- 1Department of Biology, Faculty of Science, Chiang Mai University, Chiang Mai, Thailand
- 2Graduate School, Chiang Mai University, Chiang Mai, Thailand
- 3School of Microbiology, University College Cork, Cork, Ireland
- 4APC Microbiome Ireland, University College Cork, Cork, Ireland
- 5Teagasc Food Research Centre, Moorepark, Fermoy, Ireland
- 6Research Center in Bioresources for Agriculture, Industry and Medicine, Chiang Mai University, Chiang Mai, Thailand
Bacillus velezensis ML122-2 is an antimicrobial-producing strain isolated from the leaf of Assam tea or Miang [Camellia sinensis var. assamica (J.W.Mast.) Kitam.]. The cell-free supernatant (CFS) of strain ML122-2 exhibits a broad-spectrum antimicrobial activity against various Gram-positive and Gram-negative bacteria as well as the mold Penicillium expansum. The genome of B. velezensis ML122-2 was sequenced and in silico analysis identified three potential bacteriocin-associated gene clusters, that is, those involved in the production of mersacidin, amylocyclicin, and LCI. Furthermore, six gene clusters exhibiting homology (75–100% DNA sequence identity) to those associated with the secondary metabolites bacilysin, bacillibactin, surfactin, macrolactin H, bacillaene, and plipastatin were identified. Individual antimicrobial activities produced by B. velezensis ML122-2 were purified and characterized by Matrix-assisted laser desorption ionization-time of flight (MALDI-TOF) mass spectrometry analysis, revealing three antimicrobial peptides with molecular masses corresponding to surfactin, plipastatin, and amylocyclicin. Transcriptional analysis of specific genes associated with mersacidin (mrsA), amylocyclicin (acnA), plipastatin (ppsA), and surfactin (srfAA) production by B. velezensis ML122-2 showed that the first was not transcribed under the conditions tested, while the latter three were consistent with the presence of the associated peptides as determined by mass spectrometry analysis. These findings demonstrate that B. velezensis ML122-2 has the genetic capacity to produce a wide range of antimicrobial activities that may support a specific community structure and highlight the biotechnological properties of Assam tea.
Introduction
Members of the Bacillus genus, which represent Gram-positive and endospore-forming bacteria, are widespread in a variety of environments including air, soil, aquatic ecosystems, foods, skin, and the gastrointestinal tract of animals (Abriouel et al., 2011). Some species of Bacillus are believed to play a key role in biological control through the production of antimicrobial compounds (e.g., bacteriocins, non-ribosomal polypeptides, and polyketides) and in plant growth promotion, such as Bacillus amyloliquefaciens, Bacillus subtilis, and Bacillus tequilensis (Chen et al., 2007; Gao et al., 2017; Li et al., 2018). The antimicrobial metabolites produced by Bacillus spp. are used in clinical settings to achieve inhibition of pathogens, such as Bacillus cereus, Clostridium difficile, Listeria monocytogenes, and methicillin-resistant Staphylococcus aureus (MRSA; Sabaté and Audisio, 2013; Lv et al., 2020; Rungsirivanich and Thongwai, 2020). Additionally, certain species of Bacillus have been reported to elicit probiotic potential, in particular Bacillus amyloliquefaciens, Bacillus licheniformis, Bacillus pumilus, Bacillus siamensis, and Bacillus subtilis (Du et al., 2018; Jeżewska-Frąckowiak et al., 2019; Rungsirivanich et al., 2020).
Bacteriocins are ribosomally synthesized antimicrobial peptides which exhibit antimicrobial activity mostly against closely related bacterial species (Klaenhammer, 1993). Bacteriocins have been classified into three major classes: Class I bacteriocins are small peptides which undergo post-translational modifications, while Classes II and III are small (0.77–10kDa) and large (>10kDa) unmodified linear antimicrobial proteins, respectively (Abriouel et al., 2011; Cotter et al., 2013; Alvarez-Sieiro et al., 2016). In addition to bacteriocins, several species of Bacillus have been described to produce non-ribosomally synthesized peptides (NRPs) and polyketides (PKs) with antimicrobial properties (Patel et al., 1995; Pathak and Keharia, 2013). NRPs and PKs are synthesized by large multi-modular synthetases, non-ribosomal peptide synthetases (NRPSs), polyketide synthetases (PKSs), or hybrid NRPS/PKS enzymes. NRPSs typically consist of one or more modules, each responsible for the enzymatic incorporation of a specific amino acid in a growing peptide. An individual NRPS module typically consists of three core domains, that is, domains responsible for adenylation, thiolation, and condensation. Similarly, a given PKS enzyme comprises acyl transferase, acyl carrier, and ketosynthase domains (Mootz et al., 2002; Wang et al., 2014; Aleti et al., 2015). Prediction of gene clusters responsible for the biosynthesis of antimicrobial compounds using genome-mining tools has been applied for the identification and subsequent characterization of genes associated with antimicrobial compound production (Medema et al., 2011; Sekurova et al., 2019). BAGEL is a powerful prediction tool aimed at the identification of bacteriocin-associated genes (De Jong et al., 2006). AntiSMASH is a genome database for gene cluster analysis responsible for the synthesis of secondary metabolite compounds, such as NRPs, PKs, and other antimicrobials (Medema et al., 2011; Weber et al., 2015).
Matrix-assisted laser desorption ionization-time of flight mass spectrometry (MALDI-TOF MS) is an analytical technique used for evaluating chemical components which are ionized into charged molecules. It has been applied for identification and analysis of biological molecules, especially proteins and peptides (Singhal et al., 2015). MALDI-TOF MS has also been used to identify and analyze antimicrobial peptides, such as amylocyclicin (Scholz et al., 2014), iturin, fengycin, surfactin (Yang et al., 2015; Théatre et al., 2021), and mersacidin (Viel et al., 2021).
Antimicrobial peptides (AMPs) have received substantial attention as an effective treatment of bacterial infections and as an alternative to antibiotics (Cotter et al., 2005), in many cases supported by their low toxicity to human cells (Yang et al., 2014). Furthermore, specific AMPs have not only been used in the food industry as preservatives but also in agricultural applications as antimicrobial compounds (Dischinger et al., 2014). Several studies have identified antimicrobial-producing Bacillus strains associated with soils and plants and are therefore believed to contribute to the biocontrol of plant pathogens (Shafi et al., 2017; Andrić et al., 2020). The mode of action of bacteriocins may be through interaction with specific membrane receptors causing bacterial membrane disruption and associated electrolyte leakage from bacterial cells, ultimately leading to cell death (Tymoszewska et al., 2017; Perez et al., 2018). In contrast, antibiotics typically act as enzyme inhibitors in DNA replication, protein, and fatty acid synthesis, or cell wall biosynthesis (Zhang et al., 2018; O’Rourke et al., 2020). Previous studies by Rungsirivanich and Thongwai (2020) and Rungsirivanich et al. (2020) revealed the antimicrobial activity of B. velezensis ML122-2 isolated Assam tea [Camellia sinensis var. assamica (J.W.Mast.) Kitam.] leaf surface against S. aureus, including MRSA. Moreover, this strain was also shown to exhibit tannin tolerance and probiotic properties. In the current study, we describe the identification, purification, and characterization of antimicrobial compounds produced by B. velezensis ML122-2 revealing co-production of several distinct antimicrobial compounds. Genome and transcriptional analysis of B. velezensis ML122-2 revealed expression of the corresponding gene clusters for these antimicrobial activities.
Materials and Methods
Bacterial Strain and Growth Condition
B. velezensis ML122-2 (previously named Bacillus siamensis ML122-2; GenBank accession no. MH796212) was isolated from an Assam tea leaf [Camellia sinensis var. assamica (J.W.Mast.) Kitam.] harvested in the Phrae province, Thailand. Strain ML122-2 was grown in tryptic soy broth (TSB, Merck™, Germany) at 37°C with shaking at 150rpm for 24h, as previously described by Rungsirivanich and Thongwai (2020).
Antimicrobial Activity Assay
Antibacterial activity was assayed using an agar well diffusion method according to the modified protocol of Sewify et al. (2017). The indicator bacteria (listed in Table 1) were grown in brain heart infusion (BHI, Oxoid™, Basingstoke, England), de Man, Rogosa, and Sharpe (MRS, Oxoid™, Basingstoke, England), or M17 (Oxoid™, Basingstoke, England) containing 0.5% (w/v) glucose (GM17) broth for pathogenic, lactic acid bacteria (LAB), and Floricoccus penangensis ML061-4, respectively, prior to incubation at 37°C (for pathogenic bacteria) or 30°C (for LAB) overnight. Each culture broth was adjusted to a turbidity equivalent of 0.5 McFarland standard. 100μl of an indicator culture was spread onto the agar surface. The agar diffusion assay was also utilized to evaluate the antifungal potential of B. velezensis ML122-2 employing a method adapted from Yang and Chang (2010). Fungal strains Penicillium digitatum DSM 2731 and Penicillium expansum DSM 1282 were obtained from the DSMZ culture collection (Braunschweig, Germany) and were cultivated on Sabouraud 4% dextrose agar (Sigma-Aldrich™, St. Louis, MO, United States) at 30°C for at least four days or until sporulation occurred. Fungal spore suspensions were prepared by scraping spores from the surface of the mold lawn and suspending the spores in 1/4 strength Ringer’s solution containing 0.8% Tween 80. Approximately 104 to 105 spores/ml were seeded into Sabouraud 4% dextrose semi-solid agar. The agar was punctured using a sterile tip to make a hole with an 8mm diameter. 100μl of B. velezensis ML122-2 filtrate was incorporated into each well, after which plates were incubated at the appropriate temperature for 24–48h. Antimicrobial activity, as observed by a clear (due to lack of fungal growth) zone around the well, was measured in millimeters of clearing zone diameter.
