- Biosystems Engineering Laboratory, Department of Chemical Engineering, Universidad de Santiago de Chile (USACH), Santiago, Chile
Biofilm formation within the process of bioleaching of copper sulfides is a relevant aspect of iron- and sulfur-oxidizing acidophilic microorganisms as it represents their lifestyle in the actual heap/dump mining industry. Here, we used biofilm flow cell chambers to establish laminar regimes and compare them with turbulent conditions to evaluate biofilm formation and mineralogic dynamics through QEMSCAN and SEM-EDS during bioleaching of primary copper sulfide minerals at 30°C. We found that laminar regimes triggered the buildup of biofilm using Leptospirillum spp. and Acidithiobacillus thiooxidans (inoculation ratio 3:1) at a cell concentration of 106 cells/g mineral on bornite (Cu5FeS4) but not for chalcopyrite (CuFeS2). Conversely, biofilm did not occur on any of the tested minerals under turbulent conditions. Inoculating the bacterial community with ferric iron (Fe3+) under shaking conditions resulted in rapid copper recovery from bornite, leaching 40% of the Cu content after 10 days of cultivation. The addition of ferrous iron (Fe2+) instead promoted Cu recovery of 30% at day 48, clearly delaying the leaching process. More efficiently, the biofilm-forming laminar regime almost doubled the leached copper amount (54%) after 32 days. In-depth inspection of the microbiologic dynamics showed that bacteria developing biofilm on the surface of bornite corresponded mainly to At. Thiooxidans, while Leptospirillum spp. were detected in planktonic form, highlighting the role of biofilm buildup as a means for the bioleaching of primary sulfides. We finally propose a mechanism for bornite bioleaching during biofilm formation where sulfur regeneration to sulfuric acid by the sulfur-oxidizing microorganisms is crucial to prevent iron precipitation for efficient copper recovery.
Introduction
One of the most important challenges that face the copper industry is the development of sustainable technologies to leach complex ores composed of mixed copper mineral species in low grades, with a prevalence of primary copper sulfides. The major component of these materials is usually chalcopyrite (CuFeS2), followed by bornite (Cu5FeS4) (Panda et al., 2015). To this end, bioleaching has emerged as an efficient technology to leach secondary sulfides under mesophilic conditions (ambient temperature) (Bustos et al., 1993) and primary sulfides mostly under thermophilic conditions above 60°C (Duarte et al., 1993) or at moderate thermophilic (45–50°C) in stirred tank bioreactors (Cancho et al., 2007; Hedrich et al., 2018). Recently, acidophilic bacterial consortia recovered 43% of copper from chalcopyrite using shake flasks at room temperature (25–30°C) (Ma et al., 2018). In another study utilizing a bioleaching column coupled with a fuel cell, the system yielded 244 mg L–1 of copper from 150 g of chalcopyrite at 30°C in 320 days (Huang et al., 2019).
In the case of bornite, studies have shown bioleaching activity under mesophilic conditions compared with abiotic controls (Jun et al., 2008; Bevilaqua et al., 2010). Besides, researchers have proposed a galvanic effect with chalcopyrite accelerating the oxidative dissolution of bornite in the presence of microorganisms (Wang et al., 2016). Inoculation of the moderate thermophilic Leptospirillum ferriphilum and Acidithiobacillus caldus in shaking flasks at 45°C enabled the conversion of bornite into several intermediates including covellite (CuS) and isocubanite (CuFe2S3) through the bioleaching process, with a final copper extraction of 70% (Hong et al., 2019).
Finally, several works have established the high bioleaching efficiency of thermophiles over chalcopyrite (Petersen and Dixon, 2002), but none of them uncovered the role of biofilm formation in this process. Recently, a study reported biofilm development over chalcopyrite under moderate thermophilic conditions, where L. ferriphilum was inoculated at a high concentration (above 108 cells/g mineral) in the presence of under-stoichiometric iron concentrations (Bellenberg et al., 2018). A few studies have specifically addressed mesophilic biofilm formation on chalcopyrite, with researchers postulating that high precipitation of jarosite and elemental sulfur restrict biofilm development (Lei et al., 2009), while others identified microcolonies of At. ferrooxidans and L. ferriphilum forming a monolayered biofilm under short contacting periods (Africa et al., 2010).
Here, we demonstrated that mesophilic inoculation of bacteria with iron- and sulfur-oxidizing capacity in a flow cell chamber enables the detailed study of biofilm development on bornite resembling heap/dump bioleaching conditions when assuming a homogeneous packed bed behavior, where liquid percolation is driven by gravity through the ore particle surface. The biofilm-promoting laminar regime improved the copper leaching rate compared with stirred conditions in the presence of ferrous iron (Fe2+) and highlighted the importance of biofilm formation and its microbial composition to explain the mode of action and lifestyle of acidophiles carrying iron and sulfur oxidization during bornite bioleaching for copper extraction.
Materials and Methods
Consortium and Culture Conditions
The acidophilic consortium used in this study was obtained by enrichment from ore samples from mining regions in Chile. This consortium was maintained in batch aerated bubble column reactors at 30°C in modified 9K mineral salt medium [990 mg/L of (NH4)2SO4, 145 mg/L of NaH2PO4⋅H2O, 52 mg/L of KH2PO4, 100 mg/L of MgSO4⋅7H2O, and 21 mg/L of CaCl2] (Bobadilla-Fazzini et al., 2011), adjusted to pH 1.6 and containing soluble iron (5 g/L Fe2+), elemental sulfur (5 g S0/L), and chalcopyrite concentrate (0.2/L) as energy sources. The microbial composition analyses were performed by specific q-PCR determination as previously described (Bobadilla-Fazzini et al., 2011) indicated 76% abundance of Leptospirillum spp. and 24% of Acidithiobacillus spp.
Mineral Samples
High-purity chalcopyrite (optical mineralogical characterization as 90.07% chalcopyrite, 0.10 covellite, 7.11% pyrite, and 2.72% unspecified gangue) and bornite (optical mineralogical characterization as 74.91% bornite, 15,89% Cc, 0.16% chalcopyrite, 0.79% digenite, 0.09% covellite, 0.35% rutile, 1.69% hematite, and 6.13% unspecified gangue) were obtained by manual picking from ore samples from the Geological Department of the Universidad de Chile. Samples were ground and sieved with 325 Tyler mesh, which means that all particles were below 0.044 mm.
Screening Bioleaching Assays
Every mineral sample was attached to a plastic tape to cover a surface of approximately 1.5 × 1.5 cm registering the exact mass of mineral. The plastic tape with the bound mineral was attached to the bottom of a six-well plate with the mineral facing upward. Turbulent flow of culture medium (8 ml per well) was carried out using modified 9K medium either including ferric (0.7 g Fe3+/L) or ferrous iron (0.7 g Fe2+/L) as sulfate salts and incubating the plate at 30°C and 150 rpm under non-sterile conditions and including mesophilic inoculation. The acidophilic consortium was added to the solution to reach an initial density of 106 cells/g mineral. For both conditions (with and without inoculation) three to five replicates were set up for every analyzed time. The solution obtained was directly sampled at the end of the assay without solid washing. Evaporation was prevented with a hermetic plastic cover on top of each six-well plate.
Flow Cell Bioleaching Assays
A set of custom-made flow cells with a lower inlet and an overflow chamber was done in acrylic, recirculating a volume of 100–200 ml of culture minimal medium (Bobadilla-Fazzini, 2017). Every mineral sample was attached to a plastic tape in order to cover a surface of approximately 1.5 × 1.5 cm registering the exact mass of the mineral. The plastic tape with the bound mineral was attached to a glass slide that was placed on the top of the flow cell with the mineral facing downward. Flow was set at a free-stream fluid velocity of 0.02 cm/s and pipe diameter of 0.3 cm, where a laminar flow was assured (Reynolds number below 10) of culture-modified 9K medium with ferrous iron (0.7 g Fe2+/L) with a peristaltic recirculation pump and avoiding turbulent flow placing a bubble trap between the pump and the flow cell inlet. Mesophilic inoculation was done adding the acidophilic consortium to the solution to reach an initial density of 106 cells/g of mineral just at the beginning of the incubation. All conditions were set up for every analyzed time in triplicate. All flow cells were incubated at 30°C under non-sterile conditions assuming solution saturation for oxygen and carbon dioxide. The recirculating solution was periodically sampled, and evaporation was compensated with pure water before each sampling time.
