). Stereotypes about enterotype: the old and new ideas. Genomics Proteomics Bioinformatics 17, 4–12. doi: 10.1016/j.gpb.2018.02.004
Chu, D. M., Antony, K. M., Ma, J., Prince, A. L., Showalter, L., Moller, M., et al. (2016). The early infant gut microbiome varies in association with a maternal high-fat diet. Genome Med. 8:77. doi: 10.1186/s13073-016-0330-z
Chu, D. M., Valentine, G. C., Seferovic, M. D., and Aagaard, K. M. (2019). The development of the human microbiome: why moms matter. Gastroenterol. Clin. North Am. 48, 357–375. doi: 10.1016/j.gtc.2019.04.004
Cioffi, C. C., Tavalire, H. F., Neiderhiser, J. M., Bohannan, B., and Leve, L. D. (2020). History of breastfeeding but not mode of delivery shapes the gut microbiome in childhood. PLoS One 15:e0235223. doi: 10.1371/journal.pone.0235223
Clarke, T. B. (2014). Early innate immunity to bacterial infection in the lung is regulated systemically by the commensal microbiota via nod-like receptor ligands. Infect. Immun. 82, 4596–4606. doi: 10.1128/IAI.02212-14
Coggins, S. A., Wynn, J. L., and Weitkamp, J. H. (2015). Infectious causes of necrotizing enterocolitis. Clin. Perinatol. 42, 133–54, ix. doi: 10.1016/j.clp.2014.10.012
Crawford, S. E., Ramani, S., Tate, J. E., Parashar, U. D., Svensson, L., Hagbom, M., et al. (2017). Rotavirus infection. Nat. Rev. Dis. Primers 3:17083. doi: 10.1038/nrdp.2017.83
De Filippo, C., Cavalieri, D., Di Paola, M., Ramazzotti, M., Poullet, J. B., Massart, S., et al. (2010). Impact of diet in shaping gut microbiota revealed by a comparative study in children from Europe and rural Africa. Proc. Natl. Acad. Sci. U.S.A. 107, 14691–14696. doi: 10.1073/pnas.1005963107
De Filippo, C., Di Paola, M., Ramazzotti, M., Albanese, D., Pieraccini, G., Banci, E., et al. (2017). Diet, environments, and gut microbiota. a preliminary investigation in children living in rural and urban burkina faso and Italy. Front. Microbiol. 8:1979. doi: 10.3389/fmicb.2017.01979
Deriu, E., Boxx, G. M., He, X., Pan, C., Benavidez, S. D., Cen, L., et al. (2016). Influenza virus affects intestinal microbiota and secondary salmonella infection in the gut through type i interferons. PLoS Pathog. 12:e1005572. doi: 10.1371/journal.ppat.1005572
Derrien, M., Alvarez, A. S., and de Vos, W. M. (2019). The gut microbiota in the first decade of life. Trends Microbiol. 27, 997–1010. doi: 10.1016/j.tim.2019.08.001
Dinleyici, E. C., Martinez-Martinez, D., Kara, A., Karbuz, A., Dalgic, N., Metin, O., et al. (2018). Time series analysis of the microbiota of children suffering from acute infectious diarrhea and their recovery after treatment. Front. Microbiol. 9:1230. doi: 10.3389/fmicb.2018.01230
Doan, T., Hinterwirth, A., Worden, L., Arzika, A. M., Maliki, R., Abdou, A., et al. (2019). Gut microbiome alteration in MORDOR I: a community-randomized trial of mass azithromycin distribution. Nat. Med. 25, 1370–1376. doi: 10.1038/s41591-019-0533-0
Dominguez-Bello, M. G., Costello, E. K., Contreras, M., Magris, M., Hidalgo, G., Fierer, N., et al. (2010). Delivery mode shapes the acquisition and structure of the initial microbiota across multiple body habitats in newborns. Proc. Natl. Acad. Sci. U.S.A. 107, 11971–11975. doi: 10.1073/pnas.1002601107
Dumas, A., Bernard, L., Poquet, Y., Lugo-Villarino, G., and Neyrolles, O. (2018). The role of the lung microbiota and the gut-lung axis in respiratory infectious diseases. Cell Microbiol. 20:e12966. doi: 10.1111/cmi.12966
Eck, A., Nbmm, R., Singendonk, M. M. J., Rijkers, G. T., Savelkoul, P. H. M., Meijssen, C. B., et al. (2020). Neonatal microbiota development and the effect of early life antibiotics are determined by two distinct settler types. PLoS One 15:e0228133. doi: 10.1371/journal.pone.0228133
Eglow, R., Pothoulakis, C., Itzkowitz, S., Israel, E. J., OKeane, C. J., Gong, D., et al. (1992). Diminished clostridium difficile toxin a sensitivity in newborn rabbit ileum is associated with decreased toxin a receptor. J. Clin. Investig. 90, 822–829. doi: 10.1172/JCI115957
Enaud, R., Prevel, R., Ciarlo, E., Beaufils, F., Wieers, G., Guery, B., et al. (2020). The gut-lung axis in health and respiratory diseases: a place for inter-organ and inter-kingdom crosstalks. Front Cell Infect. Microbiol. 10:9. doi: 10.3389/fcimb.2020.00009
Engevik, M. A., Banks, L. D., Engevik, K. A., Chang-Graham, A. L., Perry, J. L., Hutchinson, D. S., et al. (2020). Rotavirus infection induces glycan availability to promote ileum-specific changes in the microbiome aiding rotavirus virulence. Gut Microbes 11, 1324–1347. doi: 10.1080/19490976.2020.1754714
Exeni, R. A., Fernandez-Brando, R. J., Santiago, A. P., Fiorentino, G. A., Exeni, A. M., Ramos, M. V., et al. (2018). Pathogenic role of inflammatory response during Shiga toxin-associated hemolytic uremic syndrome (HUS). Pediatr. Nephrol. 33, 2057–2071. doi: 10.1007/s00467-017-3876-0
Fachi, J. L., Felipe, J. S., Pral, L. P., da Silva, B. K., Correa, R. O., de Andrade, M. C. P., et al. (2019). Butyrate protects mice from clostridium difficile-induced colitis through an hif-1-dependent mechanism. Cell Rep. 27:e7. doi: 10.1016/j.celrep.2019.03.054
Fareed, S., Sarode, N., Stewart, F. J., Malik, A., Laghaie, E., Khizer, S., et al. (2018). Applying fecal microbiota transplantation (FMT) to treat recurrent Clostridium difficile infections (rCDI) in children. PeerJ 6:e4663. doi: 10.7717/peerj.4663
Felix, K. M. I, Jaimez, A., Nguyen, T. V., Ma, H., Raslan, W. A., Klinger, C. N., et al. (2018). Gut microbiota contributes to resistance against pneumococcal pneumonia in immunodeficient rag(-/-) mice. Front. Cell Infect. Microbiol. 8:118. doi: 10.3389/fcimb.2018.00118
Feng, J., He, Y., Liu, D., Li, L., Chen, J., and Yu, J. (2019). The constitution and functional prediction of the microbiota in necrotizing enterocolitis with a gestational age of over 28 weeks. Medicine 98:e17206. doi: 10.1097/MD.0000000000017206
Feng, Y., Huang, Y., Wang, Y., Wang, P., Song, H., and Wang, F. (2019). Antibiotics induced intestinal tight junction barrier dysfunction is associated with microbiota dysbiosis, activated NLRP3 inflammasome and autophagy. PLoS One 14:e0218384. doi: 10.1371/journal.pone.0218384