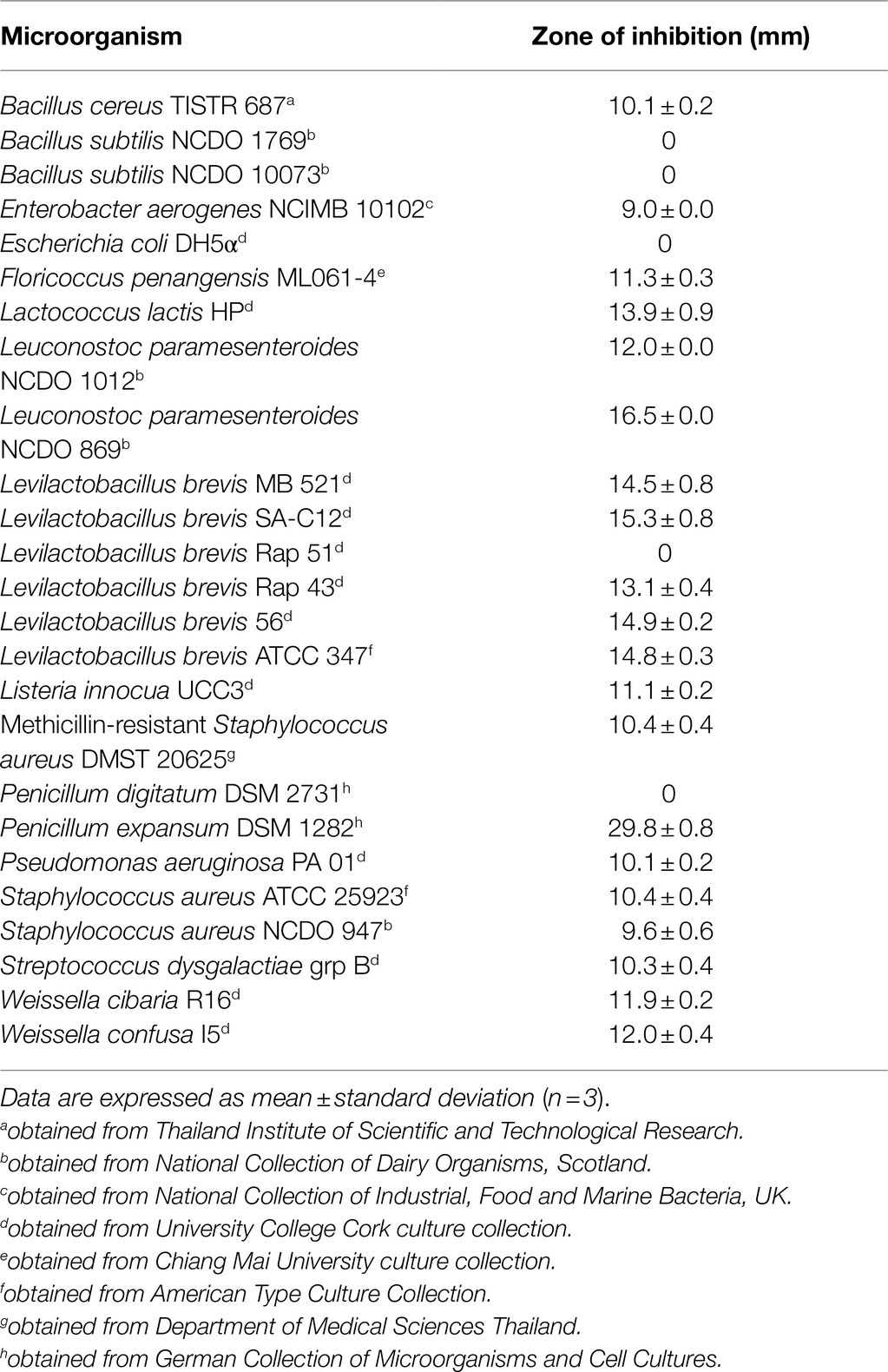
Table 1. Antimicrobial activity of cell-free supernatant (CFS) produced by B. velezensis ML122-2 against indicator microorganisms.
Draft Genome Sequencing and Sequence Analysis
Chromosomal DNA of strain ML122-2 was extracted using a NucleoBond® kit (Macherey-Nagel, Germany). Genome sequencing of B. velezensis ML122-2 was performed using the Pacific Bioscience (PacBio) SMRT RSII sequencing platform (PacBio, Menlo Park, CA, United States). The obtained raw reads were assembled with the Hierarchical Genome Assembly Process (HGAP) pipeline using the protocol RS_Assembly.2 implemented in SMRT Smart Analysis portal v.2.3 (Altschul et al., 1990). Genome sequencing was also performed using an Illumina MiSeq platform by the commercial sequencing service provider Probiogenomics (University of Parma, Italy) using the chromosomal DNA of strain ML122-2, which was extracted using a PureLink™ Genomic DNA extraction kit according to the manufacturer’s instructions (Invitrogen™, CA, United States). Genomic libraries were constructed using the TruSeq DNA PCR-Free LT Kit (Illumina®) and 2.5μg of genomic DNA, which was fragmented with a Bioruptor NGS ultrasonicator (Diagenode, United States) followed by size evaluation using Tape Station 2,200 (Agilent Technologies, Santa Clara, CA, United States). Library samples were loaded into a Flow Cell V3 600 cycles (Illumina®). Fastq files of the paired-end reads (2×250bp) were used as input for genome assemblies through the MEGAnnotator pipeline in default mode (Lugli et al., 2016). Open reading frames prediction was performed by Prodigal v2.6.3 (Strepis et al., 2020). Protein-encoding genes were automatically annotated using a BlastP v2.2.26 (cut-off value of E 0.0001) sequence alignments against the non-redundant protein (nr) database curated by NCBI.1 The bacteriocin/antimicrobial gene clusters were predicted with BAGEL4 software.2 Meanwhile, gene clusters involved in the biosynthesis of secondary metabolites, such as those involved in the production of NRPs, and PKs, were predicted by antiSMASH software.3 The genome sequence was deposited in GenBank under accession number JAGTWM000000000.
Purification and Identification of Antimicrobial Compounds in Cell Fractions
Strain ML122-2 was cultivated in 800ml clarified TSB, which had been passed through a column containing Amberlite XAD-2 resin beads (Sigma-Aldrich™, St. Louis, MO, United States) to remove hydrophobic peptides, and incubated at 150rpm, 37°C for 48h prior to centrifugation at 8,000×g at 4°C for 20min. The resulting cell pellet was removed, and the cell-free supernatant (CFS, ~800ml) was passed through an Econo column containing 30g Amberlite® XAD16N beads (Phenomenex, Cheshire, UK) prewashed with Milli Q water. Following this, the beads were washed with 250ml 40% ethanol (Fisher Scientific, UK), and bound peptides were eluted from the column with 250ml 70% (v/v) isopropanol-containing 0.1% (v/v) trifluoroacetic acid (IPA). In parallel, cells from the corresponding cell pellet were mixed with 250ml IPA and stirred at room temperature for 3–4h. Subsequently, the mixture was centrifuged at 8,000×g at 4°C for 20min. Both IPA eluent and IPA supernatant obtained from CFS and cell pellets, respectively (20ml each), were applied to a 1g Strata-E C18 SPE column (Phenomenex, Cheshire, UK) which was pre-equilibrated with 40% methanol and water. Each column was subsequently washed with 20ml of 40% ethanol and then eluted using 20ml IPA. The C18 SPE IPA eluents were assessed by matrix-assisted laser desorption ionization-time of flight (MALDI-TOF) mass spectrometry (Axima TOF2 MALDI-TOF mass spectrometer, Shimadzu Biotech, Manchester, UK) and the molecular mass of bacteriocins determined in positive ion linear mode according to the protocol described by Hill et al. (2020; Figure 1A).
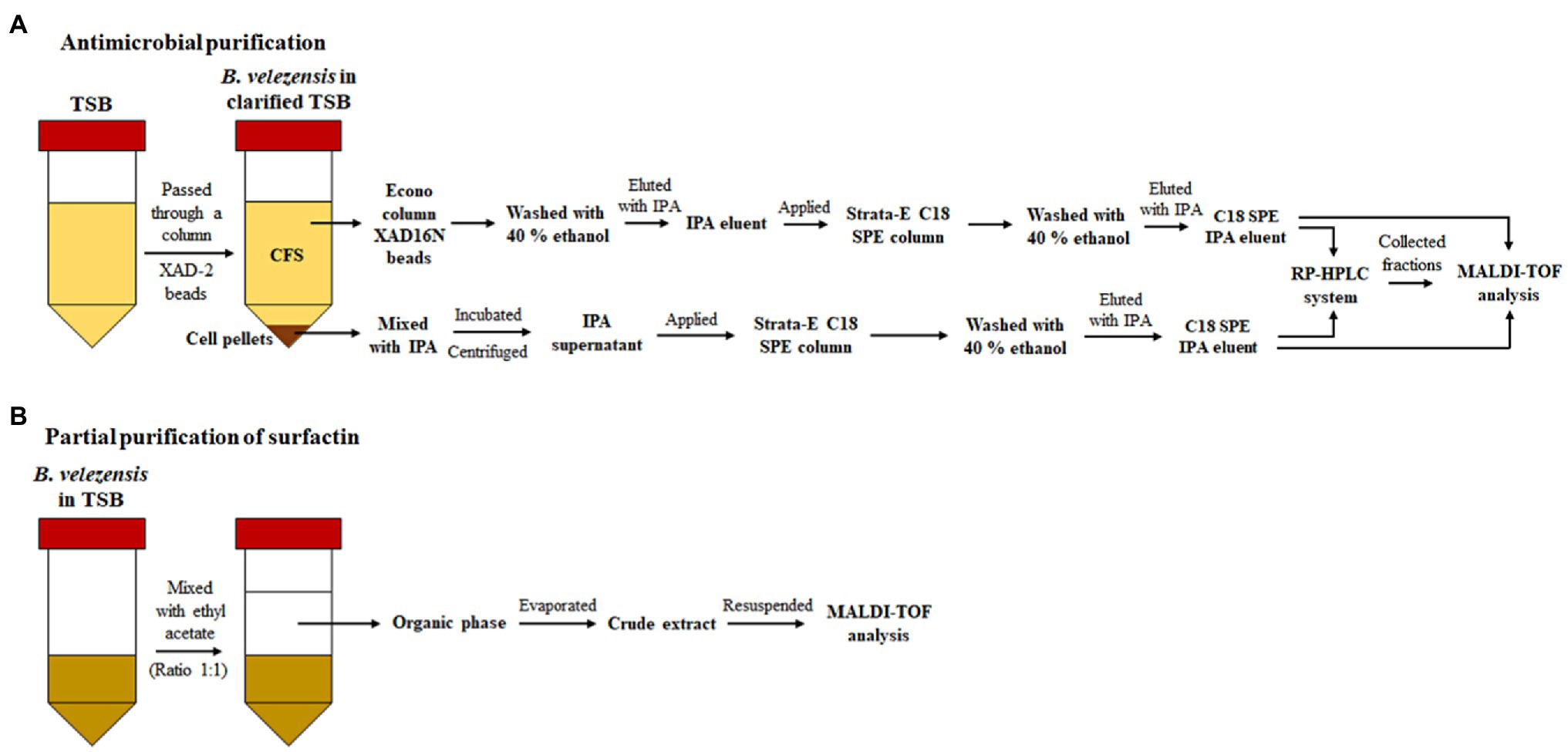
Figure 1. Schematic representation of antimicrobial purification by (A) RP-HPLC and (B) by solvent extraction assay (for the partial purification of surfactin).
RP-HPLC Purification of Antimicrobial Peptides
Antimicrobial peptides (except surfactin, see below) were purified from CFS and cell pellets using C18 SPE and a reversed phase HPLC (RP-HPLC). The C18 SPE IPA eluents obtained as described here (Figure 1A) were applied to a semi prep Proteo Jupiter C12 (250×10mm, 4μ, 90Å) followed by running a 40 to 85% isopropanol 0.1% trifluoroacetic acid (TFA) gradient. Eluent B was 99.9% isopropanol-containing 0.1% TFA at a flow rate of 2.5ml/min. Peptide-containing fractions were detected by measuring the absorbance at 214nm. Fractions that exhibited antimicrobial activity were collected and pooled, subjected to rotary evaporation, and then lyophilized. Each purified antimicrobial peptide was resuspended in 600μl 50% isopropanol (Figure 1A). Antibacterial activity of fractions was assessed in duplicate using 6mm diameter wells, and 25μl of a given fraction/well, and employing Bacillus cereus TISTR 687, B. subtilis NCDO 10073, Escherichia coli DH5α, Listeria innocua UCC3, Leuconostoc paramesenteroides NCDO 869, methicillin-resistant S. aureus (MRSA) DMST 20625, or S. aureus ATCC 25923 as indicator strains. Purified antimicrobial peptides from active fractions were then subjected to MALDI-TOF MS analysis.
