- 1Laboratorio de Investigaciones Microbiológicas de Lagunas Andinas (LIMLA), Planta Piloto de Procesos Industriales Microbiológicos (PROIMI), CCT, CONICET, San Miguel de Tucumán, Argentina
- 2Laboratorio de Bioinformática Estructural, Instituto de Química Biológica de la Facultad de Ciencias Exactas y Naturales (IQUIBICEN)-CONICET, Universidad de Buenos Aires, Buenos Aires, Argentina
- 3Centro de Investigaciones Geológicas (CIG), Universidad Nacional de La Plata (UNLP)-CONICET, La Plata, Argentina
- 4Centro de Ecología Aplicada (CEA), Santiago, Chile
The Salar de Atacama in the Chilean Central Andes harbors unique microbial ecosystems due to extreme environmental conditions, such as high altitude, low oxygen pressure, high solar radiation, and high salinity. Combining X-ray diffraction analyses, scanning electron microscopy and molecular diversity studies, we have characterized twenty previously unexplored Andean microbial ecosystems in eight different lakes and wetlands from the middle-east and south-east regions of this salt flat. The mats and microbialites studied are mainly formed by calcium carbonate (aragonite and calcite) and halite, whereas the endoevaporites are composed predominantly of gypsum and halite. The carbonate-rich mats and microbialites are dominated by Bacteroidetes and Proteobacteria phyla. Within the phylum Proteobacteria, the most abundant classes are Alphaproteobacteria, Gammaproteobacteria and Deltaproteobacteria. While in the phylum Bacteroidetes, the most abundant classes are Bacteroidia and Rhodothermia. Cyanobacteria, Chloroflexi, Planctomycetes, and Verrucomicrobia phyla are also well-represented in the majority of these systems. Gypsum endoevaporites, on the contrary, are dominated by Proteobacteria, Bacteroidetes, and Euryarchaeota phyla. The Cyanobacteria phylum is also abundant in these systems, but it is less represented in comparison to mats and microbialites. Regarding the eukaryotic taxa, diatoms are key structural components in most of the microbial ecosystems studied. The genera of diatoms identified were Achnanthes, Fallacia, Halamphora, Mastogloia, Navicula, Nitzschia, and Surirella. Normally, in the mats and microbialites, diatoms form nano-globular carbonate aggregates with filamentous cyanobacteria and other prokaryotic cells, suggesting their participation in the mineral precipitation process. This work expands our knowledge of the microbial ecosystems inhabiting the extreme environments from the Central Andes region, which is important to ensure their protection and conservation.
Introduction
The Salar de Atacama (Figure 1), located in the Chilean Central Andes, is one of the Earth’s largest evaporite basins (ca. 3,000 km2) (Alonso and Risacher, 1996). It comprises numerous hypersaline lakes, locally called “lagunas,” at the edge of which evaporitic crusts form. A combination of extreme environmental conditions, such as high altitude, low barometric pressure, high solar and UV radiation, low rates of precipitation, high rates of evaporation, high salinity, strong winds, wide daily range in temperatures and the occurrence of hydrothermal activity makes this area one of the best primitive Earth analogs. Consequently, the study of the Andean microbial ecosystems (AMEs) that thrive in this environment may help us improve our understanding of the earliest complex ecosystems on Earth, as well as provide information for the search for life on other planets (Cabrol et al., 2009; Vignale et al., 2021).
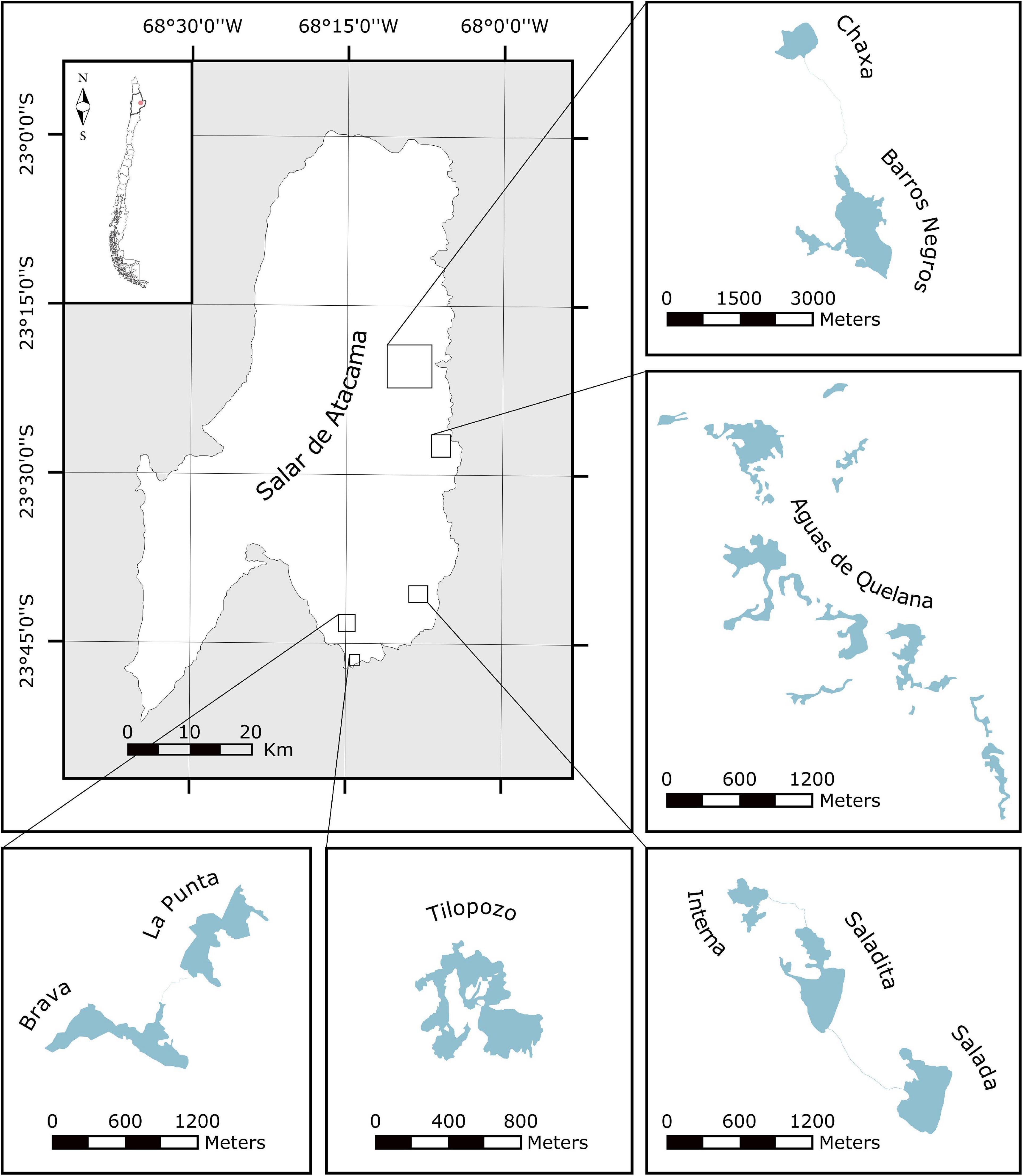
Figure 1. Location of the study area which consists of a system of several lakes and wetlands. The eight lakes and wetlands of this study are outlined in the five respective inserts.
In recent years, a variety of AMEs have been reported and studied in the Salar de Atacama. These include microbial mats at Laguna Cejar (∼2,300 m a.s.l.) (Rasuk et al., 2016), Laguna Tebenquiche (∼2,340 m a.s.l.) (Thiel et al., 2010; Farías et al., 2014; Fernandez et al., 2016; Kurth et al., 2021), Laguna Chaxa (∼2,300 m a.s.l.) (Thiel et al., 2010), and Laguna Brava (∼ 2,300 m a.s.l.) (Farías et al., 2014; Farias et al., 2017; Kurth et al., 2021); microbialites at Laguna Brava (Farías et al., 2014; Farias et al., 2017) and Laguna Interna (∼2,300 m a.s.l.) (Osman et al., 2021); and gypsum endoevaporites at Laguna de la Piedra (∼2,300 m a.s.l.) (Stivaletta et al., 2011) and Laguna Tebenquiche (Farías et al., 2014; Fernandez et al., 2016). Microbial mats are vertically laminated biofilms that occasionally lithify, forming organosedimentary structures called microbialites (Stolz, 2000). The mineralization of mats can be microbially induced or microbially influenced. In the former, microbial metabolic activities (e.g., photosynthesis, sulfate reduction, nitrate-driven sulfide oxidation) induce conditions for mineral precipitation, while in the latter, external environmental processes (e.g., degassing, evaporation) create the conditions for mineral precipitation. An organic matrix comprised of extracellular polymeric substances (EPS) is, however, involved in both microbially mediated mineralizations providing a template for mineral nucleation (Dupraz and Visscher, 2005; Dupraz et al., 2009). In the high-altitude Andean lakes (HAALs) of the Central Andes, both mineralization processes take place (Gomez et al., 2014, 2018; Vignale et al., 2021). Evaporites, on the other hand, are salt deposits precipitated from a saturated brine via solar evaporation (Warren, 2016). Occasionally, they present endolithic microbial communities named endoevaporites. The minerals that form the evaporites, mainly halite (NaCl) and gypsum (CaSO4⋅2H2O), provide protection against desiccation and UV-B radiation but allow photosynthetically active radiation (400–700 nm) to pass through, which is important for the development of photoautotrophs (Stivaletta et al., 2011; Rasuk et al., 2014; Fernandez et al., 2016; Vignale et al., 2021).
The aim of this study is to expand our knowledge of the AMEs in the extreme environments of Salar de Atacama. To fulfill this objective, first we identified previously unknown microbial ecosystems (biofilms, microbial mats, microbialites, and endoevaporites) in eight different lakes and wetlands from Salar de Atacama, and then we analyzed the composition of their mineral phase and microbiota. Finally, we performed comparative analyses of the data to determine if there was a correlation between these features.
Materials and Methods
Sample Collection
In the first place, a delimitation of the study area (Figure 1) was performed through the analysis of satellite and aerial images obtained from Google Earth and a DJI® Mavic Pro drone, respectively. Afterward, three field campaigns (24–28 November 2017, 24–26 November 2018, and 17 May 2018) were carried out to explore the respective area and search for AMEs.
