- 1Department of Infectious Diseases, Leiden University Medical Center, Leiden, Netherlands
- 2U.S. Department of Health and Human Services, Health Resources and Services Administration, Health Systems Bureau, National Hansen Disease Programme (NHDP), Baton Rouge, LA, United States
- 3Department Cell and Chemical Biology, Leiden University Medical Center, Leiden, Netherlands
- 4Department of Pathobiological Sciences, Louisiana State University, Baton Rouge, LA, United States
Leprosy is an infectious disease caused by Mycobacterium leprae with tropism for skin and peripheral nerves. Incessant transmission in endemic areas is still impeding elimination of leprosy. Although detection of M. leprae infection remains a challenge in asymptomatic individuals, the presence of antibodies specific for phenolglycolipid-I (PGL-I) correlate with bacterial load. Therefore, serosurveillance utilizing field-friendly tests detecting anti-PGL-I antibodies, can be applied to identify those who may transmit bacteria and to study (reduction of) M. leprae transmission. However, serology based on antibody detection cannot discriminate between past and present M. leprae infection in humans, nor can it detect individuals carrying low bacillary loads. In humans, anti-PGL-I IgM levels are long-lasting and usually detected in more individuals than anti-PGL-I IgG levels. Inherent to the characteristically long incubation time of leprosy, IgM/IgG relations (antibody kinetics) in leprosy patients and infected individuals are not completely clear. To investigate the antibody response directly after infection, we have measured antibody levels by ELISA, in longitudinal samples of experimentally M. leprae infected, susceptible nine-banded armadillos (Dasypus novemcinctus). In addition, we assessed the user- and field-friendly, low-cost lateral flow assay (LFA) utilizing upconverting reporter particles (UCP), developed for quantitative detection of human anti-PGL-I IgM (UCP-LFA), to detect treatment- or vaccination-induced changes in viable bacterial load. Our results show that serum levels of anti-PGL-I IgM, and to a lesser extent IgG, significantly increase soon after experimental M. leprae infection in armadillos. In view of leprosy phenotypes in armadillos, this animal model can provide useful insight into antibody kinetics in early infection in the various spectral forms of human leprosy. The UCP-LFA for quantitative detection of anti-PGL-I IgM allows monitoring the efficacy of vaccination and rifampin-treatment in the armadillo leprosy model, thereby providing a convenient tool to evaluate the effects of drugs and vaccines and new diagnostics.
Introduction
Leprosy is a severe, communicable disease characterized by skin lesions and peripheral nerve alterations associated with a wide-ranging spectrum of clinical symptoms. Worldwide, millions of people are coping with the long term consequences of this dermato-neurological disease and more than 200,000 new patients are annually diagnosed, making it the second most common mycobacterial disease after tuberculosis (WHO, 2020). The causative pathogens of leprosy, Mycobacterium leprae and Mycobacterium lepromatosis, are obligate intracellular bacteria which grow optimally in humans at lower body temperature and have a tropism for Schwann cells (Scollard et al., 2006; Eichelmann et al., 2013). If left untreated, leprosy can lead to irreversible nerve damage and lifelong handicaps and deformities in humans.
Leprosy elimination is still hampered by intense transmission of Mycobacterium leprae, which is not restricted to patients’ households (Ortuno-Gutierrez et al., 2019; Tió-Coma et al., 2020). At the individual level, detection of M. leprae infection in persons without symptoms is essential to detect patients at early, still well-treatable stages of leprosy. On a community scale, on the other hand, detection of M. leprae infection bears relevance to monitoring transmission in an area on the way to elimination of this disease, and can help to assess the effects of prophylactic community interventions. Interruption of M. leprae transmission is crucial and concomitantly are tests to detect asymptomatically infected individuals who can still perpetuate transmission.
Characteristic for leprosy is its disease spectrum, with on one end tuberculoid leprosy (TT) associated with Th1 and Th17 T cell immune responses, and on the other end lepromatous leprosy (LL) (Hungria et al., 2018; van Hooij and Geluk, 2021), the more severe type of leprosy with disseminated infection generally linked to anti-inflammatory Th2 responses (Salgame et al., 1991; Quaresma et al., 2015; Aarão et al., 2016). Moreover, LL patients, also referred to as multibacillary (MB) leprosy, display well detectable antibody levels, in particular IgM directed against M. leprae PGL-I, whereas IgG and IgA are detected mostly in lower levels. Although anti-PGL-I IgM seropositivity increases the risk of leprosy (Gormus et al., 2000), it does not predict disease (Richardus et al., 2017; TiemiNagao-Dias et al., 2019). The much less frequently observed anti-PGL-I IgG seropositivity, however, has been described to be associated with disease development (TiemiNagao-Dias et al., 2019). As severe infection is associated with high IgM antibody levels, it is improbable that these convey protective anti-M. leprae immunity. Given the correlation between anti-PGL-I IgM levels and bacterial load (Tió-Coma et al., 2020; van Hooij et al., 2020), this humoral immunity biomarker is useful to detect M. leprae infection as well as diagnose MB patients (van Hooij et al., 2017, 2019, 2021). Studies in humans on the kinetics of antibody production directly after M. leprae infection, including quantitative and qualitative differences between IgM and IgG, have been considerably constrained due to the facts that anti-PGL-I immunity cannot discriminate between past and present M. leprae infection (Pierneef et al., 2021), most humans do not develop disease after M. leprae infection and leprosy has a long incubation time (Scollard et al., 2006).
Six-banded (Euphractus sexcinctus) as well as nine-banded (Dasypus novemcinctus) armadillos represent a potential environmental reservoir for zoonotic transmission of M. leprae (Truman et al., 2011; Sharma et al., 2015; da Silva et al., 2018; da Silva Ferreira et al., 2020). In 1971, it was demonstrated that the nine-banded armadillo developed leprosy after inoculation with M. leprae (Job and Truman, 2000). Due to their low body temperature of 32–35°C, the infection disseminates to all body tissues resulting in high bacterial loads in the spleen, liver, and lymph nodes. Similar to humans, susceptibility to leprosy varies resulting in resistance in 15–20% of M. leprae infected armadillos (Balamayooran et al., 2015). Immunologically, leprosy in armadillos also resembles human leprosy as shown by the IFN-γ responses to a variety of recombinant M. leprae proteins in peripheral blood mononuclear cells (PBMCs) from M. leprae infected armadillos (Geluk et al., 2011; Pena et al., 2011). Thus, armadillos provide a useful animal model to study leprosy-specific neuropathy (Adams et al., 2012; Sharma et al., 2013; Truman et al., 2014; Balamayooran et al., 2015; Oliveira et al., 2019), skin test reagents (Duthie et al., 2020b) and vaccines against leprosy (Duthie et al., 2018).
ID93/GLA-SE is a tuberculosis candidate vaccine, composed of a fusion protein (ID93) consisting of four Mycobacterium tuberculosis antigens (Rv1813c, Rv2608, Rv3619c, and Rv3620c) combined with a synthetic Toll-like receptor 4 agonist: GLA-SE adjuvant (Glucopyranosyl Lipid Adjuvant in stable emulsion) (Bertholet et al., 2008; Day et al., 2021). In mice ID93/GLA-SE provides cross-protection against M. leprae infection (Duthie et al., 2014). LepVax (LEP-F1 adjuvanted with GLA-SE), a leprosy candidate vaccine (Duthie et al., 2020a) was formulated with a fusion protein LEP-F1 (named ML89 during preclinical development; comprised of ML2351, ML2055, ML2380, and ML2028 antigens) combined with GLA-SE. LepVax was tested in armadillos and showed reduction of M. leprae bacillary load and delayed M. leprae-induced motor nerve damage (Duthie et al., 2018).
To gain more insight into the kinetics of anti-PGL-I antibody formation directly after M. leprae infection and the meaning of differential seropositivity for IgM and IgG, we have measured both antibody levels by ELISA in longitudinal samples of experimentally M. leprae inoculated, susceptible nine-banded armadillos. In addition, we have evaluated the potential of a point-of-care (POC) test for quantitative detection of human anti-PGL-I IgM (UCP-LFA) for detection of anti-PGL-I antibodies in M. leprae infected armadillos early after infection, monitoring antibody levels during the course of infection and after treatment as well as to discriminate between resistant and susceptible animals. Successful use of the UCP-LFA in armadillos with known time points of infection, bears relevance to similar applications in humans especially in remote and resource limited leprosy endemic settings.
Materials and Methods
Ethics
The present studies were performed on armadillo serum samples, which were banked from previous M. leprae infection experiments at the National Hansen’s Disease Program (NHDP) (Duthie et al., 2018; Hazbón et al., 2018). Armadillo experiments were conducted in accordance with procedures approved by the NHDP Animal Care and Use Committee [Assurance No. D16-00019 (A3032-01)]. Based on estimates of anticipated variation of rodent models of M. leprae infection and on prior experience with the experimental systems, the armadillos were distributed randomly in different groups. The resulting group sizes provide proper statistical analysis while allowing minimal animal usage.
Animal Husbandry
Wild-caught armadillos are mainly adult animals that were captured alive in the wild and brought to the NHDP vivarium where they were housed in modified rabbit cages (Pena et al., 2016) for conditioning before experimental inoculation. During conditioning, the animals were treated for bacterial and parasitic infections and microchipped. Before experimental inoculation, the animals were screened for serum antibodies to M. leprae PGL-I to determine whether there was natural infection with M. leprae. Each animal was also lepromin tested to determine immune responsiveness to M. leprae. The lepromin test coincides with histopathology which ranges across the entire spectrum of leprosy (Adams et al., 2012). Only armadillos showing a negative lepromin response (indicating susceptibility to M. leprae) were experimentally infected with M. leprae. Regarding captive-born armadillos, pregnant females were caught in the wild and kept in captivity where they give birth to four genetically identical siblings. These young armadillos were brought to the NHDP vivarium at about 4 months of age and placed in rabbit cages for a period of conditioning of about 12–24 months. At this time the animals were microchipped, dewormed, screened for anti-PGL-I, and lepromin tested. The average age at infection was about 24 months.
Armadillo Cohort
The armadillo cohort from which serum samples were analyzed contained 52 nine-banded armadillos (Dasypus novemcinctus) and was comprised of both captive-born and wild-caught animals which were experimentally infected with one of three strains of M. leprae [NHDP 63 (n = 33), NHDP 98 (n = 7), or Brazil 4923 (n = 11)], and one animal was infected in the wild. Out of the M. leprae infected armadillos, 17 were untreated and unvaccinated and could be divided further into three groups based on full dissemination time as described (Pena et al., 2016): highly susceptible (within 12 months), susceptible (within 24 months), and resistant (lack of dissemination at 36 months); BCG vaccination was given to 8 armadillos 1 month pre- (n = 6) or post- (n = 2) infection; 24 armadillos were vaccinated with ID93 or LepVax 1–32 months post-infection; three armadillos were treated for 3 months with rifampin 8 or 10 months post-infection. ID93 = Rv1813c, Rv2608, Rv3619c, and Rv3620c adjuvanted with GLA-SE; LepVax = ML2351, ML2055, ML2380, and ML2028 adjuvanted with GLA-SE. Sampling time points were divided into ti: time of inoculation; tc: time of seroconversion (anti-PGL-I IgM ELISA > 0.45 OD450–background; for highly susceptible and susceptible animals); tv: time of vaccination; tr: time of rifampin treatment; tm: mid-stage disease; tl: late-stage disease; ts: time at sacrifice (Figure 1 and Supplementary Tables 1, 2).