Partial Purification of Surfactin From Cell-Free Supernatant
Partial purification of surfactin was achieved by organic solvent extraction according to the protocol described by Lei et al. (2020) with modifications as follows. Strain ML122-2 was cultured in TSB and incubated at 37°C, on a rotating platform at 150rpm for 48h before centrifugation at 5,000×g at 4°C for 15min. The supernatant was subsequently filtered through a 0.20μm nylon membrane filter. 25ml ethyl acetate (Sigma-Aldrich™, St. Louis, MO, United States) was mixed with 25ml filtered cell-free supernatant using a vortex mixer for 10min prior to centrifugation at 5,000×g at 4°C for 60min. Subsequently, the top phase (organic phase), approximately 25ml, was transferred into a glass bottle. Solvent evaporation was performed using Genevac™ miVac centrifugal concentrator (Genevac Limited, Suffolk, UK) at room temperature for 80min. The evaporated solvent extract was resuspended in 0.01M PBS (5ml; Figure 1B). Antibacterial activity was investigated using the agar well diffusion method described above. B. cereus TISTR 687, B. subtilis NCDO 10073, E. coli DH5α, L. innocua UCC3, Leu. paramesenteroides NCDO 869, MRSA DMST 20625, and S. aureus ATCC 25923 were used as the indicator strains. The antimicrobial-containing crude extract was then subjected to MALDI-TOF MS analysis.
RT-qPCR Analysis
Transcriptional activity of genes associated with gene clusters predicted to be responsible for mersacidin, amylocyclicin, plipastatin, and surfactin production was investigated using RT-qPCR analysis, whereby the mrsA, ancA, ppsA, and srfAA genes served as target genes, respectively. The housekeeping gene rpsE was used as reference for this analysis. Primers were designed using Primer3Plus4 and listed in Table 2. B. velezensis ML122-2 was cultivated in TSB and incubated overnight at 37°C on an orbital platform shaker (150rpm) prior to centrifugation at 5000×g at 4°C for 10min. The resulting cell pellet was washed twice with 0.85% (w/v) NaCl and adjusted to an OD600nm of 0.1. A 1% (w/w) of resuspended culture was inoculated into TSB and then incubated at 37°C at 150rpm for 48h. Cells were harvested at 24 and 48h of incubation by centrifugation at 2000×g for 5min. RNA extraction and cDNA synthesis were carried out using High Pure RNA Isolation Kit (Roche Diagnostics GmbH, Mannheim, Germany) and SuperScript™ III Reverse Transcriptase (Invitrogen™, CA, United States), respectively. RT-qPCR analysis of the genes or interest and reference gene were performed using a SYBR Green I Master Mix (Roche Diagnostics GmbH, Mannheim, Germany) on the LightCycler® 480 II System (Roche Diagnostics GmbH, Mannheim, Germany) employing the following PCR conditions: denaturation at 95°C for 10min, followed by 40cycles of 95°C for 10s, 50°C for 15s, and 72°C for 15s. The relative expression level was calculated using the comparative 2−(ΔΔCT) method (Livak and Schmittgen, 2001).
Results
B. velezensis ML122-2 Exhibits a Broad Antimicrobial Range
In a previous study, B. velezensis (formerly Bacillus siamensis) ML122-2 had been demonstrated to exert antimicrobial activity against certain S. aureus strains (Rungsirivanich et al., 2020). Conversely, the strain did not elicit any observable antimicrobial activity against Bacillus cereus TISTR 687 or E. coli O157:H7 DMST 12743. To establish the inhibitory spectrum of this strain, the antimicrobial activity of the strain was evaluated against a panel of 23 bacterial strains and two mold species. The CFS of B. velezensis ML122-2 was investigated using an agar well diffusion method. B. velezensis ML122-2 CFS was demonstrated to inhibit 19 out of 23 assessed bacterial strains, and one out of the two molds tested in agar well diffusion assays with inhibitory/clearing zones ranging between 9.0 and 16.5mm. The CFS of B. velezensis ML122-2 was shown to elicit the most potent antimicrobial activity against Leu. paramesenteroides NCDO 869 and P. expansum DSM 1282 among the assessed bacteria and fungi, respectively, while it was ineffective against B. subtilis NCDO 1769, B. subtilis NCDO 10073, E. coli DH5α, Levilactobacillus brevis Rap 51, and P. digitatum DSM 2731 (Table 1).
The Genome of B. velezensis ML122-2 Harbors Multiple Gene Clusters Associated With Antimicrobial Compound Biosynthesis
B. velezensis ML122-2 exhibits antimicrobial activity against S. aureus ATCC 25923 and MRSA DMST 20625 (Rungsirivanich et al., 2020), as well as various other microorganisms (see results above). This broad range of antimicrobial activity against a panel of microbes prompted an investigation into the nature of the antimicrobial compound(s) produced by this strain based on genome sequence analysis. To identify the antimicrobial compounds that may be produced by this strain, the genome of B. velezensis ML122-2 was sequenced using a combination of Illumina and PacBio sequencing technologies. The chromosome of B. velezensis ML122-2 was assembled into a single contig using a hybrid assembly approach employing the obtained PacBio and Illumina sequence data. This chromosomal contig consists of 4,083,790 base pairs with a 46.61% GC content, and 3,922 predicted open reading frames (ORFs). Congruently, the whole genome of B. velezensis ML122-2 exhibits 98.3% (94% query coverage) and 86.6% (54% query coverage) sequence identity with B. velezensis FZB42 (GenBank accession no. CP000560) and B. subtilis subsp. subtilis str. 168 (GenBank accession no. AL009126), respectively.
B. velezensis ML122-2 was previously assigned to the B. siamensis species based on 16S rRNA gene sequencing (Rungsirivanich et al., 2020). However, it has been suggested that rpoB represents a more robust marker (than the 16S rRNA gene) to determine the phylogeny of bacilli that belong to the so-called “operational group Bacillus amyloliquefaciens,” the latter constituting the closely related species B. amyloliquefaciens, B. velezensis, and B. siamensis (Fan et al., 2017). BlastN analysis of the rpoB gene of strain ML122-2 revealed 100% sequence identity with that of Bacillus velezensis strains and with reduced sequence identity to rpoB of B. siamensis (<98.8%) and B. amyloliquefaciens (<99.8%). This finding confirms that strain ML122-2 belongs to the B. velezensis species rather than B. siamensis. To validate this, the average nucleotide identity (ANI) of ML122-2 was analyzed in comparison with those of strains of the B. amyloliquefaciens, B. siamensis, and B. velezensis species. ML122-2 exhibits ANI values of 97.86, 97.85, and 94.65% with B. velezensis ATR2, B. amyloliquefaciens FBZ42, and B. siamensis SCSIO 05746, respectively. The ML122-2 genome was shown to lack identifiable CRISPR-Cas systems, while it is predicted to contain three prophage-associated regions (13.6, 31.8, and 28.7kb in length, respectively). Two of these appear to represent incomplete prophage regions, while one is predicted to be intact and located within positions 1,213,485-1,245,310 on the genome. This prophage region contains genes predicted to encode DNA replication enzymes, capsid and tail structural components, and lysis functions. BlastN analysis of this putative prophage region highlights that it is highly conserved among the sequenced genomes of B. velezensis strains.
Further in silico analysis was performed using BAGEL4 and antiSMASH to identify genes involved in the production of antimicrobial or bioactive compounds. A total of four putative bacteriocin or bacteriocin-like gene clusters were predicted by BAGEL4 software including those encoding the biosynthetic and immunity genes for mersacidin, amylocyclicin, ComX, and LCI (Table 3). The predicted ML122-2 mersacidin gene cluster was shown to comprise of mrsK2, mrsR2, mrsF, mrsG, mrsE, mrsA, mrsR1, mrsD, mrsM, and mrsT and is similar to that of Bacillus sp. HIL-Y85/54728 (Genbank accession no. AJ250862; 98%) which was previously described by Altena et al. (2000; Figure 2A). Therefore, it appears that a complete mersacidin gene cluster is present in the B. velezensis ML122-2 genome. The ML122-2 genome also contains a gene cluster with high identity (98%) to the amylocyclicin cluster of B. velezensis FZB42 (Scholz et al., 2014; Figure 2B). The comX gene cluster of B. velezensis ML122-2 elicits 35% identity with that of B. velezensis FZB42 which encodes the competence pheromone ComX peptide, while the lci gene encodes a putative antimicrobial peptide, and exhibits 89% identity with the corresponding lci gene of B. velezensis FZB42 (Supplementary Figure 1). Gene clusters with nucleotide sequence similarity values below 30% were deemed insignificant.
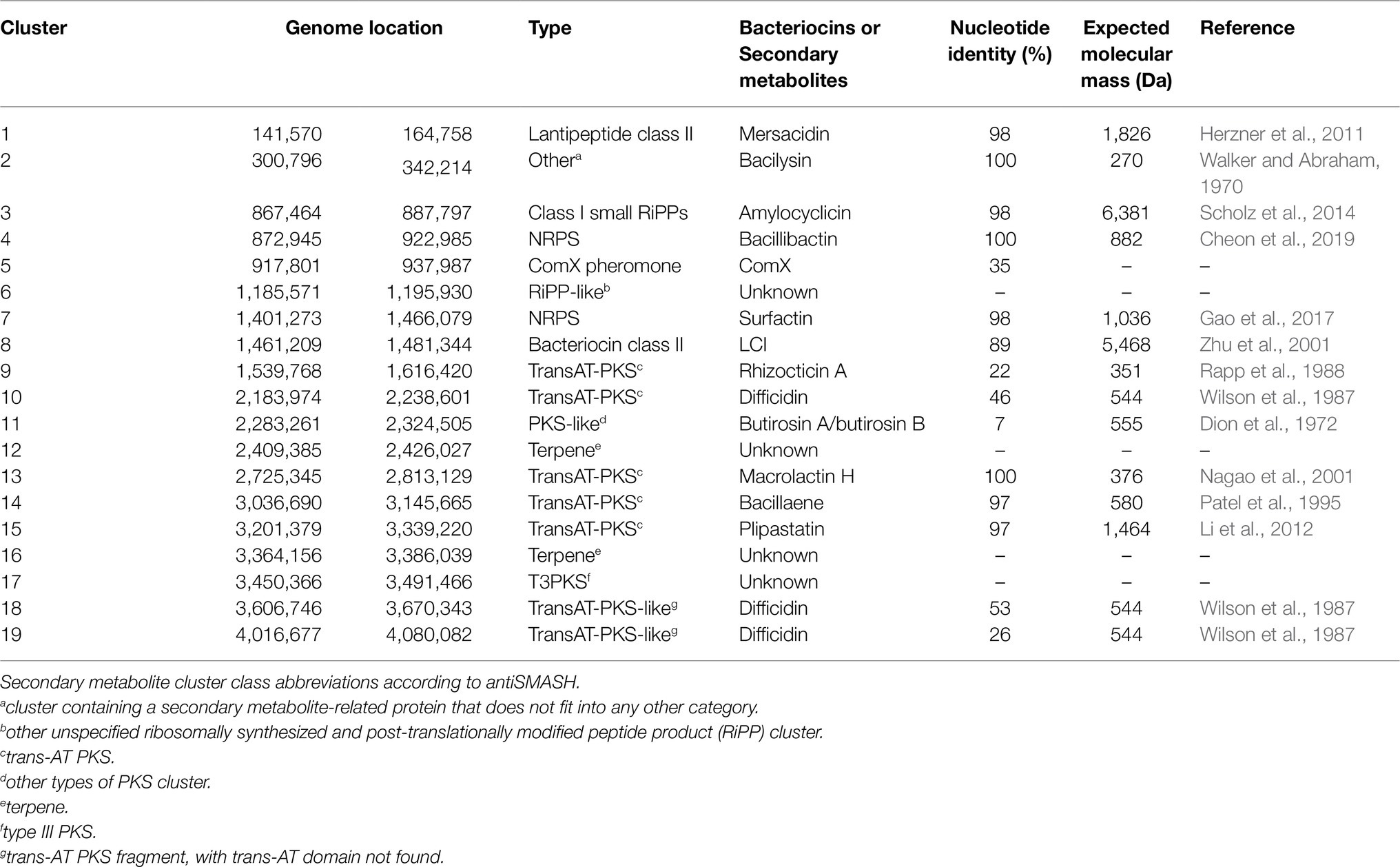
Table 3. Identification of gene clusters involved in the ribosomally synthesized bacteriocins and secondary metabolite synthesis by B. velezensis ML122-2 using BAGEL4 (clusters 1, 3, 5, and 8) and antiSMASH (remainder of presented clusters).