Flint, H. J., Scott, K. P., Duncan, S. H., Louis, P., and Forano, E. (2012). Microbial degradation of complex carbohydrates in the gut. Gut Microbes 3, 289–306. doi: 10.4161/gmic.19897
Florez, I. D., Nino-Serna, L. F., and Beltran-Arroyave, C. P. (2020). Acute infectious diarrhea and gastroenteritis in children. Curr. Infect. Dis. Rep. 22:4. doi: 10.1007/s11908-020-0713-6
Fulci, V., Stronati, L., Cucchiara, S., Laudadio, I., and Carissimi, C. (2021). Emerging roles of gut virome in pediatric diseases. Int. J. Mol. Sci. 22:4127. doi: 10.3390/ijms22084127
Furusawa, Y., Obata, Y., Fukuda, S., Endo, T. A., Nakato, G., Takahashi, D., et al. (2013). Commensal microbe-derived butyrate induces the differentiation of colonic regulatory T cells. Nature 504, 446–450. doi: 10.1038/nature12721
Gallardo, P., Izquierdo, M., Vidal, R. M., Chamorro-Veloso, N., Rossello-Mora, R., ORyan, M., et al. (2017). Distinctive gut microbiota is associated with diarrheagenic Escherichia coli infections in chilean children. Front. Cell Infect. Microbiol. 7:424. doi: 10.3389/fcimb.2017.00424
Gallardo, P., Izquierdo, M., Vidal, R. M., Soto, F., Ossa, J. C., and Farfan, M. J. (2020). Gut microbiota-metabolome changes in children with diarrhea by diarrheagenic E. coli. Front. Cell Infect. Microbiol. 10:485. doi: 10.3389/fcimb.2020.00485
Gaufin, T., Tobin, N. H., and Aldrovandi, G. M. (2018). The importance of the microbiome in pediatrics and pediatric infectious diseases. Curr. Opin. Pediatr. 30, 117–124. doi: 10.1097/MOP.0000000000000576
Gensollen, T., Iyer, S. S., Kasper, D. L., and Blumberg, R. S. (2016). How colonization by microbiota in early life shapes the immune system. Science 352, 539–544. doi: 10.1126/science.aad9378
Gibson, G. R., Hutkins, R., Sanders, M. E., Prescott, S. L., Reimer, R. A., Salminen, S. J., et al. (2017). Expert consensus document: the international scientific association for probiotics and prebiotics (ISAPP) consensus statement on the definition and scope of prebiotics. Nat. Rev. Gastroenterol. Hepatol. 14, 491–502. doi: 10.1038/nrgastro.2017.75
Gibson, M. K., Wang, B., Ahmadi, S., Burnham, C. A., Tarr, P. I., Warner, B. B., et al. (2016). Developmental dynamics of the preterm infant gut microbiota and antibiotic resistome. Nat. Microbiol. 1:16024. doi: 10.1038/nmicrobiol.2016.24
Gigliucci, F., von Meijenfeldt, F. A. B., Knijn, A., Michelacci, V., Scavia, G., Minelli, F., et al. (2018). Metagenomic characterization of the human intestinal microbiota in fecal samples from STEC-infected patients. Front. Cell Infect. Microbiol. 8:25. doi: 10.3389/fcimb.2018.00025
Greenwood, C., Morrow, A. L., Lagomarcino, A. J., Altaye, M., Taft, D. H., Yu, Z., et al. (2014). Early empiric antibiotic use in preterm infants is associated with lower bacterial diversity and higher relative abundance of Enterobacter. J. Pediatr. 165, 23–29. doi: 10.1016/j.jpeds.2014.01.010
Gregory, A. C., Zablocki, O., Zayed, A. A., Howell, A., Bolduc, B., and Sullivan, M. B. (2020). The gut virome database reveals age-dependent patterns of virome diversity in the human gut. Cell Host Microbe 28, 724–40 e8. doi: 10.1016/j.chom.2020.08.003
Groves, H. T., Cuthbertson, L., James, P., Moffatt, M. F., Cox, M. J., and Tregoning, J. S. (2018). Respiratory disease following viral lung infection alters the murine gut microbiota. Front. Immunol. 9:182. doi: 10.3389/fimmu.2018.00182
Groves, H. T., Higham, S. L., Moffatt, M. F., Cox, M. J., and Tregoning, J. S. (2020). Respiratory viral infection alters the gut microbiota by inducing inappetence. mBio 11: e03236-19. doi: 10.1128/mBio.03236-19
Grzeskowiak, L., Collado, M. C., Mangani, C., Maleta, K., Laitinen, K., Ashorn, P., et al. (2012). Distinct gut microbiota in southeastern African and northern European infants. J. Pediatr. Gastroenterol. Nutr. 54, 812–816. doi: 10.1097/MPG.0b013e318249039c
Gurram, B., and Sue, P. K. (2019). Fecal microbiota transplantation in children: current concepts. Curr. Opin. Pediatr. 31, 623–629. doi: 10.1097/MOP.0000000000000787
Hapil, F. Z., and Wingender, G. (2018). The interaction between invariant natural Killer T cells and the mucosal microbiota. Immunology 155, 164–175. doi: 10.1111/imm.12958
Harris, V. C., Haak, B. W., van Hensbroek, M. B., and Wiersinga, W. J. (2017b). The intestinal microbiome in infectious diseases: the clinical relevance of a rapidly emerging field. Open Forum Infect. Dis. 4:ofx144. doi: 10.1093/ofid/ofx144
Harris, V. C., Armah, G., Fuentes, S., Korpela, K. E., Parashar, U., Victor, J. C., et al. (2017a). Significant correlation between the infant gut microbiome and rotavirus vaccine response in rural ghana. J. Infect. Dis. 215, 34–41. doi: 10.1093/infdis/jiw518
Hasegawa, K., Stewart, C. J., Mansbach, J. M., Linnemann, R. W., Ajami, N. J., Petrosino, J. F., et al. (2017). Sphingolipid metabolism potential in fecal microbiome and bronchiolitis in infants: a case-control study. BMC Res. Notes 10:325. doi: 10.1186/s13104-017-2659-9
Hemming, M., Räsänen, S., Huhti, L., Paloniemi, M., Salminen, M., and Vesikari, T. (2013). Major reduction of rotavirus, but not norovirus, gastroenteritis in children seen in hospital after the introduction of RotaTeq vaccine into the national immunization programme in Finland. Eur. J. Pediatr. 172, 739–746. doi: 10.1007/s00431-013-1945-3
Hickman, D., Jones, M. K., Zhu, S., Kirkpatrick, E., Ostrov, D. A., Wang, X., et al. (2014). The effect of malnutrition on norovirus infection. mBio 5, e01032–13. doi: 10.1128/mBio.01032-13
Ho, N. T., Li, F., Lee-Sarwar, K. A., Tun, H. M., Brown, B. P., Pannaraj, P. S., et al. (2018). Meta-analysis of effects of exclusive breastfeeding on infant gut microbiota across populations. Nat. Commun. 9:4169. doi: 10.1038/s41467-018-06473-x
Honda, K., and Littman, D. R. (2016). The microbiota in adaptive immune homeostasis and disease. Nature 535, 75–84. doi: 10.1038/nature18848
Hu, Q., Dai, W., Zhou, Q., Fu, D., Zheng, Y., Wang, W., et al. (2017). Dynamic oropharyngeal and faecal microbiota during treatment in infants hospitalized for bronchiolitis compared with age-matched healthy subjects. Sci. Rep. 7:11266. doi: 10.1038/s41598-017-11311-z
Ianiro, G., Rizzatti, G., Plomer, M., Lopetuso, L., Scaldaferri, F., Franceschi, F., et al. (2018). Bacillus clausii for the treatment of acute diarrhea in children: a systematic review and meta-analysis of randomized controlled trials. Nutrients 10:1074. doi: 10.3390/nu10081074