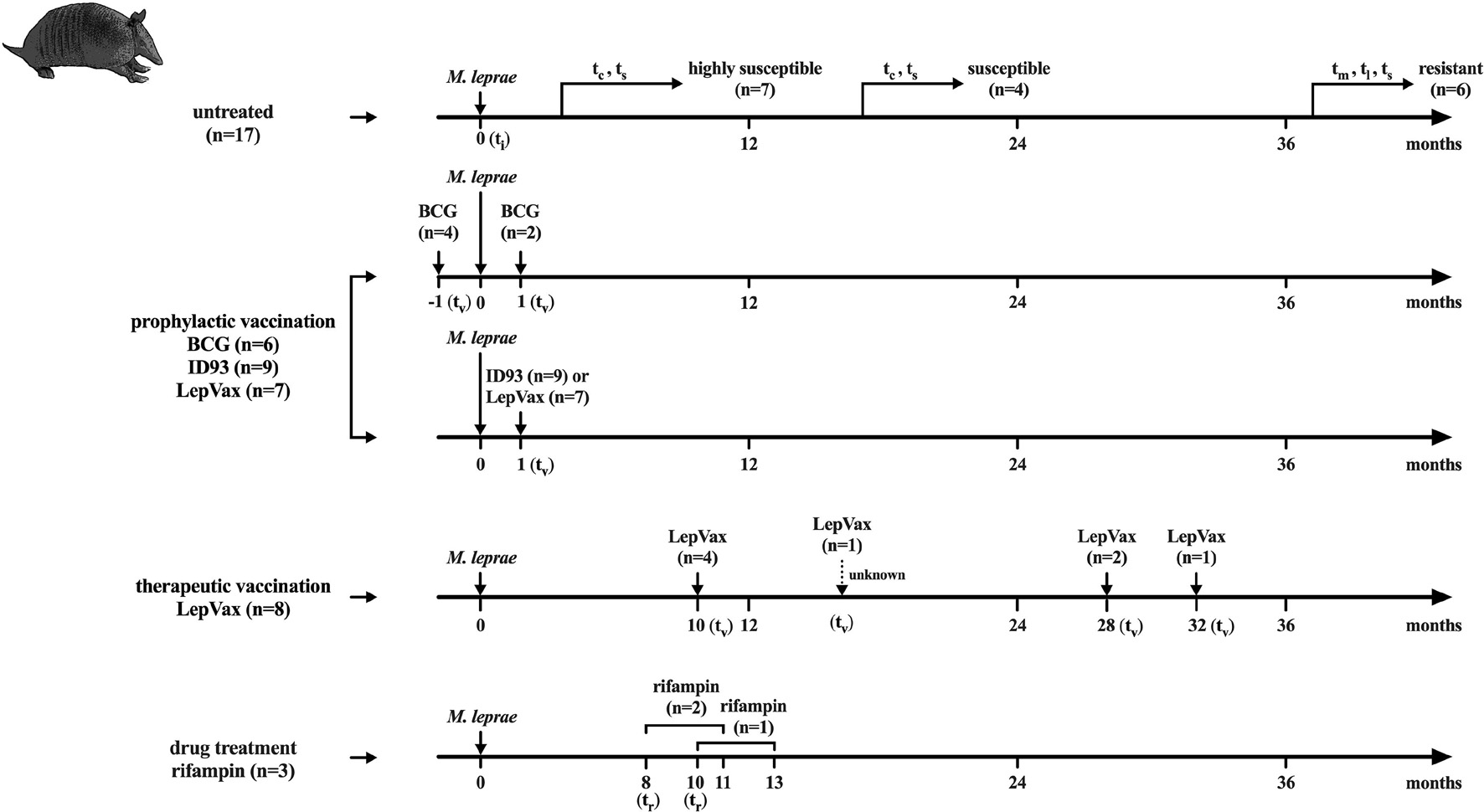
Figure 1. Flowchart of infection, treatment and sampling, Sample collected at several time points from 52 nine-banded armadillos (Dasypus novemcinctus) infected with 1 × 109 M. leprae bacilli from strains: NHDP 63 (n = 33), NHDP 98 (n = 7), or Brazil 4923 (n = 11). One animal was infected in the wild. M. leprae infected armadillos that were untreated and unvaccinated (n = 17) were divided into 3 groups according to M. leprae dissemination and disease progression after inoculation: highly susceptible (within 12 months; n = 7), susceptible (within 24 months; n = 4), and resistant (after more than 36 months; n = 6); Treated animals were divided into: Armadillos vaccinated with BCG 1 month pre- (n = 6) or post- (n = 2) infection; Armadillos prophylactically vaccinated with ID93 (n = 9) or LepVax (n = 7) 1 month post-infection; Armadillos therapeutically vaccinated LepVax (n = 8) 10–32 months post-infection; Armadillos treated for 3 months with rifampin 8 or 10 months post-infection (n = 3). For the animal that was infected in the wild and LepVax vaccinated, the inoculation time (ti) and interval between ti were unknown. ti, time of inoculation; tv, time of vaccination; tr, time of rifampin treatment; tc, time of seroconversion (anti-PGL-I IgM ELISA > 0.45 OD450–background; for the highly susceptible and susceptible group); tm, mid-stage disease; tl, late-stage disease; ts, time at sacrifice (see Supplementary Table 1).
Experimental M. leprae Inoculation
Three strains of M. leprae are cultivated in nude mouse footpads at the NHDP and serially passaged to maintain a high percent viability. M. leprae were harvested from the footpads, stored at 4°C, and used with 24 h. For inoculation, the armadillos were anesthetized and the skin at the injection site was cleaned with 70% ethanol. M. leprae were resuspended in saline at 1 × 109 bacilli per 1 ml and injected slowly into the saphenous vein (Truman and Krahenbuhl, 2001; Wallace et al., 2021).
Disease Progression
After experimental inoculation with M. leprae, a serum sample was collected every three months to screen for anti-PGL-I antibodies. When a positive ELISA assay was detected [corresponding to an optical density at 450 nm (OD450–background) of 0.45], the animals were followed for disease progression and dissemination by serology, hematology (hematocrit) and blood chemistry (lactate dehydrogenase; LDH). On average the animals were anti-PGL-I seropositive (anti-PGL-I IgM ELISA: OD450–background > 0.45) at 9 months after M. leprae inoculation. By 18–24 months post-inoculation most animals develop a severe infection. The armadillos were humanely sacrificed when generalized dissemination of bacilli was reached and the animals were seropositive, had a hematocrit lower than 20 and LDH levels were higher than 2,000.
Anti-PGL-I Antibodies ELISA
Levels of anti-M. leprae PGL-I IgM or -IgG in armadillos’ sera were measured by ELISAs described previously (Duthie et al., 2011; van Hooij et al., 2017, 2019; van Dijk et al., 2021); briefly, 96-well PolySorp NUNC plates (Thermo Fisher Scientific) were coated with 50 μl of the antigens ND-O-HSA (4 μg/ml, BEI Resources, NR-19347) in 100 mM Na2CO3/NaHCO3 buffer (pH 9.6) at 4° overnight. Coated plates were blocked with 200 μl PBS/1% BSA/0.05% Tween 80 per well for 1 h, 50 μl of 1:35 diluted sample was added and incubated for 2 h at room temperature. Then, 50 μl of anti-human IgG-HRP (1:4,000, Sigma, A0170) or IgM-HRP (1:8,000, Sigma, A6907) in 0.05%Tween 20/PBS was incubated for 2 h. In between each step the wells were washed 3 times with PBS/0.05% Tween 20. 50 μl of 3,3′,5,5′-Tetramethylbenzidine (TMB, Thermo Fisher Scientific, Rochester, NY) was added and the color reaction was stopped using H2SO4 after 10 min. Absorbance was determined at a wavelength of 450 nm. The optical density at 450 nm (OD450) of the samples was corrected with the background OD (0.1% BSA in coating buffer).
Up-Converting Reporter Particles Conjugate
LFAs were performed with luminescent up-converting reporter particles (UCP) as a sensitive background-free label for quantitative detection of targeted analytes (Corstjens et al., 2003, 2005; Zuiderwijk et al., 2003). UCP nanomaterials (200 nm NaYF4:Yb3+, Er3+ particles, functionalized with carboxyl groups) were obtained from Intelligent Material Solutions Inc. (Princeton, New Jersey, United States). UCP conjugates were prepared with goat anti-human IgM (I0759, Sigma-Aldrich, St. Louis, Missouri, United States) at a concentration of 50 μg antibody per mg UCP according to the method described previously (Bobosha et al., 2014; Corstjens et al., 2019).
Lateral Flow Strips
LF strips (4 mm width) for detection of anti-PGL-I IgM were produced as described previously (Corstjens et al., 2013; van Hooij et al., 2016, 2017, 2018, 2019). The test (T) line comprised 100 ng synthetic PGL-I (ND-O-HSA) and the flow control (FC) line comprised 100 ng rabbit anti-goat IgG (G4018, Sigma-Aldrich). UCP reporter conjugate (400 ng) was dried into the sample/conjugate-release pad using a buffer containing 5% (w/v) sucrose. LF strips were stored at ambient temperature in plastic containers with silica dry pad.
Anti-PGL-I IgM UCP-LFA
Serum samples were analyzed using 50 μl of a 1:50 (anti-PGL-I IgM) dilution in assay buffer (100 mM Tris pH 8, 270 mM NaCl, 1% (w/v) BSA, 1% (v/v) Triton X-100). Diluted serum samples (50 μl) were applied to LF strips. Upon completion of LF, strips were analyzed with a UCP dedicated benchtop reader (UPCON; Labrox, Finland). Results are displayed as the ratio value (R) of T and FC signals (peak area) based on relative fluorescence units (RFUs).
Statistical Analysis
Graphpad Prism version 9.0 for Windows (GraphPad Software, San Diego CA, United States) was used to perform Mann-Whitney U-tests, Wilcoxon matched-pairs tests, Kruskal-Wallis with Dunn’s correction for multiple testing, plot receiver operating characteristic (ROC) curves, calculate the area under the curve (AUC), and compute Spearman correlation coefficients. The optimal sensitivity and specificity were determined using Youden’s index (Fluss et al., 2005). The statistical significance level used was p < 0.05.