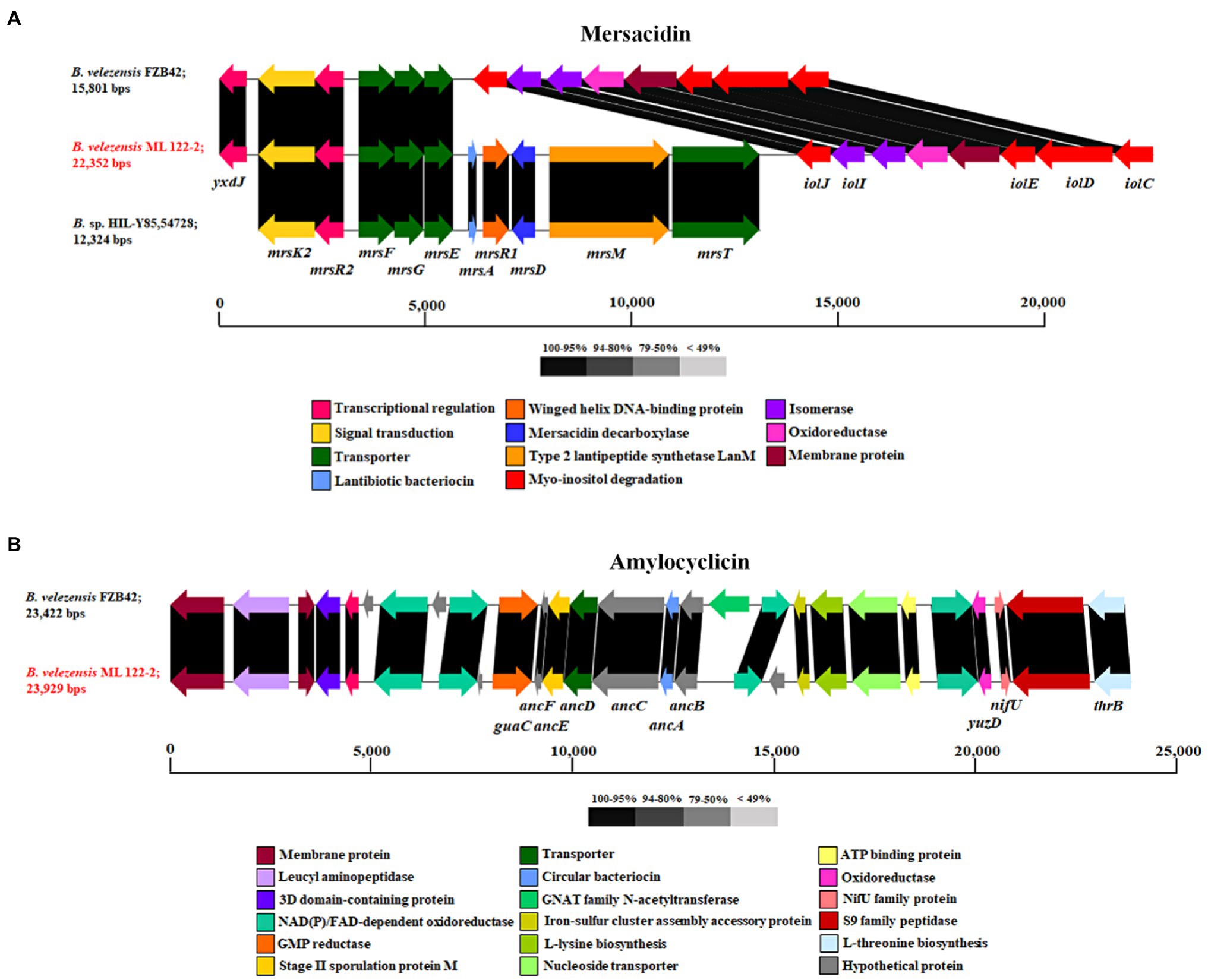
Figure 2. Schematic image of the gene clusters associated with the ribosomally synthesized peptides, Mersacidin (A) and amylocyclicin (B) of strains B. velezensis ML122-2 (red), B. velezensis FZB42 and B. sp. HIL Y-85,54,728. Predicted functions and sequence similarity are color-coded as indicated.
In addition to ribosomally synthesized antimicrobial peptides, Bacillus spp. have been reported to produce non-ribosomally synthesized antimicrobial compounds. Sequence analysis using antiSMASH identified nine gene clusters predicted to be involved in the production of secondary metabolites including NRPs and PKs, of which six were shown to exhibit 75–100% nucleotide identity to known NRP/PK clusters from strains of Bacillus spp. (Table 3). Of these latter six clusters, five are predicted to encode NRPs (bacilysin, bacillibactin, surfactin, macrolactin H, and plipastatin), while the remaining one is associated with the biosynthesis of a PK (bacillaene). Genes associated with macrolactin biosynthesis are typically identified on the genomes of B. velenzensis strains, while they have not been observed among the genomes of B. siamensis or B. amyloliquefaciens strains (Fan et al., 2017). This finding supports the reassignment of this strain as a B. velezensis strain. The bacilysin- and bacillibactin-associated clusters display 100% sequence identity with equivalent clusters in B. velezensis FZB42 which includes seven (bacABCDEFG) and five (dhbACEBF) subunit genes (Figures 3A,B), respectively. The bacillibactin biosynthesis cluster exhibits 75% nucleotide identity with its counterpart in B. subtilis subsp. subtilis str. 168, while the surfactin gene cluster (srfAA, srfAB, srfAC, and srfAD) exhibits 98 and 79% nucleotide identity with B. velezensis FZB42 and B. subtilis JH642, respectively. The genome of B. velezensis ML122-2 was shown to lack the ycxBCD genes located downstream of the sfp gene (Figure 3C). Furthermore, the macrolactin H biosynthesis gene cluster, mlnABCDEFGHI, exhibits 100% sequence identity to those of B. velezensis FZB42, whereas the plipastatin biosynthesis gene cluster, ppsABCDE, displays 97% identity with that of B. velezensis FZB42 (Figures 3D,E). The bacillaene-associated gene cluster shows 97% identity to that of B. velezensis FZB42, which consists of eight subunit genes, baeEDLMNJRS (Figure 3F).
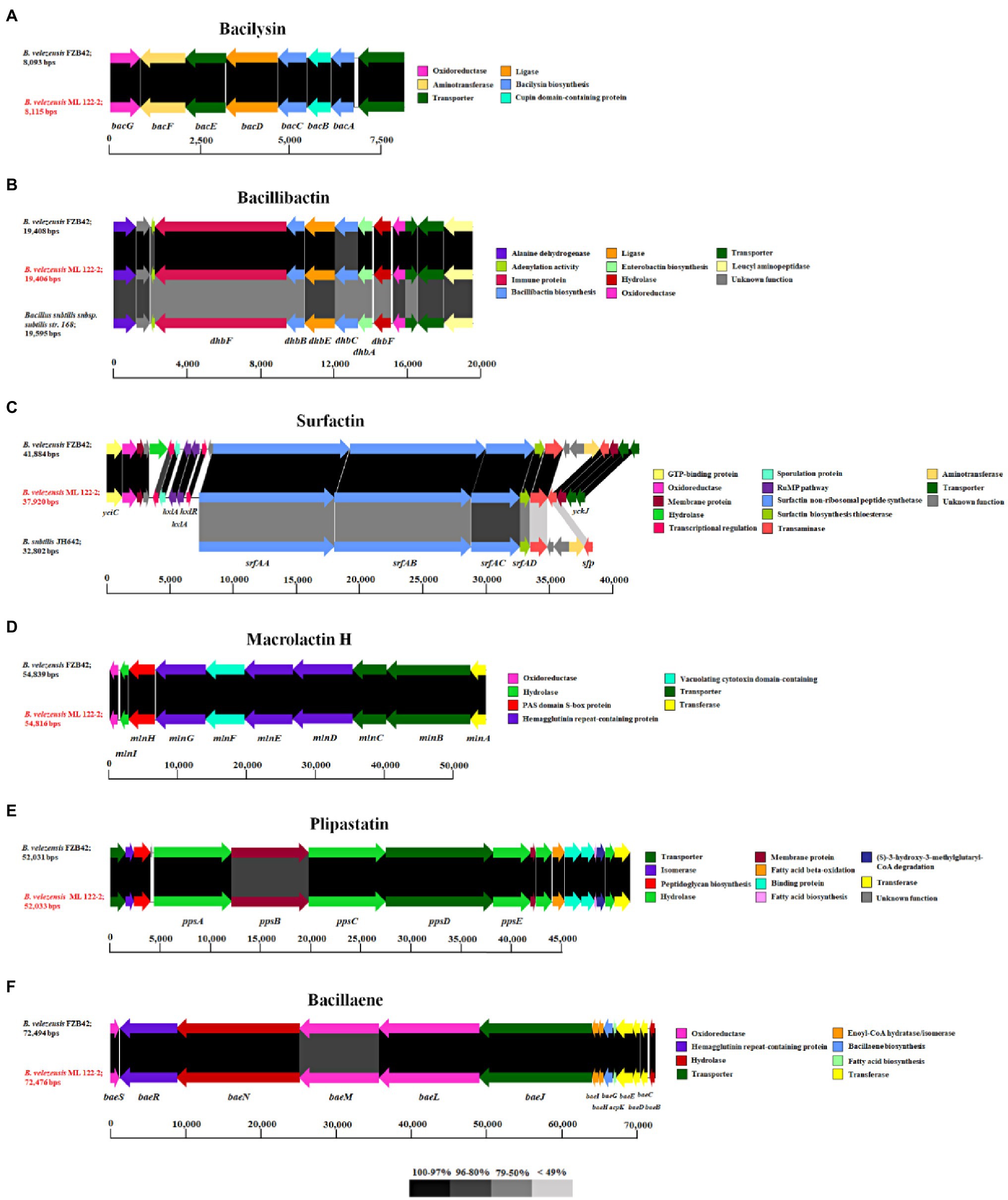
Figure 3. The gene clusters associated with the non-ribosomally synthesized peptides, bacilysin (A), bacillibactin (B), surfactin (C), macrolactin H (D), and plipastatin (E), and polyketide, bacillaene (F), of B. velezensis ML122-2 (red) compared to equivalent clusters in reference Bacillus strains. The predicted functions and sequence similarity are color-coded according to the legend.