Ichinohe, T. I, Pang, K., Kumamoto, Y., Peaper, D. R., Ho, J. H., Murray, T. S., et al. (2011). Microbiota regulates immune defense against respiratory tract influenza A virus infection. Proc. Natl. Acad. Sci. U.S.A. 108, 5354–5359. doi: 10.1073/pnas.1019378108
Ivanov, I. I., Atarashi, K., Manel, N., Brodie, E. L., Shima, T., Karaoz, U., et al. (2009). Induction of intestinal Th17 cells by segmented filamentous bacteria. Cell 139, 485–498. doi: 10.1016/j.cell.2009.09.033
James, K., Bottacini, F., Contreras, J. I. S., Vigoureux, M., Egan, M., Motherway, M. O., et al. (2019). Metabolism of the predominant human milk oligosaccharide fucosyllactose by an infant gut commensal. Sci. Rep. 9:15427. doi: 10.1038/s41598-019-51901-7
Jangi, S., and Lamont, J. T. (2010). Asymptomatic colonization by Clostridium difficile in infants: implications for disease in later life. J. Pediatr. Gastroenterol. Nutr. 51, 2–7. doi: 10.1097/MPG.0b013e3181d29767
Jimenez, E., Fernandez, L., Marin, M. L., Martin, R., Odriozola, J. M., Nueno-Palop, C., et al. (2005). Isolation of commensal bacteria from umbilical cord blood of healthy neonates born by cesarean section. Curr. Microbiol. 51, 270–274. doi: 10.1007/s00284-005-0020-3
Johansson, M. E., Jakobsson, H. E., Holmen-Larsson, J., Schutte, A., Ermund, A., Rodriguez-Pineiro, A. M., et al. (2015). Normalization of host intestinal mucus layers requires long-term microbial colonization. Cell Host Microbe 18, 582–592. doi: 10.1016/j.chom.2015.10.007
Jones, M. K., Watanabe, M., Zhu, S., Graves, C. L., Keyes, L. R., Grau, K. R., et al. (2014). Enteric bacteria promote human and mouse norovirus infection of B cells. Science 346, 755–759. doi: 10.1126/science.1257147
Keenan, J. D., Bailey, R. L., West, S. K., Arzika, A. M., Hart, J., Weaver, J., et al. (2018). Azithromycin to reduce childhood mortality in sub-saharan Africa. N. Engl. J. Med. 378, 1583–1592. doi: 10.1056/NEJMoa1715474
Kieser, S., Sarker, S. A., Sakwinska, O., Foata, F., Sultana, S., Khan, Z., et al. (2018). Bangladeshi children with acute diarrhoea show faecal microbiomes with increased Streptococcus abundance, irrespective of diarrhoea aetiology. Environ. Microbiol. 20, 2256–2269. doi: 10.1111/1462-2920.14274
Kim, H., Sitarik, A. R., Woodcroft, K., Johnson, C. C., and Zoratti, E. (2019). Birth mode, breastfeeding, pet exposure, and antibiotic use: associations with the gut microbiome and sensitization in children. Curr. Allergy Asthma Rep. 19:22. doi: 10.1007/s11882-019-0851-9
Kinnebrew, M. A., Ubeda, C., Zenewicz, L. A., Smith, N., Flavell, R. A., and Pamer, E. G. (2010). Bacterial flagellin stimulates Toll-like receptor 5-dependent defense against vancomycin-resistant Enterococcus infection. J. Infect. Dis. 201, 534–543. doi: 10.1086/650203
Klünemann, M., Andrejev, S., Blasche, S., Mateus, A., Phapale, P., Devendran, S., et al. (2021). Bioaccumulation of therapeutic drugs by human gut bacteria. Nature 597, 533–538. doi: 10.1038/s41586-021-03891-8
Korpela, K., Blakstad, E. W., Moltu, S. J., Strommen, K., Nakstad, B., Ronnestad, A. E., et al. (2018). Intestinal microbiota development and gestational age in preterm neonates. Sci. Rep. 8:2453. doi: 10.1038/s41598-018-20827-x
Korpela, K., Salonen, A., Virta, L. J., Kekkonen, R. A., Forslund, K., Bork, P., et al. (2016). Intestinal microbiome is related to lifetime antibiotic use in finnish pre-school children. Nat. Commun. 7:10410. doi: 10.1038/ncomms10410
Kumar, A., Vlasova, A. N., Deblais, L., Huang, H. C., Wijeratne, A., Kandasamy, S., et al. (2018). Impact of nutrition and rotavirus infection on the infant gut microbiota in a humanized pig model. BMC Gastroenterol. 18:93. doi: 10.1186/s12876-018-0810-2
Kwiyolecha, E., Groendahl, B., Okamo, B., Kayange, N., Manyama, F., Kidenya, B. R., et al. (2020). Patterns of viral pathogens causing upper respiratory tract infections among symptomatic children in Mwanza. Tanzania, Sci. Rep. 10:18490. doi: 10.1038/s41598-020-74555-2
La Rosa, P. S., Warner, B. B., Zhou, Y., Weinstock, G. M., Sodergren, E., Hall-Moore, C. M., et al. (2014). Patterned progression of bacterial populations in the premature infant gut. Proc. Natl. Acad. Sci. U.S.A. 111, 12522–12527. doi: 10.1073/pnas.1409497111
Lackraj, T., Kim, J. I., Tran, S. L., and Barnett Foster, D. E. (2016). Differential modulation of flagella expression in enterohaemorrhagic Escherichia coli O157: H7 by intestinal short-chain fatty acid mixes. Microbiology (Reading) 162, 1761–1772. doi: 10.1099/mic.0.000357
Lai, H. H., Chiu, C. H., Kong, M. S., Chang, C. J., and Chen, C. C. (2019). Probiotic Lactobacillus casei: effective for managing childhood diarrhea by altering gut microbiota and attenuating fecal inflammatory markers. Nutrients 11:1150. doi: 10.3390/nu11051150
Langmann, T., Moehle, C., Mauerer, R., Scharl, M., Liebisch, G., Zahn, A., et al. (2004). Loss of detoxification in inflammatory bowel disease: dysregulation of pregnane X receptor target genes. Gastroenterology 127, 26–40. doi: 10.1053/j.gastro.2004.04.019
Larsen, J. M. (2017). The immune response to Prevotella bacteria in chronic inflammatory disease. Immunology 151, 363–374. doi: 10.1111/imm.12760
Laursen, M. F., Andersen, L. B., Michaelsen, K. F., Molgaard, C., Trolle, E., Bahl, M. I., et al. (2016). Infant gut microbiota development is driven by transition to family foods Independent of maternal obesity. mSphere 1, e00069–15. doi: 10.1128/mSphere.00069-15
Laursen, R. P., and Hojsak, I. (2018). Probiotics for respiratory tract infections in children attending day care centers-a systematic review. Eur. J. Pediatr. 177, 979–994. doi: 10.1007/s00431-018-3167-1
Lawley, T. D., Clare, S., Walker, A. W., Stares, M. D., Connor, T. R., Raisen, C., et al. (2012). Targeted restoration of the intestinal microbiota with a simple, defined bacteriotherapy resolves relapsing Clostridium difficile disease in mice. PLoS Pathog. 8:e1002995. doi: 10.1371/journal.ppat.1002995
Lee, D. K., Park, J. E., Kim, M. J., Seo, J. G., Lee, J. H., and Ha, N. J. (2015). Probiotic bacteria, B. longum and L. acidophilus inhibit infection by rotavirus in vitro and decrease the duration of diarrhea in pediatric patients. Clin. Res. Hepatol. Gastroenterol. 39, 237–244. doi: 10.1016/j.clinre.2014.09.006