Biofilm and Microbial Analysis
Measurements were done directly on mineral samples and solutions stopping three flow cell replicates for every condition and analyzed time. Cell concentration in the recirculating solution was done by direct chamber counting under phase-contrast microscope (Thoma Chamber, depth 0.010 mm). Further strain proportion was determined by specific q-PCR determination as previously described (Bobadilla-Fazzini et al., 2011). Briefly, purified genomic DNA was extracted and analyzed with total bacteria 16S rRNA gene region primers (forward 5′-GTGCCAGCMGCCGCGGTAA-3′, reverse 5′-CCGTCAATTCCTTTGAGTTT-3′), total Archaea 16S rRNA gene region primers (forward 5′-ACGGGGCG CAGCAGGCGCGA-3′, reverse 5′-YCCGGCGTTGAMTCCAA TT-3′), 16S rRNA gene for Acidithiobacillus thiooxidans (forward 5′-TAATATCGCCTGCTGTTGAC-3′, reverse 5′-TTTCACGACAGACCTAATG-3) and 16S rRNA gene for Leptospirillum spp. (forward 5′-TGAGGGGACTGCCAGCGAC-3′, reverse 5′-CTAGACGGGTACCTTGTTAC-3′). Alternatively, for biofilm analysis, previously designed fluorescent in situ hybridization (FISH) method and probes were used for Leptospirillum spp. (FISH-FAM: 5′-CGCTTCCCTC TCCCAGCCT-3′) (Bond and Banfield, 2001) and Acidithiobacillus spp. (5′-ACCAAACATCTAGTATTCATCG-3′) (Peccia et al., 2000).
Chemical Analysis
Total iron and copper were determined by Atomic Absorption Spectrometry (Perkin Elmer Analyst 400), and Fe(II) by the o-phenantroline method (Kolthoff and Sandell, 1963).
Mineralogical Analysis
For every sample, one section was dried overnight for mineralogical analysis, and the other section taken for SEM-EDS analysis (FEI Quanta 250) after sputter coating with gold. Mineralogical analysis was performed directly over the dry mineral samples at different times by bulk mineralogical analysis (BMA) with QEMSCAN Express equipment (FEI). The analytic methodology chosen was a combination of bulk mineral analysis with linear intercept method and a high-density field scan analysis (2.5 μm × 2.5-μm pixel size) with a minimum of 200,000 pixel analysis/sample in order to obtain a general mineral overview and a detailed mineral association mapping.
Results and Discussion
Mesophilic bioleaching has been used at an industrial scale for secondary copper sulfide ores, but not for the primary ones like chalcopyrite, given the low copper recovery and slow kinetics (Córdoba et al., 2008). For low-grade primary copper sulfide ores, a few industrial bioleaching applications are in the advanced stages to make the copper extraction economically feasible, to some extent, because of our inability to capture the lifestyle and growth development of the leaching microorganisms during copper recovery from actual primary ores. Thus, we investigated the capabilities of Leptospirillum spp. and At. thiooxidans to form biofilm on primary copper sulfides utilizing a standardized and reproducible flow cell approach that resembles heap/dump bioleaching conditions. It is important to highlight that most studies today employed primary copper sulfide high-purity minerals with concentrates added at low pulp densities and high cell loads in shaking flasks (Corkhill et al., 2008; Wang et al., 2016; Bellenberg et al., 2018). These growth conditions are optimal for homogeneous mixing and planktonic microbial growth, but certainly not for biofilm development (Tolker-Nielsen and Sternberg, 2011). First, we set the screening assay under turbulent flow regimes to mimic shaking flask liquid homogeneous conditions (Figure 1A). On the other hand, the method for studying bacterial biofilm development uses cell flow chambers under laminar regimes (Tolker-Nielsen and Sternberg, 2011; Crusz et al., 2012). Figure 1B depicts the components that comprise the cell chambers to study metal-leaching acidophiles. We found that the bubble trap is essential for attaining a homogeneous flow and proper propagation of acidophilic microorganisms (Crusz et al., 2012). We next placed on top of the cell chamber, in various sets of experiments, high-purity natural primary copper sulfide minerals to evaluate biofilm formation and copper extraction and finally compare it with turbulent conditions. Importantly, the minerals were not cleansed by acid solutions (HCl) nor autoclaved since these procedures alter the surface of the material, provoking initial leaching of copper and eliminating native microorganisms inhabiting these primary copper sulfide materials (Cerda et al., 2018).
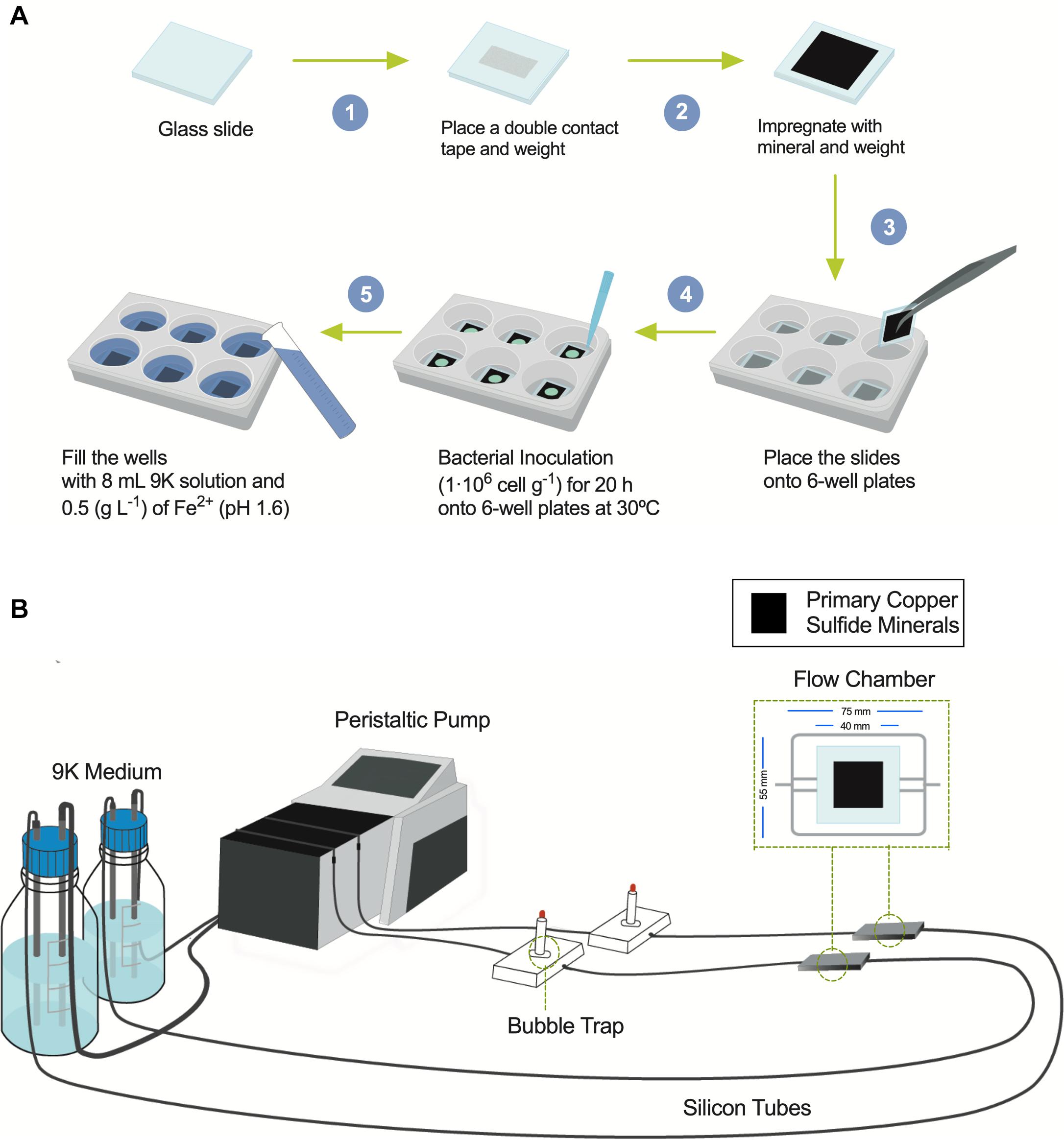
Figure 1. Diagrams showing the flow chamber for biofilm formation and the screening assay under shaking conditions. (A) Screening procedure scheme to bioleach copper from primary copper sulfides in mesophilic conditions (30°C) under shaking using six-well plates with inoculation of bacterial acidophiles. (B) Flow cell model and assembly diagram of bioleaching copper from primary copper sulfides under laminar regimes and buildup biofilm composed of acidophilic bacterial consortium growing at 30°C.