Antimicrobial Purification and Mass Spectrometry Analysis
Based on genome analysis, B. velezensis ML122-2 has the genetic capacity to produce a considerable number of distinct antimicrobial compounds. Accordingly, in order to assess which of the predicted antimicrobial compounds are responsible for the observed antimicrobial activity of B. velezensis ML122-2, we characterized the antimicrobial peptides produced in cell pellets and CFS extracts and analyzed the active fractions by MALDI-TOF MS (see “Materials and Methods”; Figure 1). The MALDI-TOF mass spectrum displayed major ion peaks [M+H]+ at m/z values of 1,059.25, 1,464.33, and 6,381.58, which correspond to the deduced molecular masses of surfactin (1,036kDa; Pathak et al., 2014), plipastatin (1,464kDa; Dimkić et al., 2017), and amylocyclicin (6,381kDa; Scholz et al., 2014), respectively (Table 3; Figure 4A).
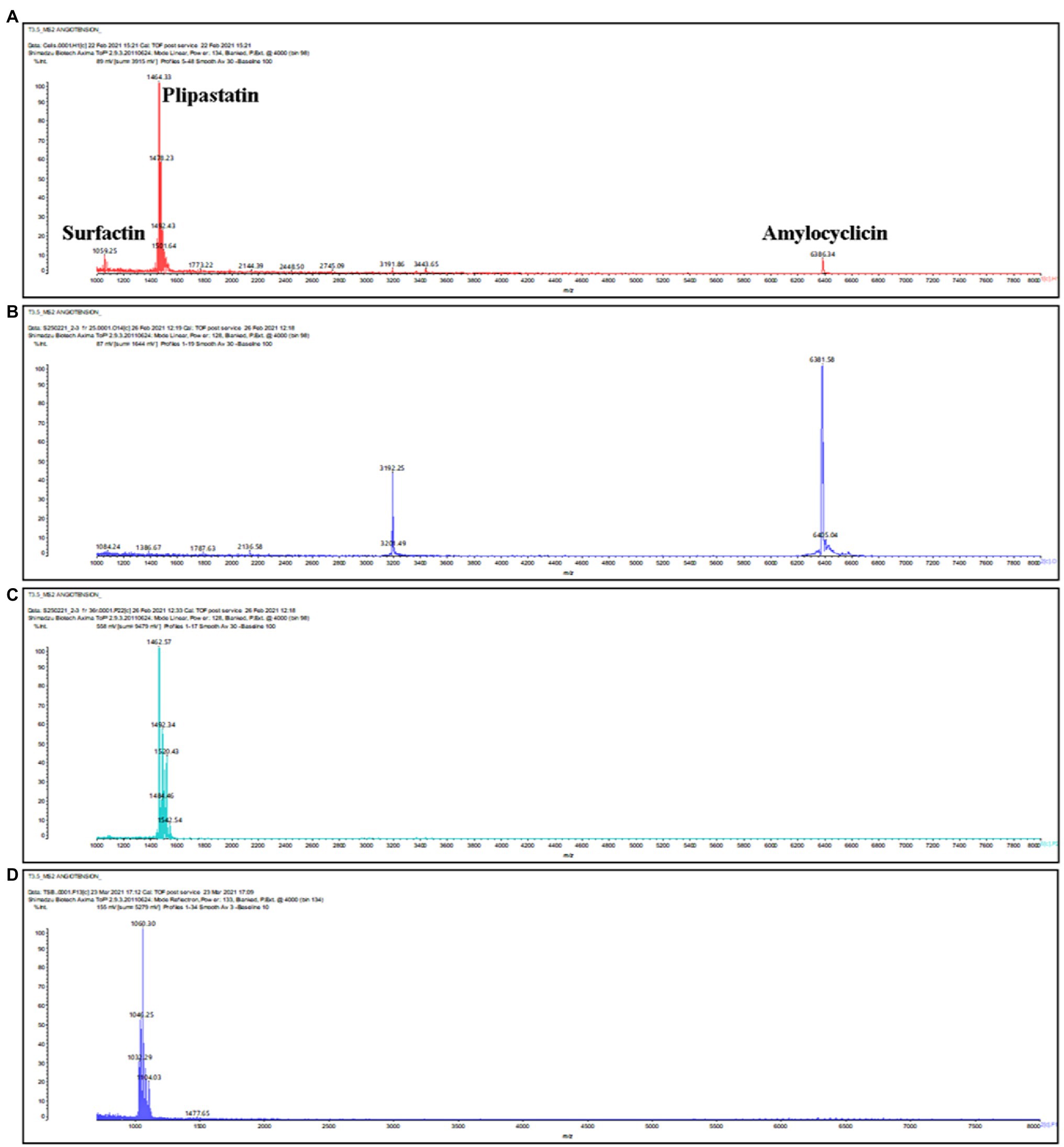
Figure 4. MALDI-TOF mass spectra of IPA supernatant from cell extract of B. velezensis ML122-2 (A). Surfactin, amylocyclicin, and plipastatin were detected at m/z 1,059.25, 1,464.33 and 6,381.58, respectively. (B,C) Purification of amylocyclicin and plipastatin by RP-HPLC. The active fractions eluted at time interval of 25–28 and 31–37min in a gradient of 40–85% propan-2-ol 0.1% trifluoroacetic acid represented the peaks of purified amylocyclicin (m/z 6,381.58 [M+H]+ and 3,192.25, the doubly charged form) and plipastatin (m/z 1,462.57, 1,484.46 1,492.32, 1520.43 and 1542.54), respectively. (D) Partial surfactin purification via solvent extraction of B. velezensis ML122-2 from supernatant revealing corresponding masses at m/z 1,032.29, 1,046.25, 1,060.30 and 1,104.03.
Further purification by RP-HPLC allowed separation of antimicrobial activities in two active fractions, one of which corresponded to ion peaks with m/z values of 1,449.9, 1,463.9, 1,471.9, 1,487.9, 1,485.9, and 1,501.9 (Figure 4B), and one which corresponded to the peaks at m/z 6,381.4 and 3,190.3 (Figure 4C). Since surfactin could not purified by RP-HPLC, possibly due to its inherent hydrophobic nature, (partial) purification of this compound was achieved by solvent extraction with ethyl acetate. Ethyl acetate possesses a lower polarity than isopropanol, which was used in the RP-HPLC purification and may explain its (near) absence in the original purification. Moreover, a previous study revealed that surfactin extraction by ethyl acetate is associated with high purity and yield of the compound (Chen and Juang, 2008). The MALDI-TOF mass spectra of unpurified extract obtained from solvent extraction represented the peaks at m/z 1,032.38, 1,046.25, 1,060.24, and 1,103.99 (Figure 4D). Different molecular weights for purified plipastatin and purified surfactin have previously been described regarding the production of surfactin and fengycins/plipastatin with fatty acid side chains of 15 to 17 carbon atoms in a Bacillus strain (Koumoutsi et al., 2004), resulting in incremental molecular mass increases of 14Da for purified plipastatin and surfactin. A previous study by Pathak et al. (2014) reported the mass spectrum [M+H]+ of surfactin from Bacillus strain at m/z 994.7, 1,008.7, 1,022.7, 1,036.7, 1,064.7, 1,078.7, and 1,092.7 consistent with unsaturated C12-C17 β-hydroxy fatty acids. Similarly, [M+H]+ ions at m/z 1,433.8, 1,447.8, 1,461.8, 1,475.8, and 1,489.9 were assigned to plipastatin isoforms that correspond to unsaturated C14-C18 β-hydroxy fatty acids (Gao et al., 2017). Here, we successfully purified surfactin via solvent extraction. Furthermore, based on peak height, it appeared that cells represented a better source of amylocyclicin and lipopeptides than CFS (Supplementary Figure 2).
Genome analysis identified intact gene clusters associated with the biosynthesis of additional ribosomally (mersacidin) and non-ribosomally (macrolactin, bacillaene, bacilysin, and bacillibactin) synthesized compounds. However, the molecular masses associated with mersacidin, bacilysin, bacillibactin, macrolactin H, and bacillaene (Table 3) were not detected through MALDI-TOF MS in either the crude or purified extracts suggesting that these compounds are not produced under the applied laboratory conditions.
The antibacterial activity of individually purified amylocyclicin, plipastatin, and surfactin against B. cereus TISTR 687, B. subtilis NCDO 10073, E. coli DH5α, L. innocua UCC3, Leu. paramesenteroides NCDO 869, MRSA DMST 20625, and S. aureus ATCC 25923 as determined by the agar well diffusion method is presented in Table 4 and Supplementary Figure 3. The purified amylocyclicin was shown to inhibit growth of all test indicator strains with the inhibitory value ranging between 7.8 and 24.0mm, while purified plipastatin represented antimicrobial activity against B. cereus TISTR 687, Leu. paramesenteroides NCDO 869, MRSA DMST 20625, and S. aureus ATCC 25923, with an associated zone of inhibition ranging from 7.5 to 7.8mm, and with no inhibition observed for B. subtilis NCDO 10073 and L. innocua UCC3. The purified surfactin obtained via solvent extraction was demonstrated to elicit antimicrobial activity against L. innocua UCC 3 and Leu. paramesenteroides NCDO 869, producing a zone of inhibition of 11.8 and 11.7mm, respectively, whereas no inhibition was observed when B. cereus TISTR 687, B. subtilis NCDO 10073, E. coli DH5α, and MRSA DMST 20625 and S. aureus ATCC 25923 were used as indicator bacteria (Table 4; Supplementary Figure 3).