In total, twenty previously undescribed AMEs were identified in eight different lakes and wetlands from the middle-east and south-east regions of Salar de Atacama. These are a floating biofilm from Tilopozo (T); microbial mats from Aguas de Quelana (Q1 and Q2), Laguna Salada (S1), Laguna La Punta (P1 and P2) and Laguna Brava (B1); microbialites from Laguna Chaxa (CH1, CH2, and CH3), Laguna Interna (I), Laguna La Punta (P3) and Laguna Brava (B2, B3, and B4); endoevaporites from Laguna Barros Negros (BN1, BN2, and BN3); a microbial community within an Andean flamingo (Phoenicoparrus andinus) mound nest from Laguna Salada (S2) and a sediment-associated microbial community from Aguas de Quelana (Q3).
Bulk samples were taken from the respective AMEs to perform mineralogy and scanning electron microscopy (SEM) analyses. These samples were stored in the dark at 4°C, and processed within 1–2 weeks. Additionally, samples were taken from different layers of the AMEs to perform 16S rRNA diversity analyses (Table 1). These samples were stored in RNAlater® solution (Thermo Fisher Scientific, United States) at 4°C in the dark, and processed within a week.
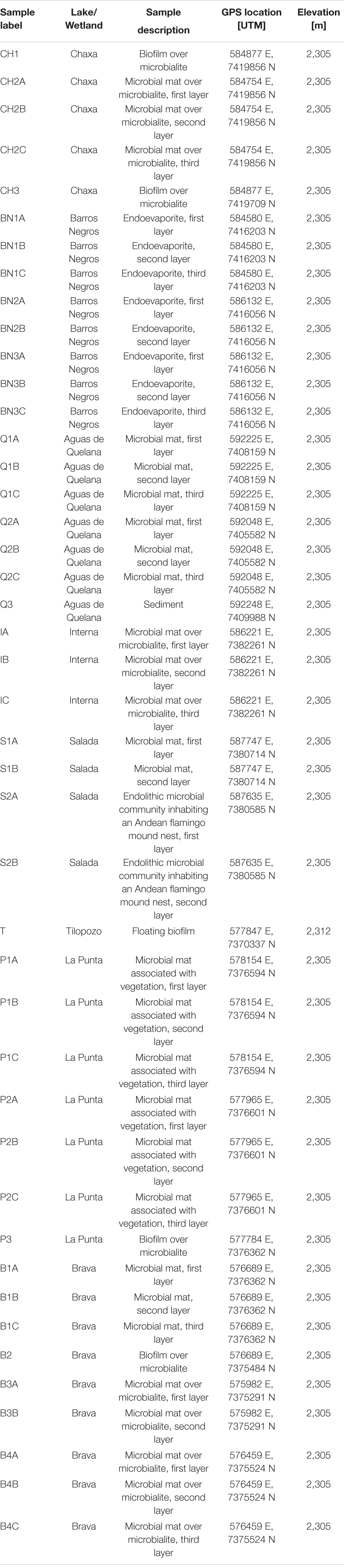
Table 1. Overview of samples used to perform 16S rRNA diversity analyses, locations and coordinates.
Bulk Mineralogy Analyses
The mineral composition of the samples was determined by X-ray diffraction (XRD) analyses, which were carried out on finely ground sample material (<20 μm) employing a PANalytical X’Pert Pro Multipurpose Powder Diffractometer, with a Cu X-ray source (kα = 1.5403 Å), operated at 40 kV and 40 mA at Centro de Investigaciones Geológicas (CIG), La Plata, Argentina. The dried and ground samples were scanned from 2 to 40 2θ, with a scanning speed of 0.04°/s and a time per step of 0.50 s. For each XRD pattern, semi-quantification of the minerals was obtained from the intensity of the main peaks (Schultz, 1964; Moore and Reynolds, 1989). The mineral composition of the samples was used to perform a chloride, sulfate and carbonate ternary diagram with the R package ggtern (Hamilton and Ferry, 2018).
Scanning Electron Microscopy (SEM) Analyses
Samples were fixed overnight at 4°C in modified Karnovsky fixative, composed of formaldehyde (8% v/v), glutaraldehyde (16% v/v) and phosphate-buffered saline (PBS; 0.2 M, pH 7.4). Afterward, the samples were washed three times with PBS and calcium chloride (CaCl2) for 10 min, and fixed with osmium tetroxide (2% v/v) overnight. Finally, after washing twice with ethanol (30% v/v) for 10 min, the samples were dried at critical point and sputtered with gold. Specimens were observed under vacuum using a Zeiss Supra 55VP (Carl Zeiss NTS GmbH, Germany) scanning electron microscope at Centro Integral de Microscopía Electrónica (CIME), Tucumán, Argentina. Biofilm from Tilopozo could not be imaged using SEM due to difficulties encountered during sample preparation. Identification of diatom taxa, based on morphological features of their frustules, was carried out employing SEM images and identification keys (Cristóbal et al., 2020). The morphological features analyzed were: size, shape and symmetry of valves; presence, location and structure of the axial area; presence, location and structure of raphe; presence of septa; presence and structure of costae; location, structure and density of striae; and presence of stauros, among others.
DNA Extraction and Amplicon Sequencing
Total genomic DNA was isolated from the samples, detailed in Table 1, using the FastDNA® SPIN Kit for Soil (MP Biomedicals, United States) according to the protocol provided by the manufacturer. DNA yield (ng μl–1) was quantified spectrophotometrically with a NanoDrop 2000c Spectrophotometer (Thermo Fisher Scientific, United States). NanoDrop was also used to estimate the purity of the extracted DNA. Sample BN3A could not be further processed as not enough DNA was extracted from it. The hypervariable regions V3 and V4 of the bacterial and archaeal 16S rRNA gene were amplified using the primers Bakt_341F (CCTACGGGNGGCWGCAG) and Bakt_805R (GACTACHVGGGTATCTAATCC). The amplicons obtained were sequenced by Macrogen Inc., (Seoul, South Korea) using an Illumina MiSeq sequencer platform. Raw sequences were submitted to the ENA Project database under the accession number PRJEB44218.
Bioinformatics
Raw Sequence data were analyzed with the software package QIIME 2 v.2018.2, following the tutorials provided by the developers (Caporaso et al., 2010; Bolyen et al., 2018). Taxonomic annotation was performed in QIIME 2 using the SILVA database (No. 132, April 2018) (Quast et al., 2013) trained with 16S rRNA genes grouped in OTUs with 97% similarity. OTUs classified as coming from chloroplast or mitochondria were removed before further analyses. The R package phyloseq (McMurdie and Holmes, 2013) was used to calculate alpha diversity metrics (Chao1, ACE, Shannon and Simpson) after rarefying the data, and perform a principal coordinate analysis (PCoA) based on Bray-Curtis dissimilarity. The R package vegan (Oksanen et al., 2013) was used to perform a redundancy analysis (RDA) and correlate mineralogy with the microbial composition of the samples. Permutational ANOVA (PERMANOVA) tests were also carried out with the R package vegan to evaluate the PCoA and RDA analyses.
Results
Microbial Ecosystems Discovered in Salar de Atacama
The exploration carried out in the middle-east and south-east regions of Salar de Atacama allowed us to identify and study twenty previously unreported AMEs in eight different lakes and wetlands: Laguna Chaxa, Laguna Barros Negros, Aguas de Quelana, Laguna Interna, Laguna Salada, Laguna La Punta, Laguna Brava, and Tilopozo.
Laguna Chaxa is a small lake (∼0.35 km2 area) from the middle-east region of Salar de Atacama (Figure 1). A previous study reported the presence of red–purple-colored microbial mats developing in this lake (Thiel et al., 2010). In addition to these microbial mats, we have discovered modern microbialites developing in this environment (Figure 2). Some of these microbialites are covered by black or pink biofilms (CH1 and CH3, respectively), while others are covered by microbial mats that usually display a sequence of (from top to bottom) pink/orange, green and purple colored layers (CH2).
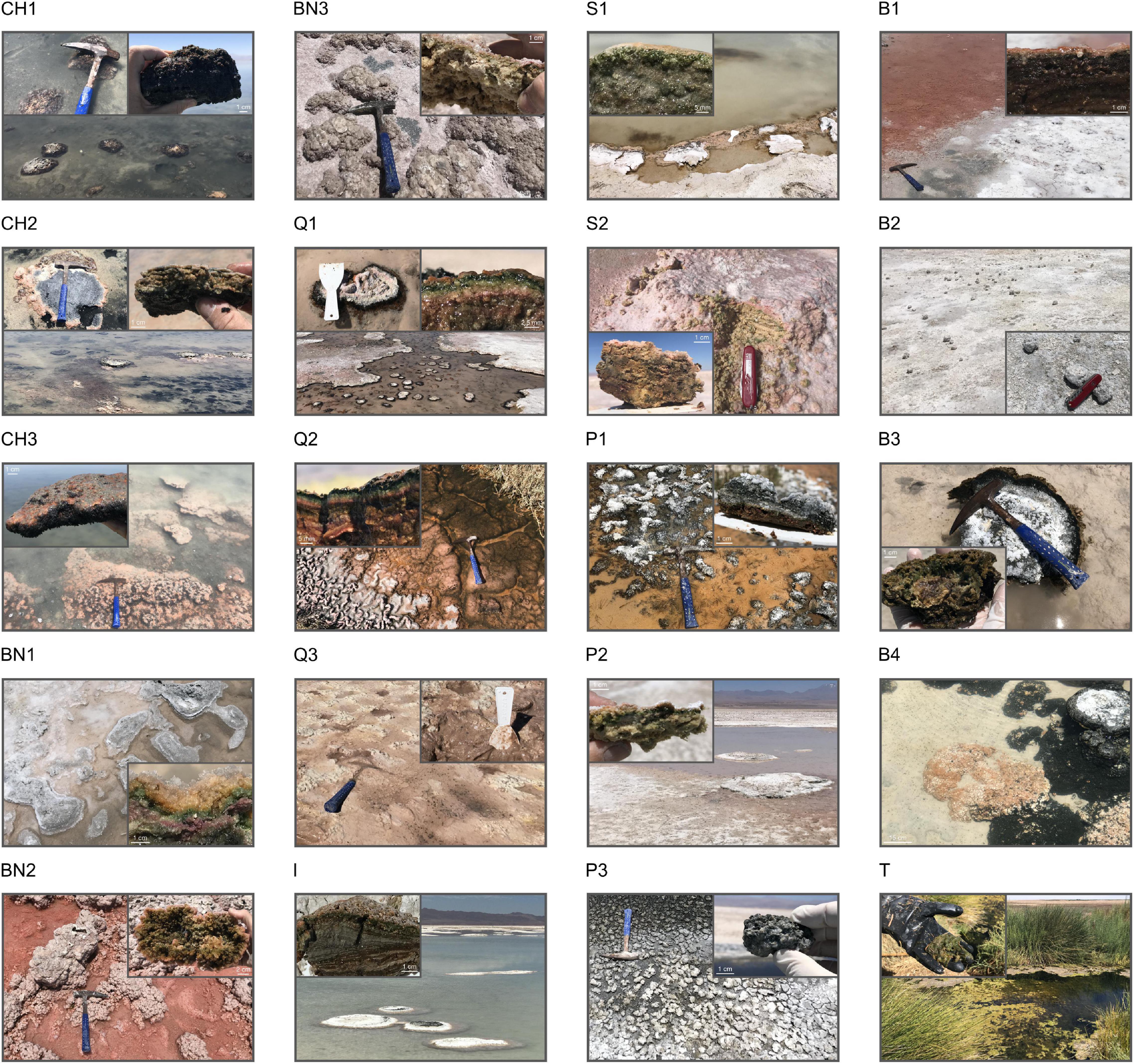
Figure 2. General views of the AMEs discovered in Salar de Atacama. CH1, CH2 and CH3, Microbialites from Laguna Chaxa; BN1, BN2 and BN3, Endoevaporites from Laguna Barros Negros; Q1 and Q2, Microbial mats from Aguas de Quelana; Q3, Sediment-associated microbial community from Aguas de Quelana; I, Microbialite from Laguna Interna; S1, Microbial mat from Laguna Salada; S2, Microbial community within an Andean flamingo mound nest from Laguna Salada; P1 and P2, Microbial mats from Laguna La Punta; P3, Microbialite from Laguna La Punta; B1, Microbial mat from Laguna Brava; B2, B3 and B4, Microbialites from Laguna Brava; T, Floating biofilm from Tilopozo.