Results
Longitudinal Anti-PGL-I Antibody Levels in M. leprae Infected Armadillos
In this study, serum samples were analyzed that were previously collected in different experiments from M. leprae infected armadillo including. untreated, vaccinated, and drug-treated armadillos (Figure 1). To assess the kinetics of humoral anti-M. leprae responses in armadillos, we first assessed the levels of anti-PGL-I antibodies by ELISA in serum obtained from 17 armadillos that were experimentally infected with M. leprae [1 × 109 bacilli; NHDP 63 (n = 9), NHDP 98 (n = 4), or Brazil 4923 (n = 4)]. Armadillos were sampled at multiple time points and divided into three categories according to the time of dissemination of M. leprae infection (Pena et al., 2016; Supplementary Table 2). The (highly) susceptible group is the highly susceptible- combined with the susceptible group. At the time of sacrifice (ts), bacillary burden in the liver and spleen of (highly) susceptible armadillos was higher than in resistant animals. Highly susceptible armadillos also had slightly increased numbers of bacteria compared to susceptible animals (Supplementary Figure 1).
Before M. leprae infection (ti), no anti-PGL-I IgM or -IgG antibodies were detected in any of the armadillos (Figure 2). Upon infection with M. leprae, the anti-PGL-I IgM levels significantly increased in highly susceptible armadillos compared to pre-infection levels (ti vs. tc, p < 0.05; ti vs. ts, p < 0.05). In line with the classification of the armadillos, all susceptible animals became seropositive, but the antibody levels did not reach similarly high values (ti vs. tc, p = 0.25; ti vs. ts, p = 0.125). In resistant armadillos, on the other hand, after M. leprae infection, the animals remained seronegative during mid-stage disease (tm) while only one out of three became seropositive. At the late stage (tl) only two animals were tested which were seropositive (Figure 2A). In all animals, IgG levels were lower than IgM levels at all-time points. IgG after M. leprae infection was detected in six armadillos, three of which belonged to the resistant group. In the resistant group 50% (n = 3) showed detectable IgG, compared to 25% (n = 1) of the susceptible animals and 29% of the highly susceptible animals (Figure 2B).
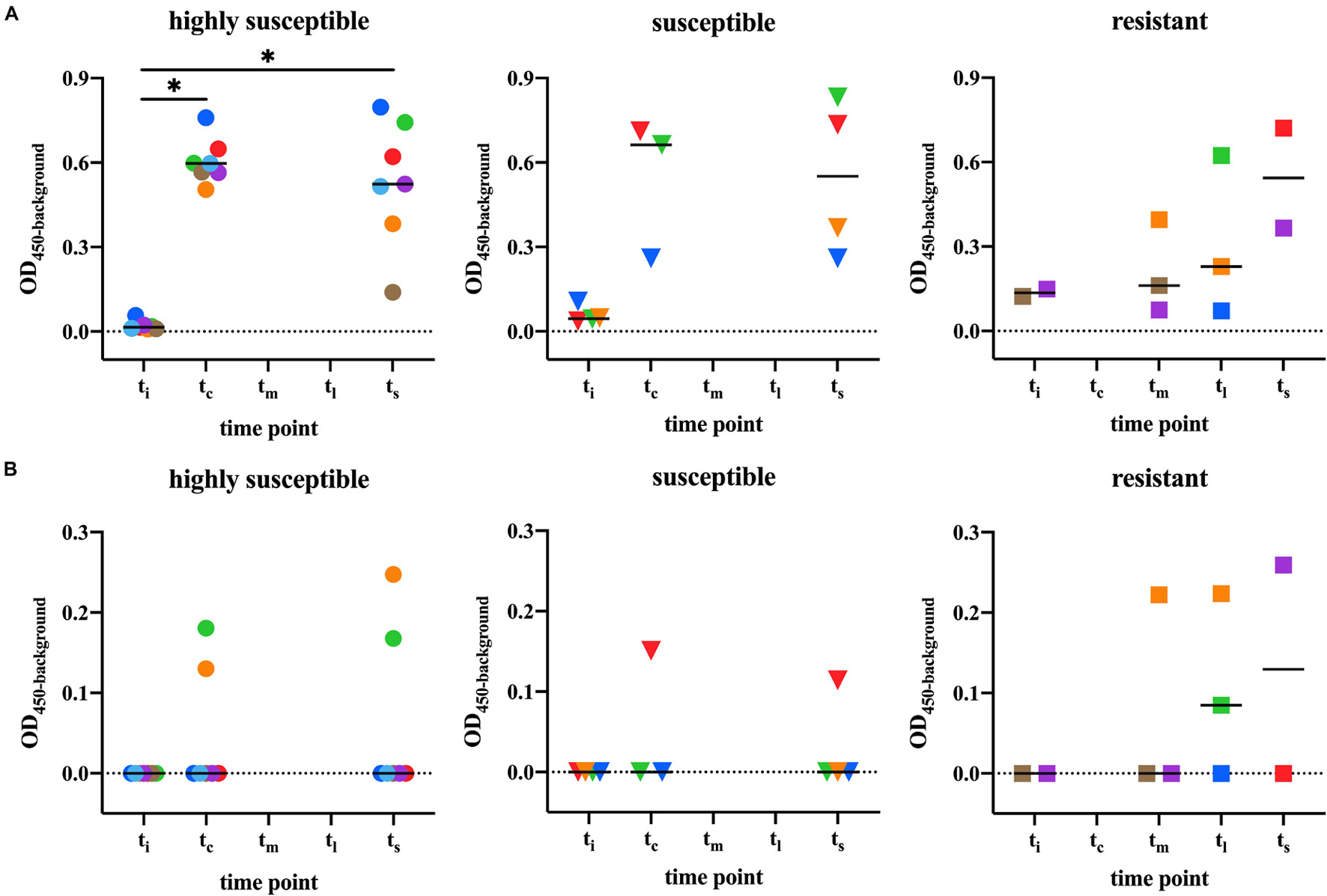
Figure 2. Anti-PGL-I antibody levels in M. leprae infected armadillos. Anti-PGL-I antibody levels were measured by ELISA in serum from M. leprae [1 × 109 bacilli; NHDP 63 (n = 9), NHDP 98 (n = 4), or Brazil 4923 (n = 4)] infected armadillos. Sample derived from each animal is represented by the same symbol. After infection, seven armadillos disseminated before 12 months (highly susceptible; dots), four disseminated and progressed to disease within 24 months (susceptible; triangles), and six armadillos did not disseminate even 36 months post-infection (resistant; squares). ELISA results of anti-PGL-I IgM (A) and anti-PGL-I IgG (B) are displayed as optical density at 450 nm corrected for background OD values (OD450–background; y-axis). The median values per group are indicated by horizontal lines. Differences between pre-infection (ti) vs. post-infection (tc/tm/tl/ts) were determined by Wilcoxon matched-pairs test. P-values: ∗p < 0.05. ti, time of inoculation; tc, time of seroconversion (for highly susceptible and susceptible animals); tm, mid-stage disease; tl, late-stage disease; ts, time at sacrifice.
The kinetics of anti-PGL-I IgM and IgG after M. leprae infection varied with susceptibility to M. leprae infection, as IgM levels were lower in the resistant- compared with highly susceptible and susceptible animals, while IgG levels were more frequently detected in resistant animals.
To analyze the correlation between anti-PGL-I antibodies and other blood parameters that indicate disease severity, we compared anti-PGL-I antibodies with white blood cell counts (WBC), hematocrit (HCT), lactate dehydrogenase (LDH), aspartate aminotransferase (AST), and alanine aminotransferase (ALT) from the same animals. However, there were no or only weak correlations between anti-PGL-I antibodies with the different blood values (R2 ≤ 0.17; Supplementary Figure 2), indicating that anti-PGL-I antibody levels provide improved diagnostic value for M. leprae infection.
Anti-PGL-I IgM UCP-LFA Correlates With ELISA
To assess whether anti-PGL-I specific antibodies could be detected in infected armadillos using UCP-LFA for detection of anti-PGL-I human IgM (van Hooij et al., 2017, 2020), serum samples (n = 41) from M. leprae infected armadillos (n = 17) were analyzed at multiple timepoints post-infection. As previously observed in humans (van Hooij et al., 2016, 2017), results of the UCP-LFA (Ratio values) correlated well (R2 = 0.887, p < 0.0001) with ELISA data (OD450–background) (Figure 3). As in the ELISA, anti-PGL-I IgM levels were not detected before M. leprae infection. After infection, the anti-PGL-I antibodies were detectable by UCP-LFA and significantly increased in time in the highly susceptible- and susceptible- armadillos (tc/ts), whereas no relevant difference in Ratio values were observed by UCP-LFA in resistant armadillos before the experimental endpoint (ti vs. tm/tl) (Figure 4).
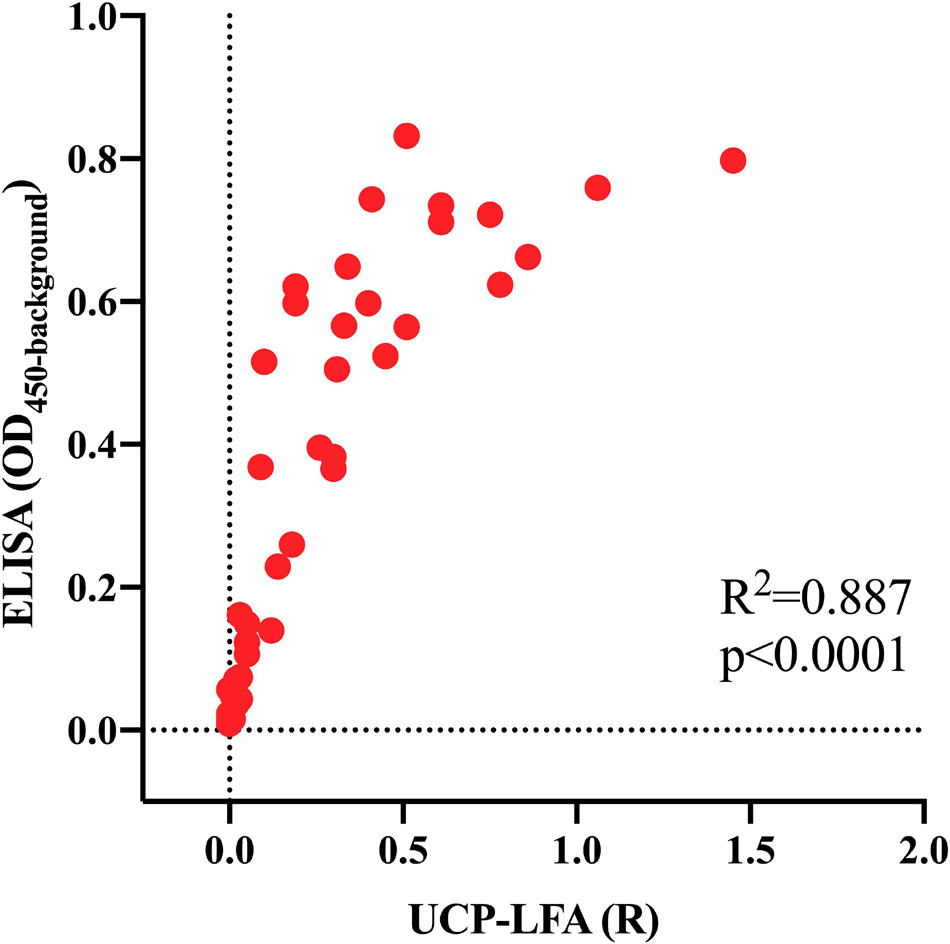
Figure 3. Correlation of anti-PGL-I IgM levels in M. leprae infected armadillos in UCP-LFA and ELISA. Serum samples (n = 41) of M. leprae [1 × 109 bacilli; NHDP 63 (n = 9), NHDP 98 (n = 4), or Brazil 4923 (n = 4)] infected armadillos at multiple time points, were assessed for anti-PGL-I IgM by UCP-LFA, and for anti-PGL-I IgM by ELISA. UCP-LFA results are displayed as the Ratio value (R) between Test (T) and Flow-Control (FC) signal (x-axis); ELISA results are displayed as optical density at 450 nm corrected by background (OD450–background; y-axis). R2 is the square of the Spearman correlation coefficient.
