- 1Univ Lyon, Université Claude Bernard Lyon 1, CNRS, INRAe, VetAgro Sup, UMR 5557 Ecologie Microbienne, Villeurbanne, France
- 2Univ Lyon, Université de St Etienne, St Etienne, France
The beneficial effects of plant growth–promoting Rhizobacteria (PGPR) entail several interaction mechanisms with the plant or with other root-associated microorganisms. These microbial functions are carried out by multiple taxa within functional groups and contribute to rhizosphere functioning. It is likely that the inoculation of additional PGPR cells will modify the ecology of these functional groups. We also hypothesized that the inoculation effects on functional groups are site specific, similarly as the PGPR phytostimulation effects themselves. To test this, we assessed in the rhizosphere of field-grown maize the effect of seed inoculation with the phytostimulatory PGPR Azospirillum lipoferum CRT1 on the size and/or diversity of selected microbial functional groups important for plant growth, using quantitative polymerase chain reaction and/or Illumina MiSeq metabarcoding. The functional groups included bacteria able to fix nitrogen (a key nutrient for plant growth), producers of 1-aminocyclopropane-1-carboxylate (ACC) deaminase (which modulate ethylene metabolism in plant and stimulate root growth), and producers of 2,4-diacetylphloroglucinol (an auxinic signal enhancing root branching). To test the hypothesis that such ecological effects were site-specific, the functional groups were monitored at three different field sites, with four sampling times over two consecutive years. Despite poor inoculant survival, inoculation enhanced maize growth. It also increased the size of functional groups in the three field sites, at the maize six-leaf and flowering stages for diazotrophs and only at flowering stage for ACC deaminase and 2,4-diacetylphloroglucinol producers. Sequencing done in the second year revealed that inoculation modified the composition of diazotrophs (and of the total bacterial community) and to a lesser extent of ACC deaminase producers. This study revealed an ecological impact that was field specific (even though a few taxa were impacted in all fields) and of unexpected magnitude with the phytostimulatory Azospirillum inoculant, when considering microbial functional groups. Further methodological developments are needed to monitor additional functional groups important for soil functioning and plant growth under optimal or stress conditions.
Introduction
Plant growth–promoting Rhizobacteria (PGPR) benefit plants mainly by (i) stimulating root system development, thereby allowing seedlings to explore larger soil volumes to gain access to water and mineral nutrients (Vacheron et al., 2013); (ii) enhancing nutrient availability, e.g., via N2 reduction or P solubilization (Dobbelaere et al., 2003; Basu et al., 2021); (iii) improving root system functioning in terms of ion uptake, by stimulating nutrient transporter expressions and/or activities in roots (Bertrand et al., 2000; Vacheron et al., 2013; Pii et al., 2015); and/or (iv) controlling root parasites via competition or antagonism, which leads to parasite inhibition (Raaijmakers et al., 2009; Basu et al., 2021). Effective PGPR strains have increased crop yields in many (but not all) field trials (Okon and Labandera-Gonzalez, 1994; El Zemrany et al., 2006; García de Salamone et al., 2010). Their use as crop inoculants is promising to reduce chemical inputs and improve farming sustainability, despite plant growth-promotion effects that can fluctuate according to the field, the year, or other farming/environmental parameters (Okon and Labandera-Gonzalez, 1994; Dobbelaere et al., 2003; Castro-Sowinski et al., 2007; Raaijmakers et al., 2009; Rozier et al., 2017; Basu et al., 2021).
Azospirillum is an emblematic PGPR genus and is widely used in certain countries to stimulate maize, wheat, and rice (Castro-Sowinski et al., 2007; Rozier et al., 2017; Schmidt and Gaudin, 2018). The main mode of action is the secretion of phytohormones especially indole acetic acid, involved in stimulation of root branching and growth (Steenhoudt and Vanderleyden, 2000). In certain Azospirillum strains, plant hormonal effects may also take place via synthesis of root-branching signal nitric oxide (Creus et al., 2005; Molina-Favero et al., 2008) or deamination of 1-aminocyclopropane-1-carboxylate (ACC), the ethylene precursor in plants (Vacheron et al., 2013; Glick, 2014). Associative nitrogen fixation also occurs in Azospirillum, although its contribution is considered minor (Steenhoudt and Vanderleyden, 2000).