Lee, W., Kim, M., Lee, S. H., Jung, H. G., and Oh, J. W. (2018). Prophylactic efficacy of orally administered Bacillus poly-gamma-glutamic acid, a non-LPS TLR4 ligand, against norovirus infection in mice. Sci. Rep. 8:8667. doi: 10.1038/s41598-018-26935-y
Lees, E. A., Carrol, E. D., Ellaby, N. A. F., Roberts, P., Corless, C. E., Lenzi, L., et al. (2020). Characterization of circulating clostridium difficile strains, host response and intestinal microbiome in hospitalized children with diarrhea. Pediatr. Infect. Dis. J. 39, 221–228. doi: 10.1097/INF.0000000000002559
Levy, M., Thaiss, C. A., Zeevi, D., Dohnalova, L., Zilberman-Schapira, G., Mahdi, J. A., et al. (2015). Microbiota-modulated metabolites shape the intestinal microenvironment by regulating NLRP6 inflammasome signaling. Cell 163, 1428–1443. doi: 10.1016/j.cell.2015.10.048
Li, D., Breiman, A., Le Pendu, J., and Uyttendaele, M. (2015). Binding to histo-blood group antigen-expressing bacteria protects human norovirus from acute heat stress. Front. Microbiol. 6:659. doi: 10.3389/fmicb.2015.00659
Li, N., Yang, J., Zhang, J., Liang, C., Wang, Y., Chen, B., et al. (2019). Correlation of gut microbiome between ASD children and mothers and potential biomarkers for risk assessment. Genomics Proteomics Bioinformatics 17, 26–38. doi: 10.1016/j.gpb.2019.01.002
Li, X., Gao, X., Hu, H., Xiao, Y., Li, D., Yu, G., et al. (2018). Clinical efficacy and microbiome changes following fecal microbiota transplantation in children with recurrent Clostridium difficile infection. Front. Microbiol. 9:2622. doi: 10.3389/fmicb.2018.02622
Li, Y., Johnson, E. K., Shi, T., Campbell, H., Chaves, S. S., Commaille-Chapus, C., et al. (2021). National burden estimates of hospitalisations for acute lower respiratory infections due to respiratory syncytial virus in young children in 2019 among 58 countries: a modelling study. Lancet Respir. Med. 9, 175–185. doi: 10.1016/S2213-2600(20)30322-2
Li, Z., Quan, G., Jiang, X., Yang, Y., Ding, X., Zhang, D., et al. (2018). Effects of metabolites derived from gut microbiota and hosts on pathogens. Front. Cell. Infect. Microbiol. 8:314. doi: 10.3389/fcimb.2018.00314
Liang, G., Zhao, C., Zhang, H., Mattei, L., Sherrill-Mix, S., Bittinger, K., et al. (2020). The stepwise assembly of the neonatal virome is modulated by breastfeeding. Nature 581, 470–474. doi: 10.1038/s41586-020-2192-1
Libertucci, J., and Young, V. B. (2019). The role of the microbiota in infectious diseases. Nat. Microbiol. 4, 35–45. doi: 10.1038/s41564-018-0278-4
Lim, E. S., Rodriguez, C., and Holtz, L. R. (2018). Amniotic fluid from healthy term pregnancies does not harbor a detectable microbial community. Microbiome 6:87. doi: 10.1186/s40168-018-0475-7
Lim, E. S., Zhou, Y., Zhao, G. I, Bauer, K., Droit, L. I, Ndao, M., et al. (2015). Early life dynamics of the human gut virome and bacterial microbiome in infants. Nat. Med. 21, 1228–1234. doi: 10.1038/nm.3950
Ling, Z., Liu, X., Jia, X., Cheng, Y., Luo, Y., Yuan, L., et al. (2014). Impacts of infection with different toxigenic Clostridium difficile strains on faecal microbiota in children. Sci. Rep. 4:7485. doi: 10.1038/srep07485
Liwen, Z., Yu, W., Liang, M., Kaihong, X., and Baojin, C. (2018). A low abundance of Bifidobacterium but not Lactobacillius in the feces of Chinese children with wheezing diseases. Medicine (Baltimore) 97:e12745. doi: 10.1097/MD.0000000000012745
Lu, Q., Cheng, S., Zhou, M., and Yu, J. (2017). Risk Factors for necrotizing Enterocolitis in neonates: a retrospective case-control study. Pediatr. Neonatol. 58, 165–170. doi: 10.1016/j.pedneo.2016.04.002
Lubiech, K., and Twaruzek, M. (2020). Lactobacillus bacteria in breast milk. Nutrients 12:3783. doi: 10.3390/nu12123783
Lundgren, S. N., Madan, J. C., Emond, J. A., Morrison, H. G., Christensen, B. C., Karagas, M. R., et al. (2018). Maternal diet during pregnancy is related with the infant stool microbiome in a delivery mode-dependent manner. Microbiome 6: 109. doi: 10.1186/s40168-018-0490-8
Lynn, D. J., Benson, S. C., Lynn, M. A., and Pulendran, B. (2021). Modulation of immune responses to vaccination by the microbiota: implications and potential mechanisms. Nat. Rev. Immunol. 1–14. doi: 10.1038/s41577-021-00554-7 [Epub ahead of print].
Maher, S. E., O’Brien, E. C., Moore, R. L., Byrne, D. F., Geraghty, A. A., Saldova, R., et al. (2020). The association between the maternal diet and the maternal and infant gut microbiome: a systematic review. Br. J. Nutr. 1–29. doi: 10.1017/S0007114520000847 [Epub ahead of print].
Malm, M., Hyoty, H., Knip, M., Vesikari, T., and Blazevic, V. (2019). Development of T cell immunity to norovirus and rotavirus in children under five years of age. Sci. Rep. 9:3199. doi: 10.1038/s41598-019-39840-9
Martinez-Gili, L., McDonald, J. A. K., Liu, Z., Kao, D., Allegretti, J. R., Monaghan, T. M., et al. (2020). Understanding the mechanisms of efficacy of fecal microbiota transplant in treating recurrent Clostridioides difficile infection and beyond: the contribution of gut microbial-derived metabolites. Gut Microbes 12:1810531. doi: 10.1080/19490976.2020.1810531
Mathew, S., Smatti, M. K., Al Ansari, K., Nasrallah, G. K., Al Thani, A. A., and Yassine, H. M. (2019). Mixed viral-bacterial infections and their effects on gut microbiota and clinical illnesses in children. Sci. Rep. 9:865. doi: 10.1038/s41598-018-37162-w
Mazzola, G., Murphy, K., Ross, R. P., Di Gioia, D., Biavati, B., Corvaglia, L. T., et al. (2016). Early gut microbiota perturbations following intrapartum antibiotic prophylaxis to prevent group B streptococcal disease. PLoS One 11:e0157527. doi: 10.1371/journal.pone.0157527
McAtee, C. L., Webman, R., Gilman, R. H., Mejia, C., Bern, C., Apaza, S., et al. (2016). Burden of norovirus and rotavirus in children after rotavirus vaccine introduction, Cochabamba, Bolivia. Am. J. Trop. Med. Hyg. 94, 212–217. doi: 10.4269/ajtmh.15-0203
McBurney, M. I., Davis, C., Fraser, C. M., Schneeman, B. O., Huttenhower, C., Verbeke, K., et al. (2019). Establishing what constitutes a healthy human gut microbiome: state of the science, regulatory considerations, and future directions. J. Nutr. 149, 1882–1895. doi: 10.1093/jn/nxz154