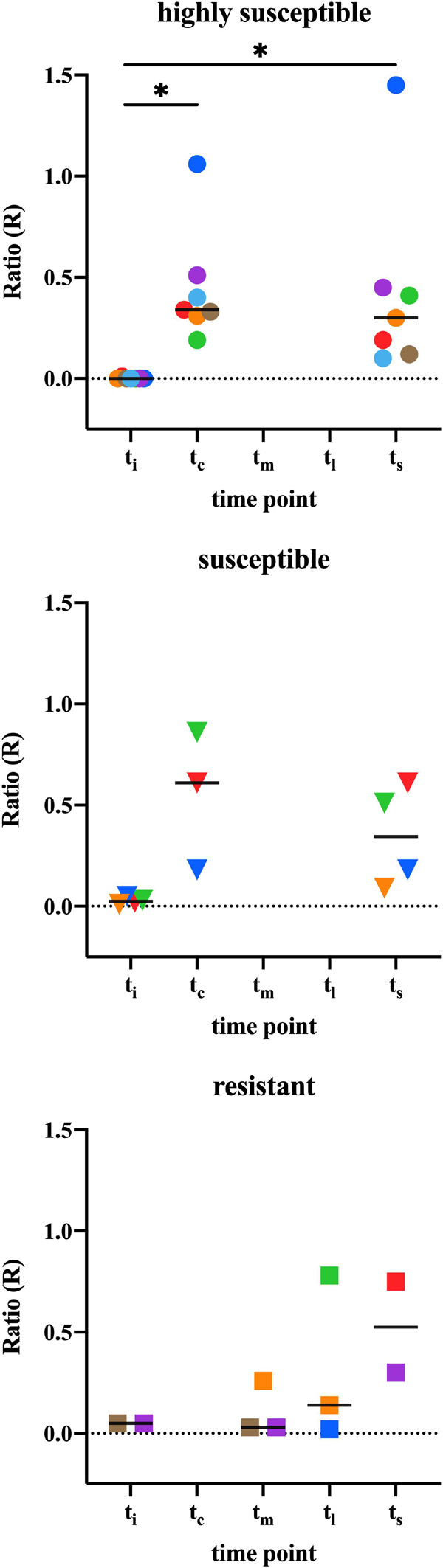
Figure 4. Anti-PGL-I IgM levels by UCP-LFA in M. leprae infected armadillos. Anti-PGL-I IgM levels were measured by UCP-LFA in serum from M. leprae [1 × 109 bacilli; NHDP 63 (n = 9), NHDP 98 (n = 4), or Brazil 4923 (n = 4)] infected armadillos divided into highly susceptible (n = 12; dots; upper panel), susceptible (n = 4; triangles; middle panel), and resistant (n = 6; squares, lower panel); sample derived from each animal is represented by the same symbol. UCP-LFA results are displayed as the Ratio value (R) between Test (T) and Flow-Control (FC) signal (y-axis). The cut-off value for discrimination between infected vs. uninfected animals is R > 0.07. The median values of each group are indicated by horizontal lines. Differences between pre-infection (ti) vs. post-infection (tc/tm/tl/ts) were determined by Wilcoxon matched-pairs test. P-values: ∗p < 0.05. ti, time of inoculation; tc, time of seroconversion (for highly susceptible and susceptible animals); tm, mid-stage disease; tl, late-stage disease; ts, time at sacrifice (<12 months for highly susceptible; 12–24 months for susceptible; >36 months for resistant).
Monitoring Active M. leprae Infection in Armadillos
To assess the potential of the UCP-LFA and ELISA to detect intraindividual levels in anti-PGL-I antibodies, we selected 12 out 17 animals with both pre (ti)- and post (ts)-infection sampling time points from the above mentioned armadillos for further analysis [highly susceptible (n = 7)-, susceptible (n = 4)-, resistant-armadillos (n = 1)] (Supplementary Table 2). The anti-PGL-I antibody levels were significantly increased post-infection and good discriminatory performance was obtained for anti-PGL-I IgM ELISA and -UCP-LFA with ROC-AUC values of 0.993 (p < 0.0001) and 1.000 (p < 0.0001) (Figure 5A and Table 1A).
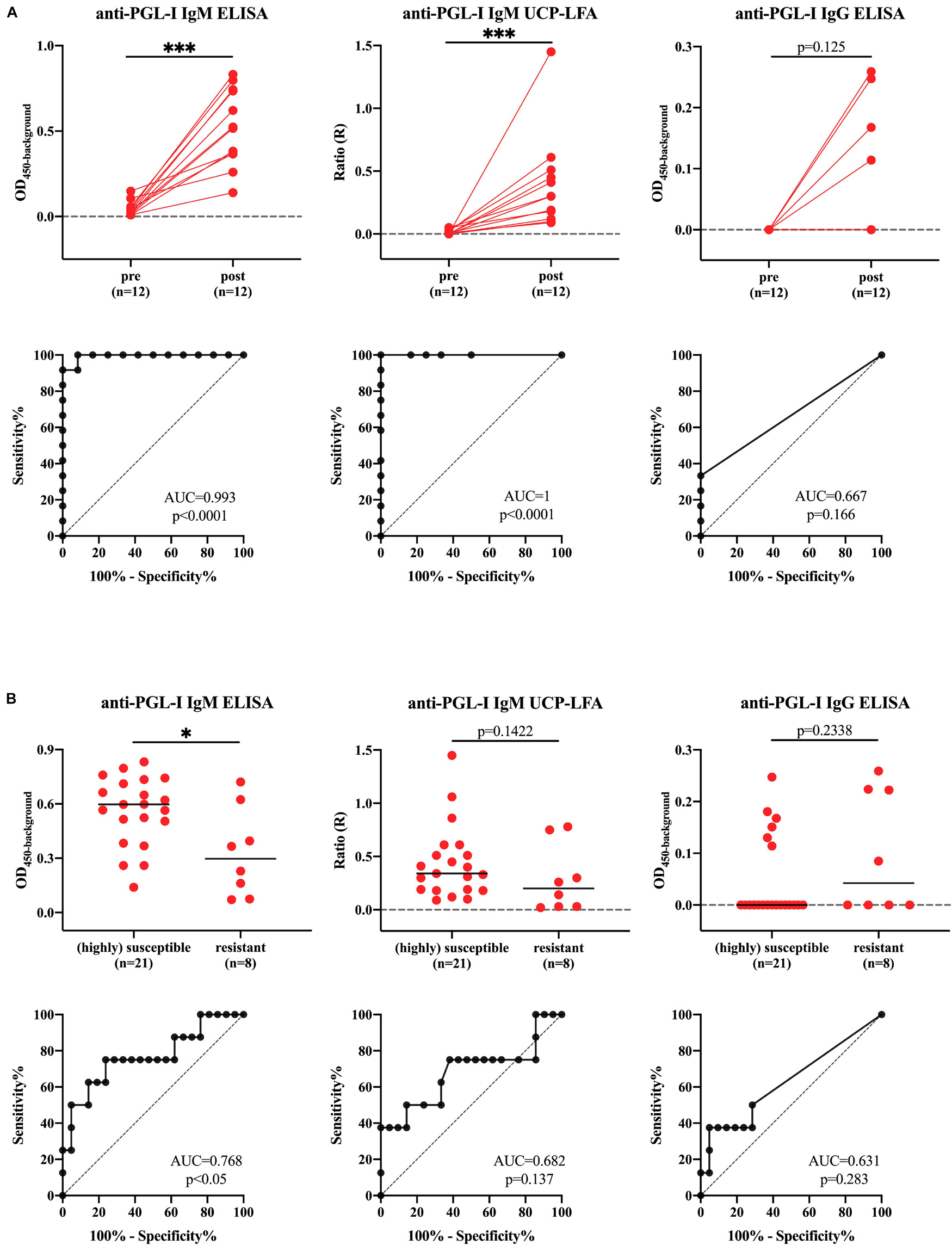
Figure 5. Differences in anti-PGL-I antibody levels between uninfected- and M. leprae infected highly susceptible, susceptible and resistant armadillos. Anti-PGL-I antibody levels were measured by UCP-LFA (IgM) and ELISA (IgM/IgG) in serum from armadillos infected with 1 × 109 M. leprae bacilli of the following strains: NHDP 63 (n = 9), NHDP 98 (n = 4), or Brazil 4923 (n = 4). Samples were analyzed pre-infection (ti) for all animals (n = 21) and post-infection (tc/tm/tl/ts) for highly susceptible (n = 7), susceptible (n = 4) and resistant (n = 6) armadillos UCP-LFA results are displayed as the Ratio value (R; y-axis) between Test (T) and Flow-Control (FC) signal; ELISA results are displayed as optical density at 450 nm corrected by the background for each sample (OD450–background). (A) For animals for which both samples were available, significant differences between levels in pre (ti)- vs. post (ts)-infection sera were determined by Wilcoxon matched-paired tests; (B) Antibody levels in all sera after inoculation were determined by Mann-Whitney U-tests; P-values: ∗p < 0.05, ∗∗∗p < 0.001. ti: time of inoculation; tc: time of seroconversion (for highly susceptible and susceptible animals); tm, mid-stage disease; tl, late-stage disease; ts, time at sacrifice.
In order to determine whether anti-PGL-I antibody levels can distinguish differential susceptibility to dissemination, we further analyzed all 17 armadillos, including (highly) susceptible (n = 11) and resistant (n = 6) armadillo samples at all available time points (tc/tm/tl/ts) after inoculation (21 samples of (highly) susceptible armadillos, 8 samples of resistant armadillos, respectively) (Supplementary Table 2). The anti-PGL-I IgM antibody levels detected by ELISA and UCP-LFA were more increased after infection in (highly) susceptible animals compared to resistant animals (p < 0.05, p = 0.1422, respectively). Based on the anti-PGL-I levels, the (highly) susceptible- could be discriminated from the resistant armadillos (AUC = 0.7679, p < 0.05) (Figure 5B and Table 1B).