Chalcopyrite Bioleaching
Mineralogical analyses were done on the head mineral to confirm chalcopyrite purity (Figure 2A, time 0) and at the end of the bioleaching assay to record possible transformations (Figure 2A, 30 and 60 days). Chalcopyrite inspection before bioleaching by QEMSCAN-BMA indicated a purity of 70% (previously estimated over 90% by optical microscopy), with a significant 16% of unclassified minerals (Figure 2A), with a clean surface as observed by SEM (Figure 2C). Under shaking conditions, precipitates completely covered the chalcopyrite surface after 30 days of bioleaching, partially classified as potassium and/or sodium jarosite based on the EDS elemental identification (Figure 2D). This was not the case for chalcopyrite bioleached under laminar regimes as the micrographs show low precipitates, and EDS analysis remained constant (Figure 2E).
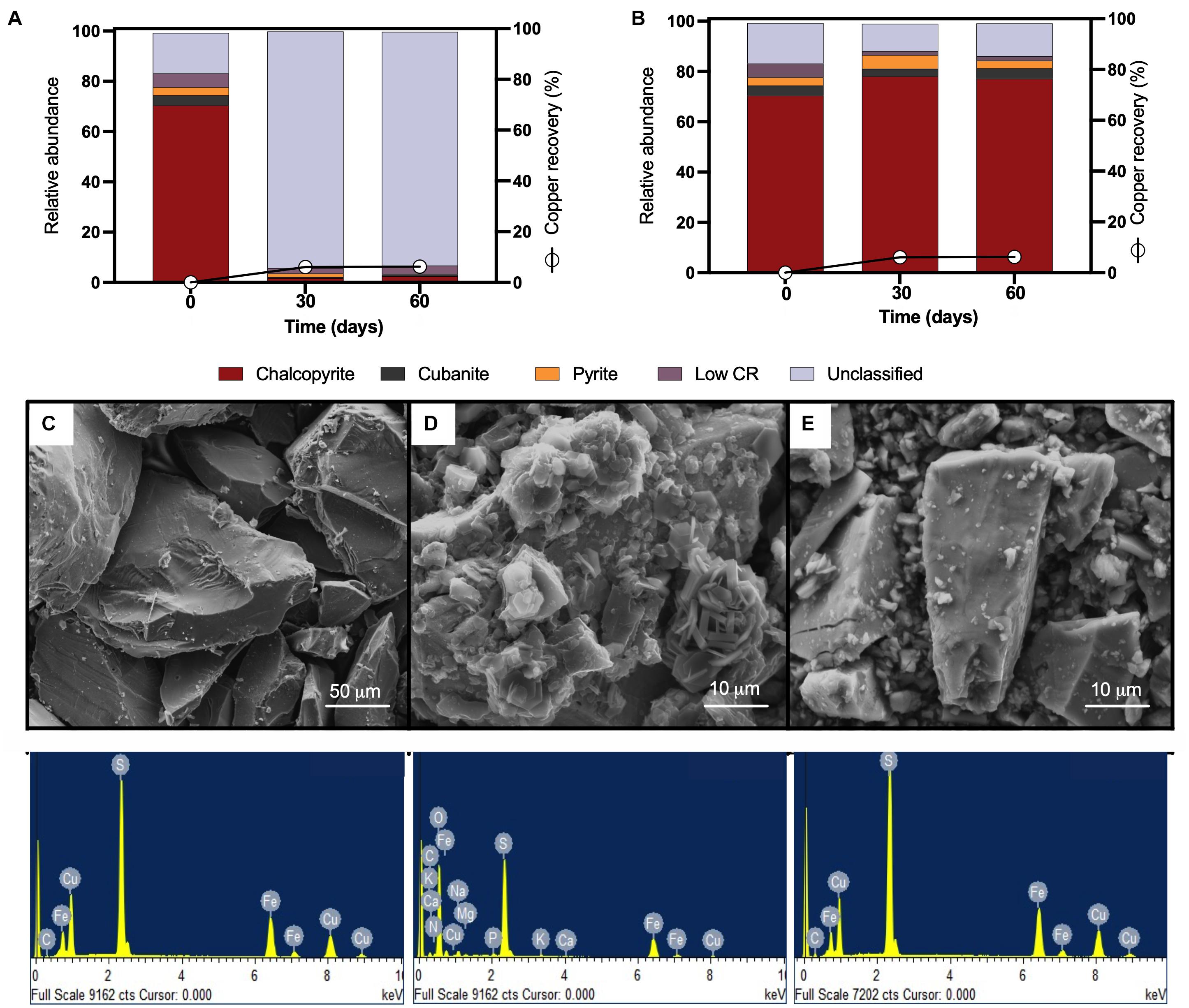
Figure 2. QEMSCAN-BMA analysis of high-purity natural chalcopyrite before bioleaching (t0) and after flow cell bioleaching assay (30 days) for (A) turbulent and (B) laminar conditions. (C) SEM image and EDS spectrum of high purity chalcopyrite before bioleaching. SEM images and EDS spectra of high-purity chalcopyrite after 30 days of (D) turbulent (screening method) and (E) laminar flow mesophilic bioleaching (flow cell method). Low CR: no mineral detection.
Using the laminar flow chamber, the final mineralogical analysis showed significant variations with an increment in chalcopyrite proportion to 78% and reduced unclassified material to 11%, probably indicating the removal of initially present impurities from the mineral surface. On the contrary, in the turbulent flow regime, the mineralogical analysis was almost unable to identify chalcopyrite at the end of the assay (only 2% identification at day 30), while the unclassified raised to 94% attributed mainly to not identified mixed phases (Figure 2A, 30 and 60 days), indicating major mineral surface coverage by formed precipitates including potassium and/or sodium jarosite as indicated by EDS (Figure 2D). The screening bioleaching assay and the laminar flow condition showed a low copper recovery of 6% in 30 days incubated at 30°C and maintaining this copper level until day 60 (Figures 2A,B). Biofilm formation did not occur under turbulent or laminar flow regimes (Figures 2D,E). These results are in agreement with those previously published by Lei et al. (2009). Some studies describe biofilm buildup on the altered surface of the chalcopyrite in a short period where chalcopyrite was either previously electro-oxidized resulting in S0, CuS, and S2– that promote bacterial attachment (García-Meza et al., 2013), or crushed down to a size of less than 80 μm and finally polished (Yang et al., 2015). Other works using batch stirred bioreactors set at 42°C and inoculated with L. ferriphilum, Sulfobacillus spp., and A. caldus showed 94% extraction of the available copper in the concentrate, where chalcopyrite remained intact during the leaching process (6% of the remaining Cu) (Spolaore et al., 2011; Hedrich et al., 2016). This confirms that even at moderate thermophilic conditions and employing a different acidophilic consortium from this study, it is challenging to leach the copper content from this primary sulfide mineral.