The interaction between Azospirillum and plant leads to major changes in the physiology of both partners. On the bacterial side, more than 400 genes of Azospirillum lipoferum 4B were differentially expressed when the bacterium was in contact with the host plant rather than in the absence of the host (Drogue et al., 2014a). They were involved especially in detoxification of reactive oxygen species and multidrug efflux, which could be important for root colonization. On the plant side, Azospirillum resulted in modified expression of thousands of genes, including many involved in plant defense or ethylene/auxin pathways (Drogue et al., 2014b). Therefore, an Azospirillum inoculant is likely to change the nature or amount of compounds released by roots (i.e., rhizodeposition patterns), and indeed inoculation resulted in physiological changes in terms of rice content in secondary metabolites (Chamam et al., 2013); metabolite profiles of roots, shoots (Brusamarello-Santos et al., 2017), and xylem sap (Rozier et al., 2016) in maize; protein accumulation in maize seedlings (Cangahuala-Inocente et al., 2013); and production of flavonoids by roots of rice (Chamam et al., 2013) and alfalfa (Volpin et al., 1996). These chemical changes may lead to modified ecological conditions for microorganisms residing in the rhizosphere. Therefore, Azospirillum inoculation can be expected to trigger a range of indirect effects on other root-colonizing microorganisms, in addition to direct competition effects with resident rhizosphere populations. However, maize inoculation with A. lipoferum CRT1 caused only modest (but statistically significant) changes at the scale of the whole rhizobacterial community (Baudoin et al., 2009), as typically found also for other Azospirillum inoculants (Herschkovitz et al., 2005; Lerner et al., 2006; Felici et al., 2008; Naiman et al., 2009; Baudoin et al., 2010; García de Salamone et al., 2012; Bao et al., 2013; da Costa et al., 2018; Di Salvo et al., 2018). This may seem surprising considering the pronounced effects of Azospirillum on plant physiology, but perhaps methodological limitations account for these observations. Both direct and indirect microbial interactions between PGPR inoculants and the indigenous microbiota may, in turn, have an impact on rhizosphere functioning (Trabelsi and Mhamdi, 2013; Florio et al., 2017; Di Salvo et al., 2018; Dal Cortivo et al., 2020; Kusstatscher et al., 2020), but this possibility remains poorly documented as microbial functional groups have been neglected. In light of the low reproducibility of plant-beneficial effects of PGPR inoculants in field situations, there is a need to develop our knowledge on the ecological consequences of such inoculations. It is also important because indirect PGPR effects, that is, effects taking place via interactions with indigenous microorganisms, are increasingly considered important for phytostimulation (Trabelsi and Mhamdi, 2013; Vacheron et al., 2013; Ambrosini et al., 2016; Di Salvo et al., 2018; Kusstatscher et al., 2020). In other words, it might be that the low reproducibility of phytostimulation performance in fields could result, in part, from a low reproducibility of the interactions between microbial inoculants and resident microorganisms. To explore this possibility, a prerequisite is to understand the variability of PGPR effects on the indigenous microbiota, as the latter displays spatiotemporal heterogeneity in field conditions (Baudoin et al., 2009; Raaijmakers et al., 2009; García de Salamone et al., 2010; Schmidt and Gaudin, 2018; Kusstatscher et al., 2020; Renoud et al., 2020).
Microbial functioning of the rhizosphere involves a broad range of particular ecological functions carried out by functional groups, for example, nitrogen fixers, phytohormone producers, and so on. Often, each functional group comprised multiple taxa, which may contribute differently to the corresponding ecological function. In addition, the effects of environmental conditions (climate, soil type, plant genotype and phenology, etc.) are likely to differ from one functional group to the other (Fuhrman, 2009; Reed et al., 2011; Schimel and Schaeffer, 2012; Renoud et al., 2020). Therefore, it is not feasible to infer the impact of PGPR inoculation on rhizosphere-relevant microbial functional groups based solely on our knowledge of inoculant impact on the taxonomic composition of the rhizomicrobial community (Trabelsi and Mhamdi, 2013; Ambrosini et al., 2016). Here, we tested the hypotheses that (i) PGPR inoculation can lead to major changes in root-associated functional microbial communities important for plant development/growth, and (ii) these PGPR effects on functional communities may differ in a field site-specific manner.
To address this issue, we assessed the effect of maize seed inoculation with A. lipoferum CRT1 on the size (i.e., the number of microbial cells) and/or diversity (i.e., richness, genetic structure, and composition) of three microbial functional groups, that is, nitrogen fixers (using nifH marker), ACC deaminase producers (acdS marker), and producers of 2,4-diacetylphloroglucinol, a root-branching signal (Brazelton et al., 2008) that may also act as an antimicrobial compound if present at high concentration (Keel et al., 1990) (phlD marker). These functional groups were selected based on (i) their importance for phytostimulation (Dobbelaere et al., 2003; Brazelton et al., 2008; Vacheron et al., 2013; Schmidt and Gaudin, 2018); for example, N is a key nutrient for plant, and therefore, N fixation is a key aspect of rhizosphere functioning; (ii) the availability of knowledge on their mode of action and corresponding regulatory processes (Keel et al., 1990; Steenhoudt and Vanderleyden, 2000; Dobbelaere et al., 2003; Brazelton et al., 2008; Vacheron et al., 2013; Glick, 2014; Rozier et al., 2017); (iii) the possibility to monitor them by focusing on a single gene marker shared by all members of the functional group, that is, nifH, acdS, and phlD, respectively (Vacheron et al., 2013; Renoud et al., 2020); (iv) the possibility to infer taxonomic affiliations of individual functional group members from marker gene sequences (for nitrogen fixers and ACC deaminase producers) (Renoud et al., 2020); and (v) the availability of validated polymerase chain reaction (PCR) methodology to enable monitoring in soil systems (Poly et al., 2001; Bouffaud et al., 2016, 2018; Renoud et al., 2020). For instance, auxin synthesis and phosphate solubilization were not considered despite their ecological significance, as they rely on several different pathways, some of them probably not yet documented, which restricts the possibilities of monitoring. It is important to note that the inoculant used here (i.e., A. lipoferum CRT1) belongs to nitrogen fixers but not to ACC deaminase producers or 2,4-diacetylphloroglucinol producers.