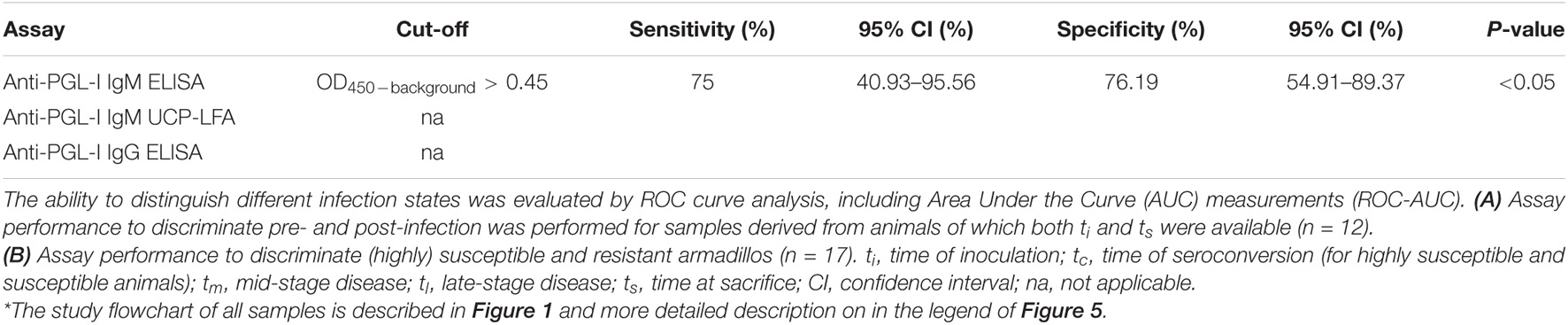
Table 1B. Assay performance to discriminate (highly) susceptible and resistant armadillos (n = 17)*.
Since anti-PGL-I IgG levels were not detectable in most samples (20 out of 29) from infected armadillos, discrimination based on anti-PGL-I IgG levels was not possible (Table 1 and Figure 5).
Anti-PGL-I Antibodies in Vaccinated and Rifampin Treated Armadillos
To determine whether the levels of anti-PGL-I antibody measured by UCP-LFA could detect changes reflecting efficient vaccination or drug treatment, we analyzed samples from 24 armadillos that were prophylactically vaccinated with ID93, LepVax, or BCG 1 month pre- (n = 2 for BCG) or post- (n = 9, 7, 6 for ID93, LepVax, BCG, respectively) experimental M. leprae infection. We also added a therapeutically vaccinated group, which included eight armadillos that were vaccinated with LepVax 10–32 months post-infection. In addition, three armadillos were included that were treated for 3 months with rifampin 8 or 10 months post-infection (Supplementary Table 2). Armadillos vaccinated with ID93 or LepVax had less bacilli in the liver and spleen compared to highly susceptible- and susceptible animals, but still a higher bacillary load than resistant animals at the endpoint (Supplementary Figure 1). Anti-PGL-I antibody levels in armadillos prophylactically vaccinated with LepVax (n = 1) or ID93 (n = 5), were similar to those in untreated, infected animals at the time of sacrifice. However, anti-PGL-I antibody levels in the two vaccinated groups (median ts: 1,137 and 1,342 days, respectively) were comparable to those in resistant armadillos (median ts: 1,215 days) as dissemination took considerably longer than in (highly) susceptible armadillos (ts median = 292 days). This indicates that both ID93 and LepVax prophylactic vaccination can delay the increase of anti-PGL-I antibody levels and thus bacterial load/disease progression as observed in the resistant animals (Figures 6A,B, 7 and Supplementary Table 2). At the timepoint of sacrifice, anti-PGL-I antibody levels of armadillos that were therapeutically vaccinated with LepVax (n = 5), were similar to those of the prophylactically vaccinated animals, which was also reflected by the prolonged time to severe disease (ts median = 981 days) (Figures 6C, 7 and Supplementary Table 2).
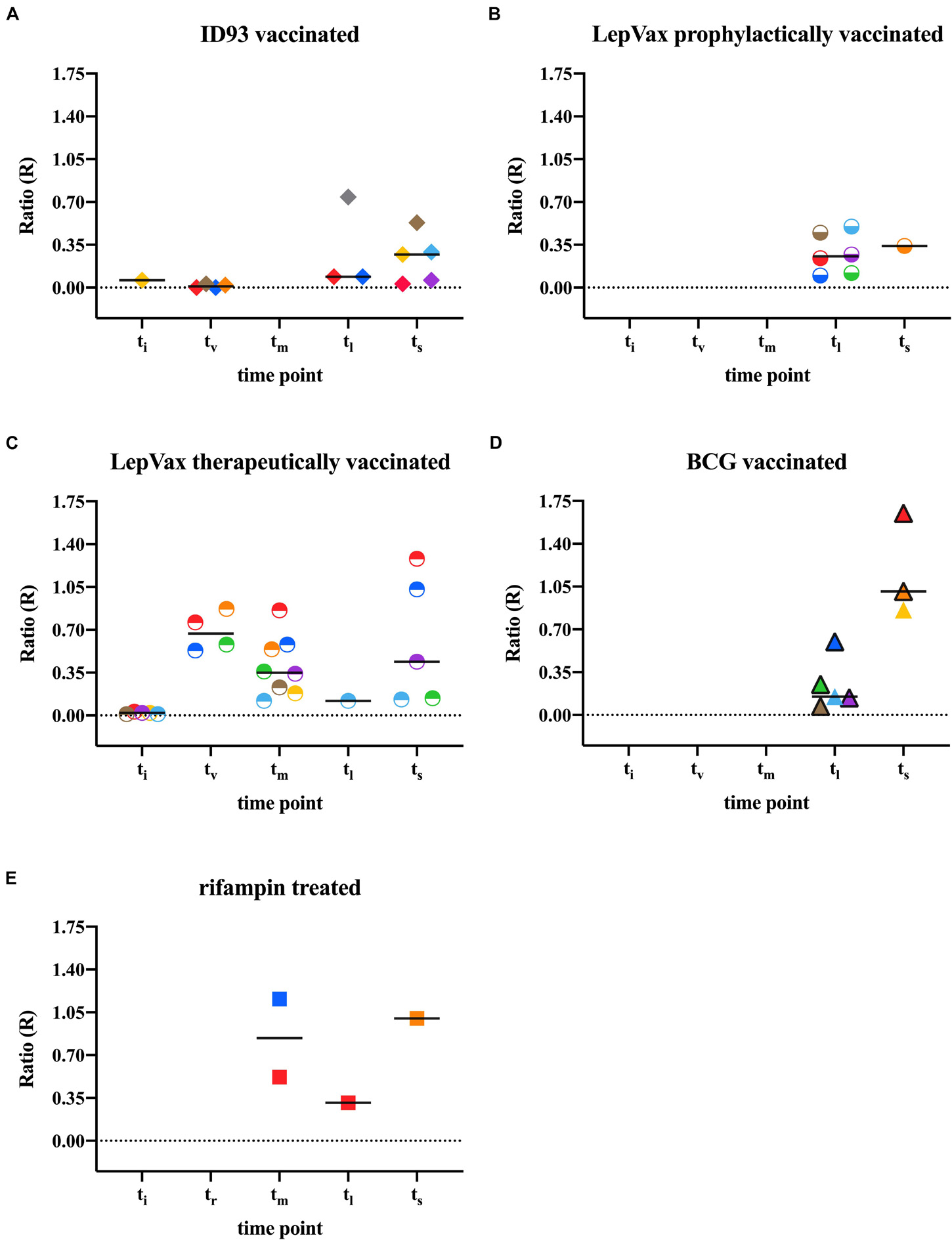
Figure 6. Anti-PGL-I IgM levels by UCP-LFA in M. leprae infected treated/vaccinated armadillos. Anti-PGL-I IgM levels were measured by UCP-LFA in serum from M. leprae [1 × 109 bacilli; NHDP 63 (n = 24), NHDP 98 (n = 3), or Brazil 4923 (n = 7)], and one animal was infected in the wild) infected armadillos (n = 35). 16 armadillos were prophylactically vaccinated with ID93 (n = 9; A) or LepVax (n = 7, B) 1 month post-infection; eight armadillos were therapeutically vaccinated with LepVax (C) 10–32 months post-infection; Eight armadillos were vaccinated with BCG (D) 1 month pre- (n = 6, black frame) or post- (n = 2, colored triangle) infection; Rifampin was provided for 3 months to armadillos (n = 3) 8 or 10 months post-infection (E). Sample derived from each animal is represented by the same symbol. UCP-LFA results are displayed as the Ratio value (R; y-axis) between Test (T) and Flow-Control (FC) signal. The median values of each group are indicated by horizontal lines. Differences in antibody levels between pre-infection (ti) vs. post-infection (tv/tr/tm/tl/ts) were determined by Mann-Whitney U-tests. ti, time of inoculation; tv, time of vaccination; tr, time of rifampin treatment; tm, mid-stage disease; tl, late-stage disease; ts, time at sacrifice.
