- PHIM Plant Health Institute, Université Montpellier, IRD, CIRAD, INRAE, Institut Agro, Montpellier, France
Burkholderia sensu lato species are prominent for their diversity of hosts. The type 3 secretion system (T3SS) is a major mechanism impacting the interactions between bacteria and eukaryotic hosts. Besides the human pathogenic species Burkholderia pseudomallei and closely affiliated species, the T3SS has received little attention in this genus as in taxonomically and evolutionary close genera Paraburkholderia, Caballeronia, Trinickia, and Mycetohabitans. We proceeded to identify and characterize the diversity of T3SS types using the genomic data from a subset of 145 strains representative of the species diversity found in the Burkholderia s.l. group. Through an analysis of their phylogenetic distribution, we identified two new T3SS types with an atypical chromosomal organization and which we propose to name BCI (Burkholderia cepacia complex Injectisome) and PSI (Paraburkholderia Short Injectisome). BCI is the dominant T3SS type found in Burkholderia sensu stricto (s.s.) species and PSI is mostly restricted to the Paraburkholderia genus. By correlating their distribution with the ecology of their strains of origin, we propose a role in plant interaction for these T3SS types. Experimentally, we demonstrated that a BCI deficient B. vietnamiensis LMG10929 mutant was strongly affected in its rice colonization capacity.
Importance
The type 3 secretion system (T3SS) is a major driver of the interaction between bacteria and their hosts. Bacterial species of the Burkholderia genus sensu lato (s.l.) are prone to engage with a diversity of hosts yet little is known about the underlying mechanisms driving these interactions. The current interest in these bacteria is significant as they include human and plant pathogens but also symbiotic nitrogen fixing bacteria (i.e., rhizobia) and plant growth promoting species. The T3SS of Burkholderia s.l. have rarely been investigated theoretically or experimentally. Here we undertook a characterization of the T3SS landscape using genomic sequence data of 145 representative strains of the species diversity described in Burkholderia s.l. We identified unexpected T3SS distribution patterns and uncovered the existence of two new T3SS families. Through an experimental approach, we established a role in plant-interaction for one of the novel T3SS family.
Introduction
The type three secretion system (T3SS) or injectisome, is a specialized and complex macromolecular structure which is embedded in the membranes of Gram-negative bacteria and acts as a protein delivery machine for intra-cellular secretion into eukaryotic cells. T3SS are relatively rare among plant beneficial strains and endophytes and it is generally considered as a marker of pathogenicity (Reinhold-Hurek and Hurek, 2011; Angus et al., 2014). On these grounds, the T3SS was mainly investigated in human, animal, and plant pathogen models including species of Yersinia, Salmonella, Shigella, Vibrio, Chlamydia, Pseudomonas, Erwinia, Xanthomonas, and Ralstonia (Coburn et al., 2007). Indeed, the T3SS has proven to be a promising target for therapy as a number of severe pathogens are depending on it for cell invasion and manipulation as well as long term survival inside their host (Deng et al., 2017). Still, the T3SS sparked much interest for its role in rhizobium symbioses as it is involved in a Nod-factor independent symbiotic signalization pathway between the bacteria and the legume host (Okazaki et al., 2016; Teulet et al., 2019). Nod-factor dependent symbionts also frequently possess a T3SS, which influences their host-range (Skorpil et al., 2005; Jiménez-Guerrero et al., 2015).
Species of the Burkholderia sensu lato (s.l.) group (i.e., Burkholderia, Caballeronia, Mycetohabitans, Paraburkholderia, and Trinickia genera) are especially diverse regarding interactions with eukaryotic hosts. Indeed, they have been described in close associations with mammals, insects, plants, fungi and amoebae among others, with varying outcomes depending on the bacterial strain and the host involved. Species that are closely related at the genetic level can have radically different impacts on their host, either displaying pathogenic or mutualistic behavior or even engaging in highly specialized nitrogen-fixing symbiosis with legumes (Compant et al., 2008). Both beneficial and virulent strains have been described to possess injectisome-coding genes (Holden et al., 2009; Lackner et al., 2011a; Vander Broek and Stevens, 2017; Dias et al., 2019).
The T3SS coding regulon is clustered within a ca. 22–50 kb region and encompasses more than 20 genes involved in the machinery’s assembly. Despite being highly conserved across genera, the components were assigned unique names depending on the studied bacteria, making comparisons confusing. We will use the widely adopted secretion and cellular translocation (Sct) nomenclature throughout this study (Hueck, 1998). The core structure of the T3SS machinery is composed of sixteen different proteins among which thirteen are highly conserved across all T3SS types i.e., SctC, SctD, SctJ, SctK, SctL, SctN, SctO, SctQ, SctR, SctS, SctT, SctU, SctV. Additionally, the sct cluster can englobe genes coding for secreted effector proteins, transcription regulators and chaperones that maintain the secreted proteins folded until they pass the injection rod. The main phenotypical difference between T3SS used for plant invasion (i.e., Hrp-1, Hrp-2, and Rhc) and those used for animal infection (i.e., SPI-1, SPI-2, Ysc, and Cds) lies in their sheath structure. Animal pathogens have an extracellular extension that resembles a short needle of 50–80 nm (Kubori et al., 1998). Plant pathogens have to get through the plant cell wall and thus have a significantly longer extracellular filament termed pilus, reaching a size of several micrometers (Roine et al., 1997).
All T3SS have a common evolutionary origin with the bacterial flagellum. The diversity of injectisomes was broadened recently and 13 different types were recognized with several additional sub-categories (Abby and Rocha, 2012; Hu et al., 2017). In Burkholderia s.l. species, most attention was brought to the melioidosis-causing human pathogen B. pseudomallei and the closely related but avirulent B. thailandensis. B. pseudomallei is known to harbor three different T3SS operons in its genome and notably one from the Inv/Mxi-Spa (SPI-1) family. Indeed, this secretion system was described as being fundamental for host invasion and vacuolar escape and several SPI-1 secreted effectors were identified as responsible for these abilities (Stevens et al., 2002, 2003; Burtnick et al., 2008; Bast et al., 2014). The SPI-1 of B. pseudomallei displays homology with the secretion system of other human pathogens such as Salmonella typhimurium and Shigella flexneri (Attree and Attree, 2001). Beyond the SPI-1, B. pseudomallei bears two further injectisomes which are specialized for plant interaction and both belong to the Hrp-2 family (Lee et al., 2010). While the Hrp-2 contribute to disease development on tomato plants, they are not adapted for the infection of rice plants (Manivanh et al., 2017). B. pseudomallei is mainly found in paddy fields but has indeed never been observed to cause symptoms on rice plants.
This is unlike B. glumae and B. gladioli, two specialized rice pathogens causing various symptoms from seedling rot to panicle blight (Ura et al., 2006; Zhou-qi et al., 2016). Both bear a single T3SS of the Hrp-2 type. In B. glumae, the T3SS is efficient at translocating effectors to plant cells (Sharma et al., 2013). Still, many effectors under control of the central Hrp-2 regulator hrpB are secreted by a T2SS (Kang et al., 2008). The T3SS of B. gladioli has yet to be investigated for its implication in rice pathogenesis. It is however involved in mycophagy, which is mediated by the injection of a prophage-tail like effector by the T3SS of B. gladioli (Swain et al., 2017). Injectisomes have only scarcely been characterized in Paraburkholderia species. The Hrp-2 injectisome of Paraburkholderia terrae is involved in the attachment to fungal hyphae, thus promoting their co-migration (Yang et al., 2016). The very first evidence of T3SS driven fungi-bacteria symbiosis was demonstrated for the obligate association between Mycetohabitans rhizoxinica and Rhizopus microsporus. In the absence of its T3SS, M. rhizoxinica was unable to induce the sporulation of its host (Lackner et al., 2011b).
In this study, we took advantage of the increasing amount and quality of publicly available genomic data to conduct the identification and characterization of the T3SS across the genera of the Burkholderia s.l. group, and search for correlations with bacterial ecologies and host-interactions. In the process, we identified two novel T3SS types which are exclusively present in Burkholderia s.l. species. We further used the rice growth promotion models Paraburkholderia kururiensis M130 and Burkholderia vietnamiensis LMG10929, bearer of an Hrp-2 and a novel T3SS, respectively, to conduct a preliminary characterization of its role in the interaction with rice.
Materials and Methods
Type 3 Secretion System Homology Screening in Burkholderia s.l.
We used the SctC, SctN, SctT, and SctV sequences of seven well characterized T3SS representatives (Abby and Rocha, 2012) to identify the T3SS clusters of Burkholderia s.l. species through homology screening using BLASTp (Supplementary Table 1). The amino acid sequences of SctC, SctN, SctT, and SctV from the representatives of each T3SS type were individually screened for in a database representing the diversity of Burkholderia s.l. with one representative strain for each species (mostly the type strain and alternatively the strain with the genome of highest quality; Supplementary Table 2). The database additionally holds 10 new strains that were isolated from rice roots and belong to either Burkholderia or Paraburkholderia (Bioproject PRJEB31911 and PRJEB42536). Strains were collected, and genomes sequenced as described in Wallner et al. (2019). Genome annotation was performed by the MicroScope platform (Vallenet et al., 2020). Duplicate hits from different BLAST runs were curated and the hit of highest homology conserved.
Phylogenetic Reconstructions
To infer the phylogenetic evolution of Burkholderia s.l. strains nine coding sequences (dnaG, ftsZ, glnA, gyrB, pykA, recA, rho, rpoB, and secA) of 146 Burkholderia s. l. strains and two outgroup strains (i.e., Ralstonia solanacearum K60 and Cupriavidus metallidurans CH34) were used. The genes were aligned separately using the MUSCLE software and subsequently concatenated resulting in a 16,630 bp alignment. An independent alignment was generated using the same loci for the 80 T3SS-bearing Burkholderia s.l. strains.