McDonald, J. A. K., Mullish, B. H., Pechlivanis, A., Liu, Z., Brignardello, J., Kao, D., et al. (2018). Inhibiting growth of clostridioides difficile by restoring valerate, produced by the intestinal microbiota. Gastroenterology 155, 1495–507 e15. doi: 10.1053/j.gastro.2018.07.014
Millar, M., Seale, J., Greenland, M., Hardy, P., Juszczak, E., Wilks, M., et al. (2017). The microbiome of infants recruited to a randomised placebo-controlled probiotic trial (PiPS Trial). EBioMedicine 20, 255–262. doi: 10.1016/j.ebiom.2017.05.019
Miura, T., Sano, D., Suenaga, A., Yoshimura, T., Fuzawa, M., Nakagomi, T., et al. (2013). Histo-blood group antigen-like substances of human enteric bacteria as specific adsorbents for human noroviruses. J. Virol. 87, 9441–9451. doi: 10.1128/JVI.01060-13
Mizutani, T., Aboagye, S. Y., Ishizaka, A., Afum, T., Mensah, G. I., Asante-Poku, A., et al. (2021). Gut microbiota signature of pathogen-dependent dysbiosis in viral gastroenteritis. Sci. Rep. 11:13945. doi: 10.1038/s41598-021-93345-y
Mullish, B. H., McDonald, J. A. K., Pechlivanis, A., Allegretti, J. R., Kao, D., Barker, G. F., et al. (2019). Microbial bile salt hydrolases mediate the efficacy of faecal microbiota transplant in the treatment of recurrent Clostridioides difficile infection. Gut 68, 1791–1800. doi: 10.1136/gutjnl-2018-317842
Muscogiuri, G., Cantone, E., Cassarano, S., Tuccinardi, D., Barrea, L., Savastano, S., et al. (2019). Gut microbiota: a new path to treat obesity. Int. J. Obes. Suppl. 9, 10–19. doi: 10.1038/s41367-019-0011-7
Nair, H., Simões, E. A., Rudan, I., Gessner, B. D., Azziz-Baumgartner, E., Zhang, J. S. F., et al. (2013). Global and regional burden of hospital admissions for severe acute lower respiratory infections in young children in 2010: a systematic analysis. Lancet 381, 1380–1390. doi: 10.1016/S0140-6736(12)61901-1
Navarro-Garcia, F. (2014). Escherichia coli O104:H4 pathogenesis: an enteroaggregative E. coli/Shiga toxin-producing E. coli explosive cocktail of high virulence. Microbiol. Spectr. 2. doi: 10.1128/microbiolspec.EHEC-0008-2013
Nelson, A. M., Elftman, M. D., Pinto, A. K., Baldridge, M., Hooper, P., Kuczynski, J., et al. (2013). Murine norovirus infection does not cause major disruptions in the murine intestinal microbiota. Microbiome 1:7. doi: 10.1186/2049-2618-1-7
Neu, J., and Pammi, M. (2018). Necrotizing enterocolitis: the intestinal microbiome, metabolome and inflammatory mediators. Semin. Fetal Neonatal Med. 23, 400–405. doi: 10.1016/j.siny.2018.08.001
Ng, K. M., Ferreyra, J. A., Higginbottom, S. K., Lynch, J. B., Kashyap, P. C., Gopinath, S., et al. (2013). Microbiota-liberated host sugars facilitate post-antibiotic expansion of enteric pathogens. Nature 502, 96–99. doi: 10.1038/nature12503
Nicholson, M. R. I, Thomsen, P., Slaughter, J. C., Creech, C. B., and Edwards, K. M. (2015). Novel risk factors for recurrent Clostridium difficile infection in children. J. Pediatr. Gastroenterol. Nutr. 60, 18–22. doi: 10.1097/MPG.0000000000000553
O’Brien, K. L., Baggett, H. C., Abdullah Brooks, W., Feikin, D. R., Hammitt, L. L., Higdon, M. M., et al. (2019). Causes of severe pneumonia requiring hospital admission in children without HIV infection from Africa and Asia: the PERCH multi-country case-control study. Lancet 394, 757–779. doi: 10.1016/S0140-6736(19)30721-4
Olszak, T., An, D., Zeissig, S., Vera, M. P., Richter, J., Franke, A., et al. (2012). Microbial exposure during early life has persistent effects on natural killer T cell function. Science 336, 489–493. doi: 10.1126/science.1219328
Osbelt, L., Thiemann, S., Smit, N., Lesker, T. R., Schroter, M., Galvez, E. J. C., et al. (2020). Variations in microbiota composition of laboratory mice influence Citrobacter rodentium infection via variable short-chain fatty acid production. PLoS Pathog. 16:e1008448. doi: 10.1371/journal.ppat.1008448
Ossa, J. C., Yanez, D., Valenzuela, R., Gallardo, P., Lucero, Y., and Farfan, M. J. (2018). Intestinal inflammation in chilean infants fed with bovine formula vs. breast milk and its association with their gut microbiota. Front. Cell Infect. Microbiol. 8:190. doi: 10.3389/fcimb.2018.00190
Pabst, O., Cerovic, V., and Hornef, M. (2016). Secretory IgA in the coordination of establishment and maintenance of the microbiota. Trends Immunol. 37, 287–296. doi: 10.1016/j.it.2016.03.002
Pacheco, A. R., Curtis, M. M., Ritchie, J. M., Munera, D., Waldor, M. K., Moreira, C. G., et al. (2012). Fucose sensing regulates bacterial intestinal colonization. Nature 492, 113–117. doi: 10.1038/nature11623
Pais, P., Almeida, V., Yilmaz, M., and Teixeira, M. C. (2020). Saccharomyces boulardii: what makes it tick as successful probiotic? J. Fungi (Basel) 6:78. doi: 10.3390/jof6020078
Paone, P., and Cani, P. D. (2020). Mucus barrier, mucins and gut microbiota: the expected slimy partners? Gut 69, 2232–2243. doi: 10.1136/gutjnl-2020-322260
Paparo, L., Tripodi, L., Bruno, C., Pisapia, L., Damiano, C., Pastore, L., et al. (2020). Protective action of Bacillus clausii probiotic strains in an in vitro model of rotavirus infection. Sci. Rep. 10:12636. doi: 10.1038/s41598-020-69533-7
Pausan, M. R., Csorba, C., Singer, G., Till, H., Schopf, V., Santigli, E., et al. (2019). Exploring the Archaeome: detection of archaeal signatures in the human body. Front. Microbiol. 10:2796. doi: 10.3389/fmicb.2019.02796
Perez-Munoz, M. E., Arrieta, M. C., Ramer-Tait, A. E., and Walter, J. (2017). A critical assessment of the “sterile womb” and “in utero colonization” hypotheses: implications for research on the pioneer infant microbiome. Microbiome 5:48. doi: 10.1186/s40168-017-0268-4
Petersson, J., Schreiber, O., Hansson, G. C., Gendler, S. J., Velcich, A., Lundberg, J. O., et al. (2011). Importance and regulation of the colonic mucus barrier in a mouse model of colitis. Am. J. Physiol. Gastrointest. Liver Physiol. 300, G327–G333. doi: 10.1152/ajpgi.00422.2010
Petnicki-Ocwieja, T., Hrncir, T., Liu, Y. J., Biswas, A., Hudcovic, T., Tlaskalova-Hogenova, H., et al. (2009). Nod2 is required for the regulation of commensal microbiota in the intestine. Proc. Natl. Acad. Sci. U.S.A. 106, 15813–15818. doi: 10.1073/pnas.0907722106