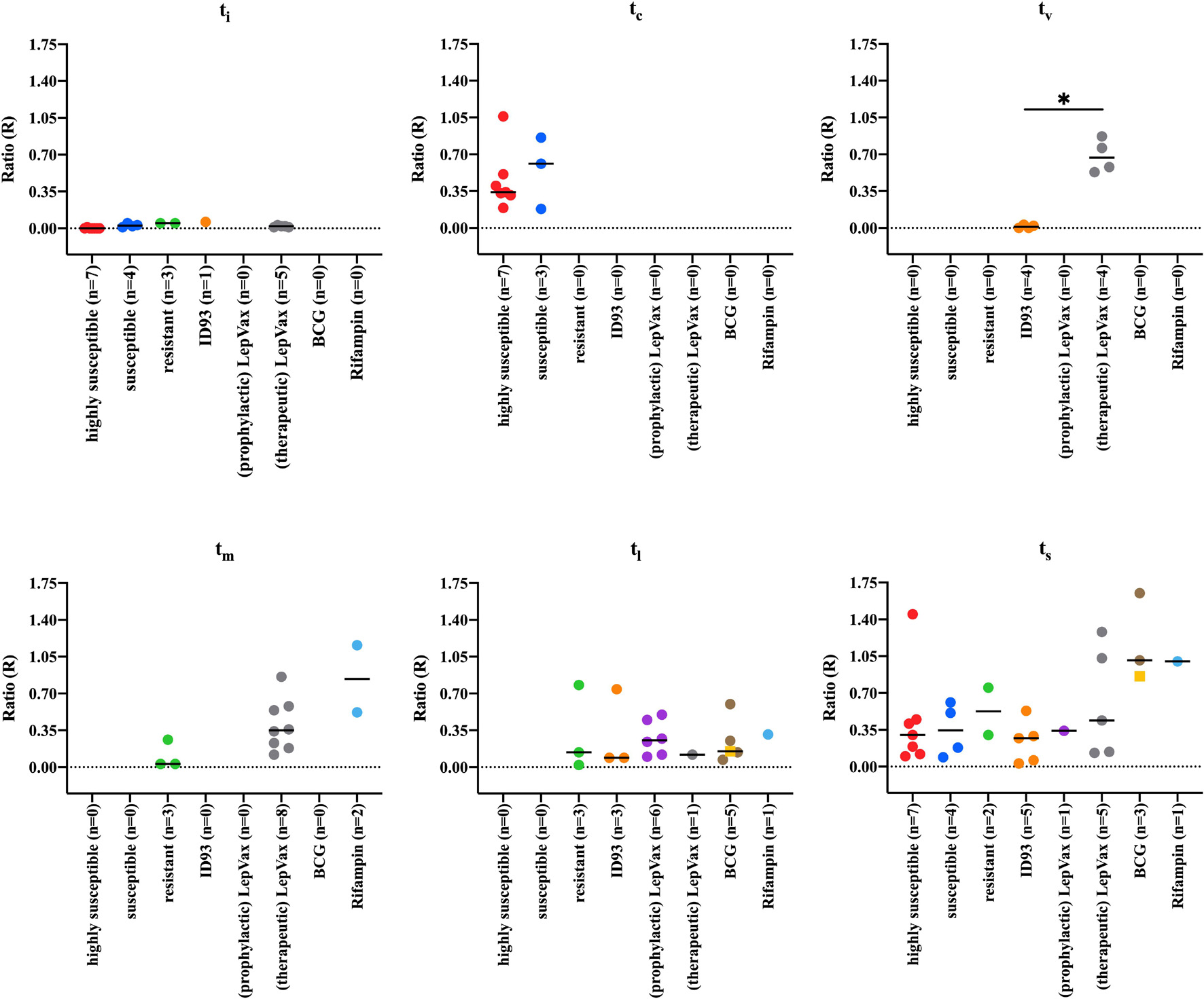
Figure 7. Anti-PGL-I IgM levels by UCP-LFA in M. leprae infected armadillos after interventions. Anti-PGL-I IgM levels were measured by UCP-LFA in serum from M. leprae [1 × 109 bacilli; NHDP 63 (n = 33), NHDP 98 (n = 7), or Brazil 4923 (n = 11)], and one animal was infected in the wild) infected armadillos (n = 17) divided into highly susceptible (n = 7, red dots), susceptible (n = 4, blue dots), and resistant (n = 6, green dots). 24 armadillos were prophylactically vaccinated with ID93 (n = 9, orange) or LepVax (n = 7, purple dots) 1 month post-infection; eight armadillos were therapeutically vaccinated LepVax (gray dots) 10–32 months post-infection; Eight armadillos were vaccinated with BCG 1 month pre- (n = 6, brown dots) or post- (n = 2, yellow squares) infection. Rifampin was provided for 3 months to armadillos (n = 3, teal dots) 8 or 10 months post-infection. UCP-LFA results are displayed as the Ratio value (R) between Test (T) and Flow-Control (FC) signal (y-axis). The median values of each group are indicated by horizontal lines. Differences between groups were determined by Kruskal-Wallis test with Dunn’s correction for multiple testing. P-values: ∗p < 0.05. ti, time of inoculation; tc, time of seroconversion (for highly susceptible and susceptible animals); tv, time of vaccination; tm, mid-stage disease; tl, late-stage disease; ts, time at sacrifice.
After BCG vaccination, armadillos had slightly lower bacillary levels than highly susceptible animals, but higher than ID93 and LepVax vaccinated animals (Supplementary Figure 1). The anti-PGL-I antibody levels detected after BCG (n = 5) remained at similarly low levels as after ID93 (n = 3) vaccination at tl. Furthermore, the antibody levels observed at ts after BCG vaccination (n = 3), were higher than after ID93 or LepVax vaccination (Figures 6D, 7), and a shorter time to sacrifice due to M. leprae dissemination was observed (ts median = 469 days, Supplementary Table 2). This may indicate that defined subunit vaccines such as LepVax and ID93 are more effective than BCG in reducing M. leprae load.
After rifampin treatment of M. leprae infected armadillos (n = 2), anti-PGL-I antibody levels were increased compared to those in resistant armadillos (n = 3) at tm, and declined at tl to levels similar to what was observed for resistant armadillos (n = 1), reflecting the therapeutic effect of rifampin on M. leprae infection. At time of sacrifice (ts), rifampin treated armadillos (n = 1) showed higher anti-PGL-I antibody levels than untreated susceptible armadillos, although the bacillary load was comparable (Figures 6E, 7 and Supplementary Figure 1). Rifampin treatment for 3 months likely did not completely sterilize M. leprae infected armadillos, allowing residual bacteria to continue replicating, but rifampicin treatment still prolonged the time to full dissemination of infected animals (ts median = 674 days). Thus, anti-PGL-I IgM UCP-LFA can monitor the efficacy of vaccination and rifampin treatment in M. leprae infected armadillo.
Discussion
IgM antibodies against M. leprae-PGL-I are specific for infection with mycobacteria causing leprosy (Hunter and Brennan, 1981; van Dijk et al., 2021) and anti-PGL-I IgM levels have been amply shown to be quantitively associated with bacterial load in leprosy patients (Zenha et al., 2009; van Hooij et al., 2017; Tió-Coma et al., 2020). However, the presence of these antibodies does not reveal sufficient information about the timing of infection in humans. The wide spectrum of immunology and histopathology in armadillos is similar to human leprosy, which renders this animal model very useful for research on diagnostic markers for active M. leprae infection and leprosy disease (Job, 1991; Sharma et al., 2013; Truman et al., 2014). Studies on experimentally infected armadilllos have previously shown that lower ratios between IgM/IgG anti-PGL-I antibodies indicate lower susceptibility for M. leprae dissemination in armadillos (Vadiee et al., 1988). In the current study the serological monitoring of anti-PGL-I antibodies in serum of experimentally M. leprae infected armadillos with or without vaccination allowed the examination of the kinetics of IgM and IgG anti-PGL-I antibodies early after inoculation.
In humans, detection of M. leprae infection before clinical symptoms have occurred is vital to reduce transmission but also to reduce leprosy-associated disabilities. Scarcity of laboratory infrastructure in, often resource limited, leprosy endemic areas, demand low complexity tests that cannot only detect M. leprae infection at early stages, but also identify which levels are associated with active infection, and determine leprosy phenotypes at point-of-care and genuine near-patient level. Better insight into the kinetics of antibody formation, particularly directly after M. leprae infection, is required for full evaluation of newly developed diagnostic tools (rapid tests) and can be provided by the armadillo leprosy model.
Previously we have shown that a rapid LFA utilizing the luminescent UCP label for detection and quantitation of human anti-PGL-I IgM (van Hooij et al., 2016, 2017, 2018, 2019, 2021), also allows detection of antibodies against PGL-I in M. leprae infected red squirrels (Truman et al., 1986; Gormus et al., 1995; da Silva et al., 2018; Schilling et al., 2019, 2021; da Silva Ferreira et al., 2020). The protected status of the red squirrels in the United Kingdom, however, precluded any experiments on kinetics directly after M. leprae infection.
In this study, we have successfully examined the use of the anti-PGL-I UCP-LFA in a more assessable animal model by testing sera of nine-banded armadillos and measured longitudinal changes in anti-PGL-I antibody levels in experimentally infected armadillos with different susceptibility to M. leprae.
Our data show that the anti-PGL-I UCP-LFA can be used to detect M. leprae infection and even monitor the efficacy of vaccination and rifampin-treatment in susceptible armadillos. Thus, as demonstrated in the armadillo model for leprosy, the UCP-LFA provides a low-complexity tool for detection of infection and is a promising monitoring tool to evaluate the efficacy of vaccines and drugs.
Anti-PGL-I UCP-LFA data were only weakly correlated with other blood indicators that were used for bacterial load and severity evaluation of leprosy in the armadillos, such as serum lactate dehydrogenase (LDH), aspartate aminotransferase (AST), and alanine aminotransferase (ALT) (Rojas-Espinosa et al., 1985). This emphasizes the improved diagnostic value of anti-PGL-I IgM UCP-LFA as a biomarker for active M. leprae infection, especially since anti-PGL-I IgM could be detected in all infected animals.
In humans anti-PGL-I IgM has been reported on more often in leprosy research than anti-PGL-I IgG or -IgA (Pierneef et al., 2021). Monitoring the dynamic differences of anti-PGL-I IgM and IgG antibodies in sera from experimentally M. leprae infected armadillo provides insight into the formation and kinetics of these antibodies in humans at the early stage of infection. The current study showed that anti-PGL-I IgM as well as IgG antibodies increased after M. leprae infection. Both antibody types were associated with susceptibility to infection, which was most apparent for the IgM isotype which increased rapidly in highly susceptible- and susceptible animals. The UCP-LFA could detect early phases of M. leprae infection (after 140 days) with 100% sensitivity and specificity comparing pre- to post-infection. However, since only armadillos showing a negative lepromin response (indicating susceptibility to M. leprae) were experimentally infected with M. leprae in this study, we were not able to assess whether the UCP-LFA can be used to discriminate susceptible from resistant armadillos as is the case for MB and PB leprosy in humans (van Hooij et al., 2017).
Anti-PGL-I IgG was only detected in a limited number of samples and also more frequently in resistant animals. This may indicate that the anti-PGL-I IgM and IgG play different roles in anti-M. leprae humoral immunity and vary in dynamic changes during M. leprae infection. The IgM response, however, was long-lasting in all animals in which IgM antibodies were observed after infection and did not wane over time (maximal 1,325 days in resistant animals). This is similar to our observation in squirrels, as IgM levels specifically increased in red squirrels developing leprosy symptoms (Schilling et al., 2021). In both animal models anti-PGL-I specific IgM was still present at higher levels years after infection, indicating that these antibodies not only indicate recent M. leprae infection. These data corroborate the finding that in human leprosy the presence of IgM antibodies does not only indicate recent infection.
Determining the ratio of the two types of antibodies could advance leprosy diagnosis in humans, particularly for active infection and disease classification.
Since armadillos infected with M. leprae are a potential environmental reservoir for the zoonotic transmission of leprosy (Truman et al., 2011; Sharma et al., 2015; da Silva et al., 2018; da Silva Ferreira et al., 2020), detecting M. leprae infection in armadillos is essential to eliminate the spread of leprosy. The UCP-LFA is a minimally invasive, rapid, low-cost, and user-friendly test. This study has demonstrated that UCP-LFA can effectively detect anti-PGL-I antibodies in armadillos which correlated well with ELISA for IgM. The anti-PGL-I IgM by UCP-LFA could be applied as a low complexity screening tool to detect environmental reservoirs of M. leprae. Since in this study only armadillos showing a lepromatous leprosy (LL) type response to lepromin were included, representing the majority of the M. leprae infected armadillos (Job et al., 1983; Job and Truman, 2000), follow-up studies should also include armadillos with tuberculoid leprosy (TT) responses to lepromin.
In summary, this study shows that the UCP-LFA allows quantitative measurements of anti-PGL-I levels in serum of nine-banded armadillos directly after experimental M. leprae infection, allowing discrimination between susceptible and resistant animals. In view of the spectral pathology of armadillos, this animal model provides useful insight into antibody kinetics in early infection and spectral forms of human leprosy. The armadillo leprosy model indicates that the UCP-LFA detecting anti-PGL-I IgM can be used for detection of infection in humans at early stages as well as monitoring changes in bacillary load. Thus, this UCP-LFA provides a rapid and low-cost tool to screen for infection and evaluate the effect of prophylactic and therapeutic treatments (Richardus et al., 2019; Richardus and Geluk, 2020).