Two kilometers south from Laguna Chaxa is Laguna Barros Negros (∼1.38 km2 area) (Figure 1). In this lake, contrary to Laguna Chaxa, we have identified numerous microbial communities developing inside evaporite deposits (endoevaporites) forming colored strata (Figure 2). Normally, a yellow/orange layer (ca. 0.5–2 cm thick) is visible on the top, followed by a green layer of similar thickness (BN2). In some cases, a third purple layer is visible at the bottom as well (BN1 and BN3).
Aguas de Quelana is a wetland complex (∼1.5 km2 area) also located in the middle-east region of Salar de Atacama (Figure 1). Neither microbialites nor endoevaporites could be identified in the explored area of this environment. Nevertheless, microbial mats (Q1 and Q2) and pink/orange sediment-associated microbial communities (Q3) were observed along the shorelines (Figure 2). Like the endoevaporites from Laguna Barros Negros, the microbial mats form colored strata. Normally, the first layer (rich in EPS) varies in color and thickness (ca. 0.1–0.3 cm) from one mat to another, while the second and third layers are green and purple, respectively, and usually have a similar thickness (ca. 0.2 cm). Occasionally, a dark layer can be found at the bottom as well. When mats are exposed to the air, a white evaporitic crust covers their surface. Cerebroid, snake and globular morphologies have been observed in these microbial mats, which usually accumulate gas at the subsurface.
Laguna Interna (∼0.08 km2 area) and Laguna Salada (∼0.18 km2 area) are two connected lakes located in the south-east region of Salar de Atacama (Figure 1). In Laguna Interna, we have discovered modern microbialites (I) that exhibit a laminated mesostructure (stromatolites), covered by microbial mats that usually display a sequence of (from top to bottom) pink/orange, green and purple colored layers (Figure 2). These microbialites are similar to those recently reported in this environment (Osman et al., 2021). In Laguna Salada, on the contrary, we have identified microbial mats (S1) that form colored strata (Figure 2). Typically, a pink/orange layer (ca. 0.1–0.2 cm thick) is visible on the top, followed by a green layer of similar thickness. Some of these microbial mats are close to Andean flamingo mound nests which are, in most of the cases, no longer used by the wading birds. The dissection of these mound nests revealed endolithic microbial communities (S2) forming colored strata, similar to the ones present in the evaporite deposits from Laguna Barros Negros (Figure 2). As far as we know, this is the first work to report a microbial ecosystem of this kind in the Central Andes.
Laguna La Punta (∼0.16 km2 area) and Laguna Brava (∼0.20 km2 area) are two connected lakes from the south of Salar de Atacama that have already been explored (Figure 1). Nevertheless, in both lakes, we have identified previously unknown microbial mats and microbialites. In Laguna La Punta, we have discovered microbial mats associated with vegetation (P1 and P2) that usually display a sequence of (from top to bottom) pink/orange, green and purple colored layers, with cerebroid, snake and globular morphologies, as well as microbialites covered by black biofilms (P3) (Figure 2). Whereas in Laguna Brava, we have discovered red-green-colored microbial mats (B1), oncoids (microbialites that display a concentrically laminated mesostructure) (Logan et al., 1964) covered by black biofilms (B2) and microbialites covered by microbial mats (B3 and B4) that usually display a sequence of (from top to bottom) pink/orange, green and purple colored layers (Figure 2).
The last system prospected was Tilopozo (∼0.34 km2 area), a wetland complex located in the south region of Salar de Atacama (Figure 1). Compared to the other systems studied, we have not identified in this environment carbonate or evaporite deposits associated with microbial communities. Instead, we have discovered green floating biofilms (T) covering a great proportion of the wetland surface (Figure 2).
Mineralogical Analyses
X-ray diffraction analyses were carried out on the samples collected (Supplementary Figures 1–3) to determine and compare the mineralogical composition of the AMEs studied (Figure 3 and Supplementary Table 1). The endoevaporites from Laguna Barros Negros (BN1, BN2 and BN3), as well as the microbial community within the Andean flamingo mound nest from Laguna Salada (S2) are mainly formed by gypsum (ca. 40–65%) and halite (ca. 10–65%), which probably explain their morphological similarities. As the endoevaporite BN3 was located in a drier area compared to the rest of the endoevaporites from Laguna Barros Negros, it also contains a considerable amount (ca. 25%) of anhydrite (CaSO4).
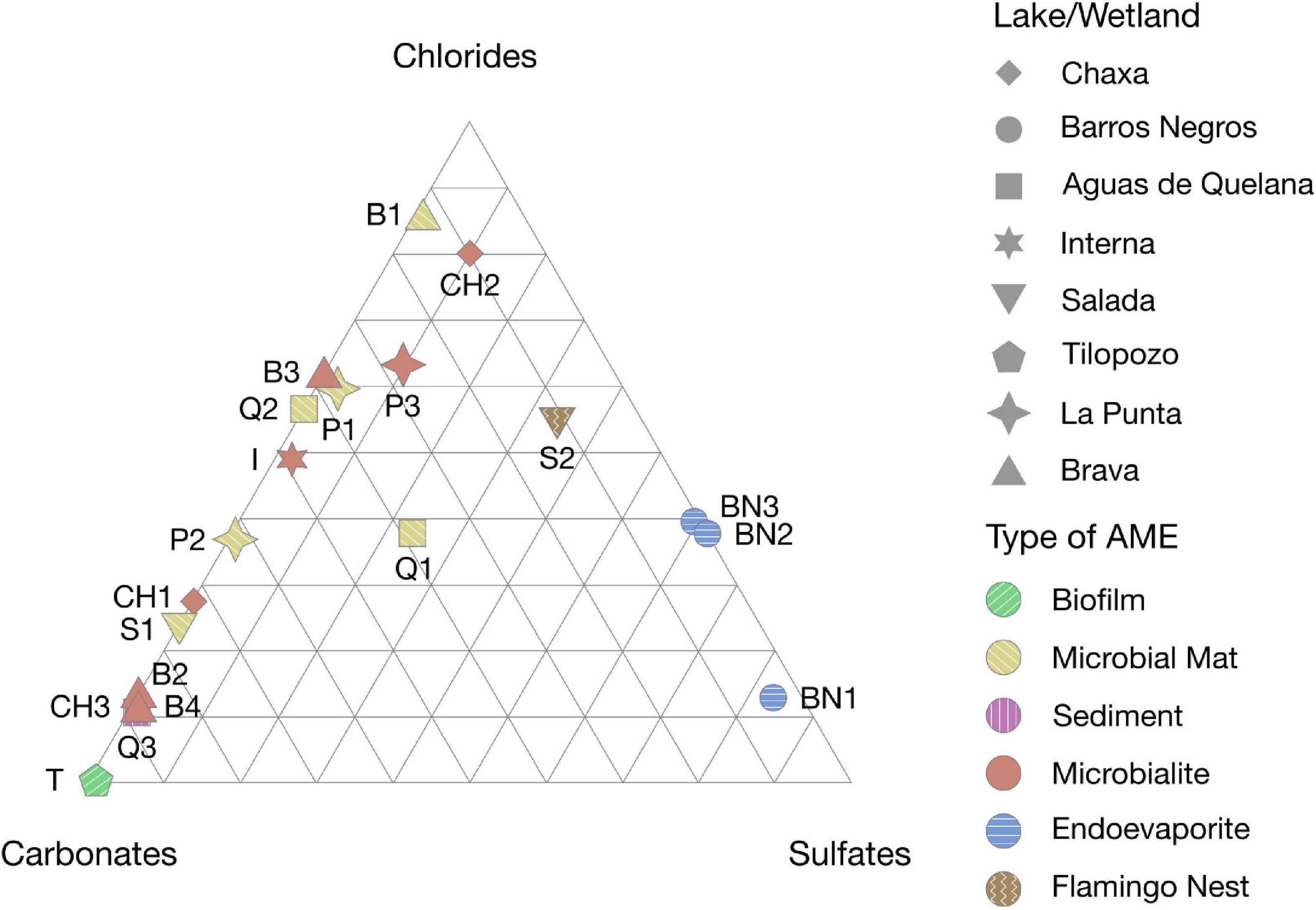
Figure 3. Ternary diagram representing the mineral composition of the AMEs. The shapes and colors indicate the location and type of AME, respectively.