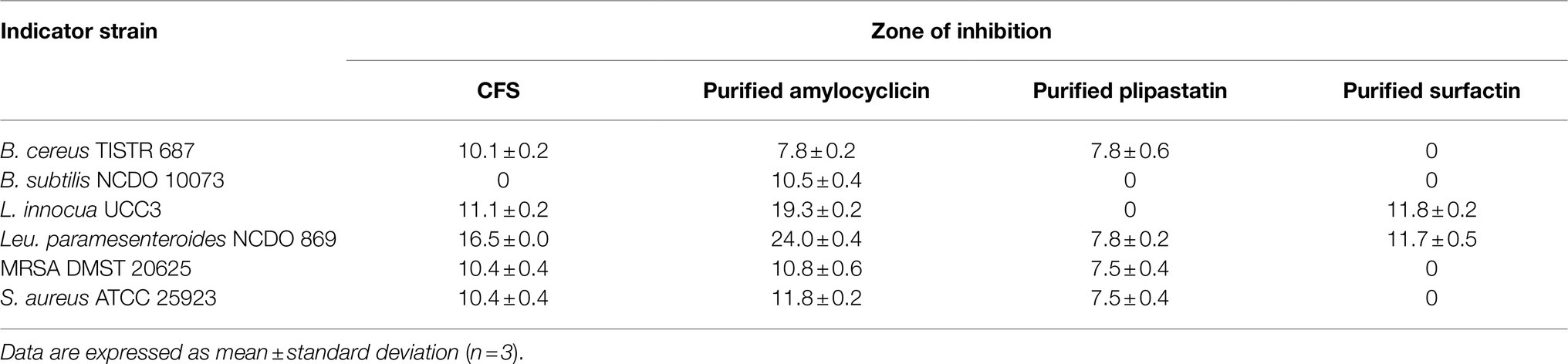
Table 4. Antibacterial activity of CFS, purified amylocyclicin and purified plipastatin and purified surfactin against indicator strains using an agar well diffusion assay.
Transcriptional Activity of Genes Associated With the Gene Clusters
To validate the mass spectrometry-based identification of the (partially) purified compounds, transcriptional analysis of genes associated with amylocyclicin, surfactin, and plipastatin biosynthesis was undertaken. RT-qPCR analysis was performed for variation analysis of specific genes associated with amylocyclicin (acnA), plipastatin (ppsA), and surfactin (srfAA) production at different time points (24 and 48h) of B. velezensis ML122-2 cultivation. The rpsE gene which encodes the 30S-associated ribosomal protein S5 was used as a reference. Furthermore, since mersacidin was not detected (among others) in the analysis, it was selected as a representative negative control for the transcriptional analysis. At 24-h cultivation, ancA, ppsA, and srfAA genes were upregulated 1.57-, 2.80-, and 1.16-fold, while after 48-h incubation, the transcription levels were upregulated 1.23-, 1.75-, and 2.53-fold, respectively. The relative expression level of gene mrsA at 48-h incubation (0.18-fold) was not significant when compared with 24-h incubation (0.15-fold; Figure 5). The low transcription levels measured for the mrsA gene suggest lack of expression of this gene cluster, being consistent with a failure to detect mersacidin.
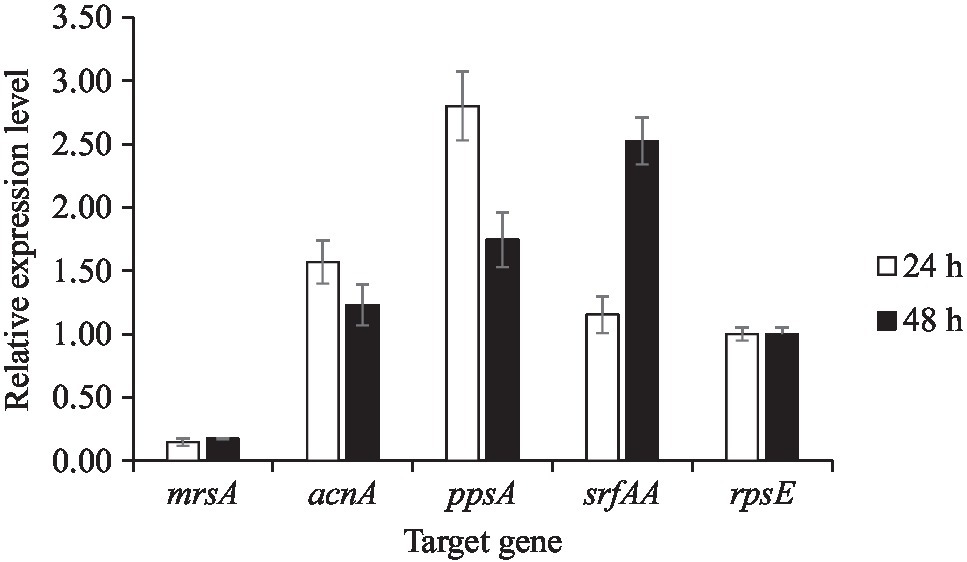
Figure 5. Transcriptional activity of mrsA, ancA, ppsA, and srfAA genes in 24 and 48h of B. velezensis cultivation. The housekeeping gene rpsE was used as the reference gene.
Discussion
A previous study by Rungsirivanich and Thongwai (2020) revealed antibacterial activity of B. velezensis ML122-2 against S. aureus ATCC 25923 and MRSA DMST 20625 which may play a role in microbiological control strategy in Assam tea plantation areas as well as Assam tea fermentation processes. Consequently, the aim of the current study was to identify, purify, and characterize antimicrobial compounds produced by B. velezensis ML122-2 isolated from an Assam tea leaf. Antimicrobial activity assays revealed that various indicator bacteria are inhibited by the CFS of B. velezensis ML122-2. Analysis of the genome sequence of B. velezensis ML122-2 using BAGEL4 identified two distinct bacteriocin gene clusters including those associated with the production of mersacidin and amylocyclicin genes, while six gene clusters potentially involved in the synthesis of secondary metabolites consisting of bacilysin, bacillibactin, surfactin, macrolactin H, bacillaene, and plipastatin identified using antiSMASH. Although the genome of B. velezensis ML122-2 appeared to contain the complete mersacidin gene cluster, mersacidin did not appear to be synthesized by B. velezensis ML122-2 under laboratory conditions which is consistent with the study by Herzner et al. (2011). The failure to detect mersacidin may be due to low expression levels of mrsA and associated genes. Possibly, the expression of the mersacidin-associated genes may be induced under stress conditions or specific media and deserves further investigation. It has previously been shown that inactivation of mrsR1 causes loss of mersacidin production in synthetic medium by inhibiting MrsA synthesis (Guder et al., 2002), while knockouts of mrsR2K2 genes explicitly have been shown to prevent induction of mersacidin transcription (Schmitz et al., 2006).
Several species of Bacillus can produce secondary metabolites with antimicrobial activity against plant pathogenic bacteria and fungi (Lv et al., 2020), and plant growth-promoting activity with plant hormone production ability, such as indole-3-acetic acid (IAA) and 2,3-butanediol (Chen et al., 2007). Various studies have reported the production of multiple antimicrobial compounds by Bacillus strains. A previous study by Han et al. (2018) revealed a broad-spectrum of antimicrobial activity produced by B. amyloliquefaciens WY047 as a result of the simultaneous production of six antimicrobial substances. Gao et al. (2017) presented an engineered B. subtilis pB2-L with the ability to co-produce surfactin and plipastatin. In the current study, B. velezensis ML122-2 was observed to co-produce amylocyclicin, plipastatin, and surfactin. Several reports highlight the role of amylocyclicin (Chen et al., 2009), plipastatin/fengycin, and surfactin (Ongena et al., 2007) in the biological control of plant pathogens. The study by Scholz et al. (2014) revealed that amylocyclicin produced by B. velezensis FZB42 exhibits high antibacterial activity against Gram-positive bacteria (e.g., B. subtilis, B. cereus, Micrococcus luteus, and Paenibacillus granivorans). Surfactin exhibits antimicrobial and emulsification activities and inhibits biofilm formation (Chen et al., 2015). Plipastatin, also known as fengycin, has been reported to demonstrate antibacterial (e.g., L. monocytogenes, S. aureus, and Salmonella Typhimurium) and antifungal (e.g., Fusarium oxysporum and Pythium ultimum) activities causing cellular membrane distortion and cell membrane pore formation and ultimately death of cells (Gao et al., 2017; Jeong et al., 2018; Lin et al., 2020). The ability to produce multiple antimicrobial compounds has been described to increase the potential for biological control (Han et al., 2018). Our findings support the notion that B. velezensis ML122-2 found on Assam tea leaf plays a role in microbiological control in Assam tea or Miang cultivation via the production of antimicrobial peptides (Rungsirivanich et al., 2019). Consequently, B. velezensis that can be found both on Assam tea leaves (Rungsirivanich et al., 2020) and in fermented Assam tea products (Unban et al., 2020) may exert a powerful biocontrol function in environments, preventing food spoilage through the production of antimicrobial compounds, such as amylocyclicin, plipastatin, and surfactin.
Conclusion
B. velezensis ML122-2 exhibits strong and broad-spectrum antimicrobial activity. Three antimicrobial peptides produced by B. velezensis ML122-2, that is, amylocyclicin, plipastatin, and surfactin, were purified from CFS and cell pellets, and their masses confirmed by MALDI-TOF mass spectrometry, this being consistent with transcriptional activity of specific marker genes for the corresponding gene clusters. Each purified peptide was shown to be antimicrobial, with amylocyclicin, in particular, eliciting substantial antimicrobial activity. These findings show that B. velezensis has the potential to play an important role in microbial biocontrol in Assam tea cultivation and Assam tea fermentation.
Data Availability Statement
The datasets presented in this study can be found in online repositories. The names of the repository/repositories and accession number(s) can be found at: https://www.ncbi.nlm.nih.gov/genbank/, JAGTWM000000000, MH796212, CP000560, AL009126, AJ250862, and X70356.
Author Contributions
PR, EP, JM, NT, and DS designed the experiments. PR, EP, PO'C, and DF analyzed the data. PR, JM, and DS investigated the data. NT and DS acquired the funding. PR and NT prepared the original draft. PR, PO'C, JM, NT, and DS reviewed and edited the manuscript. All authors contributed to the article and approved the submitted version.
Funding
This research was funded by the National Research Council of Thailand, grant number PHD60I0089; Tea Gallery Group (Thailand) Co., Ltd.; Amazing Tea Limited Partnership; the scholarship for Teaching Assistant and Research Assistant (TA/RA) awarded by the Graduate School, Chiang Mai University, Thailand and the APC Microbiome Ireland, University College Cork, Ireland. This publication has emanated from research conducted with the financial support of Science Foundation Ireland under Grant numbers 15/SIRG/3430, SFI/12/RC/2273-P1, and SFI/12/RC/2273-P2.
Conflict of Interest
The authors declare this study received funding from Tea Gallery Group (Thailand) Co., Ltd. and Amazing Tea Limited Partnership. The funders were not involved in the study design, collection, analysis, interpretation of data, the writing of this article, or the decision to submit it for publication. All authors declare no other competing interests.
Publisher’s Note
All claims expressed in this article are solely those of the authors and do not necessarily represent those of their affiliated organizations, or those of the publisher, the editors and the reviewers. Any product that may be evaluated in this article, or claim that may be made by its manufacturer, is not guaranteed or endorsed by the publisher.
Acknowledgments
This research was partially supported by the Biology Department, Faculty of Science; the Research Center in Bioresources for Agriculture, Industry, and Medicine, Chiang Mai University, Thailand; the School of Microbiology; and the APC Microbiome Ireland, University College Cork, Ireland.