To specifically study the evolution dynamics of the T3SS, four protein sequences (SctC, SctN, SctT and SctV) were aligned separately using MUSCLE and subsequently concatenated to form a 1,652 aa alignment for 136 T3SS found in Burkholderia s.l. and 15 reference strains (Supplementary Table 2). Additional sequences of the protein homologs in the flagella coding cluster (FliI, FliR, FlhA) of E. coli were added (No SctC homolog exists) to form the outgroup.
All phylogenetic trees were constructed using the BEAST v1.10.2 software with GTR + G + I and LG + G + I substitution models for nucleotide and amino acid sequences, respectively (as determined to be the most suitable using the MEGA-X built-in tool for model selection), and assuming constant lineage birth rate for each branch with a Yule tree prior (best suited for describing the relationships between individuals from different species). The analysis was run for 5,000,000 generations, sampling every 1,000 generations, and a burn-in of 500,000 was applied. Posterior probabilities above 95% are reported in the form of black dots at the nodes.
Screening for Promoter Regions and Effectors
The PIP-box promoter region was screened for using the prosite format sequence: T-T-C-G-[CGT]-N(15)-T-T-C-G-[CGT]-N(30,32)-[CT]-A-N(3)-T and the EMBOSS v6.5.7.0 fuzznuc software to infer abundance in target genomes. To specifically detect PIP-box regions that are concurrently found in promoting regions of genes, the EDGAR2.3 platform was used (Blom et al., 2016). Screening for homologs of experimentally validated effectors was conducted using BLASTp with a threshold of 30% alignment identity and 1–10 E-value, on a database of 515 T3SS effectors involved in pathogenesis or symbiosis (Hu et al., 2017). The 1,538 hits were analyzed with the EffectiveT3 prediction tool (Eichinger et al., 2016) resulting in 464 putative effectors with a prediction score > 0.1. The list was further refined by removing hits in strains lacking any kind of T3SS type and hits resulting from homology with effectors that yielded homologs with low identity in > 90% of screened Burkholderia s.l. strains.
Bacteria Growth Conditions and Insertional Mutagenesis
Burkholderia vietnamiensis LMG10929 (Bv) and Paraburkholderia kururiensis M130 (Pk) were cultured using Luria’s low salt LB (LBm; Sigma-Aldrich). Fragments of Bv’s hrcV and Pk’s hrcC genes were inserted into a pGEM-T Easy vector (Promega) using specific primers (hrcV-fwd: 5′-GTTCATCGTGTCGGTGGTC-3′, hrcV-rev: 5′-GAACGACCATTTCGGGAAG-3′, hrcC-fwd: 5′-GTTGAAGGCAAAGATCTCAAGG-3′, hrcC-rev: 5′-CGCCACCATTACCACCTC-3′) and cloned into heat-shock competent E. coli JM109 cells. Positive cells were multiplied, and their plasmid extracted using the Wizard Minipreps DNA purification system (Promega). The gene fragments were liberated from the plasmid by an EcoRI digestion and separated on a 1% agarose gel followed by a purification using a NucleoSPin Gel and PCR Clean-up kit (Macherey-Nagel). The target vector pSHAFT2 (Shastri et al., 2017) carrying a chloramphenicol (Cm) resistance gene was opened by an EcoRI digestion and treated with an alkaline phosphatase. Insert and plasmid were mixed in a 5:1 ratio regarding their respective molecular size and ligated using a T4 DNA ligase (Promega). The resulting vectors, pSHAFT2-hrcC and pSHAFT2-hrcV, were cloned into heat-shock competent E. coli CC118 (λpir) which were to be used as donor cells during the subsequent triparental mating process (Supplementary Table 3). Spontaneous streptomycin (St) resistant Bv and Pk mutants were used as acceptor cells. Donor, acceptor (i.e., Pk-StR and Bv-StR) and E. coli DH5α carrying a pRK2013 helper plasmid were grown overnight (in liquid LB at 37°C for E. coli species with 50 μg.mL–1 kanamycin for helper strain and 50 μg.mL–1 chloramphenicol for donor strain; in liquid LBm at 28°C with 100 μmol.L–1 streptomycin for Bv and Pk). The cultures were spun down and the cells were concentrated to OD600≈50. Acceptor, donor and helper cells were mixed in a 10:1:1 ratio and 50 μL spots were spread out on square LBm plates. The plates were incubated at 28°C for 12 and 48 h for Bv and Pk, respectively. The bacterial spots were then resuspended in liquid LBm before being plated on LBm plates supplemented with 100 μg.mL–1 streptomycin and chloramphenicol to counter select for the presence of E. coli cells and acceptor cells having not integrated the plasmid. To validate the insertion of the plasmid through homologous recombination at the desired T3SS sites, we checked for the positive amplification of the cat2 Cm-resistance gene and the negative amplification of a set of primers binding upstream and downstream of the targeted area.
Plant Growth Conditions and Colonization Tests
Oryza sativa subsp. japonica cv. Nipponbare plants were cultured as described in King et al. (2019). Briefly, seeds were sterilized using successive 70% ethanol and 3.6% sodium hypochlorite treatments and germinated seedlings were transferred onto sterile perlite in an airtight hydroponic system. Plants were grown for 5 days before being inoculated with Pk-StR, Bv-StR, Pk-ΔhrcC, and Bv-ΔhrcV. Each individual hydroponic system contained 5 plants. Each plant was inoculated at the stem basis with 1 mL of water containing 1 × 108 bacterial cells. Control plants were inoculated with 1 mL of water. Two systems (2 × 5 plants) were prepared for each condition and time point. At 7 and 14 dpi, the five fittest looking plants were harvested for each condition. The entire root system was sampled and transferred to a screw cap tube containing 1 mL of sterile water and a sterile ceramic bead. Roots were weighted and then pulverized using a Fastprep-24 (MPbio) at 6 m.s–1 for 40 s. A serial dilution of the resulting solution was spotted out on square LBm plates supplemented with 100 μg.mL–1 streptomycin and incubated at 28°C for 48 h before counting CFU.
Results
Phylogenetic Analysis Suggests Two Novel Type 3 Secretion System Types in Burkholderia s.l.
The screening for injectisome coding regions within 146 Burkholderia s.l. strains, representing 139 different species yielded a total of 134 T3SS clusters distributed in 84 strains. Our selection of four core proteins, i.e., SctC, SctN, SctN, SctV, concatenated into a 1652 amino acid alignment and used for the phylogenetic reconstruction resulted in the clustering of Burkholderia s.l. T3SS into seven different clades (Figure 1 and Supplementary Table 4). Five of those clades cluster together with reference T3SS which were included to infer homology to validly described T3SS, i.e., Hrp-1, Hrp-2, SPI-1, SPI-2, and Ysc (see Experimental procedures). No Burkholderia s.l. T3SS is phylogenetically close to the Rhizobiales- or Chlamydiales-like T3SS. However, two additional categories distinctly appear which do not cluster with any reference sequence (named BCI and PSI, described in detail below). One unique T3SS, belonging to Paraburkholderia madseniana RP11 is phylogenetically isolated (Figure 1) and could potentially represent an eighth T3SS category.
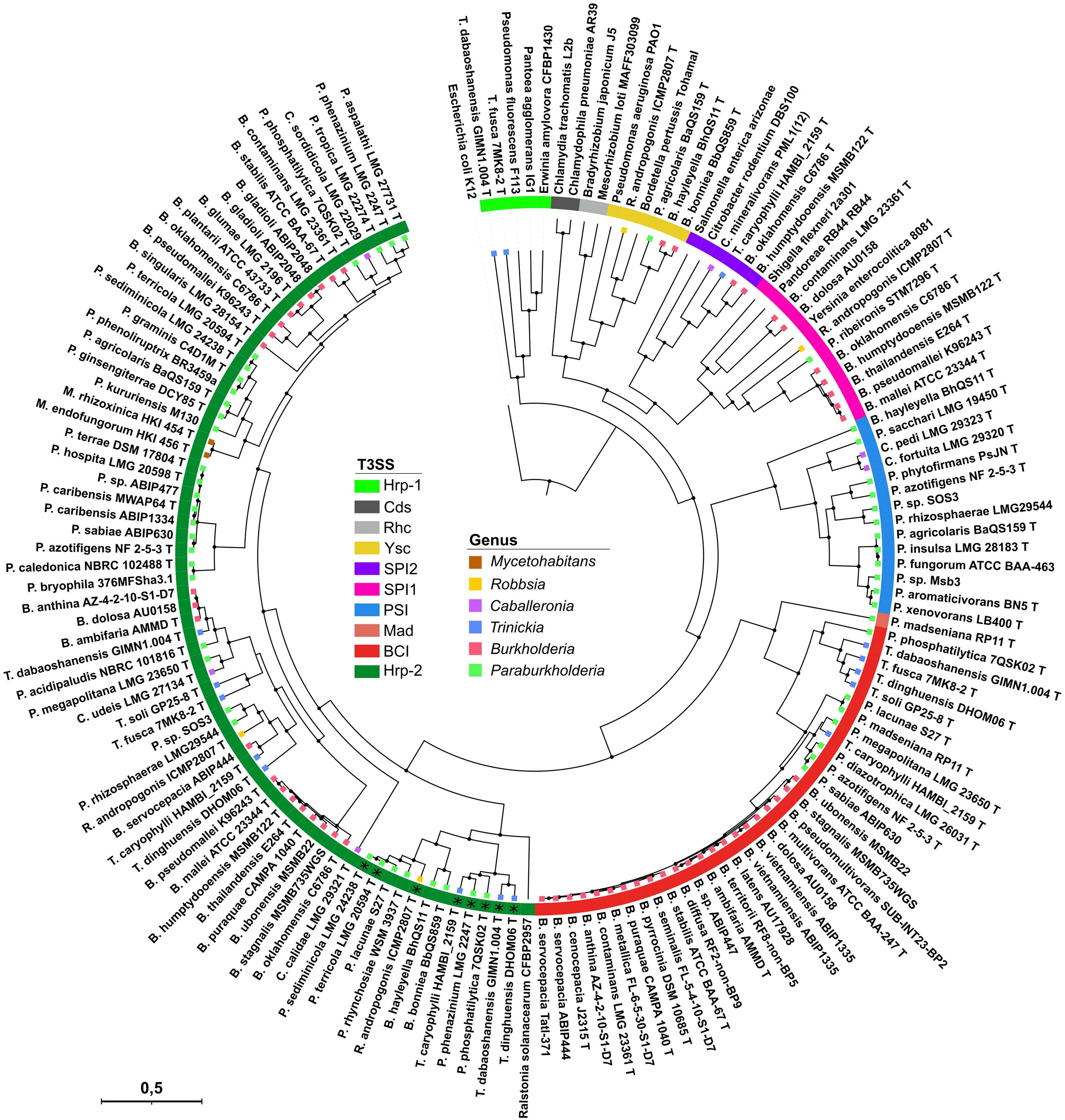
Figure 1. Phylogenetic reconstruction of T3SS cluster evolution amongst Burkholderia s.l. species. 80 Burkholderia s.l. strains containing a total of 136 secretion systems are represented. Their phylogenetic distribution was inferred using a Bayesian prediction on the concatenated SctC, SctN, SctT, SctV protein sequences resulting in a 1,652 aa alignment. Homologous flagellum components were used for the outgrouping E. coli strain in order to root the tree. The colored strip indicates the T3SS types and the colored squares at the branch tips represent the genus of each strain. (*) Eight strains possess a second Hrp-2 injectisome which is phylogenetically close to the one from Ralstonia solanacearum.