The microbial mats from Aguas de Quelana (Q2) and Laguna Salada (S1) are comprised predominantly of halite (ca. 25–65%), aragonite (CaCO3) (ca. 25–40%) and calcite (CaCO3) (ca. 25–40%). Whereas the microbial mats from Laguna La Punta (P1 and P2) and Laguna Brava (B1), as well as the pink/orange sediment from Aguas de Quelana (Q3), are composed mainly of halite (ca. 10–80%) and aragonite (ca. 10–80%), but do not contain great amounts of calcite (ca. 1–3%). This is also observed in the microbial mat Q1 from Aguas de Quelana, which is deficient in calcite (ca. 1%) but contains a considerable amount of aragonite (ca. 40%), halite (ca. 40%) and gypsum (ca. 25%).
The microbialites from Laguna Chaxa (CH1, CH2 and CH3), Laguna Brava (B3 and B4) and Laguna La Punta (P3), the stromatolite from Laguna Interna (I), and the oncoid from Laguna Brava (B2) are mostly formed by aragonite (ca. 10–80%) and halite (ca. 10–80%). In addition to these minerals, the stromatolite from Laguna Interna (I) contains a great proportion of calcite (ca. 25%), and the microbialite from Laguna La Punta (P3) contains considerable amounts of gypsum (ca. 10%) and opal-A (SiO2⋅nH2O) (ca. 10%).
The floating biofilm from Tilopozo (T) has a mineralogical composition quite different from the rest of the microbial ecosystems studied. Although its principal component is calcite (ca. 65%), it also contains clay (ca. 25%), dolomite (CaMg(CO3)2) (ca. 25%), quartz (SiO2) (ca. 10%), siderite (FeCO3) (ca. 10%) and dawsonite (NaAlCO3(OH)2) (ca. 10%) in great proportion.
Scanning Electron Microscopy Analyses
Scanning electron microscopy analyses were performed on the samples to study the association of the prokaryotic and eukaryotic microorganisms with the minerals present in the AMEs studied (Figure 4 and Supplementary Figures 4–6). The SEM images obtained from the microbialite samples (Figure 4A and Supplementary Figure 4) revealed the presence of diatoms (Bacillariophyceae), filamentous cyanobacteria and other prokaryotic cells (cocci and bacilli) forming nano-globular carbonate aggregates, which suggests their participation in the mineral precipitation process (Gomez et al., 2018). Based on morphological characteristics, we could identify at least four families of diatoms: Achnanthaceae (Achnanthes spp.), Bacillariaceae (Nitzschia spp.), Catenulaceae (Halamphora spp.), and Naviculaceae (Navicula spp.). A multicellular eukaryotic organism was also identified in one of the microbialite samples (CH1). This was a free-living roundworm (Nematoda) that, based on the shape of its mouth (hollow tube) (Supplementary Figure 4), feeds on the prokaryotes that cover the microbialites (McSorley, 1997).
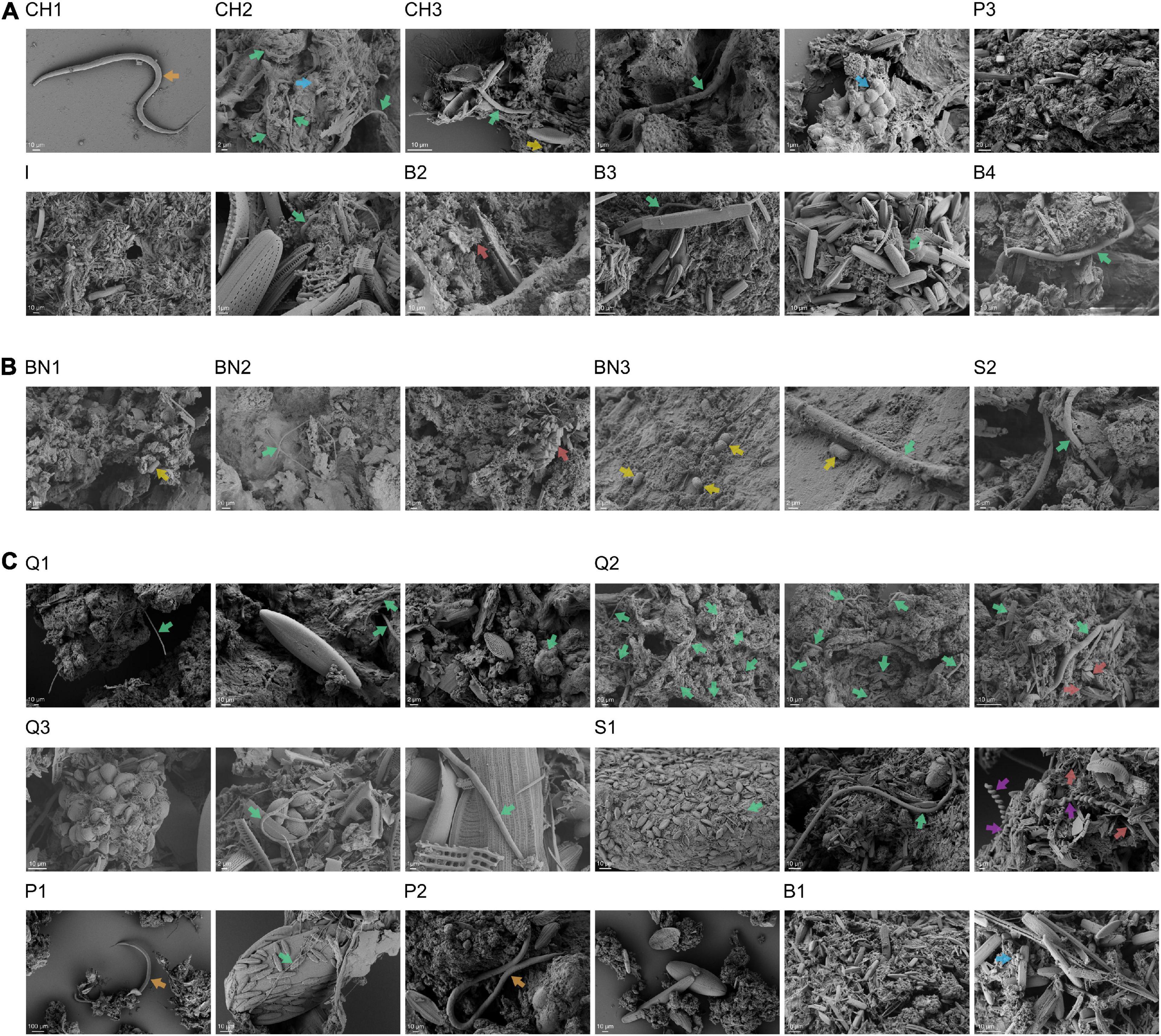
Figure 4. Diatoms, filamentous cyanobacteria and other prokaryotic cells form nano-globular carbonate aggregates in most of the AMEs. (A) SEM images of the microbialites. CH1, CH2 and CH3, Microbialites from Laguna Chaxa; P3, Microbialite from Laguna La Punta; I, Microbialite from Laguna Interna; B2, B3 and B4, Microbialites from Laguna Brava. (B) SEM images of the endolithic microbial communities. BN1, BN2 and BN3, Endoevaporites from Laguna Barros Negros; S2, Microbial community within an Andean flamingo mound nest from Laguna Salada. (C) SEM images of the microbial mats and the pink/orange sediment. Q1 and Q2, Microbial mats from Aguas de Quelana; Q3, Sediment-associated microbial community from Aguas de Quelana; S1, Microbial mat from Laguna Salada; P1 and P2, Microbial mats from Laguna La Punta; B1, Microbial mat from Laguna Brava. Different recognized structures are marked with an arrow. Green arrow, Filamentous cyanobacterium; Blue arrow, Coccus; Yellow arrow, Bacillus; Purple arrow, Spirochete; Orange arrow, Nematode; Red arrow, Mineral grain.
The SEM images of the endoevaporites and the endolithic microbial community inhabiting the Andean flamingo mound nest (Figure 4B and Supplementary Figure 5) also revealed the presence of diatoms, filamentous cyanobacteria and other prokaryotic cells (bacilli). In this case, the identified families of diatoms were Achnanthaceae (Achnanthes spp.) and Naviculaceae (Navicula spp.). But compared to the microbialite systems, the number of diatoms and filamentous cyanobacteria in these systems seemed to be lower.
In the microbial mats and pink/orange sediment (Figure 4C and Supplementary Figure 6), the SEM images showed a great number of diatoms, filamentous cyanobacteria, and other prokaryotic cells (cocci and spirochetes). Like in the microbialites, these microorganisms form aggregates with precipitation of nano-globular carbonates, suggesting their participation in the mineral precipitation process (Gomez et al., 2018). The families of diatoms identified in the microbial mats were Achnanthaceae (Achnanthes spp.), Bacillariaceae (Nitzschia spp.), Catenulaceae (Halamphora spp.), Mastogloiaceae (Mastogloia spp.), Naviculaceae (Navicula spp.), and Surirellaceae (Surirella spp.), while the families of diatoms identified in the pink/orange sediment were Achnanthaceae (Achnanthes spp.), Bacillariaceae (Nitzschia spp.), Catenulaceae (Halamphora spp.), Mastogloiaceae (Mastogloia spp.), Naviculaceae (Navicula spp.), Sellaphoraceae (Fallacia spp.), and Surirellaceae (Surirella spp.). Free-living roundworms were also observed in the microbial mats (P1 and P2), supporting their role as bacterivorous in these systems.
Taxonomy-Based Analyses
Molecular diversity analyses based on the small subunit ribosomal (SSU) rRNA of Bacteria and Archaea were carried out on the samples (Table 1) to determine the prokaryotic composition of the AMEs studied. The results revealed that the taxonomic composition of prokaryotes not only differs between types of AMEs, but also between their layers (Figure 5).