Plummer, E. L., Bulach, D. M., Murray, G. L., Jacobs, S. E., Tabrizi, S. N., Garland, S. M., et al. (2018). Gut microbiota of preterm infants supplemented with probiotics: sub-study of the ProPrems trial. BMC Microbiol. 18:184. doi: 10.1186/s12866-018-1326-1
Pop, M., Walker, A. W., Paulson, J., Lindsay, B., Antonio, M., Hossain, M. A., et al. (2014). Diarrhea in young children from low-income countries leads to large-scale alterations in intestinal microbiota composition. Genome Biol. 15:R76. doi: 10.1186/gb-2014-15-6-r76
Rautava, S., Collado, M. C., Salminen, S., and Isolauri, E. (2012). Probiotics modulate host-microbe interaction in the placenta and fetal gut: a randomized, double-blind, placebo-controlled trial. Neonatology 102, 178–184. doi: 10.1159/000339182
Rees, C. M., Pierro, A., and Eaton, S. (2007). Neurodevelopmental outcomes of neonates with medically and surgically treated necrotizing enterocolitis. Arch. Dis. Child Fetal Neonatal Ed. 92, F193–F198. doi: 10.1136/adc.2006.099929
Rehbinder, E. M., Lodrup Carlsen, K. C., Staff, A. C. I, Angell, L., Landro, L., Hilde, K., et al. (2018). Is amniotic fluid of women with uncomplicated term pregnancies free of bacteria? Am. J. Obstet. Gynecol. 219, 289.e1–289.e12. doi: 10.1016/j.ajog.2018.05.028
Restori, K. H., Srinivasa, B. T., Ward, B. J., and Fixman, E. D. (2018). Neonatal immunity, respiratory virus infections, and the development of asthma. Front. Immunol. 9:1249. doi: 10.3389/fimmu.2018.01249
Reyman, M., van Houten, M. A., van Baarle, D., Aatm, B., Man, W. H., Mljn, Chu, et al. (2019). Impact of delivery mode-associated gut microbiota dynamics on health in the first year of life. Nat. Commun. 10:4997. doi: 10.1038/s41467-019-13014-7
Rinninella, E., Raoul, P., Cintoni, M., Franceschi, F., Miggiano, G. A. D., Gasbarrini, A., et al. (2019). What is the healthy gut microbiota composition? A changing ecosystem across age, environment, diet, and diseases. Microorganisms 7:14. doi: 10.3390/microorganisms7010014
Romero, R., Dey, S. K., and Fisher, S. J. (2014). Preterm labor: one syndrome, many causes. Science 345, 760–765. doi: 10.1126/science.1251816
Rosshart, S. P., Vassallo, B. G., Angeletti, D., Hutchinson, D. S., Morgan, A. P., Takeda, K., et al. (2017). Wild mouse gut microbiota promotes host fitness and improves disease resistance. Cell 171, 1015–28 e13. doi: 10.1016/j.cell.2017.09.016
Roswall, J., Olsson, L. M., Kovatcheva-Datchary, P., Nilsson, S., Tremaroli, V., Simon, M. C., et al. (2021). Developmental trajectory of the healthy human gut microbiota during the first 5 years of life. Cell Host Microbe 29, 765–76 e3. doi: 10.1016/j.chom.2021.02.021
Roswall, J., Peng, Y., Feng, Q., Jia, H., Kovatcheva-Datchary, P., Li, Y., et al. (2015). Dynamics and stabilization of the human gut microbiome during the first year of life. Cell Host Microbe 17, 690–703. doi: 10.1016/j.chom.2015.04.004
Ruf, B. R., and Knuf, M. (2014). The burden of seasonal and pandemic influenza in infants and children. Eur. J. Pediatr. 173, 265–276. doi: 10.1007/s00431-013-2023-6
Russell, C. D., Unger, S. A., Walton, M., and Schwarze, J. (2017). The human immune response to respiratory syncytial virus infection. Clin. Microbiol. Rev. 30, 481–502. doi: 10.1128/CMR.00090-16
Russell, J. T., Roesch, L. F. W., Ordberg, M., Ilonen, J., Atkinson, M. A., Schatz, D. A., et al. (2019). Genetic risk for autoimmunity is associated with distinct changes in the human gut microbiome. Nat. Commun. 10:3621. doi: 10.1038/s41467-019-11460-x
Rutayisire, E., Huang, K., Liu, Y., and Tao, F. (2016). The mode of delivery affects the diversity and colonization pattern of the gut microbiota during the first year of infants life: a systematic review. BMC Gastroenterol. 16:86. doi: 10.1186/s12876-016-0498-0
Salminen, S., Collado, M. C., Endo, A., Hill, C., Lebeer, S., Quigley, E. M. M., et al. (2021). The International Scientific Association of Probiotics and Prebiotics (ISAPP) consensus statement on the definition and scope of postbiotics. Nat. Rev. Gastroenterol. Hepatol. 18, 649–667. doi: 10.1038/s41575-021-00440-6
Samb-Ba, B., Mazenot, C., Gassama-Sow, A., Dubourg, G., Richet, H., Hugon, P., et al. (2014). MALDI-TOF identification of the human Gut microbiome in people with and without diarrhea in Senegal. PLoS One 9:e87419. doi: 10.1371/journal.pone.0087419
Schorer, M., Lambert, K., Rakebrandt, N., Rost, F., Kao, K. C., Yermanos, A., et al. (2020). Rapid expansion of Treg cells protects from collateral colitis following a viral trigger. Nat. Commun. 11: 1522. doi: 10.1038/s41467-020-15309-6
Schuijt, T. J., Lankelma, J. M., Scicluna, B. P., de Sousa e Melo, F., Roelofs, J. J., de Boer, J. D., et al. (2016). The gut microbiota plays a protective role in the host defence against pneumococcal pneumonia. Gut 65, 575–583. doi: 10.1136/gutjnl-2015-309728
Sebina, I., and Phipps, S. (2020). The contribution of neutrophils to the pathogenesis of RSV Bronchiolitis. Viruses 12:808. doi: 10.3390/v12080808
Seekatz, A. M., Theriot, C. M., Rao, K., Chang, Y. M., Freeman, A. E., Kao, J. Y., et al. (2018). Restoration of short chain fatty acid and bile acid metabolism following fecal microbiota transplantation in patients with recurrent Clostridium difficile infection. Anaerobe 53, 64–73. doi: 10.1016/j.anaerobe.2018.04.001
Segata, N., Izard, J., Waldron, L., Gevers, D., Miropolsky, L., Garrett, W. S., et al. (2011). Metagenomic biomarker discovery and explanation. Genome Biol. 12:R60. doi: 10.1186/gb-2011-12-6-r60
Sencio, V., Machado, M. G., and Trottein, F. (2021). The lung–gut axis during viral respiratory infections: the impact of gut dysbiosis on secondary disease outcomes. Mucosal Immunol. 14, 296–304. doi: 10.1038/s41385-020-00361-8
Shao, Y., Forster, S. C., Tsaliki, E., Vervier, K., Strang, A., Simpson, N., et al. (2019). Stunted microbiota and opportunistic pathogen colonization in caesarean-section birth. Nature 574, 117–121. doi: 10.1038/s41586-019-1560-1
Shi, Z., Zou, J., Zhang, Z., Zhao, X., Noriega, J., Zhang, B., et al. (2019). Segmented filamentous bacteria prevent and cure rotavirus infection. Cell 179, 644–58 e13. doi: 10.1016/j.cell.2019.09.028