We analyzed conservation levels of T3SS-related operon synteny within and between the different phylogenetic types found amongst Burkholderia s.l. strains (Figure 2). The thirteen core components are consistently found in every T3SS type. The overall chromosomal organization of the T3SS coding operons is strongly varying from type to type. Within T3SS types however, there is little variation even between phylogenetically distant strains sharing the same type of injectisomes, e.g., Ralstonia solanacearum CFBP2957 and Paraburkholderia kururiensis M130 (Supplementary Figure 1). The new clusters identified in the previous section (BCI and PSI) display a distinct chromosomal organization from the remaining T3SS types (Figure 2).
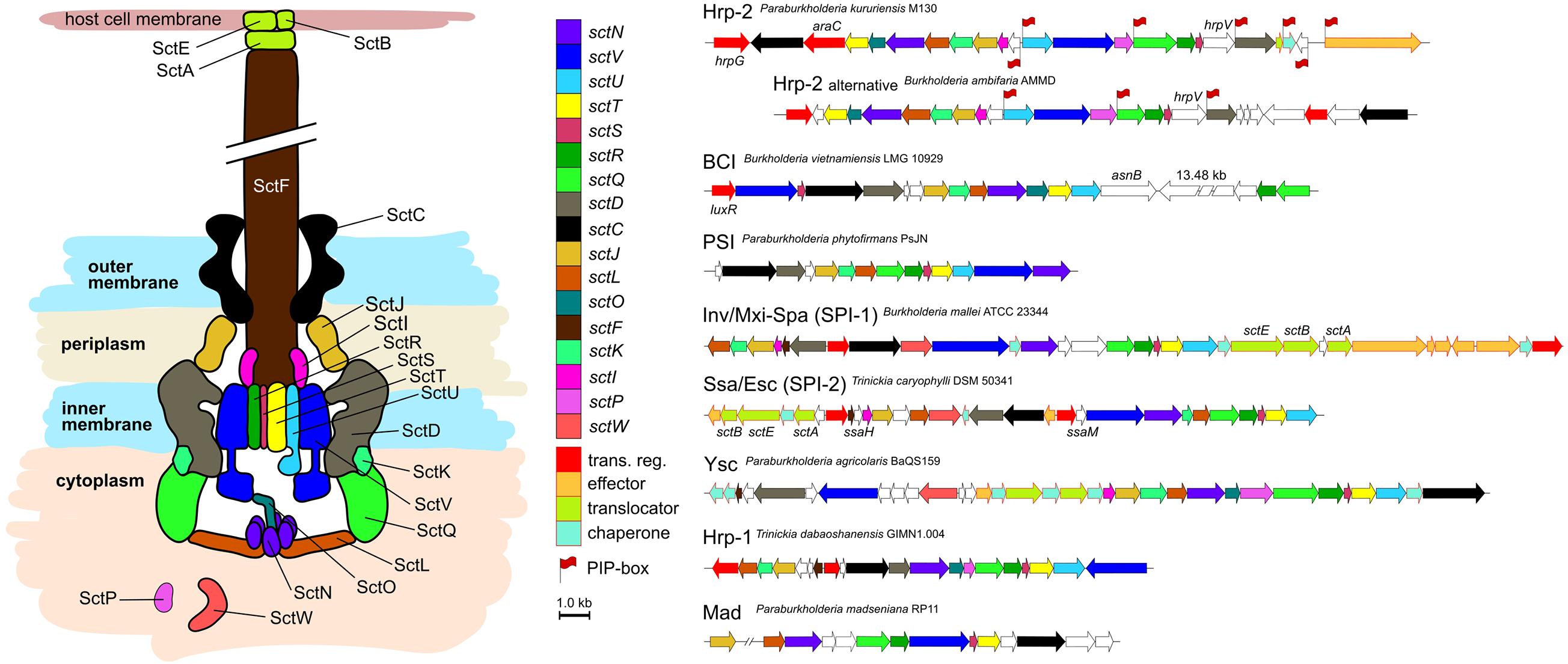
Figure 2. Schematic overview of the T3SS and synteny of coding regions Burkholderia s.l. species. Seven different major types of injectisomes are found in Burkholderia s.l. species. They can be distinguished by their genetic proximity but also by the chromosomic organization of their coding region as depicted here. These regions vary in size and composition while a subset of 12–13 core components is ubiquitously present (sctO is absent in PSI, SPI-1, and SPI2; sctK is absent in Hrp1). Secretion and cellular translocation genes have an individual color code with a black outline. Further genes involved in transcription or coding for effectors, their translocators and chaperones are depicted with a red outline. Genes without sct nomenclature or with unknown functions are left blank. Red flags at gene beginnings indicate the presence of a PIP-box regulation sequence in the region preceding the CDS.
Burkholderia Cepacia Complex Injectisome and Paraburkholderia Short Injectisome: Novel Injectisomes With Differential Distribution
Based on phylogenetic analysis and operonic organization, we observed two novel injectisomes. The first one, which we refer to as Paraburkholderia Short Injectisome (PSI), is dominantly present in Paraburkholderia with 11 representative strains. Additionally, two Caballeronia species harbor this new type as well as Burkholderia hayleyella (Figures 1, 3). Its existence was already reported in studies on P. phytofirmans which insisted on its atypical chromosomic organization (Mitter et al., 2013; Angus et al., 2014). Compared to other injectisome families present in Burkholderia s.l. the regulon coding for this peculiar T3SS is remarkably short with only 12–14 kb and containing 14–16 genes. Although most structural sct components are present, we could not identify sctF, the canonical gene coding for the needle-forming component. Still, several genes with unidentified functions within the operon could fulfill this function as they are in the size range of previously reported sctF genes. The needle component sctF is extremely variable (Lombardi et al., 2019) and is usually undetectable by simple homology approaches. To confirm its presence would require a thorough and dedicated investigation, including protein structure analyses and functional validation (Lombardi et al., 2019).