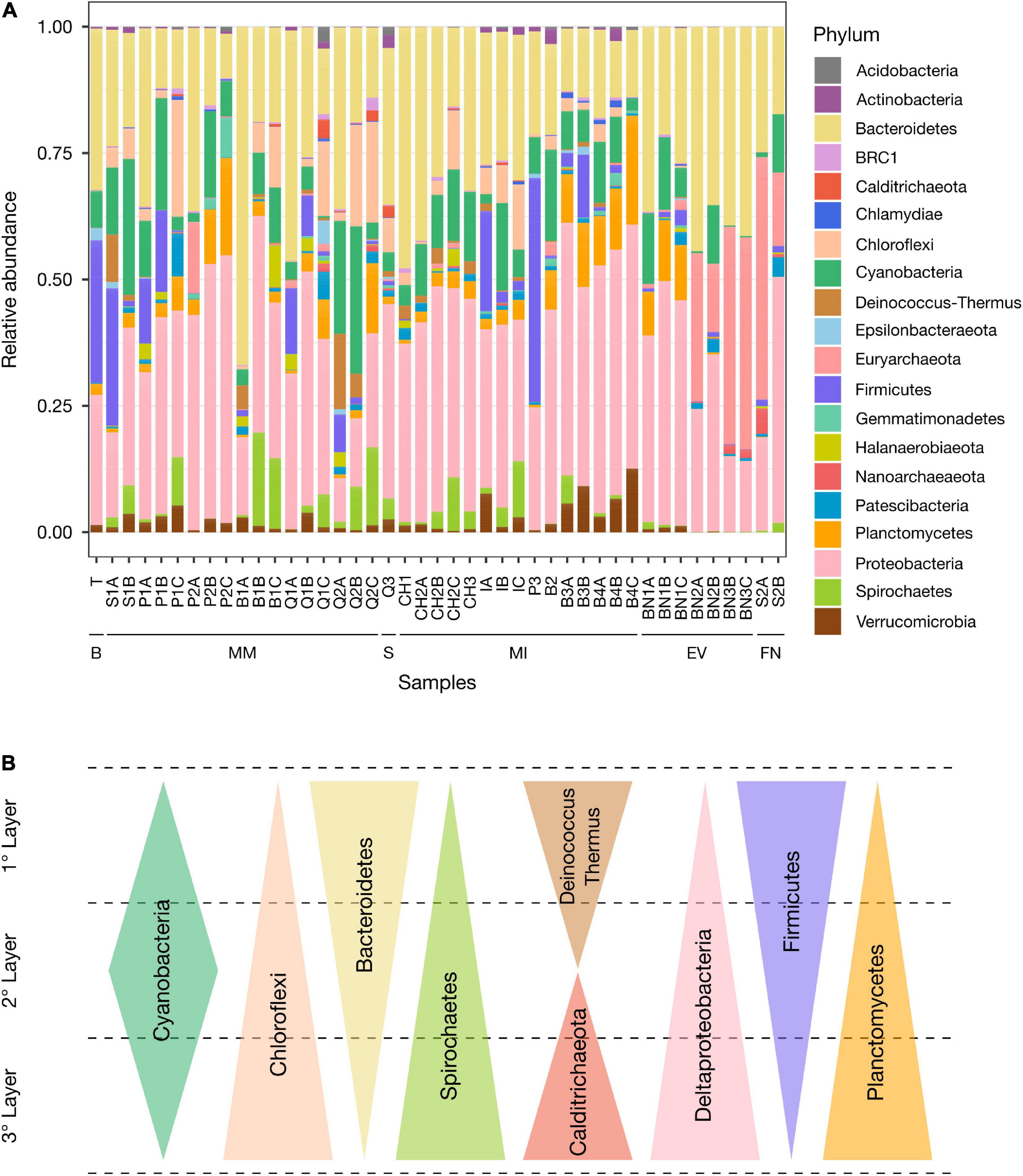
Figure 5. Taxonomic composition of prokaryotes in the AMEs. (A) Relative abundance of the twenty major phyla in the different samples. B, Biofilm; MM, Microbial mat; S, Sediment; MI, Microbialite; EV, Endoevaporite; FN, Andean flamingo mound nest. (B) Graphical summary of taxa depth distributions observed in the microbial mats.
The dominant phyla in the majority of the microbial mats are Bacteroidetes (ca. 8.78–66.95%) and Proteobacteria (ca. 8.59–52.96%), followed by Cyanobacteria (ca. 0.41–29.15%), Firmicutes (ca. 0.02–27.19%), Chloroflexi (ca. 0–23.10%), and Spirochetes (ca. 0.02–18.44%). Planctomycetes (ca. 0.48–19.27%) and Verrucomicrobia (ca. 0.41–5.33%) phyla are also well-represented (Figure 5A). Within the phylum Proteobacteria, the most abundant classes are Alphaproteobacteria (ca. 5.23–31.23%), Gammaproteobacteria (ca. 1.09–27.24%), and Deltaproteobacteria (ca. 0.35–17.29%) (Supplementary Figures 7, 8). While in the phylum Bacteroidetes, the most abundant classes are Bacteroidia (ca. 0.54–62.70%) and Rhodothermia (ca. 0.38–29.85%) (Supplementary Figures 7, 9).
In most carbonate microbialites, Proteobacteria (ca. 24.28–50.00%) and Bacteroidetes (ca. 11.14–47.73%) are the dominant phyla, followed by Cyanobacteria (ca. 1.87–18.08%) and Chloroflexi (ca. 0.01–12.89%). Planctomycetes (ca. 0.51–21.55%) and Verrucomicrobia (ca. 0.30–12.59%) phyla are also abundant, especially in Laguna Brava samples. Contrary to microbial mats, the phylum Firmicutes is underrepresented in the majority of the microbialites, except in the ones from Laguna La Punta (P3), Laguna Interna (I) and Laguna Brava (B3) (ca. 44.37%, 1.84–19.83%, and 2.68–12.45%, respectively) (Figure 5A). Within the phylum Proteobacteria, the most abundant classes are Alphaproteobacteria (ca. 10.97–32.96%), Gammaproteobacteria (ca. 3.74–24.20%) and Deltaproteobacteria (ca. 0.62–18.12%) (Supplementary Figures 7, 8). Whereas in the phylum Bacteroidetes, the most abundant classes are Bacteroidia (ca. 1.82–29.32%) and Rhodothermia (ca. 0.59–26.29%) (Supplementary Figures 7, 9). Like in microbial mats and microbialites, the dominant phyla in the pink/orange sediment are Proteobacteria (ca. 38.47%) and Bacteroidetes (ca. 31.01%), followed by Chloroflexi (ca. 6.79%), Spirochetes (ca. 4.15%), and Cyanobacteria (ca. 3.74%) (Figure 5A).
The taxonomic composition of gypsum endoevaporites differs greatly from that of microbial mats and microbialites. Proteobacteria (ca. 14.03–48.24%), Bacteroidetes (ca. 21.29–44.39%), and Euryarchaeota (ca. 0.40–43.04%) phyla dominate the majority of these microbial ecosystems. The Cyanobacteria phylum is also abundant (ca. 0.24–14.36%), but in comparison to microbial mats and microbialites, it is less represented (Figure 5A). Within the phylum Proteobacteria, the most abundant classes are Alphaproteobacteria (ca. 8.39–23.79%) and Gammaproteobacteria (ca. 4.58–23.04%). Deltaproteobacteria class is present, but underrepresented (ca. 1.06–2.63%) (Supplementary Figures 7, 8). The most abundant classes in the Bacteroidetes phylum are Rhodothermia (ca. 20.40–41.99%) and Bacteroidia (ca. 0.88–4.98%). Interestingly, in all the endoevaporites, Rhodothermia class is more abundant than Bacteroidia class. However, in most of the microbial mats and microbialites, Bacteroidia class is more abundant than Rhodothermia class (Supplementary Figures 7, 9). Within the Euryarchaeota phylum, the dominant class is Halobacteria (ca. 0.40–43.05%) (Supplementary Figure 7). Another interesting observation is the low proportion of Firmicutes (ca. 0.06–3.05%), Spirochetes (ca. 0.02–1.39%), Verrucomicrobia (ca. 0.02–1.23%), Chloroflexi (ca. 0.00–0.39%), and Deinococcus-Thermus (0.01–0.29%) phyla, which are normally well-represented in the microbial mats and microbialites. The taxonomic composition of the Andean flamingo mound nest resulted to be similar to that of gypsum endoevaporites, with Proteobacteria (ca. 18.45–48–63%), Euryarchaeota (ca. 14.56–47.94%) and Bacteroidetes (ca. 17.27–24.60%) as the dominant phyla (Figure 5A).
Regarding the taxonomic depth distributions (Figure 5B), in the microbial mats, Deinococcus-Thermus (1° layer: 0–14.93%, 2° layer: 0–4.66%, 3° layer: 0–0.51%), Firmicutes (1° layer: 0.09–27.19%, 2° layer: 0.02–16.16%, 3° layer: 0.03–0.90%) and Bacteroidetes (1° layer: 23.04–66.95%, 2° layer: 11.79–25.72%, 3° layer: 8.78–18.78%) taxa normally reduce their abundance with depth. While Calditrichaeota (1° layer: 0–0.22%, 2° layer: 0–0.12%, 3° layer: 0–3.76%), Chloroflexi (1° layer: 0–3.98%, 2° layer: 0–20.11%, 3° layer: 0.05–23.10%), Spirochetes (1° layer: 0.02–1.98%, 2° layer: 0.08–18.44%, 3° layer: 0.02–15.43%), Deltaproteobacteria (1° layer: 0.35–2.73%, 2° layer: 1.48–5.82%, 3° layer: 5.68–17.29%), and Planctomycetes (1° layer: 0.48–3.09%, 2° layer: 1.63–10.83%, 3° layer: 2.17–19.27%) taxa increase it. The Cyanobacteria phylum, contrary to other taxa, is more abundant in the middle layers than in the upper or lower ones (1° layer: 1.73–22.32%, 2° layer: 4.55–29.15%, 3° layer: 0.41–10.90%). With regard to Gammaproteobacteria (1° layer: 1.09–26.45%, 2° layer: 2.18–27.03%, 3° layer: 1.75–27.24%) and Alphaproteobacteria (1° layer: 5.79–22.04%, 2° layer: 5.24–31.22%, 3° layer: 5.79–19.38%) taxa, no common patterns are observed. Although the microbialites CH2, I, B3, and B4 are covered by microbial mats, their taxa do not present depth-related distribution patterns. In the endoevaporites and the Andean flamingo mound nest, the only depth-related distribution pattern observed is the abundance increase of Patescibacteria phylum with depth (1° layer: 0–1.15%, 2° layer: 0.29–3.90%, 3° layer: 0.51–2.58%).
Diversity Analyses
Alpha diversity metrics (Chao1 and Shannon indexes) were estimated, after even subsampling (rarefaction), to determine the community structure of each sample (Figure 6A and Supplementary Table 2). The comparison of these metrics, evaluated with Wilcoxon rank-sum test (Mann-Whitney) (Wilcoxon, 1945), revealed that both richness and diversity do not differ significantly (P > 0.05) between types of AMEs, nor between layers.