Bornite Bioleaching
Considering that chalcopyrite is commonly associated with bornite in nature and that a galvanic couple between these two minerals has been described (Wang et al., 2016), the next step was to analyze the mesophilic bioleaching process correlated with highly pure bornite. We first inoculated the same proportion of the acidophilic bacterial consortium used for chalcopyrite along with the addition of ferric iron (0.7 g Fe3+/L), which enabled the rapid recovery of copper from bornite (40%) after 10 days of cultivation under stirring conditions (Figure 3A). Previous studies showed accelerated bornite leaching in the presence of external ferric iron addition (Hidalgo et al., 2019), and on the contrary, the screening bioleaching assays carried out with the addition of ferrous iron (0.7 g Fe2+/L) exhibited a copper extraction close to 30% after 48 days at 30°C (Figure 3B). In parallel, the laminar regime leached 54% of the Cu content at day 32, with no further Cu recovery until day 48 (Figure 3C), illustrating the importance of the process set up and bacterial attachment to accelerate copper bioleaching from bornite at 30°C.
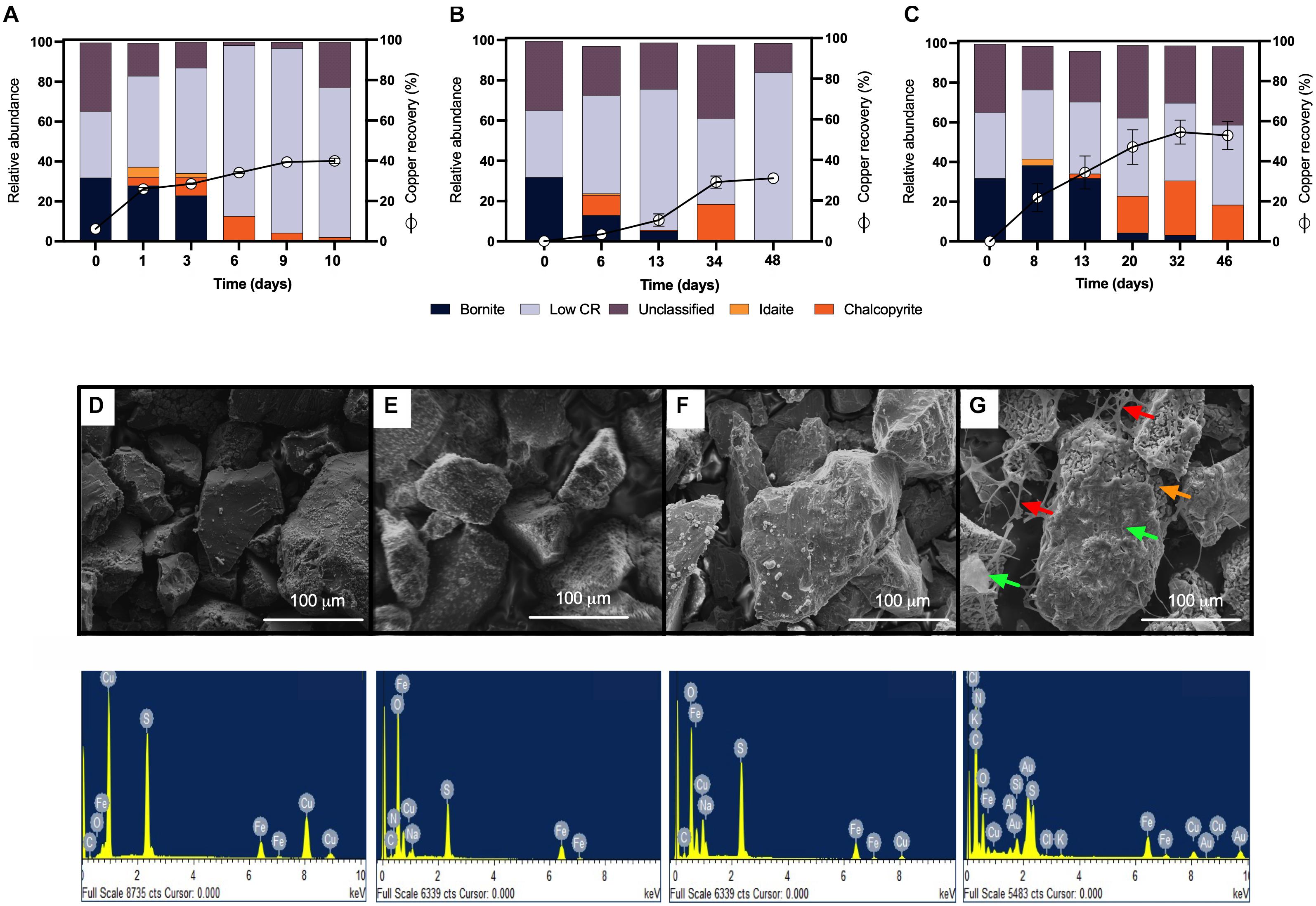
Figure 3. Mineralogical dynamics, SEM images, and EDS spectra of high-purity natural bornite bioleaching under mesophilic (30°C) inoculation. (A) Turbulent flow bioleaching with addition of ferric iron (screening method), (B) turbulent flow bioleaching with addition of ferrous iron (screening method), (C) laminar flow bioleaching (flow cell method). SEM images and EDS spectra for (D) bornite before bioleaching, (E) bornite after 10 days (40% Cu recovery) under turbulent flow mesophilic bioleaching (screening method), (F) bornite after 48 days (30% Cu recovery) under turbulent flow mesophilic bioleaching with addition of ferrous iron (screening method), (G) bornite after 46 days (54% Cu recovery) under laminar flow mesophilic bioleaching (flow cell method). Red, orange, and green arrows indicate exopolysaccharide (EPS) network, altered mineral, biofilm formation, respectively. Low CR: no mineral detection.
QEMSCAN-BMA recorded 32% bornite purity before bioleaching (estimated at 75% by optical microscopy) with an unclassified mineral fraction close to a third (time 0 in Figures 3A–C). The mineralogical dynamics of mesophilic bioleaching indicated in both regimens (turbulent and laminar flow) the intermediate formation of idaite (Cu3FeS4) in the first stage of bioleaching. Chalcopyrite detection was attributed to ferric ion precipitation masking further bornite/idaite detection (Figures 3A–C), though former studies consider it as part of the bornite (bio)leaching mechanism (Pal-ing, 2018). Turbulent and laminar flow regimes were initially inoculated including external ferrous iron (Fe2+) addition; in the turbulent regime, only a slight iron-oxidizing activity was observed until day 20 (Figure 4A) with a concomitant microbial growth, stopped by a significant pH increase (pH 4.25, data not shown) and consequent iron precipitation (Figure 4A). Interestingly, biofilm formation was observed only under laminar flow conditions, with a significant alteration in the bornite surface and an exopolysaccharide (EPS) network (Figure 3G, indicated with red arrows), compared with turbulent flow conditions where no biofilm formation was encountered with the initial addition of ferric nor ferrous iron (Figure 3F). Biofilm development mediated initially by EPS production during bioleaching depends on several factors including mineral type (Gehrke et al., 1998), process temperature (Zeng et al., 2010; Barahona et al., 2014), and the presence of organic compounds such as D-galactose (Saavedra et al., 2020) and sodium glucoronate (Bellenberg et al., 2015). For instance, pyrite is an excellent sulfide mineral for biofilm formation under acidic conditions as a strong electrochemical interaction occurs between the synthesized EPS-Fe3+ and the negatively charged mineral surface (Gehrke et al., 1998; Bellenberg et al., 2015). It differs highly compared with chalcopyrite, where EPS synthesis occurs only when inoculated at a high cell density (Lei et al., 2009; Yang et al., 2015; Bellenberg et al., 2018), with some studies using more than 109 cells/g mineral as initial biomass (Lei et al., 2009). Unfortunately, this biomass loading is impractical at the industrial mining scale for cooper bioleaching of primary sulfur-bearing ores. It is clear that further investigation aiming at promoting biofilm development on chalcopyrite at mesophilic conditions is necessary under different flow regimes, varying the type and abundance of acidophilic consortia, resembling industrial operations, and taking care of the mineral characteristics, initial cell density, and avoiding major mineral pretreatments to make the process economically feasible.