Supplementary Material
The Supplementary Material for this article can be found online at: https://www.frontiersin.org/articles/10.3389/fmicb.2021.789362/full#supplementary-material
Footnotes
1. ^ftp://ftp.ncbi.nih.gov/blast/db/
2. ^http://bagel4.molgenrug.nl/
3. ^https://antismash.secondarymetabolites.org
4. ^https://www.bioinformatics.nl/cgi-bin/primer3plus/primer3plus.cgi
References
Abriouel, H., Franz, C. M. A. P., Omar, N. B., and Gálvez, A. (2011). Diversity and applications of bacillus bacteriocins. FEMS Microbiol. Rev. 35, 201–232. doi: 10.1111/j.1574-6976.2010.00244.x
Aleti, G., Sessitsch, A., and Brader, G. (2015). Genome mining: prediction of lipopeptides and polyketides from bacillus and related Firmicutes. Comput. Struct. Biotechnol. J. 13, 192–203. doi: 10.1016/j.csbj.2015.03.003
Altena, K., Guder, A., Cramer, C., and Bierbaum, G. (2000). Biosynthesis of the lantibiotic mersacidin: organization of a type B lantibiotic gene cluster. Appl. Environ. Microbiol. 66, 2565–2571. doi: 10.1128/aem.66.6.2565-2571.2000
Altschul, S. F., Gish, W., Miller, W., Myers, E. W., and Lipman, D. J. (1990). Basic local alignment search tool. J. Mol. Biol. 215, 403–410. doi: 10.1016/S0022-2836(05)80360-2
Alvarez-Sieiro, P., Montalban-Lopez, M., Mu, D., and Kuipers, O. P. (2016). Bacteriocins of lactic acid bacteria: extending the family. Appl. Microbiol. Biotechnol. 100, 2939–2951. doi: 10.1007/s00253-016-7343-9
Andrić, S., Meyer, T., and Ongena, M. (2020). Bacillus responses to plant-associated fungal and bacterial communities. Front. Microbiol. 11:1350. doi: 10.3389/fmicb.2020.01350
Chen, H.-L., and Juang, R.-S. (2008). Recovery and separation of surfactin from pretreated fermentation broths by physical and chemical extraction. Biochem. Eng. J. 38, 39–46. doi: 10.1016/j.bej.2007.06.003
Chen, W. C., Juang, R. S., and Wei, Y. H. (2015). Applications of a lipopeptide biosurfactant, surfactin, produced by microorganisms. Biochem. Eng. J. 103, 158–169. doi: 10.1016/j.bej.2015.07.009
Chen, X. H., Koumoutsi, A., Scholz, R., Eisenreich, A., Schneider, K., Heinemeyer, I., et al. (2007). Comparative analysis of the complete genome sequence of the plant growth-promoting bacterium bacillus amyloliquefaciens FZB42. Nat. Biotechnol. 25, 1007–1014. doi: 10.1038/nbt1325
Chen, X. H., Koumoutsi, A., Scholz, R., Schneider, K., Vater, J., Süssmuth, R., et al. (2009). Genome analysis of bacillus amyloliquefaciens FZB42 reveals its potential for biocontrol of plant pathogens. J. Biotechnol. 140, 27–37. doi: 10.1016/j.jbiotec.2008.10.011
Cheon, H. I., Yeo, M. S., Kim, K. M., Kang, J. S., and Pyo, J. (2019). Determination of siderophore from Bacillus mojavensis using liquid chromatography quadrupole time-of-flight tandem mass spectrometry. J. Life Sci. 29, 198–201. doi: 10.5352/JLS.2019.29.2.198
Cotter, P. D., Hill, C., and Ross, R. P. (2005). Bacterial lantibiotics: strategies to improve therapeutic potential. Curr. Protein Pept. Sci. 6, 61–75. doi: 10.2174/1389203053027584
Cotter, P. D., Ross, R. P., and Hill, C. (2013). Bacteriocins - a viable alternative to antibiotics? Nat. Rev. Microbiol. 11, 95–105. doi: 10.1038/nrmicro2937
De Jong, A., van Hijum, S. A., Bijlsma, J. J., Kok, J., and Kuipers, O. P. (2006). BAGEL: a web-based bacteriocin genome mining tool. Nucleic Acids Res. 34, W273–W279. doi: 10.1093/nar/gkl237
Dimkić, I., Stanković, S., Nišavić, M., Petković, M., Ristivojević, P., Fira, D., et al. (2017). The profile and antimicrobial activity of bacillus lipopeptide extracts of five potential biocontrol strains. Front. Microbiol. 8:925. doi: 10.3389/fmicb.2017.00925
Dion, H. W., Woo, P. W., Willmer, N. E., Kern, D. L., Onaga, J., and Fusari, S. A. (1972). Butirosin, a new aminoglycosidic antibiotic complex: isolation and characterization. Antimicrob. Agents Chemother. 2, 84–88. doi: 10.1128/aac.2.2.84
Dischinger, J., Chipalu, S. B., and Bierbaum, G. (2014). Lantibiotics: promising candidates for future applications in health care. Int. J. Med. Microbiol. 304, 51–62. doi: 10.1016/j.ijmm.2013.09.003
Du, R., Jiao, S., Dai, Y., An, J., Lv, J., Yan, X., et al. (2018). Probiotic bacillus amyloliquefaciens C-1 improves growth performance, stimulates GH/IGF-1, and regulates the gut microbiota of growth-retarded beef calves. Front. Microbiol. 9:2006. doi: 10.3389/fmicb.2018.02006
Fan, B., Blom, J., Klenk, H. -P., and Borriss, R. (2017). Bacillus amyloliquefaciens, bacillus velezensis, and Bacillus siamensis form an “operational group B. amyloliquefaciens” within the B. subtilis species complex. Front. Microbiol. 8:22. doi: 10.3389/fmicb.2017.00022
Gao, L., Han, J., Liu, H., Qu, X., Lu, Z., and Bie, X. (2017). Plipastatin and surfactin coproduction by Bacillus subtilis pB2-L and their effects on microorganisms. Antonie Van Leeuwenhoek 110, 1007–1018. doi: 10.1007/s10482-017-0874-y
Guder, A., Schmitter, T., Wiedemann, I., Sahl, H. G., and Bierbaum, G. (2002). Role of the single regulator MrsR1 and the two-component system MrsR2/K2 in the regulation of mersacidin production and immunity. Appl. Environ. Microbiol. 68, 106–113. doi: 10.1128/AEM.68.1.106-113.2002
Han, Y., Li, X., Guo, Y., Sun, W., and Zhang, Q. (2018). Co-production of multiple antimicrobial compounds by bacillus amyloliquefaciens WY047, a strain with broad-spectrum activity. Trans. Tianjin Univ. 24, 160–171. doi: 10.1007/s12209-017-0097-3
Herzner, A. M., Dischinger, J., Szekat, C., Josten, M., Schmitz, S., Yakéléba, A., et al. (2011). Expression of the lantibiotic mersacidin in bacillus amyloliquefaciens FZB42. PLoS One 6:e22389. doi: 10.1371/journal.pone.0022389
Hill, D., O’Connor, P. M., Altermann, E., Day, L., Hill, C., Stanton, C., et al. (2020). Extensive bacteriocin gene shuffling in the Streptococcus bovis/Streptococcus equinus complex reveals gallocin D with activity against vancomycin resistant enterococci. Sci. Rep. 10:13431. doi: 10.1038/s41598-020-70328-z
Jeong, D. E., So, Y., Lim, H., Park, S. H., and Choi, S. K. (2018). Scarless genomic point mutation to construct a Bacillus subtilis strain displaying increased antibiotic plipastatin production. J. Microbiol. Biotechnol. 28, 1030–1036. doi: 10.4014/jmb.1710.10034
Jeżewska-Frąckowiak, J., Żebrowska, J., Czajkowska, E., Jasińska, J., Pęksa, M., Jędrzejczak, G., et al. (2019). Identification of bacterial species in probiotic consortiums in selected commercial cleaning preparations. Acta Biochim. Pol. 66, 215–222. doi: 10.18388/abp.2018_2782
Jordan, S., Junker, A., Helmann, J. D., and Mascher, T. (2006). Regulation of LiaRS-dependent gene expression in Bacillus subtilis: identification of inhibitor proteins, regulator binding sites, and target genes of a conserved cell envelope stress-sensing two-component system. J. Bacteriol. 188, 5153–5166. doi: 10.1128/JB.00310-06
Klaenhammer, T. R. (1993). Genetics of bacteriocins produced by lactic acid bacteria. FEMS Microbiol. Rev. 12, 39–85. doi: 10.1111/j.1574-6976.1993.tb00012.x
Koumoutsi, A., Chen, X.-H., Henne, A., Liesegang, H., Hitzeroth, G., Franke, P., et al. (2004). Structural and functional characterization of gene clusters directing nonribosomal synthesis of bioactive cyclic lipopeptides in bacillus amyloliquefaciens strain FZB42. J. Bacteriol. 186, 1084–1096. doi: 10.1128/JB.186.4.1084-1096.2004
Lei, S., Zhao, R., Sun, J., Ran, J., Ruan, X., and Zhu, Y. (2020). Partial purification and characterization of a broad-spectrum bacteriocin produced by a lactobacillus plantarum zrx03 isolated from infant's feces. Food Sci. Nutr. 8, 2214–2222. doi: 10.1002/fsn3.1428
Li, H., Guan, Y., Dong, Y., Zhao, L., Rong, S., Chen, W., et al. (2018). Isolation and evaluation of endophytic bacillus tequilensis GYLH001 with potential application for biological control of Magnaporthe oryzae. PLoS One 13:e0203505. doi: 10.1371/journal.pone.0203505
Li, X. Y., Mao, Z. C., Wang, Y. H., Wu, Y. X., He, Y. Q., and Long, C. L. (2012). ESI LC-MS and MS/MS characterization of antifungal cyclic lipopeptides produced by Bacillus subtilis XF-1. J. Mol. Microbiol. Biotechnol. 22, 83–93. doi: 10.1159/000338530
Lin, L.-Z., Zheng, Q.-W., Wei, T., Zhang, Z.-Q., Zhao, C.-F., Zhong, H., et al. (2020). Isolation and characterization of fengycins produced by bacillus amyloliquefaciens JFL21 and its broad-spectrum antimicrobial potential against multidrug-resistant foodborne pathogens. Front. Microbiol. 11:579621. doi: 10.3389/fmicb.2020.579621
Livak, K. J., and Schmittgen, T. D. (2001). Analysis of relative gene expression data using real-time quantitative PCR and the 2(-Delta Delta C(T)) method. Methods 25, 402–408. doi: 10.1006/meth.2001.1262
Lugli, G. A., Milani, C., Mancabelli, L., van Sinderen, D., and Ventura, M. (2016). MEGAnnotator: a user-friendly pipeline for microbial genomes assembly and annotation. FEMS Microbiol. Lett. 363:fnw049. doi: 10.1093/femsle/fnw049
Lv, J., Da, R., Cheng, Y., Tuo, X., Wei, J., Jiang, K., et al. (2020). Mechanism of antibacterial activity of bacillus amyloliquefaciens C-1 lipopeptide toward anaerobic Clostridium difficile. Biomed. Res. Int. 2020, 1–12. doi: 10.1155/2020/3104613
Medema, M. H., Blin, K., Cimermancic, P., de Jager, V., Zakrzewski, P., Fischbach, M. A., et al. (2011). antiSMASH: rapid identification, annotation and analysis of secondary metabolite biosynthesis gene clusters in bacterial and fungal genome sequences. Nucleic Acids Res. 39, W339–W346. doi: 10.1093/nar/gkr466