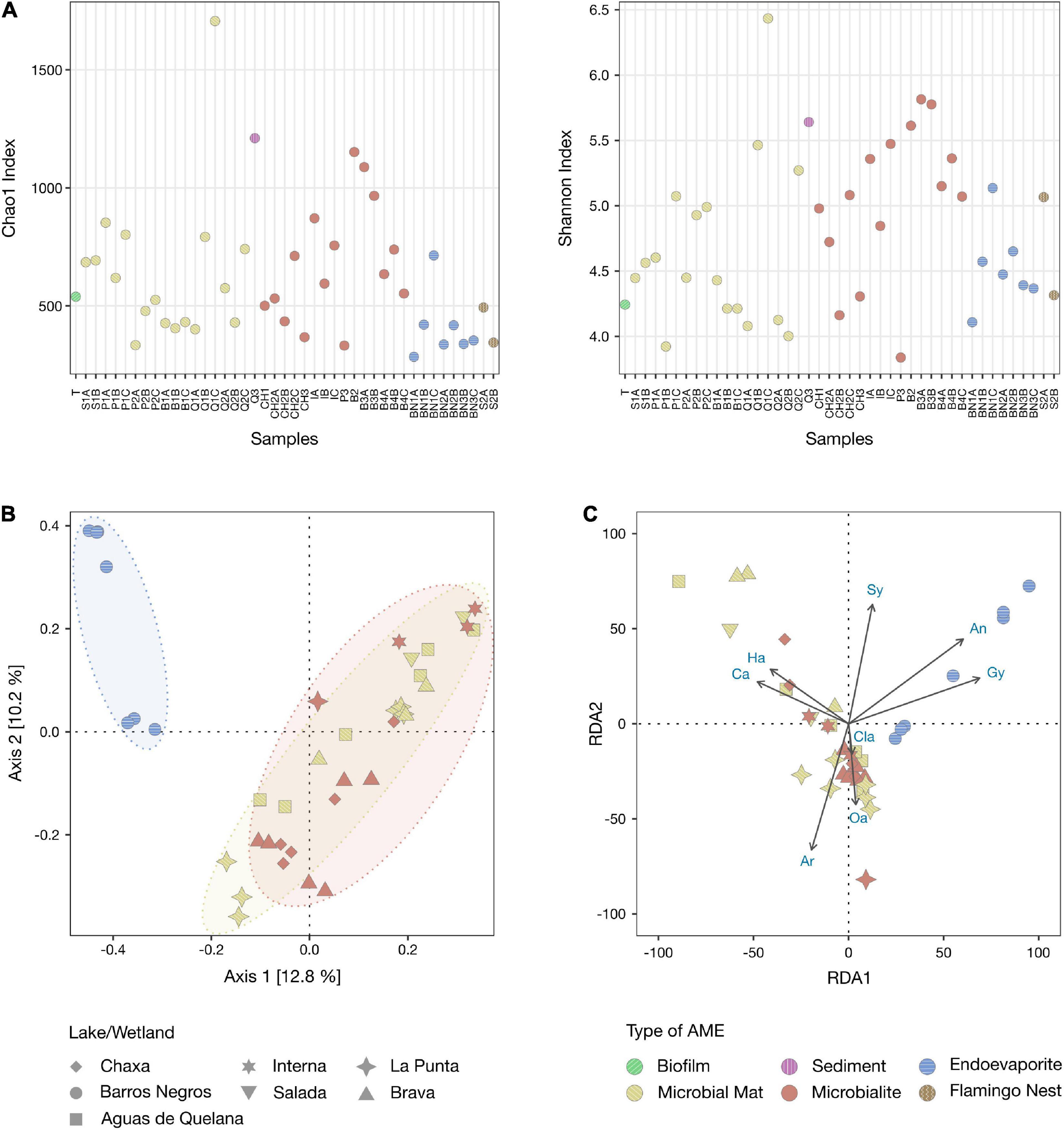
Figure 6. Diversity analyses of the AMEs. (A) Comparison of alpha diversity metrics between samples. Left, richness estimates (Chao1 index) for the different samples. Right, diversity estimates (Shannon index) for the different samples. (B) Principal coordinate analysis (PCoA) based on Bray-Curtis dissimilarity between endoevaporite, microbial mat, and microbialite samples. (C) Redundancy analysis (RDA) of the relationship between minerals and endoevaporite, microbial mat, and microbialite samples. Arrows indicate the direction and magnitude of minerals associated with the samples. Ha, Halite; Ca, Calcite; Ar, Aragonite; Oa, Opal-A; Cla, Clay; Gy, Gypsum; An, Anhydrite; Sy, Sylvite.
Beta diversity was assessed through a principal coordinate analysis (PCoA) based on Bray-Curtis dissimilarity between endoevaporite, microbial mat, and microbialite samples (Figure 6B). Floating biofilm, sediment and Andean flamingo mound nest samples were not included as random factors as they do not present replicates. The PCoA plot showed a clear separation of the samples based on the type of AME (PERMANOVA, R2 = 0.152, P < 0.001) and the site (PERMANOVA, R2 = 0.335, P < 0.001) (Supplementary Table 3), with the largest variation observed between gypsum endoevaporites, and carbonate mats and microbialites (Axis 1, Figure 6B). Therefore, a redundancy analysis (RDA) was carried out to evaluate further the relationship between the mineralogy and the community composition of the samples (Figure 6C). The RDA plot confirmed what was observed in the PCoA analysis. Gypsum (PERMANOVA, P < 0.001) and anhydrite (PERMANOVA, P < 0.01) compositions are significantly correlated with the community composition of the endoevaporites, while calcite (PERMANOVA, P < 0.001) and aragonite (PERMANOVA, P < 0.001) compositions are significantly correlated with the community composition of the mats and microbialites (Supplementary Table 4).
Discussion
The exploration of high-altitude lakes and wetlands in the middle-east and south-east regions of Salar de Atacama revealed a great number of previously undescribed AMEs, including floating biofilms, sediment-associated microbial communities, microbial mats, microbialites, endoevaporites and endolithic microbial communities inhabiting Andean flamingo mound nests. Nevertheless, not all types of AMEs were found in every prospected environment. Floating biofilms were described in Tilopozo; sediment-associated microbial communities were reported in Laguna Salada; microbial mats were found in Aguas de Quelana, Laguna Salada, Laguna La Punta and Laguna Brava; microbialites were observed in Laguna Chaxa, Laguna Interna, Laguna La Punta and Laguna Brava; endoevaporites were detected in Laguna Barros Negros; and endolithic microbial communities inhabiting Andean flamingo mound nests were discovered in Laguna Salada. The presence of different types of AMEs in the lakes and wetlands studied could be partially explained by their distinct environmental and geochemical conditions (Stivaletta et al., 2011; Gomez et al., 2014; Suosaari et al., 2016; Bougeault et al., 2020; Vignale et al., 2021). Among these AMEs, the endolithic microbial community inhabiting the Andean flamingo mound nest could be considered one of the most interesting findings. Thus far, endoevaporites were the only microbial endolithic communities reported in the Central Andes environments (Vignale et al., 2021). Therefore, this discovery opens the possibility of finding new types of microbial endolithic communities in the salt flats of the region. Endolithic microorganisms are often the main form of life in the most extreme terrestrial climates, and thus could provide important clues about ancient life on the Earth or elsewhere in the Solar System (Walker et al., 2005).
The XRD analyses revealed diverse mineralogical compositions in the AMEs studied. Microbial mats and microbialites found in Laguna Chaxa, Aguas de Quelana, Laguna Interna, Laguna Salada, Laguna La Punta and Laguna Brava are mainly formed by halite and calcium carbonate, like the majority of the mats and microbialites previously described in Salar de Atacama (Thiel et al., 2010; Farías et al., 2014; Farias et al., 2017; Farías, 2020). However, in all the microbialites, calcium carbonate is mostly present as aragonite. While, in some microbial mats, calcium carbonate is present both as aragonite and calcite. There are several factors that could favor the precipitation of calcium carbonate as calcite or as aragonite. The presence of sulfate (SO42–) or magnesium (Mg2+) in solution (Berner, 1975; Walter, 1986), as well as a high rate of carbonate precipitation (Given and Wilkinson, 1985), could favor precipitation of aragonite. Whereas, the presence of phosphate (PO43–) or iron (Fe2+) in solution (Meyer, 1984; Walter, 1986) could favor precipitation of calcite. As we do not have measurements of these parameters, we cannot be certain which factor favors the precipitation of calcium carbonate as calcite or as aragonite in the microbial mats and microbialites from this study. Therefore, water geochemical analyses should be carried out in the future to assess this interrogation. Contrary to microbial mats and microbialites, the endoevaporites from Laguna Barros Negros are mainly formed by gypsum and halite, like the previously studied endoevaporites from Laguna Tebenquiche (Farías et al., 2014; Fernandez et al., 2016). This mineral composition is also observed in the Andean flamingo mound nest from Laguna Salada, which could be explained by the fact that Andean flamingos build their mound nests with mud from the salt flat (Brown and King, 2005).
The SEM images of the microbial mats and microbialites studied revealed filamentous cyanobacteria and other prokaryotic cells (cocci, bacilli, and spirochetes) associated with diatom frustules forming nano-globular carbonate aggregates. Diatoms and cyanobacteria fix carbon dioxide and release oxygen through photosynthesis, which increases alkalinity and promotes carbonate precipitation (Dupraz and Visscher, 2005; Dupraz et al., 2009). The large amounts of EPS produced by them also influence carbonate precipitation, as the EPS matrix provides a template for carbonate nucleation (Dupraz and Visscher, 2005; Dupraz et al., 2009). Therefore, the formation of these nano-globular carbonate aggregates might suggest their participation in the mineral precipitation process (Gomez et al., 2018). Although diatoms and cyanobacteria seem to be key structural components in all the AMEs studied, a lower number of them were observed in the endoevaporites than in the microbial mats and microbialites. Microorganisms that colonize gypsum evaporites extract water from the gypsum crystals to withstand desiccation (Huang et al., 2020). However, under severe xeric conditions, this mechanism might not be enough for the development of diatoms and cyanobacteria, which could explain their lower abundance in the evaporite deposits. This hypothesis is supported by the lower abundance of cyanobacteria in the endoevaporite BN3 (ca. 0.24–0.28%), which is rich in anhydrite, than in the endoevaporites BN1 and BN2 (ca. 5.72–14.36% and 0.35–11.57%, respectively). The growth of diatoms in the gypsum evaporites could also be affected by a low silicon concentration in these systems (Martin-Jezequel et al., 2000). Most genera of diatoms identified in this work (Navicula, Halamphora, Nitzschia, Achnanthes, Surirella, and Mastogloia) have also been reported in other AMEs from the Central Andes (Farías et al., 2013, 2014; Rasuk et al., 2014; Albarracín et al., 2015; Gomez et al., 2018), which suggests a wide distribution of these taxa in the Central Andes environments.