Singh, P., Teal, T. K., Marsh, T. L., Tiedje, J. M., Mosci, R., Jernigan, K., et al. (2015). Intestinal microbial communities associated with acute enteric infections and disease recovery. Microbiome 3:45. doi: 10.1186/s40168-015-0109-2
Sivieri, K., Bassan, J., Peixoto, G., and Monti, R. (2017). Gut microbiota and antimicrobial peptides. Curr. Opin. Food Sci. 13, 56–62. doi: 10.1016/j.cofs.2017.02.010
Steed, A. L., Christophi, G. P., Kaiko, G. E., Sun, L., Goodwin, V. M., Jain, U., et al. (2017). The microbial metabolite desaminotyrosine protects from influenza through type I interferon. Science 357, 498–502. doi: 10.1126/science.aam5336
Stewart, C. J., Ajami, N. J., OBrien, J. L., Hutchinson, D. S., Smith, D. P., Wong, M. C., et al. (2018). Temporal development of the gut microbiome in early childhood from the TEDDY study. Nature 562, 583–588. doi: 10.1038/s41586-018-0617-x
Stockinger, S., Hornef, M. W., and Chassin, C. (2011). Establishment of intestinal homeostasis during the neonatal period. Cell. Mol. Life Sci. 68, 3699–3712. doi: 10.1007/s00018-011-0831-2
Strati, F., Di Paola, M., Stefanini, I., Albanese, D., Rizzetto, L., Lionetti, P., et al. (2016). Age and gender affect the composition of fungal population of the human gastrointestinal tract. Front. Microbiol. 7:1227. doi: 10.3389/fmicb.2016.01227
Su, M., Nie, Y., Shao, R., Duan, S., Jiang, Y., Wang, M., et al. (2018). Diversified gut microbiota in newborns of mothers with gestational diabetes mellitus. PLoS One 13:e0205695. doi: 10.1371/journal.pone.0205695
Swanson, K. S., Gibson, G. R., Hutkins, R., Reimer, R. A., Reid, G., Verbeke, K., et al. (2020). The International Scientific Association for Probiotics and Prebiotics (ISAPP) consensus statement on the definition and scope of synbiotics. Nat. Rev. Gastroenterol. Hepatol. 17, 687–701. doi: 10.1038/s41575-020-0344-2
Szajewska, H., Guarino, A., Hojsak, I., Indrio, F., Kolacek, S., Orel, R., et al. (2020). Use of probiotics for the management of acute gastroenteritis in children: an update. J. Pediatr. Gastroenterol. Nutr. 71, 261–269. doi: 10.1097/MPG.0000000000002751
Tanaka, S., Kobayashi, T., Songjinda, P., Tateyama, A., Tsubouchi, M., Kiyohara, C., et al. (2009). Influence of antibiotic exposure in the early postnatal period on the development of intestinal microbiota. FEMS Immunol. Med. Microbiol. 56, 80–87. doi: 10.1111/j.1574-695X.2009.00553.x
Tanner, S. M., Berryhill, T. F., Ellenburg, J. L., Jilling, T., Cleveland, D. S., Lorenz, R. G., et al. (2015). Pathogenesis of necrotizing enterocolitis: modeling the innate immune response. Am. J. Pathol. 185, 4–16. doi: 10.1016/j.ajpath.2014.08.028
Tapiainen, T., Koivusaari, P., Brinkac, L., Lorenzi, H. A., Salo, J., Renko, M., et al. (2019). Impact of intrapartum and postnatal antibiotics on the gut microbiome and emergence of antimicrobial resistance in infants. Sci. Rep. 9:10635. doi: 10.1038/s41598-019-46964-5
Tauchi, H., Yahagi, K., Yamauchi, T., Hara, T., Yamaoka, R., Tsukuda, N., et al. (2019). Gut microbiota development of preterm infants hospitalised in intensive care units. Benef. Microbes 10, 641–651. doi: 10.3920/BM2019.0003
The, H. C., Florez de Sessions, P., Jie, S., Pham Thanh, D., Thompson, C. N., Nguyen Ngoc Minh, C., et al. (2018). Assessing gut microbiota perturbations during the early phase of infectious diarrhea in Vietnamese children. Gut Microbes 9, 38–54. doi: 10.1080/19490976.2017.1361093
Theriot, C. M., Koenigsknecht, M. J., Carlson, P. E. Jr., Hatton, G. E., Nelson, A. M., Li, B., et al. (2014). Antibiotic-induced shifts in the mouse gut microbiome and metabolome increase susceptibility to Clostridium difficile infection. Nat. Commun. 5:3114. doi: 10.1038/ncomms4114
Theriot, C. M., and Young, V. B. (2015). Interactions between the gastrointestinal microbiome and Clostridium difficile. Annu. Rev. Microbiol. 69, 445–461. doi: 10.1146/annurev-micro-091014-104115
Thiagarajah, J. R., Donowitz, M., and Verkman, A. S. (2015). Secretory diarrhoea: mechanisms and emerging therapies. Nat.Rev. Gastroenterol. Hepatol. 12, 446–457. doi: 10.1038/nrgastro.2015.111
Tiew, P. Y., Mac Aogain, M., Nabm, Ali, Thng, K. X., Goh, K., Lau, K. J. X., et al. (2020). The mycobiome in health and disease: emerging concepts, methodologies and challenges. Mycopathologia 185, 207–231. doi: 10.1007/s11046-019-00413-z
Tirone, C., Pezza, L., Paladini, A., Tana, M., Aurilia, C., Lio, A., et al. (2019). Gut and lung microbiota in preterm infants: immunological modulation and implication in neonatal outcomes. Front. Immunol. 10:2910. doi: 10.3389/fimmu.2019.02910
Tobe, T., Nakanishi, N., and Sugimoto, N. (2011). Activation of motility by sensing short-chain fatty acids via two steps in a flagellar gene regulatory cascade in enterohemorrhagic Escherichia coli. Infect. Immun. 79, 1016–1024. doi: 10.1128/IAI.00927-10
Toro Monjaraz, E. M., Ignorosa Arellano, K. R., Loredo Mayer, A., Palacios-González, B., Cervantes Bustamante, R., and Ramírez Mayans, J. A. (2021). Gut microbiota in mexican children with acute diarrhea: an observational study. Pediatr. Infect. Dis. J. 40, 704–709. doi: 10.1097/INF.0000000000003128
Townsend, E. M., Kelly, L., Muscatt, G., Box, J. D., Hargraves, N., Lilley, D., et al. (2021). The human gut phageome: origins and roles in the human gut microbiome. Front. Cell. Infect. Microbiol. 11:643214. doi: 10.3389/fcimb.2021.643214
Trompette, A., Gollwitzer, E. S., Yadava, K., Sichelstiel, A. K., Sprenger, N., Ngom-Bru, C., et al. (2014). Gut microbiota metabolism of dietary fiber influences allergic airway disease and hematopoiesis. Nat. Med. 20, 159–166. doi: 10.1038/nm.3444
Tyler, A. D., Smith, M. I., and Silverberg, M. S. (2014). Analyzing the human microbiome: a “how to” guide for physicians. Am. J. Gastroenterol. 109, 983–993. doi: 10.1038/ajg.2014.73
Uchiyama, R., Chassaing, B., Zhang, B., and Gewirtz, A. T. (2014). Antibiotic treatment suppresses rotavirus infection and enhances specific humoral immunity. J. Infect. Dis. 210, 171–182. doi: 10.1093/infdis/jiu037
United Nations Inter-agency Group for Child Mortality Estimation (2019). Levels & Trends in Child Mortality: Report 2019, Estimates Developed by the United Nations Inter-Agency Group for Child Mortality Estimation. New York, NY: United Nations Childrens Fund.