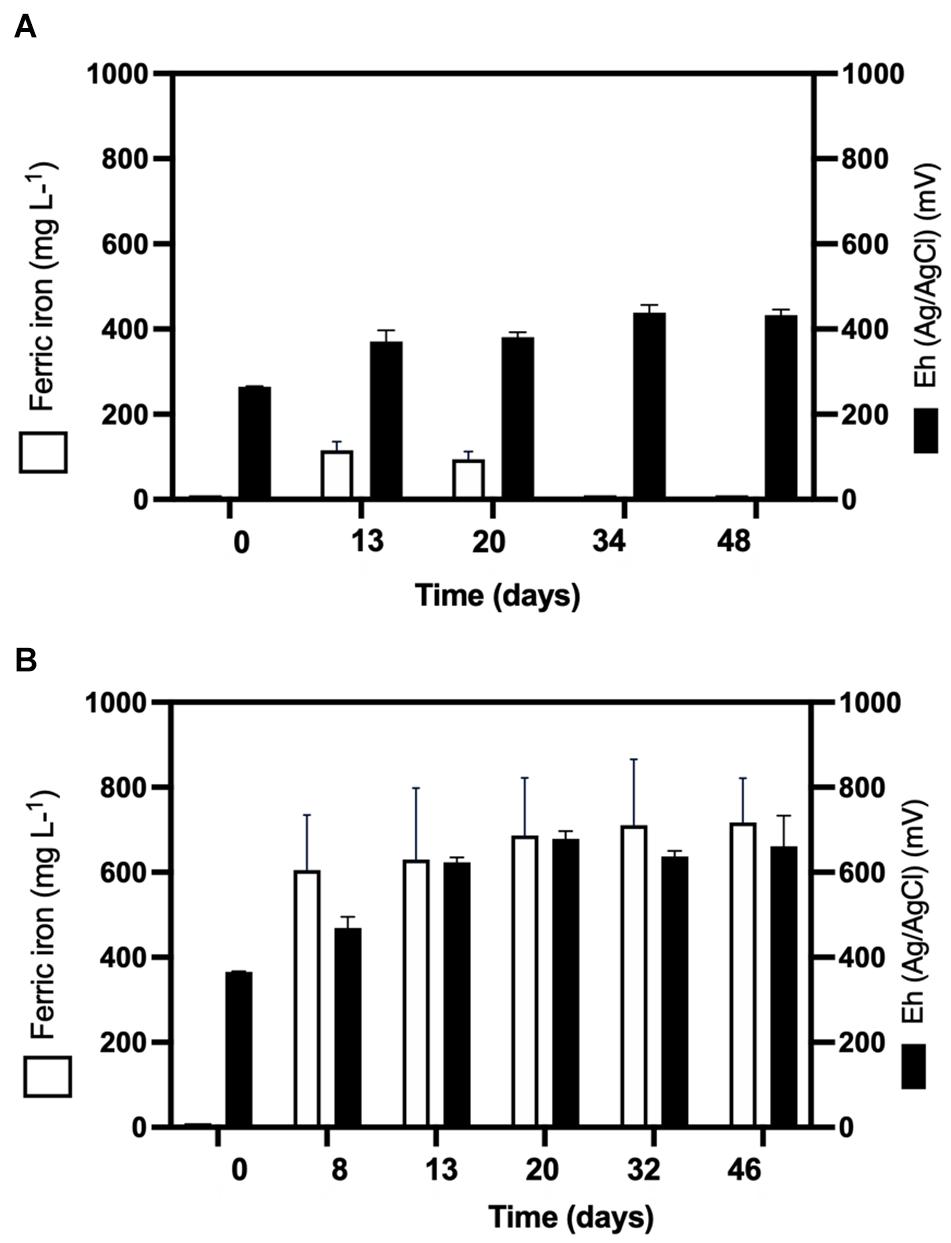
Figure 4. Ferric ion (Fe3+) formation and oxidation–reduction potential (Eh) during (A) turbulent flow bioleaching with addition of ferrous iron (700 mg/L) (screening method) and (B) laminar flow mesophilic bioleaching with addition of ferrous iron (700 mg/L) (flow cell method).
Microbial Analysis During Bornite Bioleaching
Considering the extensive copper recovery from bornite under the laminar flow regime, which was not seen here for the turbulent condition in the presence of ferrous iron (Fe2+), we next performed a dynamic microbial analysis during biofilm formation. Initial inoculation of the acidophilic consortium in a proportion 3:1 (Leptospirillum spp.:At. thiooxidans) was followed under the laminar flow condition, revealing the predominance of the iron oxidizers in solution until the end of the assay and the appearance of unidentified (possibly mineral native bacteria) in planktonic form (Figure 5A). After day 20, the iron present in the solution was detected in the ferric form (Figure 4B), correlating with the increment in the total iron-oxidizing bacteria in the recirculating solution (Figure 4B). However, FISH analysis indicated the prevalence of Acidithiobacillus spp. in the biofilm (Figure 5B), opening the discussion concerning the interaction and relevance of both planktonic and sessile microbial populations. On the one hand, the planktonic iron oxidizers recycle the ferric ion that actively (bio)leach the mineral surface, a phenomenon initially favored under turbulent conditions (Hong et al., 2019) based on the screening copper recovery kinetics observed (Figure 3). However, the lack of biofilm formation with the presence of microbes with sulfur-oxidizing activity did not generate the required acid production to balance its consumption during the microbial iron-oxidation process (Figure 4A), avoiding further copper extraction from bornite (Figure 3B). Clearly, this is not representative of the industrial heap/dump copper mining operations, where the kinetics of microbial iron and sulfur oxidation occur at a different pace. In this sense, the laminar flow biofilm analysis shows that the attached microbial population is mainly composed of sulfur-oxidizing bacterial species (Figure 5B), most likely due to mineral surface elemental sulfur accumulation (Lei et al., 2009; Bobadilla-Fazzini, 2017). This phenomenon can be considered useful in terms of initial mineral surface exposure to the ferric leaching agent, though detrimental due to extensive biofilm growth and mineral surface hindering at a later process stage, perhaps requiring specific conditions to tailor microbial composition for efficient copper extraction.