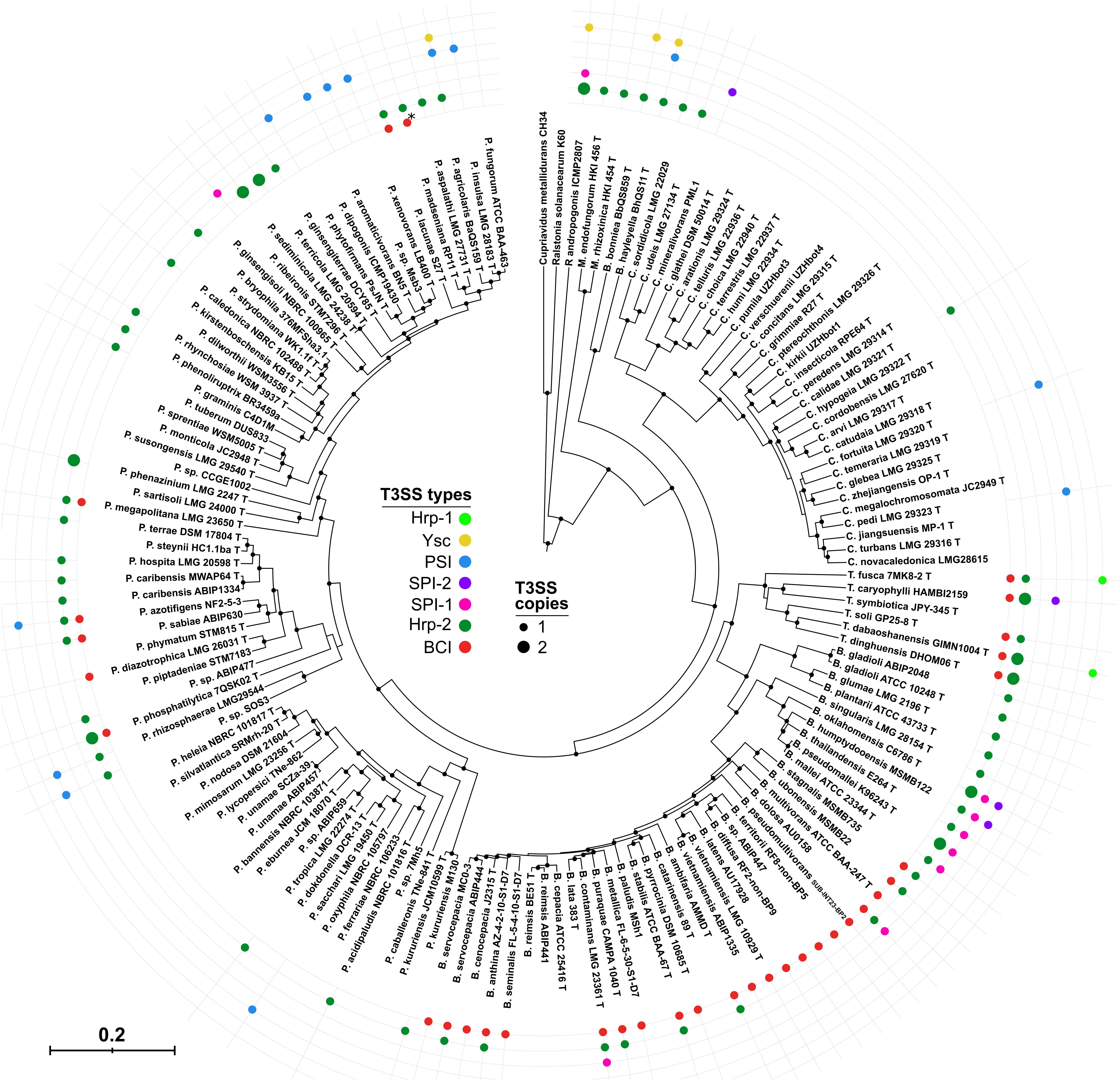
Figure 3. Distribution of T3SS types in Burkholderia s.l. species. Nine concatenated coding sequences (dnaG, ftsZ, glnA, gyrB, pykA, recA, rho, rpoB, and secA) of 146 Burkholderia s.l. strains were used to infer the Burkholderia s.l. phylogeny using a Bayesian approach. From the outermost circle inwards, colored circles are representative of the presence of an operon coding for the corresponding T3SS. Large Hrp-2 circles indicate the presence of two similar operons in the genome. ∗P. madseniana displays a peculiar T3SS, Mad, similar to the BCI but phylogenetically distinct.
The second uncharacterized T3SS family, which we refer to as Burkholderia cepacia complex Injectisome (BCI), is almost ubiquitously present among species of the Burkholderia cepacia complex (BCC). It is further found in four Trinickia and seven Paraburkholderia species (Figures 1, 3). The operonic structures coding for this injectisome contains the thirteen core genes. A confident identification of the needle component sctF proved here again to be unsuccessful. Still, both BCI and PSI types have been transferred independently to multiple species and been conserved throughout speciation (Figure 1). This strongly suggests that the PSI and BCI remain functional and that selection favored their conservation.
The particularity of the BCI is the presence of a large > 10 kb element separating the sctR and sctQ genes from the rest of the operon (Figure 2). The protein encoded by this large element is predicted to possess a peptidoglycan binding LysM domain. An adjacent gene is encoding a putative lytic transglycolase, allowing peptidoglycan degradation. Another interesting feature is the upstream luxR-type regulator which could hint toward a quorum sensing dependent activation of the operon.
By a homology search using the concatenated sequences also used in the T3SS phylogeny, we verified if the PSI and BCI types are present outside Burkholderia s.l. species. For the PSI type, the closest hits had a similarity of less than 50% sequence homology and a divergent chromosomal organization. For the BCI, however, there were several hits with > 55% sequence homology and a concordant operon structure. These BCI are mainly found in Variovorax species but also in other families of the Burkholderiales order which it seems restricted to Supplementary Figure 2.
The last new T3SS presents no taxonomical closeness to any model used in our phylogenetical reconstruction where it is placed at the interface between the BCI and the PSI (Figure 1). It is found in P. madseniana RP11 and also has a chromosomal organization that diverges from the remaining types found in Burkholderia s.l. (Figure 2). The available genome for this strain is relatively fragmented (393 contigs) and the T3SS operon is split. Nine out of thirteen core genes could nonetheless be detected and do not show signs of degeneration suggesting that these divergent genes remain active. However, as we do not have any diversity supporting the description of this non-canonical T3SS type we will not further insist on the characterization of this putative injectisome.
The Injectisome Is a Frequent Feature of Burkholderia s.l. Species
Among the Burkholderia s.l. representatives tested here, 58% possess a T3SS. However, there are great disparities between genera. Indeed, only six T3SS-positive strains were detected amongst 31 Caballeronia species (Figure 3). Several species belonging to this genus were described in endosymbiotic associations with insects or plant leaves (Miller, 1990; Kikuchi et al., 2005) but none are T3SS-positive.
It is not uncommon for Burkholderia s.l. species to bear two or more T3SS clusters (22% of strains). For instance, out of the six validly described Trinickia species, four have three injectisome-coding clusters of different types.
We attempted to infer correlations between the T3SS type carried by a strain and its ecologic isolation source. The presence of a SPI-1 type is strongly correlated with a strain’s isolation from a clinical setting as it is present essentially in the Burkholderia mallei complex (Figure 3 and Supplementary Table 2). While species of the BCC are also frequently described as potential human opportunistic pathogens, they are equally present in association with plants, in the rhizosphere or as environmental strains. The BCI which is the most frequent T3SS among BCC strains, is dominantly found in environmental or plant-associated bacteria (Figure 3 and Supplementary Table 2). The Hrp-2, which has a demonstrated role in plant-interaction, is also occasionally found in clinical species but dominantly in environmental and plant-isolates. Finally, the PSI type T3SS was never detected in clinical isolates among the surveyed Burkholderia s.l. strains. Furthermore, 10 out of 16 legume nodulating Paraburkholderia and Trinickia species lack a T3SS (Figure 3).
The Ysc type injectisome is rare in Burkholderia s.l. species (Figure 3). Beside Robbsia andropogonis it is restricted to three species which share amoeba as a common host (Haselkorn et al., 2019), Paraburkholderia agricolaris and the genetically distant Burkholderia bonniea and B. hayleyella (Figure 3). Unlike the remaining T3SS types they share, the Ysc appears to be well conserved among these 3 species (Figure 1).
Injectisomes Have a Complex Evolutionary History in Burkholderia s.l. Species
There are great disparities in the distribution of the different T3SS types between different Burkholderia s.l. species (Figure 3 and Supplementary Figure 3). The Hrp2 distribution along every genus, and especially in the early diverging genera Mycetohabitans and Robbsia, suggests that it could have been present in the common ancestor of the Burkholderia s.l. genera, subsequently lost in different species and regularly reacquired, supporting its central role in several species. The second most abundant injectisome is the newly described BCI type which is present in almost every species of the BCC and Trinickia spp. as well as several Paraburkholderia species. The BCI sequences recovered from Burkholderia s.s. species form a single clade (Figure 1), suggesting not only a single emergence event in this group, but also its maintenance all along the diversification of this genus, reflecting its evolutionary importance. Both SPI-1 and PSI injectisomes are more occasional and are almost completely restricted to the Burkholderia mallei complex (BMC) and a Paraburkholderia sub-clade, respectively. The three remaining T3SS types, SPI-2, Hrp-1, and Ysc are only sporadically present in Burkholderia s.l. species.
Many Burkholderia s.l. species harbor two or more different injectisomes which always belong to different types except for Hrp-2. Eight of the ten species bearing two Hrp-2 have one copy closely related to the Hrp-2 of R. solanacearum suggesting the existence of Hrp-2 subfamilies with potential diverging functions.
We further verified if the complex gain and loss dynamics of T3SS are also displayed at the intraspecific level. Many Burkholderia s.l. strains were only recently described and are consequently represented by a single whole-genome sequence. Still, several species with either clinical importance or peculiar ecologies and functions (such as nodulation capacity) have received increased attention and had several genomes sequenced.
Using all 87 publicly available genomes, we validated that B. vietnamiensis uniformly possesses a single T3SS of the BCI type (screened strains and T3SS types for the following species are listed in Supplementary Table 5). This was likewise verified for all 11 P. tropica representatives which uniformly display an Hrp-2 type. Trinickia symbiotica is the only representative of its genus lacking a T3SS (Figure 3) and we confirmed that this was consistently valid in all six available strains (Supplementary Table 5). Similarly, the species-wide absence of T3SS was validated for eight P. caballeronis, and five P. silvatlantica strains. P. fungorum is the only representative of PSI-bearing species with more than four representative and sequenced strains. All P. fungorum uniformly possess a single T3SS of the PSI type. For all these species, there is therefore a uniformity of their genomic content of secretion systems.
While B. lata strain 383 was identified to lack any kind of T3SS, screening of the remaining 23 available genomes of this species yielded a more diverse landscape with eight strains being positive for one T3SS (Supplementary Table 5). Still, the absence of injectisome is the main trend in this species. While not a ubiquitous feature, two B. servocepacia strains were detected to have a Hrp-2 injectisome in addition to their BCI. The recently described B. servocepacia species englobes strains that were affiliated to B. cenocepacia but show significant genomic divergence (Wallner et al., 2019). The Hrp-2 was never observed in B. cenocepacia strains despite a wider diversity of available genomes and could thus be a sign of adaptation toward improved interaction with plants that is displayed by B. servocepacia.
Our prediction for B. contaminans using strain LMG23361, however, seems to be the exception among the diversity of strains for this species. Indeed, the Hrp-2/BCI/SPI-1 trinity of injectisomes was not detected in any other of the 35 genomes available for B. contaminans. Only five strains display both an Hrp-2 and a BCI type while the remaining strains present either one of those injectisome types (Supplementary Table 5).