van de Pol, J. A., van Best, N., Mbakwa, C. A., Thijs, C., Savelkoul, P. H., Arts, I. C., et al. (2017). Gut colonization by methanogenic archaea is associated with organic dairy consumption in children. Front. Microbiol. 8:355. doi: 10.3389/fmicb.2017.00355
van den Akker, C. H. P., van Goudoever, J. B., Shamir, R., Domellof, M., Embleton, N. D., Hojsak, I., et al. (2020). Probiotics and preterm infants: a position paper by the European society for paediatric gastroenterology hepatology and nutrition committee on nutrition and the European society for paediatric gastroenterology hepatology and nutrition working group for Probiotics and Prebiotics. J. Pediatr. Gastroenterol. Nutr. 70, 664–680. doi: 10.1097/MPG.0000000000002655
Varyukhina, S., Freitas, M., Bardin, S., Robillard, E., Tavan, E., Sapin, C., et al. (2012). Glycan-modifying bacteria-derived soluble factors from Bacteroides thetaiotaomicron and Lactobacillus casei inhibit rotavirus infection in human intestinal cells. Microbes Infect. 14, 273–278. doi: 10.1016/j.micinf.2011.10.007
Vemuri, R., Shankar, E. M., Chieppa, M., Eri, R., and Kavanagh, K. (2020). Beyond just bacteria: functional biomes in the gut ecosystem including Virome, Mycobiome, Archaeome and Helminths. Microorganisms 8:483. doi: 10.3390/microorganisms8040483
Villena, J., Vizoso-Pinto, M. G., and Kitazawa, H. (2016). Intestinal innate antiviral immunity and immunobiotics: beneficial effects against rotavirus infection. Front. Immunol. 7:563. doi: 10.3389/fimmu.2016.00563
Vissing, N. H., Chawes, B. L., Rasmussen, M. A., and Bisgaard, H. (2018). Epidemiology and risk factors of infection in early childhood. Pediatrics 141:e20170933. doi: 10.1542/peds.2017-0933
Wahl, B., O’Brien, K. L., Greenbaum, A., Majumder, A., Liu, L., Chu, Y., et al. (2018). Burden of Streptococcus pneumoniae and Haemophilus influenzae type b disease in children in the era of conjugate vaccines: global, regional, and national estimates for 2000–15. Lancet Glob. Health 6, e744–e757. doi: 10.1016/S2214-109X(18)30247-X
Wandro, S., Osborne, S., Enriquez, C., Bixby, C., Arrieta, A., and Whiteson, K. (2018). The microbiome and metabolome of preterm infant stool are personalized and not driven by health outcomes, including necrotizing enterocolitis and late-onset sepsis. mSphere 3, e104–e118. doi: 10.1128/mSphere.00104-18
Wang, J., Li, F., Wei, H., Lian, Z. X., Sun, R., and Tian, Z. (2014). Respiratory influenza virus infection induces intestinal immune injury via microbiota-mediated Th17 cell-dependent inflammation. J. Exp. Med. 211, 2397–2410. doi: 10.1084/jem.20140625
Wang, X., Li, Y., O’Brien, K. L., Madhi, S. A., Widdowson, M. A., Byass, P., et al. (2020). Global burden of respiratory infections associated with seasonal influenza in children under 5 years in 2018: a systematic review and modelling study. Lancet Glob. Health 8, e497–e510. doi: 10.1016/S2214-109X(19)30545-5
Wang, Y., Li, X., Ge, T., Xiao, Y., Liao, Y., Cui, Y., et al. (2016). Probiotics for prevention and treatment of respiratory tract infections in children: a systematic review and meta-analysis of randomized controlled trials. Medicine (Baltimore) 95:e4509. doi: 10.1097/MD.0000000000004509
Wang, Y., Yin, Y., Chen, X., Zhao, Y., Wu, Y., Li, Y., et al. (2019). Induction of Intestinal Th17 Cells by flagellins from segmented filamentous bacteria. Front. Immunol. 10:2750. doi: 10.3389/fimmu.2019.02750
Ward, D. V., Scholz, M., Zolfo, M., Taft, D. H., Schibler, K. R., Tett, A., et al. (2016). Metagenomic sequencing with strain-level resolution implicates uropathogenic E. coli in necrotizing enterocolitis and mortality in preterm infants. Cell Rep. 14, 2912–2924. doi: 10.1016/j.celrep.2016.03.015
Warner, B. B., Deych, E., Zhou, Y., Hall-Moore, C., Weinstock, G. M., Sodergren, E., et al. (2016). Gut bacteria dysbiosis and necrotising enterocolitis in very low birthweight infants: a prospective case-control study. Lancet 387, 1928–1936.
Wei, Y., Gao, J., Kou, Y., Liu, M., Meng, L., Zheng, X., et al. (2020). The intestinal microbial metabolite desaminotyrosine is an anti-inflammatory molecule that modulates local and systemic immune homeostasis. FASEB J. 34, 16117–16128. doi: 10.1096/fj.201902900RR
Wingender, G., Stepniak, D., Krebs, P., Lin, L., McBride, S., Wei, B., et al. (2012). Intestinal microbes affect phenotypes and functions of invariant natural killer T cells in mice. Gastroenterology 143, 418–428. doi: 10.1053/j.gastro.2012.04.017
Wlodarska, M., Willing, B., Keeney, K. M., Menendez, A., Bergstrom, K. S., Gill, N., et al. (2011). Antibiotic treatment alters the colonic mucus layer and predisposes the host to exacerbated Citrobacter rodentium-induced colitis. Infect. Immun. 79, 1536–1545. doi: 10.1128/IAI.01104-10
Wu, X., Xia, Y., He, F., Zhu, C., and Ren, W. (2021). Intestinal mycobiota in health and diseases: from a disrupted equilibrium to clinical opportunities. Microbiome 9:60. doi: 10.1186/s40168-021-01024-x
Xiong, L., Li, Y., Li, J., Yang, J., Shang, L., He, X., et al. (2021). Intestinal microbiota profiles in infants with acute gastroenteritis caused by Rotavirus and Norovirus infection:a prospective cohort study. Int. J. Infect. Dis. 111, 76–84. doi: 10.1016/j.ijid.2021.08.024
Yarza, P., Yilmaz, P., Pruesse, E., Glockner, F. O., Ludwig, W., Schleifer, K. H., et al. (2014). Uniting the classification of cultured and uncultured bacteria and archaea using 16S rRNA gene sequences. Nat.Rev. Microbiol. 12, 635–645. doi: 10.1038/nrmicro3330
Yassour, M., Vatanen, T., Siljander, H., Hämäläinen, A. M., Härkönen, T., Ryhänen, S. J., et al. (2016). Natural history of the infant gut microbiome and impact of antibiotic treatment on bacterial strain diversity and stability. Sci. Transl. Med. 8:343ra81. doi: 10.1126/scitranslmed.aad0917
Yildiz, S., Mazel-Sanchez, B., Kandasamy, M., Manicassamy, B., and Schmolke, M. (2018). Influenza A virus infection impacts systemic microbiota dynamics and causes quantitative enteric dysbiosis. Microbiome 6:9. doi: 10.1186/s40168-017-0386-z
Yin, Y., Wang, Y., Zhu, L., Liu, W., Liao, N., Jiang, M., et al. (2013). Comparative analysis of the distribution of segmented filamentous bacteria in humans, mice and chickens. ISME J. 7, 615–621. doi: 10.1038/ismej.2012.128
Zhao, Y., Chen, F., Wu, W., Sun, M., Bilotta, A. J., Yao, S., et al. (2018). GPR43 mediates microbiota metabolite SCFA regulation of antimicrobial peptide expression in intestinal epithelial cells via activation of mTOR and STAT3. Mucosal Immunol. 11, 752–762. doi: 10.1038/mi.2017.118
Zheng, N., Gao, Y., Zhu, W., Meng, D., and Walker, W. A. (2020). Short chain fatty acids produced by colonizing intestinal commensal bacterial interaction with expressed breast milk are anti-inflammatory in human immature enterocytes. PLoS One 15:e0229283. doi: 10.1371/journal.pone.0229283
Zhou, Y., Shan, G., Sodergren, E., Weinstock, G., Walker, W. A., and Gregory, K. E. (2015). Longitudinal analysis of the premature infant intestinal microbiome prior to necrotizing enterocolitis: a case-control study. PLoS One 10:e0118632. doi: 10.1371/journal.pone.0118632
Keywords: gut microbiota, necrotizing enterocolitis, Clostridioides difficile, rotavirus, norovirus, diarrheagenic Escherichia coli (DEC), RSV, childhood infections
Citation: George S, Aguilera X, Gallardo P, Farfán M, Lucero Y, Torres JP, Vidal R and O’Ryan M (2022) Bacterial Gut Microbiota and Infections During Early Childhood. Front. Microbiol. 12:793050. doi: 10.3389/fmicb.2021.793050
Received: 11 October 2021; Accepted: 10 December 2021;
Published: 05 January 2022.
Edited by:
Rebeca Martín, INRAE Centre Jouy-en-Josas, FranceReviewed by:
Ana Griselda Binetti, CONICET Instituto de Lactología Industrial (INLAIN), ArgentinaAmir Ardeshir, University of California, Davis, United States
Copyright © 2022 George, Aguilera, Gallardo, Farfán, Lucero, Torres, Vidal and O’Ryan. This is an open-access article distributed under the terms of the Creative Commons Attribution License (CC BY). The use, distribution or reproduction in other forums is permitted, provided the original author(s) and the copyright owner(s) are credited and that the original publication in this journal is cited, in accordance with accepted academic practice. No use, distribution or reproduction is permitted which does not comply with these terms.
*Correspondence: Miguel O’Ryan, moryan@med.uchile.cl