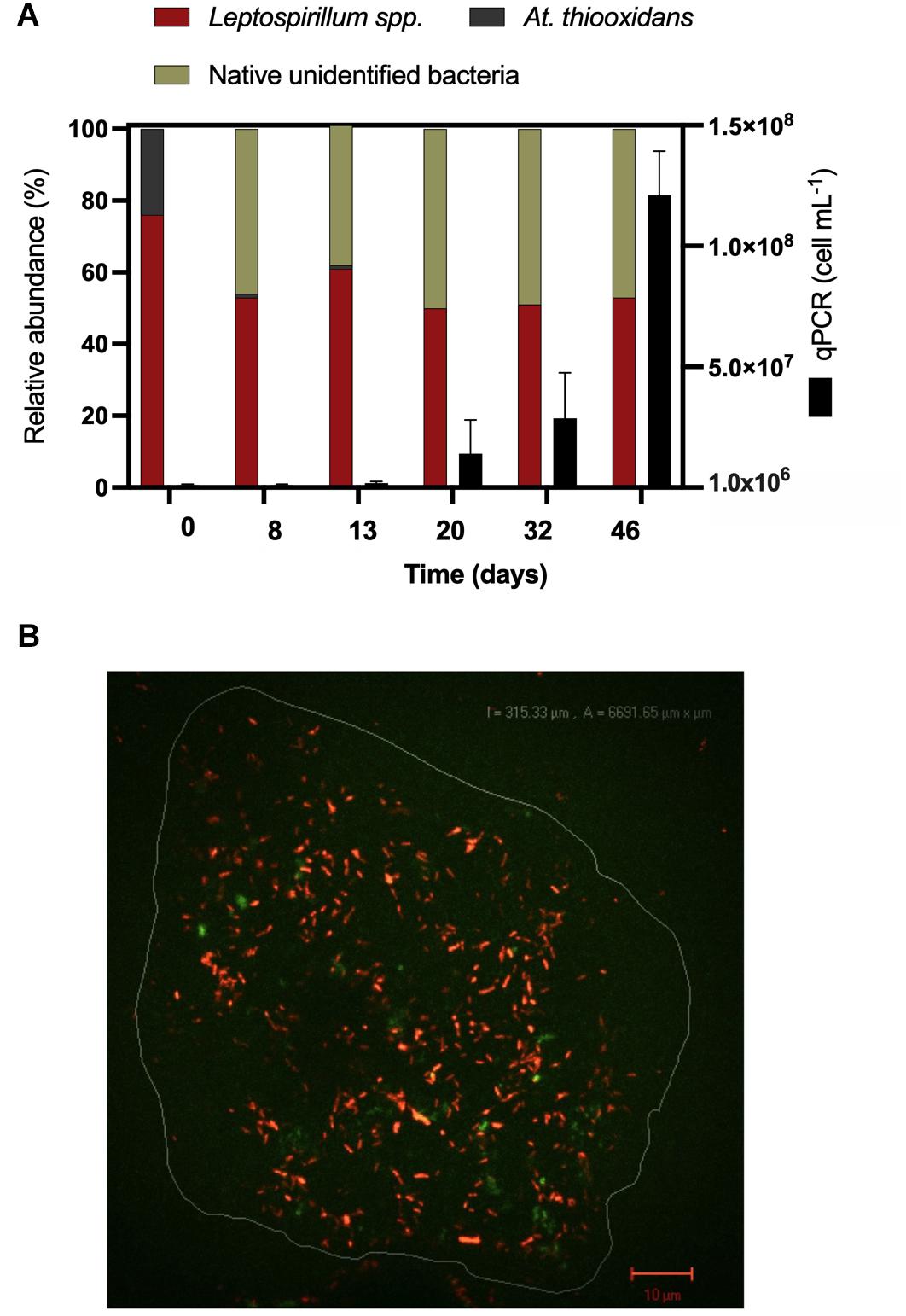
Figure 5. Microbiological determinations during high-purity natural bornite bioleaching under mesophilic (30°C) inoculation with external ferrous iron (Fe2+) addition in laminar flow bioleaching (flow cell method). (A) Microbial dynamics in the recirculating solution via qPCR and cell counts. (B) Representative biofilm composition by FISH (30 days) where the image corresponds to overlap of FISH-Cy3 (red, Acidithiobacillus spp.) and FISH-FAM (green, Leptospirillum spp.).
EDS analyses confirmed the expected bornite atomic composition before bioleaching (Figure 3D), and the altered bornite surface with the impoverishment of copper during bioleaching (Figure 3F), validating the identification of idaite by QEMSCAN. Moreover, EDS analysis showed that during the turbulent flow screening method, the precipitates formed over the surface of bornite appears to be sodium jarosite [Na Fe3(OH)6(SO4)2] due to notable increments in oxygen and sodium (Figure 3E), as recently reported for ferric sulfate pretreatment followed by moderate thermophilic bioleaching (Liu et al., 2020). On the other hand, the surface EDS analysis of laminar flow cell bornite showed no sodium at all but high carbon proportions indicating the organic nature of the biofilm formed (Figure 3G).
Mechanism for Bornite Bioleaching During Biofilm Formation
Combining the biofilm development, ferric profile, and the mineralogical QEMSCAN and EDS analyses, it is possible to propose a mechanism for bornite bioleaching under laminar flow conditions (Figure 6). Previous studies have shown two stages for bornite ferric leaching (Pesic and Olson, 1984), with an initial leaching rate significantly dependent on ferric ion concentration and with no elemental sulfur formation leading to idaite:
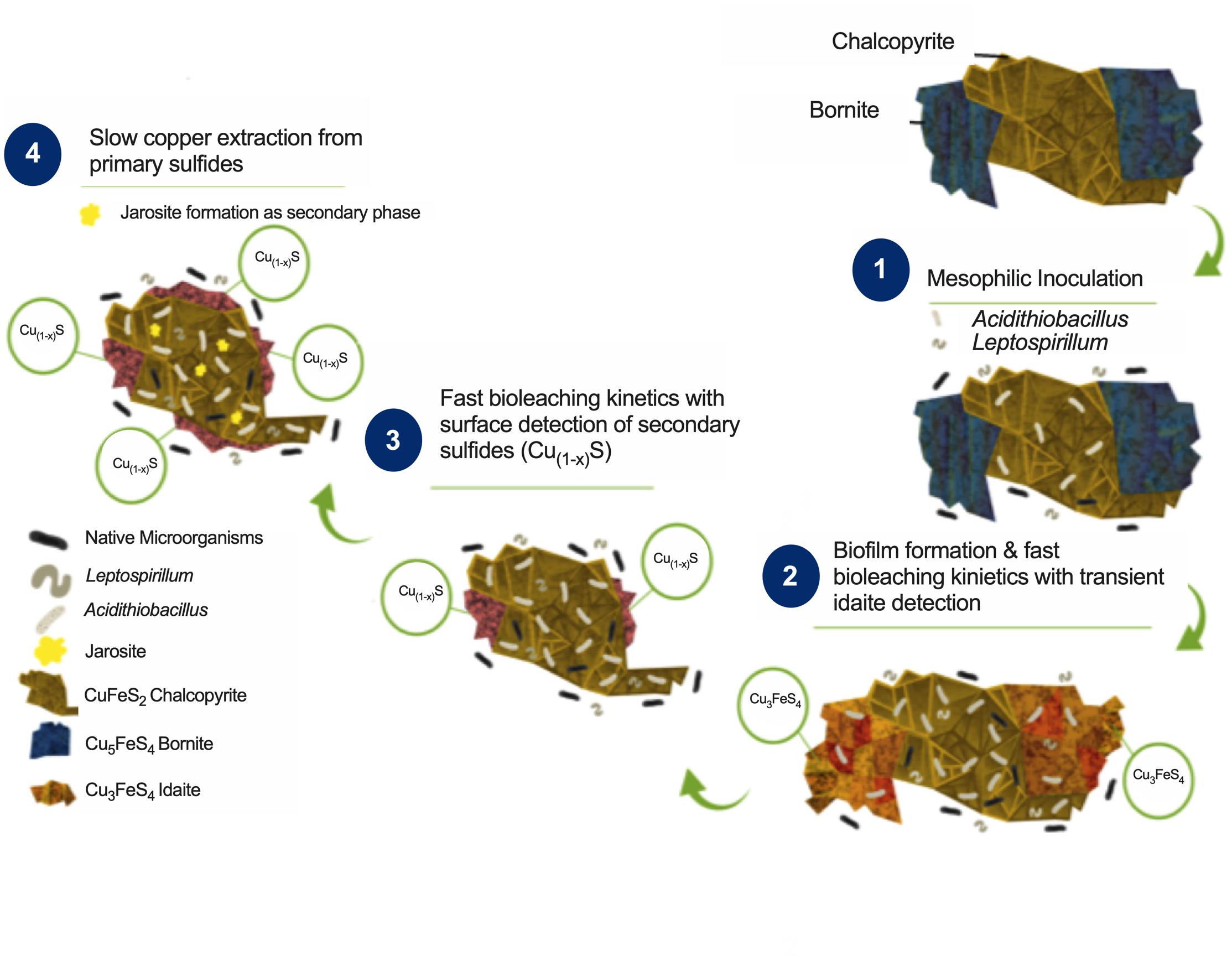
Figure 6. Proposed bioleaching mechanism for primary copper sulfides bornite and chalcopyrite under mesophilic inoculation of bacteria with iron- and sulfur-oxidizing activity forming biofilm.