Effectors of Type 3 Secretion System -Positive “Environmental” Strains Remain Elusive
In Burkholderia s.l. species, the Hrp-2, Hrp-1, Ysc, and SPI-2 type encoding genetic regions are lacking several genes coding for effectors and chaperones compared to the pathogenic bacteria where these systems have been described (Figures 4A–D). The same trend can be observed for the SPI-1 type of P. ribeironis STM7296, a legume nodulating species (Bournaud et al., 2017), which also underwent substantial gene loss compared to other SPI-1 carriers such as B. pseudomallei (Figure 4E).
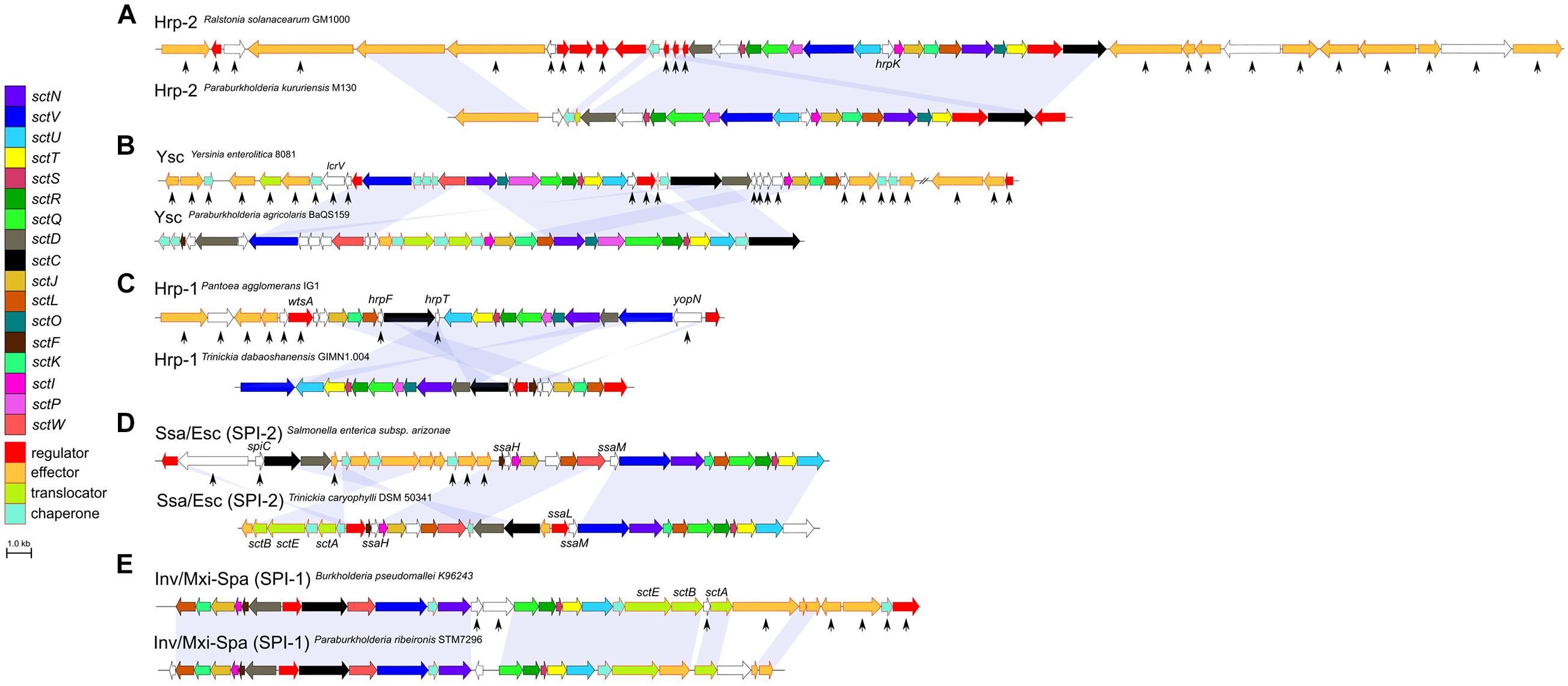
Figure 4. Loss of accessory genes in T3SS synteny blocks of Burkholderia s.l. species. The Hrp-2 type (A) is the most frequent T3SS in Burkholderia s.l. species. The Ysc (B), Hrp-1 (C) and SPI-2 (D) are T3SS types which rarely occur in Burkholderia s.l. species. The SPI-1 type (E) is only represented in a single Paraburkholderia species. In each case, several genes coding for effectors and chaperones as well as proteins with unknown functions are lost (black arrows) compared to pathogens were these genes have a described role in virulence.
Using a database of 515 validated effectors from animal and plant pathogens but also rhizobia (Hu et al., 2017), we screened for homologies in the genomes of Burkholderia s.l. species. We used the effector predictive tool EffectiveT3 to cross-check the resulting list of putative effectors (Eichinger et al., 2016). This approach yielded hits for 62 Burkholderia s.l. species (Supplementary Table 6). The species for which we detected the most effectors are the well-studied plant pathogens T. caryophylli and R. andropogonis (17 and 16 hits, respectively). We also detect the animal pathogens of the Burkholderia mallei complex, the plant pathogens of the Burkholderia glumae complex and the fungal endosymbionts of the Mycetohabitans genus among the species with the most effectors (Supplementary Table 6). Our survey identified several putative effectors in commensal Paraburkholderia and Trinickia species. Mostly, these candidates share homology with previously validated effectors originating from Ralstonia. However, we observed no homology to nodulation enabling Nop effectors amongst the legume nodulating Paraburkholderia and Trinickia species.
As the Hrp-2 is the dominant T3SS of Burkholderia s.l. species, we screened the genomes used in this study for the presence of Plant Inducible Promoter regions (PIP-box; TTCG[CGT]N(15)TTCG[CGT]N(30,32)[CT]AN(3T), indicative of T3SS-linked genetic regulation (Koebnik et al., 2006). Two to eight of these regulatory sequences were found within the operon coding for the T3SS machinery depending on the species (Figure 2). As expected, the total number of PIP-box matching sequences is significantly higher in species possessing an Hrp-2 type injectisome compared to those lacking this type (Wilcoxon test, p < 1 × 10–6). However, the three polyphyletic Hrp-2 bearing amoeba symbionts (B. bonniea, B. hayleyella and P. agricolaris) are not enriched in PIP-box sequences despite the presence of the PIP-box binding regulator HrpB, suggesting that their Hrp-2 has drifted from its initial function to another purpose.
Novel Burkholderia Cepacia Complex Injectisome Injectisome Is Involved in Rice Root Colonization by B. vietnamiensis LMG10929
Two model species of the Burkholderia group, P. kururiensis strain M130 (Pk) and B. vietnamiensis strain LMG10929 (Bv), are known to form beneficial and intricate relationships with rice. Both heavily colonize the root surface and have been observed as endophytes (Mattos et al., 2008; King et al., 2019). They are potent plant growth promoters which is linked to their ability to transfer fixed atmospheric nitrogen to the plant and to synthetize growth promoting phytohormones (Trân Van et al., 2000; Dias et al., 2019).
Pk and Bv possess a single injectisome of Hrp-2 and BCI type, respectively (Figure 3). We constructed insertion mutants targeting the sctC and sctV genes in Pk and Bv, respectively (Figure 1). The deletion of any of these structural components has repeatedly been used to efficiently inactivate T3 secretion in Burkholderia s.l. species (Muangsombut et al., 2008; Lackner et al., 2011a; Gavrilin et al., 2012; Swain et al., 2017).
We then compared their colonization efficiency to that of WT strains at different time-points (Figure 5). The mutation has no impact on the colonization capacity of rice roots by Pk in the first 2 weeks after inoculation. Bv however, is strongly affected by the loss of its T3SS. At 7 dpi, the mutant’s colonization capacity is only at 13% of the WT stain’s capacity. At 14 dpi the mutant’s population collapses further to 0.75% compared to the WT strain.