Data Availability Statement
The original contributions presented in the study are included in the article/Supplementary Material, further inquiries can be directed to the corresponding author/s.
Ethics Statement
The animal study was reviewed and approved by the NHDP Animal Care and Use Committee.
Author Contributions
AG: conceptualization. AG, AH, MP, and ZZ: data curation. RS and RW: animal experiments. DJ and LP: production and QC UCP-LFA. AG, AH, LP, and ZZ: formal analysis. AG and ZZ: writing—original draft. AG, AH, LA, MP, PC, RT, and ZZ: writing—review and editing. All authors agreed with manuscript results and conclusion.
Funding
This study was supported with grants from the Q.M. Gastmann-Wichers Foundation (to AG). ZZ was supported with grant from the China Scholarship Council. Armadillo work was funded by the NIH/NIAID through an Interagency Agreement (No. AAI21005-000-00000) with HRSA/HSB/NHDP.
Conflict of Interest
The authors declare that the research was conducted in the absence of any commercial or financial relationships that could be construed as a potential conflict of interest.
The reviewer WL declared a past collaboration with one of the author MP to the handling editor.
Publisher’s Note
All claims expressed in this article are solely those of the authors and do not necessarily represent those of their affiliated organizations, or those of the publisher, the editors and the reviewers. Any product that may be evaluated in this article, or claim that may be made by its manufacturer, is not guaranteed or endorsed by the publisher.
Supplementary Material
The Supplementary Material for this article can be found online at: https://www.frontiersin.org/articles/10.3389/fmicb.2021.763289/full#supplementary-material
References
Aarão, T. L., de Sousa, J. R., Botelho, B. S., Fuzii, H. T., and Quaresma, J. A. (2016). Correlation between nerve growth factor and tissue expression of IL-17 in leprosy. Microb. Pathog. 90, 64–68. doi: 10.1016/j.micpath.2015.11.019
Adams, L. B., Pena, M. T., Sharma, R., Hagge, D. A., Schurr, E., and Truman, R. W. (2012). Insights from animal models on the immunogenetics of leprosy: a review. Mem. Inst. Oswaldo Cruz. 107(Suppl. 1), 197–208. doi: 10.1590/s0074-02762012000900028
Balamayooran, G., Pena, M., Sharma, R., and Truman, R. W. (2015). The armadillo as an animal model and reservoir host for Mycobacterium leprae. Clin. Dermatol. 33, 108–115. doi: 10.1016/j.clindermatol.2014.07.001
Bertholet, S., Ireton, G. C., Kahn, M., Guderian, J., Mohamath, R., Stride, N., et al. (2008). Identification of human T cell antigens for the development of vaccines against Mycobacterium tuberculosis. J. Immunol. 181, 7948–7957. doi: 10.4049/jimmunol.181.11.7948
Bobosha, K., Fat, E., van den Eeden, S. J. F., Bekele, Y., van der Ploeg-van, Schip, J. J., et al. (2014). Field-evaluation of a new lateral flow assay for detection of cellular and humoral immunity against Mycobacterium leprae. PLoS Negl. Trop. Dis. 8:5. doi: 10.1371/journal.pntd.0002845
Corstjens, P., van Hooij, A., Tjon Kon Fat, E. M., Alam, K., Vrolijk, L. B., Dlamini, S., et al. (2019). Fingerstick test quantifying humoral and cellular biomarkers indicative for M. leprae infection. Clin. Biochem. 2019:7. doi: 10.1016/j.clinbiochem.2019.01.007
Corstjens, P., Zuiderwijk, M., Nilsson, M., Feindt, H., Niedbala, R. S., and Tanke, H. J. (2003). Lateral-flow and up-converting phosphor reporters to detect single-stranded nucleic acids in a sandwich-hybridization assay. Anal. Biochem. 312, 191–200. doi: 10.1016/s0003-2697(02)00505-5
Corstjens, P. L., Fidder, H. H., Wiesmeijer, K. C., de Dood, C. J., Rispens, T., Wolbink, G. J., et al. (2013). A rapid assay for on-site monitoring of infliximab trough levels: a feasibility study. Anal. Bioanal. Chem. 405, 7367–7375. doi: 10.1007/s00216-013-7154-0
Corstjens, P. L., Li, S., Zuiderwijk, M., Kardos, K., Abrams, W. R., Niedbala, R. S., et al. (2005). Infrared up-converting phosphors for bioassays. IEE Proc. Nanobiotechnol. 152, 64–72. doi: 10.1049/ip-nbt:20045014
da Silva, M. B., Portela, J. M., Li, W., Jackson, M., Gonzalez-Juarrero, M., Hidalgo, A. S., et al. (2018). Evidence of zoonotic leprosy in Para, Brazilian Amazon, and risks associated with human contact or consumption of armadillos. PLoS Negl. Trop. Dis. 12:e0006532. doi: 10.1371/journal.pntd.0006532
da Silva Ferreira, J., de Carvalho, F. M., Vidal Pessolani, M. C., de Paula Antunes, J. M. A., de Medeiros Oliveira, I. V. P., et al. (2020). Serological and molecular detection of infection with Mycobacterium leprae in Brazilian six banded armadillos (Euphractus sexcinctus). Comp. Immunol. Microbiol. Infect. Dis. 68:101397. doi: 10.1016/j.cimid.2019.101397
Day, T. A., Penn-Nicholson, A., Luabeya, A. K. K., Fiore-Gartland, A., Du Plessis, N., Loxton, A. G., et al. (2021). Safety and immunogenicity of the adjunct therapeutic vaccine ID93+GLA-SE in adults who have completed treatment for tuberculosis: a randomised, double-blind, placebo-controlled, phase 2a trial. Lancet Respir. Med. 9, 373–386. doi: 10.1016/s2213-2600(20)30319-2
Duthie, M. S., Coler, R. N., Laurance, J. D., Sampaio, L. H., Oliveira, R. M., Sousa, A. L., et al. (2014). Protection against Mycobacterium leprae infection by the ID83/GLA-SE and ID93/GLA-SE vaccines developed for tuberculosis. Infect Immun. 82, 3979–3985. doi: 10.1128/iai.02145-14
Duthie, M. S., Pena, M. T., Ebenezer, G. J., Gillis, T. P., Sharma, R., Cunningham, K., et al. (2018). LepVax, a defined subunit vaccine that provides effective pre-exposure and post-exposure prophylaxis of M. leprae infection. NPJ Vaccines 3:12. doi: 10.1038/s41541-018-0050-z
Duthie, M. S., Pena, M. T., Khandhar, A. P., Picone, A., Mac, M. Z., Truman, R. W., et al. (2020b). Development of LepReact, a defined skin test for paucibacillary leprosy and low-level M. leprae infection. Appl. Microbiol. Biotechnol. 104, 3971–3979. doi: 10.1007/s00253-020-10505-2
Duthie, M. S., Frevol, A., Day, T., Coler, R. N., Vergara, J., Rolf, T., et al. (2020a). A phase 1 antigen dose escalation trial to evaluate safety, tolerability and immunogenicity of the leprosy vaccine candidate LepVax (LEP-F1 + GLA-SE) in healthy adults. Vaccine 38, 1700–1707. doi: 10.1016/j.vaccine.2019.12.050
Duthie, M. S., Truman, R. W., Goto, W., O’Donnell, J., Hay, M. N., Spencer, J. S., et al. (2011). Insight toward early diagnosis of leprosy through analysis of the developing antibody responses of Mycobacterium leprae-infected armadillos. Clin. Vacc. Immunol. 18, 254–259. doi: 10.1128/cvi.00420-10
Eichelmann, K., González González, S. E., Salas-Alanis, J. C., and Ocampo-Candiani, J. (2013). Leprosy. An update: definition, pathogenesis, classification, diagnosis, and treatment. Actas Dermosifiliogr. 104, 554–563. doi: 10.1016/j.adengl.2012.03.028
Fluss, R., Faraggi, D., and Reiser, B. (2005). Estimation of the Youden Index and its associated cutoff point. Biom. J. 47, 458–472. doi: 10.1002/bimj.200410135
Geluk, A., Duthie, M. S., and Spencer, J. S. (2011). Postgenomic Mycobacterium leprae antigens for cellular and serological diagnosis of M. leprae exposure, infection and leprosy disease. Lepr. Rev. 82, 402–421.
Gormus, B. J., Baskin, G. B., Xu, K., Ratterree, M. S., Martin, L. N., Mack, P. A., et al. (2000). Antileprosy protective vaccination of sooty mangabey monkeys with BCG or BCG plus heat-killed Mycobacterium leprae: immunologic observations. Int. J. Lepr. Other. Mycobact. Dis. 68, 434–443.
Gormus, B. J., Xu, K., Cho, S. N., Baskin, G. B., Bohm, R. P., Martin, L. N., et al. (1995). Experimental leprosy in monkeys. II. Longitudinal serological observations in sooty mangabey monkeys. Lepr. Rev. 66, 105–125. doi: 10.5935/0305-7518.19950013
Hazbón, M. H., Rigouts, L., Schito, M., Ezewudo, M., Kudo, T., Itoh, T., et al. (2018). Mycobacterial biomaterials and resources for researchers. Pathog. Dis. 76:4. doi: 10.1093/femspd/fty042
Hungria, E. M., Bührer-Sékula, S., Oliveira, R. M., Aderaldo, L. C., Pontes, M. A. A., Cruz, R., et al. (2018). Mycobacterium leprae-specific antibodies in multibacillary leprosy patients decrease during and after treatment with either the regular 12 doses multidrug therapy (MDT) or the uniform 6 doses MDT. Front. Immunol. 9:915. doi: 10.3389/fimmu.2018.00915
Hunter, S. W., and Brennan, P. J. (1981). A novel phenolic glycolipid from Mycobacterium leprae possibly involved in immunogenicity and pathogenicity. J. Bacteriol. 147, 728–735. doi: 10.1128/jb.147.3.728-735.1981
Job, C. K. (1991). Nine-banded armadillo (Dasypus novemcinctus) as an animal model for leprosy. Indian J. Lepr. 63, 356–361.
Job, C. K., Kirchheimer, W. F., and Sanchez, R. M. (1983). Variable lepromin response to Mycobacterium leprae in resistant armadillos. Int. J. Lepr. Mycobact. Dis. 51, 347–353.
Job, C. K., and Truman, R. W. (2000). Comparative study of Mitsuda reaction to nude mouse and armadillo lepromin preparations using nine-banded armadillos. Int. J. Lepr. Mycobact. Dis. 68, 18–22.
Oliveira, I., Deps, P. D., and Antunes, J. (2019). Armadillos and leprosy: from infection to biological model. Rev. Inst. Med. Trop. Sao Paulo 61:e44. doi: 10.1590/s1678-9946201961044
Ortuno-Gutierrez, N., Baco, A., Braet, S., Younoussa, A., Mzembaba, A., Salim, Z., et al. (2019). Clustering of leprosy beyond the household level in a highly endemic setting on the Comoros, an observational study. BMC Infect. Dis. 19:501. doi: 10.1186/s12879-019-4116-y
Pena, M., Geluk, A., Van Der Ploeg-Van Schip, J. J., Franken, K. L., Sharma, R., and Truman, R. (2011). Cytokine responses to Mycobacterium leprae unique proteins differentiate between Mycobacterium leprae infected and naive armadillos. Lepr. Rev. 82, 422–431.