Indeed, the ferrous iron oxidation is mediated by Leptospirillum spp. yielding the ferric form:
This first stage is observed in our analysis from the beginning of the process until days 34 and 13 for turbulent and laminar flow with ferrous iron addition, respectively, with a corresponding copper extraction close to 30% (Figure 3). In the second stage, a slow leaching rate of idaite arises independent of ferric ion concentration (Figure 3C) where the sulfur produced is proportional to copper dissolved and tending to envelop the residue mineral particles, therefore, being the limiting step:
Here, the iron- and sulfur-oxidizing microorganisms exert a catalyzing role, with the pH maintenance as a critical aspect in order to prevent ferric ion precipitation (Figure 4B), a role exerted by the sulfur-oxidizing biofilm controlling the leaching kinetics (Figure 5B):
These results confirm that mesophilic biofilm occurs extensively over bornite under laminar flow regimes, modifying its surface in a very different way than ferric leaching does under turbulent flow conditions (Hong et al., 2019; Liu et al., 2020). In addition, as the buildup of biofilm comprises several stages, initiating with the bacterial adherence to the surface, then the cells aggregate entering a phase of irreversible attachment, maturation, and finally dispersion (Garnett and Matthews, 2012). This process could explain the significantly higher copper recovery levels compared with shaking conditions (Figures 3B,C).
More precipitates are observed under turbulent conditions masking the proper quantification of the resulting iron sulfide compounds (Figure 3). Overall, the flow cell chamber is a more appropriated approach to studying biofilm formation and mineralogic dynamics that resemble the heap bioleaching of this abundant primary copper sulfide.
Conclusion
The main copper minerals worldwide correspond to primary sulfides chalcopyrite and bornite, so it is crucial to understand the microbial variations affecting their efficient copper extraction. In this sense, this work shows that the standard approaches of bioleaching tests under stirring conditions prevent biofilm formation on bornite and chalcopyrite at 30°C. In addition, we determine that biofilm development is not feasible on chalcopyrite even under laminar flow mesophilic conditions, posing a great challenge for copper hydrometallurgy. Successfully, the biofilm-forming laminar regime improves almost twice the leached copper content of bornite compared with stirring conditions in the presence of ferrous iron (Fe2+), reducing the time from 48 to 32 days of cultivation. For the first time, we demonstrated that the main microorganisms comprising the biofilm development on bornite are sulfur-oxidizing bacteria, while the ferrous-oxidizing bacteria are in planktonic form. This lifestyle of acidophiles explains the accelerated pace of copper recovery during biofilm formation, where the action of sulfur-oxidizing bacteria maintains the low pH via sulfuric acid formation, thus, preventing iron precipitation. The next step toward sustainable copper hydrometallurgy is to develop a process that promotes biofilm formation on the surface of chalcopyrite under mesophilic or moderate thermophilic conditions to extract copper from this bulk material efficiently, pointing to the creation of novel acidophilic microbial consortia.
Data Availability Statement
The raw data supporting the conclusions of this article will be made available by the authors, without undue reservation.
Author Contributions
RB-F performed experiments, analyzed the data, and wrote the manuscript. IP-C analyzed the data and wrote the manuscript. Both authors contributed to the article and approved the submitted version.
Funding
This work was supported in part by ANID-PIA-Anillo INACH ACT192057 and by DICYT-USACH.
Conflict of Interest
The authors declare that the research was conducted in the absence of any commercial or financial relationships that could be construed as a potential conflict of interest.
Publisher’s Note
All claims expressed in this article are solely those of the authors and do not necessarily represent those of their affiliated organizations, or those of the publisher, the editors and the reviewers. Any product that may be evaluated in this article, or claim that may be made by its manufacturer, is not guaranteed or endorsed by the publisher.
References
Africa, C.-J., Harrison, S. T. L., Becker, M., and van Hille, R. P. (2010). In situ investigation and visualisation of microbial attachment and colonisation in a heap bioleach environment: the novel biofilm reactor. Miner. Eng. 23, 486–491. doi: 10.1016/j.mineng.2009.12.011
Barahona, S., Dorador, C., Zhang, R., Aguilar, P., Sand, W., Vera, M., et al. (2014). Isolation and characterization of a novel Acidithiobacillus ferrivorans strain from the Chilean Altiplano: attachment and biofilm formation on pyrite at low temperature. Res. Microbiol. 165, 782–793. doi: 10.1016/j.resmic.2014.07.015
Bellenberg, S., Barthen, R., Boretska, M., Zhang, R., Sand, W., and Vera, M. (2015). Manipulation of pyrite colonization and leaching by iron-oxidizing Acidithiobacillus species. Appl. Microbiol. Biotechnol. 99, 1435–1449. doi: 10.1007/s00253-014-6180-y
Bellenberg, S., Buetti-Dinh, A., Galli, V., Ilie, O., Herold, M., Christel, S., et al. (2018). Automated microscopic analysis of metal sulfide colonization by acidophilic microorganisms. Appl. Environ. Microbiol. 84:e01835-18. doi: 10.1128/AEM.01835-18
Bevilaqua, D., Garcia, O. Jr., and Tuovinen, O. H. (2010). Oxidative dissolution of bornite by Acidithiobacillus ferrooxidans. Process Biochem. 45, 101–106. doi: 10.1016/j.procbio.2009.08.013
Bobadilla-Fazzini, R. A. (2017). Mineralogical dynamics of primary copper sulfides mediated by acidophilic biofilm formation. Solid State Phenomena 262, 325–329. doi: 10.4028/www.scientific.net/SSP.262.325
Bobadilla-Fazzini, R. A., Levican, G., and Parada, P. (2011). Acidithiobacillus thiooxidans secretome containing a newly described lipoprotein Licanantase enhances chalcopyrite bioleaching rate. Appl. Microbiol. Biotechnol. 89, 771–780. doi: 10.1007/s00253-010-3063-8
Bond, P. L., and Banfield, J. F. (2001). Design and performance of rRNA targeted oligonucleotide probes for in situ detection and phylogenetic identification of microorganisms inhabiting acid mine drainage environments. Microb. Ecol. 41, 149–161. doi: 10.1007/s002480000063
Bustos, S., Castro, S., and Montealegre, R. (1993). The Sociedad Minera Pudahuel bacterial thin-layer leaching process at Lo Aguirre. FEMS Microbiol. Rev. 11, 231–235. doi: 10.1111/j.1574-6976.1993.tb00289.x
Cancho, L., Blázquez, M. L., Ballester, A., González, F., and Muñoz, J. A. (2007). Bioleaching of a chalcopyrite concentrate with moderate thermophilic microorganisms in a continuous reactor system. Hydrometallurgy 87, 100–111. doi: 10.1016/j.hydromet.2007.02.007
Cerda, C. P., Taboada, M. E., Jamett, N. E., Ghorbani, Y., and Hernández, P. C. (2018). Effect of pretreatment on leaching primary copper sulfide in acid-chloride media. Minerals 8:1. doi: 10.3390/min8010001