Regarding the prokaryotic composition, differences have been observed between gypsum endoevaporites and carbonate mats and microbialites. Gypsum endoevaporites are dominated by Proteobacteria, Bacteroidetes, and Euryarchaeota phyla. The high halotolerance of Bacteroidetes and Euryarchaeota species could explain their great abundance in this type of AME (Farías et al., 2014). On the contrary, Firmicutes, Spirochetes, Verrucomicrobia, Chloroflexi, and Deinococcus-Thermus phyla are underrepresented. Probably, the moisture conditions of the evaporite deposits are not suitable for the correct growth of these microorganisms. Interestingly, a similar taxonomic composition has been observed in other gypsum endoevaporites from Salar de Atacama (Farías et al., 2014; Fernandez et al., 2016), which suggests a correlation between the microbiota and the mineralogy. Carbonate mats and microbialites are dominated by Bacteroidetes and Proteobacteria phyla, followed by Cyanobacteria, Chloroflexi, Planctomycetes, and Verrucomicrobia phyla. Oxygenic and anoxygenic phototrophs from Cyanobacteria, Chloroflexi, and Proteobacteria phyla, as well as sulfate reducers from Deltaproteobacteria class, increase alkalinity and promote carbonate precipitation, which could explain their great abundance in these types of AMEs (Dupraz et al., 2009). Other carbonate-rich mats and microbialites from Salar de Atacama (Farías et al., 2014; Fernandez et al., 2016) present a similar taxonomic composition, which further suggests a correlation between the microbiota and the mineralogy. Due to the previous observations, we performed a RDA analysis evaluated with a PERMANOVA test, and confirmed that gypsum, anhydrite, calcite and aragonite compositions are significantly correlated with the community composition of the samples. In the future, this could help us better understand the role of prokaryotes in the formation of the AMEs (Dupraz and Visscher, 2005; Dupraz et al., 2009).
The taxonomy-based analyses also revealed that certain microbial groups are arranged in a vertically organized structure in the microbial mats. This structure is normally determined by steep and fluctuating gradients of light, oxygen, hydrogen sulfide and pH, among other physicochemical gradients (Dupraz and Visscher, 2005; Dupraz et al., 2009). Bacteroidetes, Firmicutes, and Deinococcus-Thermus phyla are more abundant in the upper layers, probably due to the high resistance of their species to desiccation and/or ionizing radiation (Makarova et al., 2001; Rainey et al., 2005; Galperin, 2013; Yu et al., 2015). Species of the Cyanobacteria phylum are generally found in great proportion in the intermediate layers, a few millimeters beneath the surface, where they are protected from the harsh conditions of the environment to perform oxygenic photosynthesis (Stal, 1995). Whereas species of the Chloroflexi, Deltaproteobacteria, Planctomycetes, Spirochetes, and Calditrichaeota taxa are more abundant in the lower layers, which are normally anoxic. This could be partially explained by the metabolic activities carried out by them. Chloroflexi species consume fermentation products and perform anoxygenic photosynthesis (Lee et al., 2014), Deltaproteobacteria and Planctomycetes species grow anaerobically reducing sulfate and oxidizing ammonium, respectively (Madigan et al., 2017), while Spirochetes and Calditrichaeota species degrade organic compounds under anoxic conditions (Marshall et al., 2017; Dong et al., 2018).
Conclusion
The exploration carried out in the middle-east and south-east regions of Salar de Atacama allowed us to identify twenty previously unknown AMEs. The analyses performed on these AMEs revealed that (1) their mineralogical composition consists mainly of sulfates and/or calcium carbonates, the latter mostly present as aragonite; (2) their prokaryotic composition is correlated with their mineralogy; and (3) most harbor numerous diatom frustules forming nano-globular carbonate aggregates with cyanobacteria and other prokaryotic cells, which suggests the participation of these microorganisms in the mineral precipitation process.
Nevertheless, to have a better comprehension of the AMEs in the Salar de Atacama, further analyses need to be performed to confirm the participation (directly or indirectly) of diatoms and other eukaryotes in the mineral precipitation process; determine the seasonal variations of the environmental factors and their effect on the prokaryotic composition; and elucidate the metabolic potential of their microorganisms in terms of resistance to environmental conditions.
This work has expanded our comprehension of the AMEs inhabiting the high-altitude lakes and wetlands from the Central Andes region and we hope it will provide a stimulus to further study and preserve these unique ecosystems.
Data Availability Statement
The datasets presented in this study can be found in online repositories. The names of the repository/repositories and accession number(s) can be found below: ENA, PRJEB44218.
Author Contributions
FV conducted the experiments for the project, analyzed data, and drafted the manuscript. DK and AT assisted with bioinformatic analysis. AL assisted with the interpretation of SEM images. DP assisted with mineralogy analysis. EC, NM-H, FN, and MC performed sampling. MF obtained funding for the original project idea, performed sampling and supervised the findings of this work. All authors assisted in the reviewing and editing of the manuscript.
Funding
This work was supported by the Company Albemarle Ltda Chile and the Scientific and Technological Research Fund from Argentina (FONCyT, PICT V 2015/3825). FV and AL are CONICET doctoral fellows.
Conflict of Interest
The authors declare that the research was conducted in the absence of any commercial or financial relationships that could be construed as a potential conflict of interest.
Publisher’s Note
All claims expressed in this article are solely those of the authors and do not necessarily represent those of their affiliated organizations, or those of the publisher, the editors and the reviewers. Any product that may be evaluated in this article, or claim that may be made by its manufacturer, is not guaranteed or endorsed by the publisher.
Supplementary Material
The Supplementary Material for this article can be found online at: https://www.frontiersin.org/articles/10.3389/fmicb.2021.762076/full#supplementary-material
References
Albarracín, V. H., Kurth, D., Ordoñez, O. F., Belfiore, C., Luccini, E., Salum, G. M., et al. (2015). High-up: a remote reservoir of microbial extremophiles in central Andean wetlands. Front. Microbiol. 6:1404. doi: 10.3389/fmicb.2015.01404
Alonso, H., and Risacher, F. (1996). Geoquímica del Salar de Atacama, parte 1: origen de los componentes y balance salino. Rev. Geol. Chile 23, 113–122.
Berner, R. A. (1975). The role of magnesium in the crystal growth of calcite and aragonite from sea water. Geochim. Cosmochim. Acta 39, 489–504. doi: 10.1016/0016-7037(75)90102-7
Bolyen, E., Rideout, J. R., Dillon, M. R., Bokulich, N. A., Abnet, C., Al-Ghalith, G. A., et al. (2018). QIIME 2: reproducible, interactive, scalable, and extensible microbiome data science. PeerJ [Preprint]. doi: 10.7287/peerj.preprints.27295v2
Bougeault, C., Durlet, C., Vennin, E., Muller, E., Ader, M., Ghaleb, B., et al. (2020). Variability of carbonate isotope signatures in a hydrothermally influenced system: insights from the Pastos Grandes Caldera (Bolivia). Minerals 10:989. doi: 10.3390/min10110989
Brown, C., and King, C. (2005). Flamingo Husbandry Guidelines; A Joint Effort of the AZA and EAZA in Cooperation with WWT. Dallas: Dallas Zoo.
Cabrol, N. A., McKay, C. P., Grin, E. A., Kiss, K. T., Ács, É, Tóth, B., et al. (2009). “Signatures of habitats and life in Earth’s high-altitude lakes: clues to Noachian aqueous environments on Mars,” in The Geology of Mars: Evidence From Earth-based Analogs Cambridge Planetary Science, ed. M. G. Chapman (Cambridge: Cambridge University Press), 460.
Caporaso, J. G., Kuczynski, J., Stombaugh, J., Bittinger, K., Bushman, F. D., Costello, E. K., et al. (2010). QIIME allows analysis of high-throughput community sequencing data. Nat. Methods 7, 335–336. doi: 10.1038/nmeth.f.303
Cristóbal, G., Blanco, S., and Bueno, G. (eds) (2020). Modern Trends in Diatom Identification. Cham: Springer.
Dong, X., Greening, C., Brüls, T., Conrad, R., Guo, K., Blaskowski, S., et al. (2018). Fermentative Spirochaetes mediate necromass recycling in anoxic hydrocarbon-contaminated habitats. ISME J. 12, 2039–2050. doi: 10.1038/s41396-018-0148-3
Dupraz, C., Reid, R. P., Braissant, O., Decho, A. W., Norman, R. S., and Visscher, P. T. (2009). Processes of carbonate precipitation in modern microbial mats. Earth-Sci. Rev. 96, 141–162. doi: 10.1016/j.earscirev.2008.10.005
Dupraz, C., and Visscher, P. T. (2005). Microbial lithification in marine stromatolites and hypersaline mats. Trends Microbiol. 13, 429–438. doi: 10.1016/j.tim.2005.07.008
Farías, M. E., Contreras, M., Rasuk, M. C., Kurth, D., Flores, M. R., Poiré, D. G., et al. (2014). Characterization of bacterial diversity associated with microbial mats, gypsum evaporites and carbonate microbialites in thalassic wetlands: tebenquiche and La Brava, Salar de Atacama, Chile. Extremophiles 18, 311–329. doi: 10.1007/s00792-013-0617-6
Farías, M. E. (ed.) (2020). Microbial Ecosystems in Central Andes Extreme Environments: Biofilms, Microbial Mats, Microbialites and Endoevaporites. Cham: Springer.
Farías, M. E., Rascovan, N., Toneatti, D. M., Albarracín, V. H., Flores, M. R., Poiré, D. G., et al. (2013). The discovery of stromatolites developing at 3570 m above sea level in a high-altitude volcanic Lake Socompa, Argentinean Andes. PLoS One 8:e53497. doi: 10.1371/journal.pone.0053497
Farias, M. E., Rasuk, M. C., Gallagher, K. L., Contreras, M., Kurth, D., Fernandez, A. B., et al. (2017). Prokaryotic diversity and biogeochemical characteristics of benthic microbial ecosystems at La Brava, a hypersaline lake at Salar de Atacama, Chile. PLoS One 12:e0186867. doi: 10.1371/journal.pone.0186867
Fernandez, A. B., Rasuk, M. C., Visscher, P. T., Contreras, M., Novoa, F., Poire, D. G., et al. (2016). Microbial diversity in sediment ecosystems (evaporites domes, microbial mats, and crusts) of hypersaline Laguna Tebenquiche, Salar de Atacama, Chile. Front. Microbiol. 7:1284. doi: 10.3389/fmicb.2016.01284
Galperin, M. Y. (2013). Genome diversity of spore-forming Firmicutes. Microbiol. Spectr. 1:2012. doi: 10.1128/microbiolspectrum.TBS-0015-2012
Given, R. K., and Wilkinson, B. H. (1985). Kinetic control of morphology, composition, and mineralogy of abiotic sedimentary carbonates. J. Sediment. Res. 55, 109–119.