Pena, M. T., Sharma, R., and Truman, R. W. (2016). The armadillo model for leprosy [Online]. Available: https://www.internationaltextbookofleprosy.org/chapter/armadillos [Accessed 18 September 2016]
Pierneef, L., van Hooij, A., Taal, A., Rumbaut, R., Nobre, M. L., van Brakel, W., et al. (2021). Detection of anti-M. leprae antibodies in children in leprosy-endemic areas: A systematic review. PLoS Negl. Trop. Dis. 15:e0009667. doi: 10.1371/journal.pntd.0009667
Quaresma, J. A., Aarão, T. L., Sousa, J. R., Botelho, B. S., Barros, L. F., Araujo, R. S., et al. (2015). T-helper 17 cytokines expression in leprosy skin lesions. Br. J. Dermatol. 173, 565–567. doi: 10.1111/bjd.13608
Richardus, J. H., and Geluk, A. (2020). Reply to: Single-dose rifampicin and BCG to prevent leprosy. Int. J. Infect. Dis. 92, 271–272. doi: 10.1016/j.ijid.2020.01.052
Richardus, R., Alam, K., Kundu, K., Chandra Roy, J., Zafar, T., Chowdhury, A. S., et al. (2019). Effectiveness of single-dose rifampicin after BCG vaccination to prevent leprosy in close contacts of patients with newly diagnosed leprosy: A cluster randomized controlled trial. Int. J. Infect. Dis. 88, 65–72. doi: 10.1016/j.ijid.2019.08.035
Richardus, R. A., van der Zwet, K., van Hooij, A., Wilson, L., Oskam, L., Faber, R., et al. (2017). Longitudinal assessment of anti-PGL-I serology in contacts of leprosy patients in Bangladesh. PLoS Negl. Trop. Dis. 11:e0006083. doi: 10.1371/journal.pntd.0006083
Rojas-Espinosa, O., Quesada-Pascual, F., Oltra, A., Arce, P., Estrada-Parra, S., and Buchanan, T. M. (1985). Biochemical alterations in the serum of armadillos (Dasypus novemcinctus) infected with Mycobacterium leprae. A preliminary report. Int. J. Lepr. Mycobact. Dis. 53, 262–268.
Salgame, P., Abrams, J., Clayberger, C., Goldstein, H., Convit, J., Modlin, R., et al. (1991). Differing lymphokine profiles of functional subsets of human CD4 and CD8 T cell clones. Science 254, 279–282. doi: 10.1126/science.1681588
Schilling, A. K., McCurdy, K., Fish, A., Lurz, P. W. W., Geluk, A., Van Hooij, A., et al. (2021). Diagnosing and categorizing leprosy in live eurasian red squirrels (Sciurus Vulgaris) for management, surveillance, and translocation purposes. J. Zoo Wildl. Med. 52, 648–659. doi: 10.1638/2020-0066
Schilling, A.-K., van Hooij, A., Corstjens, P., Lurz, P. W. W., DelPozo, J., Stevenson, K., et al. (2019). Detection of humoral immunity to mycobacteria causing leprosy in Eurasian red squirrels (Sciurus vulgaris) using a quantitative rapid test. Eur. J. Wildl. Res. 65:3. doi: 10.1007/s10344-019-1287-1
Scollard, D. M., Adams, L. B., Gillis, T. P., Krahenbuhl, J. L., Truman, R. W., and Williams, D. L. (2006). The continuing challenges of leprosy. Clin. Microbiol. Rev. 19, 338–381. doi: 10.1128/CMR.19.2.338-381.2006
Sharma, R., Lahiri, R., Scollard, D. M., Pena, M., Williams, D. L., Adams, L. B., et al. (2013). The armadillo: a model for the neuropathy of leprosy and potentially other neurodegenerative diseases. Dis. Model. Mech. 6, 19–24. doi: 10.1242/dmm.010215
Sharma, R., Singh, P., Loughry, W. J., Lockhart, J. M., Inman, W. B., Duthie, M. S., et al. (2015). Zoonotic Leprosy in the Southeastern United States. Emerg. Infect. Dis. 21, 2127–2134. doi: 10.3201/eid2112.150501
TiemiNagao-Dias, A., Casimiro de Macedo, A., Rodrigues, R. O., Pedroza, F. H. C., Albuquerque, A. A., Moreira, F. A., et al. (2019). Serum anti-PGL-1 IgG, IgM, and IgA in a 3-year follow-up study of 4-15-year-old leprosy contacts. Pediatr. Infect. Dis. J. 38, e193–e198. doi: 10.1097/inf.0000000000002337
Tió-Coma, M., Avanzi, C., Verhard, E. M., Pierneef, L., van Hooij, A., Benjak, A., et al. (2020). Genomic characterization of Mycobacterium leprae to explore transmission patterns identifies new subtype in Bangladesh. Front. Microbiol. 11:1220. doi: 10.3389/fmicb.2020.01220
Truman, R. W., Ebenezer, G. J., Pena, M. T., Sharma, R., Balamayooran, G., Gillingwater, T. H., et al. (2014). The armadillo as a model for peripheral neuropathy in leprosy. Ilar J. 54, 304–314. doi: 10.1093/ilar/ilt050
Truman, R. W., and Krahenbuhl, J. L. (2001). Viable M. leprae as a research reagent. Int. J. Lepr. Mycobact. Dis. 69, 1–12.
Truman, R. W., Morales, M. J., Shannon, E. J., and Hastings, R. C. (1986). Evaluation of monitoring antibodies to PGL-I in armadillos experimentally infected with M. leprae. Int. J. Lepr. Mycobact. Dis. 54, 556–559.
Truman, R. W., Singh, P., Sharma, R., Busso, P., Rougemont, J., Paniz-Mondolfi, A., et al. (2011). Probable zoonotic leprosy in the southern United States. N. Engl. J. Med. 364, 1626–1633. doi: 10.1056/NEJMoa1010536
Vadiee, A. R., Shannon, E. J., Gillis, T. P., Mshana, R. N., and Hastings, R. C. (1988). Armadillo IgG and IgM antibody responses to phenolic glycolipid-I during experimental infection with M. leprae. Int. J. Lepr. Mycobact. Dis. 56, 422–427.
van Dijk, J. H. M., van Hooij, A., Groot, L. M., Geboers, J., Moretti, R., Verhard-Seymonsbergen, E., et al. (2021). Synthetic phenolic glycolipids for application in diagnostic tests for leprosy. Chembiochem 22, 1487–1493. doi: 10.1002/cbic.202000810
van Hooij, A., and Geluk, A. (2021). In search of biomarkers for leprosy by unraveling the host immune response to Mycobacterium leprae. Immunol. Rev. 2021:12966. doi: 10.1111/imr.12966
van Hooij, A., Tió-Coma, M., Verhard, E. M., Khatun, M., Alam, K., Tjon Kon Fat, E., et al. (2020). Household contacts of leprosy patients in endemic areas display a specific innate immunity profile. Front. Immunol. 11:1811. doi: 10.3389/fimmu.2020.01811
van Hooij, A., Tjon Kon Fat, E. M., Batista da Silva, M., Carvalho Bouth, R., Cunha Messias, A. C., Gobbo, A. R., et al. (2018). Evaluation of immunodiagnostic tests for leprosy in Brazil. China and Ethiopia. Sci. Rep. 8:17920. doi: 10.1038/s41598-018-36323-1
van Hooij, A., Tjon Kon Fat, E. M., de Jong, D., Khatun, M., Soren, S., Chowdhury, A. S., et al. (2021). Prototype multi-biomarker test for point-of-care leprosy diagnostics. iScience 24:1. doi: 10.1016/j.isci.2020.102006
van Hooij, A., Tjon Kon Fat, E. M., Richardus, R., van den Eeden, S. J., Wilson, L., de Dood, C. J., et al. (2016). Quantitative lateral flow strip assays as user-friendly tools to detect biomarker profiles for leprosy. Sci. Rep. 6:34260. doi: 10.1038/srep34260
van Hooij, A., Tjon Kon Fat, E. M., van den Eeden, S. J. F., Wilson, L., Batista da Silva, M., Salgado, C. G., et al. (2017). Field-friendly serological tests for determination of M. leprae-specific antibodies. Sci. Rep. 7:8868. doi: 10.1038/s41598-017-07803-7
van Hooij, A., van den Eeden, S., Richardus, R., Fat, E. T. K., Wilson, L., Franken, K., et al. (2019). Application of new host biomarker profiles in quantitative point-of-care tests facilitates leprosy diagnosis in the field. Ebiomedicine 47, 301–308. doi: 10.1016/j.ebiom.2019.08.009
Wallace, E., Hendrickson, D., Tolli, N., Mehaffy, C., Peña, M., Nick, J. A., et al. (2021). Culturing Mycobacteria. Methods Mol. Biol. 2314, 1–58. doi: 10.1007/978-1-0716-1460-0_1
WHO (2020). Global leprosy (Hansen disease) update, 2019: time to step-up prevention initiatives Weekly Epidemiological Record, Vol. 95. Geneva: WHO, 24.
Zenha, E. M., Ferreira, M. A., and Foss, N. T. (2009). Use of anti-PGL-1 antibodies to monitor therapy regimes in leprosy patients. Braz. J. Med. Biol. Res. 42, 968–972. doi: 10.1590/s0100-879x2009001000016
Keywords: antibodies, armadillo, diagnosis, lateral flow assay, leprosy, M. leprae, PGL-I, POC
Citation: Zhou Z, Pena M, van Hooij A, Pierneef L, de Jong D, Stevenson R, Walley R, Corstjens PLAM, Truman R, Adams L and Geluk A (2021) Detection and Monitoring of Mycobacterium leprae Infection in Nine Banded Armadillos (Dasypus novemcinctus) Using a Quantitative Rapid Test. Front. Microbiol. 12:763289. doi: 10.3389/fmicb.2021.763289
Received: 23 August 2021; Accepted: 04 October 2021;
Published: 28 October 2021.
Edited by:
John S. Spencer, Colorado State University, United StatesReviewed by:
Liang Wang, Institut Pasteur of Shanghai, Chinese Academy of Sciences (CAS), ChinaWilliam Loughry, Valdosta State University, United States
Copyright © 2021 Zhou, Pena, van Hooij, Pierneef, de Jong, Stevenson, Walley, Corstjens, Truman, Adams and Geluk. This is an open-access article distributed under the terms of the Creative Commons Attribution License (CC BY). The use, distribution or reproduction in other forums is permitted, provided the original author(s) and the copyright owner(s) are credited and that the original publication in this journal is cited, in accordance with accepted academic practice. No use, distribution or reproduction is permitted which does not comply with these terms.
*Correspondence: Annemieke Geluk, YS5nZWx1a0BsdW1jLm5s