Córdoba, E. M., Muñoz, J. A., Blázquez, M. L., González, F., and Ballester, A. (2008). Leaching of chalcopyrite with ferric ion. Part IV: the role of redox potential in the presence of mesophilic and thermophilic bacteria. Hydrometallurgy 93, 106–115. doi: 10.1016/j.hydromet.2007.11.005
Corkhill, C. L., Wincott, P. L., Lloyd, J. R., and Vaughan, D. J. (2008). The oxidative dissolution of arsenopyrite (FeAsS) and enargite (Cu3AsS4) by Leptospirillum ferrooxidans. Geochim. Cosmochim. Acta 72, 5616–5633. doi: 10.1016/j.gca.2008.09.008
Crusz, S. A., Popat, R., Rybtke, M. T., Cámara, M., Givskov, M., Tolker-Nielsen, T., et al. (2012). Bursting the bubble on bacterial biofilms: a flow cell methodology. Biofouling 28, 835–842. doi: 10.1080/08927014.2012.716044
Duarte, J. C., Estrada, P. C., Pereira, P. C., and Beaumont, H. P. (1993). Thermophilic vs. mesophilic bioleaching process performance. FEMS Microbiol. Rev. 11, 97–102. doi: 10.1111/j.1574-6976.1993.tb00272.x
García-Meza, J. V., Fernández, J. J., Lara, R. H., and González, I. (2013). Changes in biofilm structure during the colonization of chalcopyrite by Acidithiobacillus thiooxidans. Appl. Microbiol. Biotechnol. 97, 6065–6075. doi: 10.1007/s00253-012-4420-6
Garnett, J. A., and Matthews, S. (2012). Interactions in bacterial biofilm development: a structural perspective. Curr. Protein Pept. Sci. 13, 739–755. doi: 10.2174/138920312804871166
Gehrke, T., Telegdi, J., Thierry, D., and Sand, W. (1998). Importance of extracellular polymeric substances from Thiobacillus ferrooxidans for bioleaching. Appl. Environ. Microbiol. 64, 2743–2747. doi: 10.1128/AEM.64.7.2743-2747.1998
Hedrich, S., Guézennec, A.-G., Charron, M., Schippers, A., and Joulian, C. (2016). Quantitative monitoring of microbial species during bioleaching of a copper concentrate. Front. Microbiol. 7:2044. doi: 10.3389/fmicb.2016.02044
Hedrich, S., Joulian, C., Graupner, T., Schippers, A., and Guézennec, A.-G. (2018). Enhanced chalcopyrite dissolution in stirred tank reactors by temperature increase during bioleaching. Hydrometallurgy 179, 125–131. doi: 10.1016/j.hydromet.2018.05.018
Hidalgo, T., Kuhar, L., Beinlich, A., and Putnis, A. (2019). Kinetics and mineralogical analysis of copper dissolution from a bornite/chalcopyrite composite sample in ferric-chloride and methanesulfonic-acid solutions. Hydrometallurgy 188, 140–156. doi: 10.1016/j.hydromet.2019.06.009
Hong, M., Wang, X., Wu, L., Fang, C., Huang, X., Liao, R., et al. (2019). Intermediates transformation of bornite bioleaching by Leptospirillum ferriphilum and Acidithiobacillus caldus. Minerals 9:159. doi: 10.3390/min9030159
Huang, T., Wei, X., and Zhang, S. (2019). Bioleaching of copper sulfide minerals assisted by microbial fuel cells. Bioresour. Technol. 288:121561. doi: 10.1016/j.biortech.2019.121561
Jun, W., Qin, W.-Q., Zhang, Y.-S., Yang, C.-R., Zhang, J.-W., Nai, S.-S., et al. (2008). Bacterial leaching of chalcopyrite and bornite with native bioleaching microorganism. Trans. Nonferrous Met. Soc. China 18, 1468–1472. doi: 10.1016/S1003-6326(09)60027-3
Kolthoff, J. M., and Sandell, E. B. (1963). Textbook of Quantitative Inorganic Chemistry. New York, NY: Macmillan.
Lei, J., Huaiyang, Z., Xiaotong, P., and Zhonghao, D. (2009). The use of microscopy techniques to analyze microbial biofilm of the bio-oxidized chalcopyrite surface. Miner. Eng. 22, 37–42. doi: 10.1016/j.mineng.2008.03.012
Liu, S., Hong, M., Wang, X., Yang, B., Lin, H., Lin, M., et al. (2020). Pretreatment with acidic ferric sulfate leaching promotes the bioleaching of bornite. Hydrometallurgy 196:105349. doi: 10.1016/j.hydromet.2020.105349
Ma, L., Wang, X., Liu, X., Wang, S., and Wang, H. (2018). Intensified bioleaching of chalcopyrite by communities with enriched ferrous or sulfur oxidizers. Bioresour. Technol. 268, 415–423. doi: 10.1016/j.biortech.2018.08.019
Pal-ing, K. J. (2018). The Mechanism of Bornite Leaching: Insights From Mineralogical and Textural Characterisation. Ph.D thesis. Perth: Murdoch University.
Panda, S., Akcil, A., Pradhan, N., and Deveci, H. (2015). Current scenario of chalcopyrite bioleaching: a review on the recent advances to its heap-leach technology. Bioresour. Technol. 196, 694–706. doi: 10.1016/j.biortech.2015.08.064
Peccia, J., Marchand, E. A., Silverstein, J., and Hernandez, M. (2000). Development and application of small-subunit rRNA probes for assessment of selected Thiobacillus species and members of the genus Acidiphilium. Appl. Environ. Microbiol. 66, 3065–3072. doi: 10.1128/AEM.66.7.3065-3072.2000
Pesic, B., and Olson, F. A. (1984). Dissolution of bornite in sulfuric acid using oxygen as oxidant. Hydrometallurgy 12, 195–215. doi: 10.1016/0304-386X(84)90034-3
Petersen, J., and Dixon, D. G. (2002). Thermophilic heap leaching of a chalcopyrite concentrate. Miner. Eng. 15, 777–785. doi: 10.1016/S0892-6875(02)00092-4
Saavedra, A., Aguirre, P., and Gentina, J. C. (2020). Biooxidation of iron by Acidithiobacillus ferrooxidans in the presence of D-galactose: understanding its influence on the production of EPS and cell tolerance to high concentrations of iron. Front. Microbiol. 11:759. doi: 10.3389/fmicb.2020.00759
Spolaore, P., Joulian, C., Gouin, J., Morin, D., and d’Hugues, P. (2011). Relationship between bioleaching performance, bacterial community structure and mineralogy in the bioleaching of a copper concentrate in stirred-tank reactors. Appl. Microbiol. Biotechnol. 89, 441–448. doi: 10.1007/s00253-010-2888-5
Tolker-Nielsen, T., and Sternberg, C. (2011). Growing and analyzing biofilms in flow chambers. Curr. Protoc. Microbiol. 21:1B–2., doi: 10.1002/9780471729259.mc01b02s21
Wang, J., Tao, L., Zhao, H., Hu, M., Zheng, X., Peng, H., et al. (2016). Cooperative effect of chalcopyrite and bornite interactions during bioleaching by mixed moderately thermophilic culture. Miner. Eng. 95, 116–123. doi: 10.1016/j.mineng.2016.06.006
Yang, Y., Tan, S. N., Glenn, A. M., Harmer, S., Bhargava, S., and Chen, M. (2015). A direct observation of bacterial coverage and biofilm formation by Acidithiobacillus ferrooxidans on chalcopyrite and pyrite surfaces. Biofouling 31, 575–586. doi: 10.1080/08927014.2015.1073720
Keywords: Leptospirillum spp., Acidithiobacillus thiooxidans, biofilm, chalcopyrite, bornite
Citation: Bobadilla-Fazzini RA and Poblete-Castro I (2021) Biofilm Formation Is Crucial for Efficient Copper Bioleaching From Bornite Under Mesophilic Conditions: Unveiling the Lifestyle and Catalytic Role of Sulfur-Oxidizing Bacteria. Front. Microbiol. 12:761997. doi: 10.3389/fmicb.2021.761997
Received: 20 August 2021; Accepted: 27 September 2021;
Published: 22 October 2021.
Edited by:
Davide Zannoni, University of Bologna, ItalyReviewed by:
Raymond J. Turner, University of Calgary, CanadaOlli Heikki Tuovinen, The Ohio State University, United States
Copyright © 2021 Bobadilla-Fazzini and Poblete-Castro. This is an open-access article distributed under the terms of the Creative Commons Attribution License (CC BY). The use, distribution or reproduction in other forums is permitted, provided the original author(s) and the copyright owner(s) are credited and that the original publication in this journal is cited, in accordance with accepted academic practice. No use, distribution or reproduction is permitted which does not comply with these terms.
*Correspondence: Ignacio Poblete-Castro, aWduYWNpby5wb2JsZXRlLmNAdXNhY2guY2w=