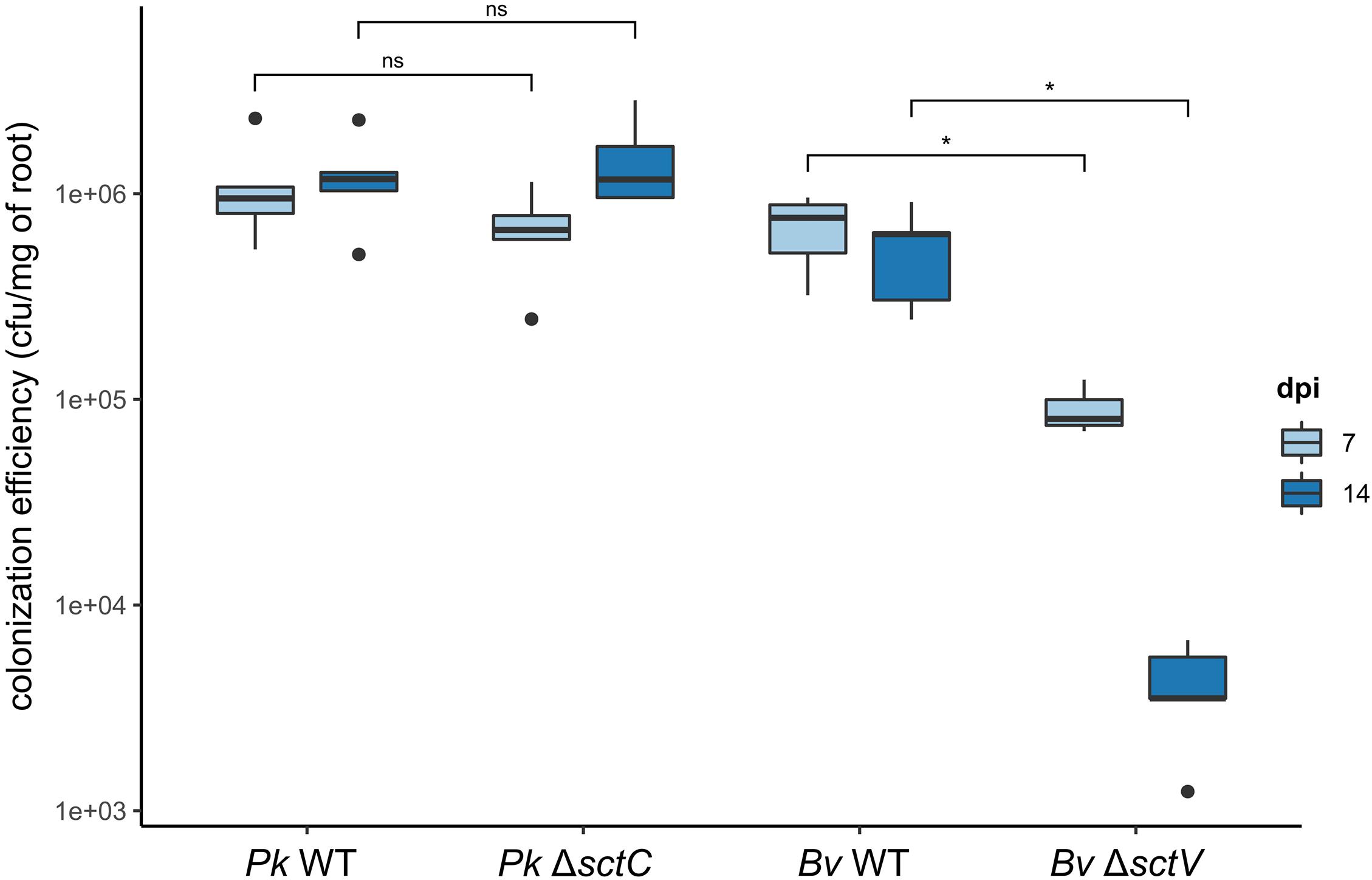
Figure 5. Effect of T3SS loss on root colonization by B. vietnamiensis LMG10929 and P. kururiensis M130. Rice seedlings were inoculated with either WT or T3SS deficient strains of P. kururiensis M130 or B. vietnamiensis LMG10929 and the roots were harvested at 7 and 14 dpi, homogenized and the colonizing bacteria population estimated using serial dilutions and spot counting. Cfu/mg of roots were compared between conditions with a Wilcoxon test (*p < 0.01; ns, not significant). Black dots represent outgrouping samples. Mock inoculations were conducted on a control set of plants which showed a complete absence of developing bacteria at both time-points.
Discussion
The Type 3 Secretion System Is a Frequent and Diversified Tool in Burkholderia s.l. Species
T3SS are one major driver of virulence in pathogenic strains and are increasingly described to play a central role in several beneficial associations between bacteria and their host. Besides the mammal pathogens associated to the Burkholderia mallei complex, almost no attention has been brought to the T3SS of Burkholderia s.l. species. These species were described to engage in a multitude of interactions ranging from symbiosis to pathogenicity with a variety of hosts. Burkholderia s.l. species present a strong diversity in T3SS types, with some strains possessing up to four T3SS-coding clusters, suggesting a central role for this macromolecular tool in the interaction capacity of Burkholderia s.l. strains. Such diversity is however not unique in the bacterial kingdom. Hu et al. (2017) had shown in a large survey that 38 genera carried multiple T3SSs, all having independent evolutionary origins (Hu et al., 2017). Among these 38 genera, Burkholderia s.l. has, however, the greatest diversity of T3SS, even though the original sampling did not cover the entire available species diversity. The T3SS plurality could represent a strategy to achieve an ecologic diversity as an adaptation to multiple hosts or to different levels of interaction with a same host. Indeed, several Burkholderia s.l. species have a broad host range that can include organisms from different kingdoms. Naturally, the use of a T3SS is by no means the only mechanism allowing an efficient host colonization. Additional secretion systems involved in the export of proteins are also considerably contributing to the success of a bacteria’s interaction with its host (Tseng et al., 2009). Interestingly, several Burkholderia s.l. species bearing one or more injectisome-coding regions have only been isolated from environmental settings to date. Thus, there are strong chances that an unknown or not yet discovered eukaryotic partner exists for those strains. For strains that have so far been isolated from a single host but bear several T3SS, alternative hosts could exist.
T3SS types display a complex distribution both between Burkholderia s.l. species but also between different strains of a same species, demonstrating how readily these systems can be acquired or lost. The selective pressures forcing these acquisition/loss dynamics could be set by the diversity of hosts these bacteria are set to colonize. Hence, intra-specific host diversity can be suggested by the heterogeneous distribution of T3SS among strains of a same species. Again, we are limited by the available knowledge on Burkholderia s.l. strain ecology to confidently interpret the consequences of intra-specific T3SS heterogeneity.
The Lack of Effector Detection for the Non-canonical Injectisomes May Suggest Another Role Than Secretion
Among the most frequent injectisomes of Burkholderia s.l. are the BCI and PSI types. Both have been noted before in individual strains and some of their particularities have already been reported (Tomich et al., 2003; Mitter et al., 2013; Angus et al., 2014) but they had never been subjected to a wide scale characterization. Here, we report the distribution and conservation of the BCI and PSI among Burkholderia s.l. species. Both could be involved in non-virulent plant-colonization as suggested by the ecology of the strains these systems are found in. This supposition is corroborated for the BCI by the rice colonization deficiency of a T3SS insertional mutant of B. vietnamiensis LMG10929. Whether it is used for adhesion, as support for biofilm formation or to inject effector proteins, remains to be investigated.
If effectors are secreted through the BCI, they are unlike the effectors which have been identified hitherto for the other T3SS types as we could not detect any of them by homology. Their de novo prediction based on the detection of T3 secretion signals was also unsuccessful. This could be resulting from the limits of these computational approaches when screening for non-canonical effectors. Still, an alternative role to protein secretion cannot be excluded at this point.
The BCI is abundant in species of the BCC which are known to efficiently colonize the rhizosphere. However, these are not described to be phytopathogens. With B. vietnamiensis as leading figure, several BCC members have demonstrated plant growth promotion as they are potent phytohormone producers and nutrient providers (Trân Van et al., 2000; Rojas-Rojas et al., 2018). To date, the most common T3SS of plant interacting bacteria were those of the Hrp-1 and Hrp-2 type as well as the Rhc for rhizobia (Abby and Rocha, 2012).
Burkholderia Cepacia Complex Injectisome and Hrp-2: Contrasted Roles in Plant-Interaction
Here we suggest that the BCI might be an additional major player in plant interaction but for which effectors remain to be investigated. On the other side, and quite unexpectedly, the rice colonization efficiency of the P. kururiensis M130 Hrp-2 mutant was not impacted in the first 14 days of interaction. This result was surprising regarding all the literature on this T3SS type reporting its major role in plant-bacteria interactions. Contrarily to Bv, Hrp-2 positive Paraburkholderia species such as Pk, are often bearing a few homologs of effectors from plant pathogens such as Ralstonia or Pseudomonas. While interpretations of negative results must always be done with care, we can hypothesize that in the specific case of the Pk/Nipponbare association, the interaction does not require the use of an injectisome for colonization. We can further suggest that the T3SS plays little or no role in this precocious stage of plant colonization, but that its absence could rather have a major impact in the later stages, particularly during the establishment of endophytic colonization. The absolute functionality of Pk’s Hrp-2 would first need to be validated to safely interpret the present results, although it seems very unlikely that a bacterium would conserve a 24 kb genomic region showing no sign of degeneration if it was not functioning.
There is still much to discover on the general implication of T3SS in beneficial interactions and specifically for Burkholderia s.l. species which are promising plant growth promoting strains. A focus on the secreted effector proteins, using a combination of in silico predictive tools, transcriptomics, and secretome-proteomics, could provide valuable knowledge on the frontier between pathogenicity and mutualism resulting from the type III secretion.
Data Availability Statement
The data presented in the study are deposited in the NCBI repository, accession numbers PRJEB31911 and PRJEB42536.
Author Contributions
AW carried out the data extractions and analyses, plant tests and observations, and drafted the manuscript. IR participated in DNA extraction and strain cultures. NB was involved in mutant construction and plant testing. GB and LM participated to the design, coordination of the work, and to the writing of the manuscript. All authors read and approved the final manuscript.
Acknowledgments
The LABGeM (CEA/Genoscope and CNRS UMR8030), the France Génomique and French Bioinformatics Institute national infrastructures (funded as part of Investissement d’Avenir program managed by Agence Nationale pour la Recherche, contracts ANR-10-INBS-09 and ANR-11-INBS-0013) are acknowledged for support within the MicroScope annotation platform. The authors gratefully acknowledge financial support from the CGIAR research program (CRP) RICE as well as from the French national research agency (ANR) funding the BURKADAPT project (ANR-19-CE20-0007). AW was supported by a fellowship from the French Ministry of Higher Education, Research and Innovation.
Conflict of Interest
The authors declare that the research was conducted in the absence of any commercial or financial relationships that could be construed as a potential conflict of interest.
Publisher’s Note
All claims expressed in this article are solely those of the authors and do not necessarily represent those of their affiliated organizations, or those of the publisher, the editors and the reviewers. Any product that may be evaluated in this article, or claim that may be made by its manufacturer, is not guaranteed or endorsed by the publisher.
Supplementary Material
The Supplementary Material for this article can be found online at: https://www.frontiersin.org/articles/10.3389/fmicb.2021.761215/full#supplementary-material
Supplementary Figure 1 | Synteny conservation within T3SS types across Burkholderia s.l. strains.