Gomez, F. J., Kah, L. C., Bartley, J. K., and Astini, R. A. (2014). Microbialites in a high-altitude Andean lake: multiple controls on carbonate precipitation and lamina accretion. Palaios 29, 233–249. doi: 10.2110/palo.2013.049
Gomez, F. J., Mlewski, C., Boidi, F. J., Farías, M. E., and Gérard, E. (2018). Calcium carbonate precipitation in diatom-rich microbial mats: the Laguna Negra hypersaline lake, Catamarca, Argentina. J. Sediment. Res. 88, 727–742. doi: 10.2110/jsr.2018.37
Hamilton, N. E., and Ferry, M. (2018). ggtern: ternary Diagrams Using ggplot2. J. Stat. Softw. 87, 1–17. doi: 10.18637/jss.v087.c03
Huang, W., Ertekin, E., Wang, T., Cruz, L., Dailey, M., DiRuggiero, J., et al. (2020). Mechanism of water extraction from gypsum rock by desert colonizing microorganisms. Proc. Natl. Acad. Sci. U. S. A. 117, 10681–10687. doi: 10.1073/pnas.2001613117
Kurth, D., Elias, D., Rasuk, M. C., Contreras, M., and Farías, M. E. (2021). Carbon fixation and rhodopsin systems in microbial mats from hypersaline lakes Brava and Tebenquiche, Salar de Atacama, Chile. PLoS One 16:e0246656. doi: 10.1371/journal.pone.0246656
Lee, J. Z., Burow, L. C., Woebken, D., Everroad, R. C., Kubo, M. D., Spormann, A. M., et al. (2014). Fermentation couples Chloroflexi and sulfate-reducing bacteria to Cyanobacteria in hypersaline microbial mats. Front. Microbiol. 5:61. doi: 10.3389/fmicb.2014.00061
Logan, B. W., Rezak, R., and Ginsburg, R. N. (1964). Classification and environmental significance of algal stromatolites. J. Geol. 72, 68–83. doi: 10.1086/626965
Madigan, M. T., Bender, K. S., Buckley, D. H., Matthew Sattley, W., and Stahl, D. A. (2017). Brock Biology of Microorganisms, Global Edition. New York: Pearson Higher Ed.
Makarova, K. S., Aravind, L., Wolf, Y. I., Tatusov, R. L., Minton, K. W., Koonin, E. V., et al. (2001). Genome of the extremely radiation-resistant bacterium Deinococcus radiodurans viewed from the perspective of comparative genomics. Microbiol. Mol. Biol. Rev. 65, 44–79. doi: 10.1128/MMBR.65.1.44-79.2001
Marshall, I. P. G., Starnawski, P., Cupit, C., Fernández Cáceres, E., Ettema, T. J. G., Schramm, A., et al. (2017). The novel bacterial phylum Calditrichaeota is diverse, widespread and abundant in marine sediments and has the capacity to degrade detrital proteins. Environ. Microbiol. Rep. 9, 397–403. doi: 10.1111/1758-2229.12544
Martin-Jezequel, V., Hildebrand, M., and Brzezinski, M. A. (2000). Silicon metabolism in diatoms: implications for growth. J. Phycol. 36, 821–840. doi: 10.1046/j.1529-8817.2000.00019.x
McMurdie, P. J., and Holmes, S. (2013). phyloseq: an R package for reproducible interactive analysis and graphics of microbiome census data. PLoS One 8:e61217. doi: 10.1371/journal.pone.0061217
McSorley, R. (1997). Soil Inhabiting Nematodes, Phylum Nematoda. Florida: Institute of Food and Agriculture Sciences.
Meyer, H. J. (1984). The influence of impurities on the growth rate of calcite. J. Cryst. Growth 66, 639–646. doi: 10.1016/0022-0248(84)90164-7
Moore, D. M., and Reynolds, R. C. Jr. (1989). X-ray Diffraction and the Identification and Analysis of Clay Minerals. Oxford: Oxford University Press.
Oksanen, J., Blanchet, F. G., Kindt, R., Legendre, P., Minchin, P. R., O’hara, R. B., et al. (2013). Community Ecology Package. R package version 2.
Osman, J. R., Viedma, P., Mendoza, J., Fernandes, G., DuBow, M. S., and Cotoras, D. (2021). Prokaryotic diversity and biogeochemical characteristics of field living and laboratory cultured stromatolites from the hypersaline Laguna Interna, Salar de Atacama (Chile). Extremophiles 25, 327–342. doi: 10.1007/s00792-021-01232-1
Quast, C., Pruesse, E., Yilmaz, P., Gerken, J., Schweer, T., Yarza, P., et al. (2013). The SILVA ribosomal RNA gene database project: improved data processing and web-based tools. Nucleic Acids Res. 41, D590–D596. doi: 10.1093/nar/gks1219
Rainey, F. A., Ray, K., Ferreira, M., Gatz, B. Z., Nobre, M. F., Bagaley, D., et al. (2005). Extensive diversity of ionizing-radiation-resistant bacteria recovered from Sonoran Desert soil and description of nine new species of the genus Deinococcus obtained from a single soil sample. Appl. Environ. Microbiol. 71, 5225–5235. doi: 10.1128/AEM.71.9.5225-5235.2005
Rasuk, M. C., Fernández, A. B., Kurth, D., Contreras, M., Novoa, F., Poiré, D., et al. (2016). Bacterial diversity in microbial mats and sediments from the Atacama Desert. Microb. Ecol. 71, 44–56. doi: 10.1007/s00248-015-0649-9
Rasuk, M. C., Kurth, D., Flores, M. R., Contreras, M., Novoa, F., Poire, D., et al. (2014). Microbial characterization of microbial ecosystems associated to evaporites domes of gypsum in Salar de Llamara in Atacama desert. Microb. Ecol. 68, 483–494. doi: 10.1007/s00248-014-0431-4
Schultz, L. G. (1964). Quantitative Interpretation of Mineralogical Composition from X-ray and Chemical Data for the Pierre Shale. Virginia: USGS.
Stal, L. J. (1995). Physiological ecology of cyanobacteria in microbial mats and other communities. New Phytol. 131, 1–32. doi: 10.1111/j.1469-8137.1995.tb03051.x
Stivaletta, N., Barbieri, R., Cevenini, F., and López-García, P. (2011). Physicochemical conditions and microbial diversity associated with the evaporite deposits in the Laguna de la Piedra (Salar de Atacama, Chile). Geomicrobiol. J. 28, 83–95. doi: 10.1080/01490451003653102
Stolz, J. F. (2000). “Structure of microbial mats and biofilms,” in Microbial Sediments, eds R. E. Riding and S. M. Awramik (Berlin: Springer), 1–8.
Suosaari, E. P., Pamela Reid, R., Abreu Araujo, T. A., Playford, P. E., Holley, D. K., Mcnamara, K. J., et al. (2016). Environmental pressures influencing living stromatolites in Hamelin Pool, Shark Bay, Western Australia. Palaios 31, 483–496. doi: 10.2110/palo.2016.023
Thiel, V., Tank, M., Neulinger, S. C., Gehrmann, L., Dorador, C., and Imhoff, J. F. (2010). Unique communities of anoxygenic phototrophic bacteria in saline lakes of Salar de Atacama (Chile): evidence for a new phylogenetic lineage of phototrophic Gammaproteobacteria from pufLM gene analyses. FEMS Microbiol. Ecol. 74, 510–522. doi: 10.1111/j.1574-6941.2010.00966.x
Vignale, F. A., Lencina, A. I., Stepanenko, T. M., Soria, M. N., Saona, L. A., Kurth, D., et al. (2021). Lithifying and non-lithifying microbial ecosystems in the wetlands and salt flats of the central Andes. Microb. Ecol. doi: 10.1007/s00248-021-01725-8 [Epub Online ahead of print].
Walker, J. J., Spear, J. R., and Pace, N. R. (2005). Geobiology of a microbial endolithic community in the Yellowstone geothermal environment. Nature 434, 1011–1014. doi: 10.1038/nature03447
Walter, L. M. (1986). Relative Efficiency of Carbonate Dissolution and Precipitation During Diagenesis: A Progress Report on the Role of Solution Chemistry. Available Online at: http://archives.datapages.com/data/sepm_sp/SP38/Relative_Efficiency_of_Carbonate_Dissolution.pdf (accessed November 05, 2020)
Wilcoxon, F. (1945). Individual comparisons by ranking methods. Biometr. Bull. 1, 80–83. doi: 10.2307/3001968
Keywords: Andean lakes, microbial mats, microbialites, endoevaporites, extremophiles
Citation: Vignale FA, Kurth D, Lencina AI, Poiré DG, Chihuailaf E, Muñoz-Herrera NC, Novoa F, Contreras M, Turjanski AG and Farías ME (2021) Geobiology of Andean Microbial Ecosystems Discovered in Salar de Atacama, Chile. Front. Microbiol. 12:762076. doi: 10.3389/fmicb.2021.762076
Received: 20 August 2021; Accepted: 28 September 2021;
Published: 28 October 2021.
Edited by:
Claudia P. Saavedra, Andres Bello University, ChileReviewed by:
Aharon Oren, The Hebrew University of Jerusalem, IsraelFrancisco Remonsellez, Catholic University of the North, Chile
Copyright © 2021 Vignale, Kurth, Lencina, Poiré, Chihuailaf, Muñoz-Herrera, Novoa, Contreras, Turjanski and Farías. This is an open-access article distributed under the terms of the Creative Commons Attribution License (CC BY). The use, distribution or reproduction in other forums is permitted, provided the original author(s) and the copyright owner(s) are credited and that the original publication in this journal is cited, in accordance with accepted academic practice. No use, distribution or reproduction is permitted which does not comply with these terms.
*Correspondence: Federico A. Vignale, dmlnbmFsZWZlZGVAZ21haWwuY29t; María E. Farías, bWVmYXJpYXMyMDA5QGdtYWlsLmNvbQ==