Mootz, H. D., Schwarzer, D., and Marahiel, M. A. (2002). Ways of assembling complex natural products on modular nonribosomal peptide synthetases. Chembiochem 3, 490–504. doi: 10.1002/1439-7633(20020603)3:6<490::AID-CBIC490>3.0.CO;2-N
Nagao, T., Adachi, K., Sakai, M., Nishijima, M., and Sano, H. (2001). Novel macrolactins as antibiotic lactones from a marine bacterium. J. Antibiot. 54, 333–339. doi: 10.7164/antibiotics.54.333
Ongena, M., Jourdan, E., Adam, A., Paquot, M., Brans, A., Joris, B., et al. (2007). Surfactin and fengycin lipopeptides of Bacillus subtilis as elicitors of induced systemic resistance in plants. Environ. Microbiol. 9, 1084–1090. doi: 10.1111/j.1462-2920.2006.01202.x
O’Rourke, A., Beyhan, S., Choi, Y., Morales, P., Chan, A. P., Espinoza, J. L., et al. (2020). Mechanism-of-action classification of antibiotics by global transcriptome profiling. Antimicrob. Agents Chemother. 64, e01207–e01219. doi: 10.1128/AAC.01207-19
Patel, P. S., Huang, S., Fisher, S., Pirnik, D., Aklonis, C., Dean, L., et al. (1995). Bacillaene, a novel inhibitor of procaryotic protein synthesis produced by Bacillus subtilis: production, taxonomy, isolation, physico-chemical characterization and biological activity. J. Antibiot. (Tokyo) 48, 997–1003. doi: 10.7164/antibiotics.48.997
Pathak, K. V., Bose, A., and Keharia, H. (2014). Characterization of novel lipopeptides produced by bacillus tequilensis P15 using liquid chromatography coupled electron spray ionization tandem mass spectrometry (LC-ESI-MS/MS). Int. J. Pept. Res. Ther. 20, 133–143. doi: 10.1007/s10989-013-9375-7
Pathak, K. V., and Keharia, H. (2013). Identification of surfactins and iturins produced by potent fungal antagonist, Bacillus subtilis K1 isolated from aerial roots of banyan (Ficus benghalensis) tree using mass spectrometry. 3. Biotech 4, 283–295. doi: 10.1007/s13205-013-0151-3
Perez, R. H., Zendo, T., and Sonomoto, K. (2018). Circular and leaderless bacteriocins: biosynthesis, mode of action, applications, and prospects. Front. Microbiol. 9:2085. doi: 10.3389/fmicb.2018.02085
Rapp, C., Jung, G., Kugler, M., and Loeffler, W. (1988). Rhizocticins - new phosphono-oligopeptides with antifungal activity. Liebigs Ann. Chem. 1988, 655–661. doi: 10.1002/jlac.198819880707
Rungsirivanich, P., Inta, A., Tragoolpua, Y., and Thongwai, N. (2019). Partial rpoB gene sequencing identification and probiotic potential of Floricoccus penangensis ML061-4 isolated from Assam tea (Camellia sinensis var. assamica). Sci. Rep. 9:16561. doi: 10.1038/s41598-019-52979-9
Rungsirivanich, P., Supandee, W., Futui, W., Chumsai-Na-Ayudhya, V., Yodsombat, C., and Thongwai, N. (2020). Culturable bacterial community on leaves of Assam tea (Camellia sinensis var. assamica) in Thailand and human probiotic potential of isolated bacillus spp. Microorganisms. 8:1585. doi: 10.3390/microorganisms8101585
Rungsirivanich, P., and Thongwai, N. (2020). Antibacterial activity and tannin tolerance of bacillus spp. isolated from leaves of Miang (Camellia sinensis (L.) Kuntze var. assamica (J.W. mast.) Kitam.). Int. J. Biosci. Biochem. Bioinform. 10, 26–33. doi: 10.17706/ijbbb.2020.10.1.26-33
Sabaté, D. C., and Audisio, M. C. (2013). Inhibitory activity of surfactin, produced by different Bacillus subtilis subsp. subtilis strains, against listeria monocytogenes sensitive and bacteriocin-resistant strains. Microbiol. Res. 168, 125–129. doi: 10.1016/j.micres.2012.11.004
Schmitz, S., Hoffmann, A., Szekat, C., Rudd, B., and Bierbaum, G. (2006). The lantibiotic mersacidin is an autoinducing peptide. Appl. Environ. Microbiol. 72, 7270–7277. doi: 10.1128/AEM.00723-06
Scholz, R., Vater, J., Budiharjo, A., Wang, Z., He, Y., Dietel, K., et al. (2014). Amylocyclicin, a novel circular bacteriocin produced by bacillus amyloliquefaciens FZB42. J. Bacteriol. 196, 1842–1852. doi: 10.1128/JB.01474-14
Sekurova, O. N., Schneider, O., and Zotchev, S. B. (2019). Novel bioactive natural products from bacteria via bioprospecting, genome mining and metabolic engineering. Microb. Biotechnol. 12, 828–844. doi: 10.1111/1751-7915.13398
Sewify, G. H., Hamada, H. M., and Alhadrami, H. A. (2017). In vitro evaluation of antimicrobial activity of alimentary canal extracts from the red palm weevil, Rhynchophorus ferrugineus Olivier larvae. Biomed. Res. Int. 2017, 1–6. doi: 10.1155/2017/8564601
Shafi, J., Tian, H., and Ji, M. (2017). Bacillus species as versatile weapons for plant pathogens: a review. Biotechnol. Biotechnol. Equip. 31, 446–459. doi: 10.1080/13102818.2017.1286950
Singhal, N., Kumar, M., Kanaujia, P. K., and Virdi, J. S. (2015). MALDI-TOF mass spectrometry: an emerging technology for microbial identification and diagnosis. Front. Microbiol. 6:791. doi: 10.3389/fmicb.2015.00791
Strepis, N., Naranjo, H. D., Meier-Kolthoff, J., Göker, M., Shapiro, N., Kyrpides, N., et al. (2020). Genome-guided analysis allows the identification of novel physiological traits in Trichococcus species. BMC Genomics 21:24. doi: 10.1186/s12864-019-6410-x
Théatre, A., Cano-Prieto, C., Bartolini, M., Laurin, Y., Deleu, M., Niehren, J., et al. (2021). The surfactin-like lipopeptides from bacillus spp.: natural biodiversity and synthetic biology for a broader application range. Front. Bioeng. Biotechnol. 9:623701. doi: 10.3389/fbioe.2021.623701
Tymoszewska, A., Diep, D. B., Wirtek, P., and Aleksandrzak-Piekarczyk, T. (2017). The non-lantibiotic bacteriocin garvicin Q targets man-PTS in a broad spectrum of sensitive bacterial genera. Sci. Rep. 7:8359. doi: 10.1038/s41598-017-09102-7
Unban, K., Kochasee, P., Shetty, K., and Khanongnuch, C. (2020). Tannin-tolerant and extracellular tannase producing bacillus isolated from traditional fermented tea leaves and their probiotic functional properties. Foods 9:490. doi: 10.3390/foods9040490
Viel, J. H., Jaarsma, A. H., and Kuipers, O. P. (2021). Heterologous expression of mersacidin in Escherichia coli elucidates the mode of leader processing. ACS Synth. Biol. 10, 600–608. doi: 10.1021/acssynbio.0c00601
Walker, J. E., and Abraham, E. P. (1970). The structure of bacilysin and other products of Bacillus subtilis. Biochem. J. 118, 563–570. doi: 10.1042/bj1180563
Wang, H., Fewer, D. P., Holm, L., Rouhiainen, L., and Sivonen, K. (2014). Atlas of nonribosomal peptide and polyketide biosynthetic pathways reveals common occurrence of nonmodular enzymes. Proc. Natl. Acad. Sci. U. S. A. 111, 9259–9264. doi: 10.1073/pnas.1401734111
Weber, T., Blin, K., Duddela, S., Krug, D., Kim, H. U., Bruccoleri, R., et al. (2015). antiSMASH 3.0-a comprehensive resource for the genome mining of biosynthetic gene clusters. Nucleic Acids Res. 43, W237–W243. doi: 10.1093/nar/gkv437
Wilson, K. E., Flor, J. E., Schwartz, R. E., Joshua, H., Smith, J. L., Pelak, B. A., et al. (1987). Difficidin and oxydifficidin: novel broad spectrum antibacterial antibiotics produced by Bacillus subtilis. II. Isolation and physico-chemical characterization. J. Antibiot 40, 1682–1691. doi: 10.7164/antibiotics.40.1682
Yang, E. J., and Chang, H. C. (2010). Purification of a new antifungal compound produced by lactobacillus plantarum AF1 isolated from kimchi. Int. J. Food Microbiol. 139, 56–63. doi: 10.1016/j.ijfoodmicro.2010.02.012
Yang, H., Li, X., Li, X., Yu, H., and Shen, Z. (2015). Identification of lipopeptide isoforms by MALDI-TOF-MS/MS based on the simultaneous purification of iturin, fengycin, and surfactin by RP-HPLC. Anal. Bioanal. Chem. 407, 2529–2542. doi: 10.1007/s00216-015-8486-8
Yang, S. C., Lin, C. H., Sung, C. T., and Fang, J. Y. (2014). Antibacterial activities of bacteriocins: application in foods and pharmaceuticals. Front. Microbiol. 5:241. doi: 10.3389/fmicb.2014.00241
Zhang, Y., Sun, J., Zhang, J., Liu, Y., and Guo, L. (2018). Enzyme inhibitor antibiotics and antibiotic-associated diarrhea in critically ill patients. Med. Sci. Monit. 24, 8781–8788. doi: 10.12659/MSM.913739
Keywords: amylocyclicin, bacteriocin, biocontrol, gene cluster, Miang, plipastatin, RT-qPCR, surfactin
Citation: Rungsirivanich P, Parlindungan E, O’Connor PM, Field D, Mahony J, Thongwai N and van Sinderen D (2021) Simultaneous Production of Multiple Antimicrobial Compounds by Bacillus velezensis ML122-2 Isolated From Assam Tea Leaf [Camellia sinensis var. assamica (J.W.Mast.) Kitam.]. Front. Microbiol. 12:789362. doi: 10.3389/fmicb.2021.789362
Edited by:
Abd El-Latif Hesham, Assiut University, EgyptReviewed by:
Sachio Tsuchida, Nihon University Itabashi Hospital, JapanDennis Ken Bideshi, California Baptist University, United States
Copyright © 2021 Rungsirivanich, Parlindungan, O’Connor, Field, Mahony, Thongwai and van Sinderen. This is an open-access article distributed under the terms of the Creative Commons Attribution License (CC BY). The use, distribution or reproduction in other forums is permitted, provided the original author(s) and the copyright owner(s) are credited and that the original publication in this journal is cited, in accordance with accepted academic practice. No use, distribution or reproduction is permitted which does not comply with these terms.
*Correspondence: Narumol Thongwai, bnRob25nd0Bob3RtYWlsLmNvbQ==; Douwe van Sinderen, ZC52YW5zaW5kZXJlbkB1Y2MuaWU=