Supplementary Figure 2 | Distribution of BCI and PSI types outside Burkholderia s.l. species. (A) Concatenated SctC, SctN, SctT and SctV sequences from the PSI and BCI of P. phytofirmans PsJN and B. vietnamiensis LMG10929, respectively, were used to screen sequence databases for homologies using BLAST and identify the closest similarities in the bacterial kingdom. (B) The SctC, SctN, SctT and SctV sequences of the closest hits (in bold) were retrieved, aligned and concatenated resulting in a 1,656 aa alignment. These sequences were used in a phylogenetic reconstruction alongside representatives of nine T3SS types as before (Figure 2).
Supplementary Figure 3 | Side by side comparison of a Burkholderia s.l. and a T3SS phylogeny. Nine concatenated coding sequences (dnaG, ftsZ, glnA, gyrB, pykA, recA, rho, rpoB, and secA) of the 80 T3SS-bearing Burkholderia s.l. strains were used to infer the Burkholderia s.l. phylogeny using a Bayesian approach. The right tree is a linearized version of Figure 1. Blue arrows on the left phylogeny exemplify the putative acquisition (right arrows) and losses (left arrow) of the PSI T3SS.
Supplementary Table 1 | Listing of T3SS types. For each type we provide a selection of genera where the respective T3SS has been described as well as the type of infection or symbiosis it is most often involved in. (∗) Specific strains which were used as reference for their T3SS in our phylogenetic analysis.
Supplementary Table 2 | List and details of strains used in this study.
Supplementary Table 3 | Bacterial strains and plasmids used in this study.
Supplementary Table 4 | T3SS repartition within Burkholderia s.l. strains.
Supplementary Table 5 | Intraspecific diversity of T3SS distribution for a selection of ten species.
Supplementary Table 6 | Curated list of candidate effectors in Burkholderia s.l. species.
References
Abby, S. S., and Rocha, E. P. C. (2012). The Non-Flagellar Type III Secretion System Evolved from the Bacterial Flagellum and Diversified into Host-Cell Adapted Systems. PLoS Genet. 8:e1002983. doi: 10.1371/journal.pgen.1002983
Angus, A. A., Agapakis, C. M., Fong, S., Yerrapragada, S., Estrada-de los Santos, P., Yang, P., et al. (2014). Plant-Associated Symbiotic Burkholderia Species Lack Hallmark Strategies Required in Mammalian Pathogenesis. PLoS One 9:e83779. doi: 10.1371/journal.pone.0083779
Attree, I., and Attree, O. (2001). A second type III secretion system in Burkholderia pseudomallei: who is the real culprit? Microbiology 147, 3197–3199. doi: 10.1099/00221287-147-12-3197
Bast, A., Krause, K., Schmidt, I. H. E., Pudla, M., Brakopp, S., Hopf, V., et al. (2014). Caspase-1-dependent and -independent cell death pathways in Burkholderia pseudomallei infection of macrophages. PLoS Pathog. 10:e1003986. doi: 10.1371/journal.ppat.1003986
Blom, J., Kreis, J., Spänig, S., Juhre, T., Bertelli, C., Ernst, C., et al. (2016). EDGAR 2.0: an enhanced software platform for comparative gene content analyses. Nucleic Acids Res. 44, W22–W28. doi: 10.1093/nar/gkw255
Bournaud, C., Moulin, L., Cnockaert, M., De Faria, S., Prin, Y., Severac, D., et al. (2017). Paraburkholderia piptadeniae sp. nov. and Paraburkholderia ribeironis sp. nov., two root-nodulatin symbiotic species of Piptadenia gonoacantha in Brazil. Int. J. Syst. Evol. Microbiol. 67, 432–440. doi: 10.1099/ijsem.0.001648
Burtnick, M. N., Brett, P. J., Nair, V., Warawa, J. M., Woods, D. E., and Gherardini, F. C. (2008). Burkholderia pseudomallei type III secretion system mutants exhibit delayed vacuolar escape phenotypes in RAW 264.7 murine macrophages. Infect. Immun. 76, 2991–3000. doi: 10.1128/IAI.00263-08
Coburn, B., Sekirov, I., and Finlay, B. B. (2007). Type III secretion systems and disease. Clin. Microbiol. Rev. 20, 535–549. doi: 10.1128/CMR.00013-07
Compant, S., Nowak, J., Coenye, T., Clément, C., and Ait Barka, E. (2008). Diversity and occurrence of Burkholderia spp. in the natural environment. FEMS Microbiol. Rev. 32, 607–626. doi: 10.1111/j.1574-6976.2008.00113.x
Deng, W., Marshall, N. C., Rowland, J. L., McCoy, J. M., Worrall, L. J., Santos, A. S., et al. (2017). Assembly, structure, function and regulation of type III secretion systems. Nat. Rev. Microbiol. 15, 323–337. doi: 10.1038/nrmicro.2017.20
Dias, G. M., de Sousa Pires, A., Grilo, V. S., Castro, M. R., de Figueiredo Vilela, L., and Neves, B. C. (2019). Comparative genomics of Paraburkholderia kururiensis and its potential in bioremediation, biofertilization, and biocontrol of plant pathogens. Microbiologyopen 8:e00801. doi: 10.1002/mbo3.801
Eichinger, V., Nussbaumer, T., Platzer, A., Jehl, M. A., Arnold, R., and Rattei, T. (2016). EffectiveDB - Updates and novel features for a better annotation of bacterial secreted proteins and Type III, IV, VI secretion systems. Nucleic Acids Res. 44, D669–D674. doi: 10.1093/nar/gkv1269
Gavrilin, M. A., Abdelaziz, D. H. A., Mostafa, M., Abdulrahman, B. A., Grandhi, J., Akhter, A., et al. (2012). Activation of the Pyrin Inflammasome by Intracellular Burkholderia cenocepacia. J. Immunol. 188, 3469–3477. doi: 10.4049/jimmunol.1102272
Haselkorn, T. S., DiSalvo, S., Miller, J. W., Bashir, U., Brock, D. A., Queller, D. C., et al. (2019). The specificity of Burkholderia symbionts in the social amoeba farming symbiosis: prevalence, species, genetic and phenotypic diversity. Mol. Ecol. 28, 847–862. doi: 10.1111/mec.14982
Holden, M. T. G., Seth-Smith, H. M. B., Crossman, L. C., Sebaihia, M., Bentley, S. D., Cerdeño-Tárraga, A. M., et al. (2009). The genome of Burkholderia cenocepacia J2315, an epidemic pathogen of cystic fibrosis patients. J. Bacteriol. 191, 261–277. doi: 10.1128/JB.01230-08
Hu, Y., Huang, H., Cheng, X., Shu, X., White, A. P., Stavrinides, J., et al. (2017). A global survey of bacterial type III secretion systems and their effectors. Environ. Microbiol. 19, 3879–3895. doi: 10.1111/1462-2920.13755
Hueck, C. J. (1998). Type III Protein Secretion Systems in Bacterial Pathogens of Animals and Plants. Microbiol. Mol. Biol. Rev. 62, 379–433. doi: 10.1128/MMBR.62.2.379-433.1998
Jiménez-Guerrero, I., Pérez-Montaño, F., Medina, C., Ollero, F. J., and López-Baena, F. J. (2015). NopC Is a Rhizobium-Specific Type 3 Secretion System Effector Secreted by Sinorhizobium (Ensifer) fredii HH103. PLoS One 10:e0142866. doi: 10.1371/journal.pone.0142866
Kang, Y., Kim, J., Kim, S., Kim, H., Lim, J. Y., Kim, M., et al. (2008). Proteomic analysis of the proteins regulated by HrpB from the plant pathogenic bacterium Burkholderia glumae. Proteomics 8, 106–121. doi: 10.1002/pmic.200700244
Kikuchi, Y., Meng, X.-Y., and Fukatsu, T. (2005). Gut symbiotic bacteria of the genus Burkholderia in the broad-headed bugs Riptortus clavatus and Leptocorisa chinensis (Heteroptera: Alydidae). Appl. Environ. Microbiol. 71, 4035–4043. doi: 10.1128/AEM.71.7.4035-4043.2005
King, E., Wallner, A., Rimbault, I., Barrachina, C., Klonowska, A., Moulin, L., et al. (2019). Monitoring of rice transcriptional responses to contrasted colonizing patterns of phytobeneficial Burkholderia s.l. reveals a temporal shift in JA systemic response. Front. Plant Sci. 10:1141. doi: 10.3389/fpls.2019.01141
Koebnik, R., Krüger, A., Thieme, F., Urban, A., and Bonas, U. (2006). Specific binding of the Xanthomonas campestris pv. vesicatoria AraC-type transcriptional activator HrpX to plant-inducible promoter boxes. J. Bacteriol. 188, 7652–7660. doi: 10.1128/JB.00795-06
Kubori, T., Matsushima, Y., Nakamura, D., Uralil, J., Lara-Tejero, M., Sukhan, A., et al. (1998). Supramolecular Structure of the Salmonella typhimurium Type III Protein Secretion System. Science 280, 602–605. doi: 10.1126/science.280.5363.602
Lackner, G., Moebius, N., and Hertweck, C. (2011a). Endofungal bacterium controls its host by an hrp type III secretion system. ISME J. 5, 252–261. doi: 10.1038/ismej.2010.126
Lackner, G., Moebius, N., Partida-Martinez, L., and Hertweck, C. (2011b). Complete genome sequence of Burkholderia rhizoxinica, an Endosymbiont of Rhizopus microsporus. J. Bacteriol. 193, 783–784. doi: 10.1128/JB.01318-10
Lee, Y. H., Chen, Y., Ouyang, X., and Gan, Y. H. (2010). Identification of tomato plant as a novel host model for Burkholderia pseudomallei. BMC Microbiol:10:28. doi: 10.1186/1471-2180-10-28
Lombardi, C., Tolchard, J., Bouillot, S., Signor, L., Gebus, C., Liebl, D., et al. (2019). Structural and Functional Characterization of the Type Three Secretion System (T3SS) Needle of Pseudomonas aeruginosa. Front. Microbiol. 10:573. doi: 10.3389/fmicb.2019.00573
Manivanh, L., Pierret, A., Rattanavong, S., Kounnavongsa, O., Buisson, Y., Elliott, I., et al. (2017). Burkholderia pseudomallei in a lowland rice paddy: seasonal changes and influence of soil depth and physico-chemical properties. Sci. Rep. 7, 1–11. doi: 10.1038/s41598-017-02946-z
Mattos, K. A., Pádua, V. L. M., Romeiro, A., Hallack, L. F., Neves, B. C., Ulisses, T. M. U., et al. (2008). Endophytic colonization of rice (Oryza sativa L.) by the diazotrophic bacterium Burkholderia kururiensis and its ability to enhance plant growth. An. Acad. Bras. Cienc. 80, 477–493. doi: 10.1590/S0001-37652008000300009
Miller, I. M. (1990). Bacterial Leaf Nodule Symbiosis. Adv. Bot. Res. 17, 163–234. doi: 10.1016/S0065-2296(08)60134-2
Mitter, B., Petric, A., Shin, M. W., Chain, P. S. G., Hauberg-Lotte, L., Reinhold-Hurek, B., et al. (2013). Comparative genome analysis of Burkholderia phytofirmans PsJN reveals a wide spectrum of endophytic lifestyles based on interaction strategies with host plants. Front. Plant Sci. 4:120. doi: 10.3389/fpls.2013.00120
Muangsombut, V., Suparak, S., Pumirat, P., Damnin, S., Vattanaviboon, P., Thongboonkerd, V., et al. (2008). Inactivation of Burkholderia pseudomallei bsaQ results in decreased invasion efficiency and delayed escape of bacteria from endocytic vesicles. Arch. Microbiol. 190, 623–631. doi: 10.1007/s00203-008-0413-3
Okazaki, S., Tittabutr, P., Teulet, A., Thouin, J., Fardoux, J., Chaintreuil, C., et al. (2016). Rhizobium-legume symbiosis in the absence of Nod factors: two possible scenarios with or without the T3SS. ISME J. 10, 64–74. doi: 10.1038/ismej.2015.103
Reinhold-Hurek, B., and Hurek, T. (2011). Living inside plants: bacterial endophytes. Curr. Opin. Plant Biol. 4, 435–43. doi: 10.1016/j.pbi.2011.04.004
Roine, E., Wei, W., Yuan, J., Nurmiaho-Lassila, E. L., Kalkkinen, N., Romantschuk, M., et al. (1997). Hrp pilus: an hrp-dependent bacterial surface appendage produced by Pseudomonas syringae pv. tomato DC3000. Proc. Natl. Acad. Sci. U. S. A. 94, 3459–3464. doi: 10.1073/pnas.94.7.3459
Rojas-Rojas, F. U., Salazar-Gómez, A., Vargas-Díaz, M. E., Vásquez-Murrieta, M. S., Hirsch, A. M., and De Mot, R. (2018). Broad-spectrum antimicrobial activity by Burkholderia cenocepacia TAtl-371, a strain isolated from the tomato rhizosphere. Microbiology 164, 1072–1086. doi: 10.1099/mic.0.000675
Sharma, S., Sharma, S., Hirabuchi, A., Yoshida, K., Fujisaki, K., Ito, A., et al. (2013). Deployment of the Burkholderia glumae type III secretion system as an efficient tool for translocating pathogen effectors to monocot cells. Plant J. 74, 701–712. doi: 10.1111/tpj.12148
Shastri, S., Spiewak, H. L., Sofoluwe, A., Eidsvaag, V. A., Asghar, A. H., Pereira, T., et al. (2017). An efficient system for the generation of marked genetic mutants in members of the genus Burkholderia. Plasmid 89, 49–56. doi: 10.1016/j.plasmid.2016.11.002
Skorpil, P., Saad, M. M., Boukli, N. M., Kobayashi, H., Ares-Orpel, F., Broughton, W. J., et al. (2005). NopP, a phosphorylated effector of Rhizobium sp. strain NGR234, is a major determinant of nodulation of the tropical legumes Flemingia congesta and Tephrosia vogelii. Mol. Microbiol. 57, 1304–1317. doi: 10.1111/j.1365-2958.2005.04768.x
Stevens, M. P., Friebel, A., Taylor, L. A., Wood, M. W., Brown, P. J., Hardt, W.-D., et al. (2003). A Burkholderia pseudomallei type III secreted protein, BopE, facilitates bacterial invasion of epithelial cells and exhibits guanine nucleotide exchange factor activity. J. Bacteriol. 185, 4992–4996. doi: 10.1128/JB.185.16.4992-4996.2003
Stevens, M. P., Wood, M. W., Taylor, L. A., Monaghan, P., Hawes, P., Jones, P. W., et al. (2002). An Inv/Mxi-Spa-like type III protein secretion system in Burkholderia pseudomallei modulates intracellular behaviour of the pathogen. Mol. Microbiol. 46, 649–659. doi: 10.1046/j.1365-2958.2002.03190.x
Swain, D. M., Yadav, S. K., Tyagi, I., Kumar, R., Kumar, R., Ghosh, S., et al. (2017). A prophage tail-like protein is deployed by Burkholderia bacteria to feed on fungi. Nat. Commun. 8, 1–9. doi: 10.1038/s41467-017-00529-0
Teulet, A., Busset, N., Fardoux, J., Gully, D., Chaintreuil, C., Cartieaux, F., et al. (2019). The rhizobial type III effector ErnA confers the ability to form nodules in legumes. Proc. Natl. Acad. Sci. U. S. A. 116, 21758–21768. doi: 10.1073/pnas.1904456116
Tomich, M., Griffith, A., Herfst, C. A., Burns, J. L., and Mohr, C. D. (2003). Attenuated virulence of a Burkholderia cepacia type III secretion mutant in a murine model of infection. Infect. Immun. 71, 1405–1415. doi: 10.1128/IAI.71.3.1405-1415.2003
Trân Van, V., Berge, O., Ngô, K. êS., Balandreau, J., and Heulin, T. (2000). Repeated beneficial effects of rice inoculation with a strain of Burkholderia vietnamiensis on early and late yield components in low fertility sulphate acid soils of Vietnam. Plant Soil 218, 273–284. doi: 10.1023/A:1014986916913
Tseng, T. T., Tyler, B. M., and Setubal, J. C. (2009). Protein secretion systems in bacterial-host associations, and their description in the Gene Ontology. BMC Microbiol. BioMed. Central. 9:S2. doi: 10.1186/1471-2180-9-S1-S2
Ura, H., Furuya, N., Iiyama, K., Hidaka, M., Tsuchiya, K., and Matsuyama, N. (2006). associated with symptoms of bacterial grain rot and leaf-sheath browning of rice plants. J. Gen. Plant Pathol. 72, 98–103. doi: 10.1007/s10327-005-0256-6
Vallenet, D., Calteau, A., Dubois, M., Amours, P., Bazin, A., Beuvin, M., et al. (2020). MicroScope: an integrated platform for the annotation and exploration of microbial gene functions through genomic, pangenomic and metabolic comparative analysis. Nucleic Acids Res. 48, D579–D589. doi: 10.1093/nar/gkz926
Vander Broek, C. W., and Stevens, J. M. (2017). Type III Secretion in the Melioidosis Pathogen Burkholderia pseudomallei. Front. Cell. Infect. Microbiol. 7:255. doi: 10.3389/fcimb.2017.00255
Wallner, A., King, E., Ngonkeu, E. L. M., Moulin, L., and Béna, G. (2019). Genomic analyses of Burkholderia cenocepacia reveal multiple species with differential host-Adaptation to plants and humans. BMC Genomics 20:803. doi: 10.1186/s12864-019-6186-z
Yang, P., Zhang, M., Warmink, J. A., Wang, M., and van Elsas, J. D. (2016). The type three secretion system facilitates migration of Burkholderia terrae BS001 in the mycosphere of two soil-borne fungi. Biol Fertil Soils 52, 1037–1046. doi: 10.1007/s00374-016-1140-6
Keywords: type three secretion system (T3SS), Oryza, root colonization, Paraburkholderia, PGPR—plant growth-promoting rhizobacteria, pathogen
Citation: Wallner A, Moulin L, Busset N, Rimbault I and Béna G (2021) Genetic Diversity of Type 3 Secretion System in Burkholderia s.l. and Links With Plant Host Adaptation. Front. Microbiol. 12:761215. doi: 10.3389/fmicb.2021.761215
Received: 19 August 2021; Accepted: 29 September 2021;
Published: 20 October 2021.
Edited by:
Israel Pagan, Polytechnic University of Madrid, SpainReviewed by:
Eneas Aguirre-von-Wobeser, Centro de Investigación en Alimentación y Desarrollo, Consejo Nacional de Ciencia y Tecnología (CONACYT), MexicoVasvi Chaudhry, University of Tübingen, Germany
Yuan Jin, Institute of Biotechnology, Chinese Academy of Agricultural Sciences (CAAS), China
Copyright © 2021 Wallner, Moulin, Busset, Rimbault and Béna. This is an open-access article distributed under the terms of the Creative Commons Attribution License (CC BY). The use, distribution or reproduction in other forums is permitted, provided the original author(s) and the copyright owner(s) are credited and that the original publication in this journal is cited, in accordance with accepted academic practice. No use, distribution or reproduction is permitted which does not comply with these terms.
*Correspondence: Gilles Béna, Z2lsbGVzLmJlbmFAaXJkLmZy
†Present address: Adrian Wallner, Université de Reims Champagne-Ardenne, RIBP EA4707 USC INRAE 1488, SFR Condorcet FR CNRS 3417, Reims, France