To this end, the PGPR A. lipoferum CRT1 was used as maize seed inoculant in two consecutive years, at three field sites located in the region of Lyon, France, and corresponding to a luvisol (termed L; near Chatonnay), a fluvic cambisol (FC; near Sérézin-de-la-Tour), and a calcisol (C; near Saint Savin), and its impact was investigated by quantitative PCR (qPCR) and/or Illumina MiSeq-based metabarcoding. In addition, maize was grown under optimized or suboptimal conditions of mineral N fertilization to assess the significance of N limitation (nutriment stress) as a factor possibly modulating inoculation effects, based on measurements of the size of the three microbial functional groups. Monitoring was carried out at the six-leaf stage of growth (i.e., six leaves are produced, and maize now relies on its permanent root system rather than the seminal roots) and at flowering time, two key stages to assess maize functioning and performance (Gabriel et al., 2017; Sarabia et al., 2018).
Materials and Methods
Field Trials
The field experiments were run in 2014 and 2015 in Chatonnay (L), Sérézin-de-la-Tour (FC), and Saint Savin (C), located near Bourgoin-Jallieu (France). The soils are a luvisol (L), a fluvic cambisol (FC), and a calcisol (C) based on FAO soil classification, and chemical features are given in Supplementary Table 1.
These field experiments have been described (Rozier et al., 2017). At each location, the crop rotation includes 1 year of wheat (grown prior to the 2014 trial), 6 years of maize (starting with the 2014 and 2015 trials), and 1 year of rapeseed. Seeds of maize (Zea mays cv. Seiddi; provided by the Dauphinoise coop company, France) were sown on April 18 (at FC) and 23 (at C and L) in 2014 and on April 30 (C) and May 11 (FC and L) in 2015. The fields were not irrigated.
Three levels of mineral nitrogen fertilization were considered, that is, X (optimized N fertilization in one application carried out at the six-leaf stage), XS (the same N fertilization level but split into two applications), and 0 (no fertilizer). In 2014, the effect of inoculation with A. lipoferum CRT1 was studied at each of the three levels of mineral nitrogen fertilization (X, XS, and 0) for site FC and two levels (X and 0) for sites L and C, using a factorial design with, respectively, six or four combinations of factors (i.e., inoculation or no inoculation × three or two mineral N levels). The optimal dose X for maize (based on local agronomic assessments) was 120 kg mineral N/ha for sites FC and C and 180 kg mineral N/ha for site L. On site FC, the XS nitrogen treatment corresponded to the X fertilizer dose applied half at sowing and half on May 21 (at the six-leaf stage). Individual replicate plots were 12 (FC and C) or 8 (L) maize rows wide and 12 m long. They were organized along a randomized block design with five blocks. Mineral nitrogen fertilizer was applied on May 21 (FC and C) and 22 (L) for fertilized plots.
In 2015, CRT1 inoculation was studied at two N levels (X and 0). Inoculation (or not) and mineral N level were applied to the same plots that had received these applications the year before. In 2015, mineral nitrogen fertilizer was applied on June 5 at C and June 9 at FC and L (at the six-leaf stage).
Inoculum Preparation and Inoculant Monitoring
A. lipoferum CRT1 was isolated in France from the rhizosphere of field-grown maize (Fages and Mulard, 1988) and promoted maize growth when used as inoculant (Fages and Mulard, 1988; Jacoud et al., 1999; Walker et al., 2011; Rozier et al., 2017, 2019). For inoculation, maize seeds were mixed with CRT1 cells included in a sterile peat-based formulation supplied by Agrauxine (Lesaffre Plant care; Angers, France), and the exact same peat-based seed formulation (but without any CRT1 cell added) was used in the non-inoculated control.
Inoculant survival in the rhizosphere was assessed by qPCR, using CRT1-specific primers Q1/Q2 (Couillerot et al., 2010). For quantification on seeds at sowing, inoculum level was also estimated by colony counts on nitrogen-free agar containing 0.2 g/L ammonium chloride and Congo red (Cáceres, 1982). At sowing, the inoculant was recovered in 2014 at 2.0 × 102 colony-forming units (CFUs) (and equivalent to 6.0 × 103 cells by qPCR) per seed, and in 2015 at 3.7 × 102 CFUs (and equivalent to 3.0 × 104 cells) per seed at sites L and FC, and 8.8 × 102 CFUs (and equivalent to 1.5 × 105 cells) per seed at site C.
Sampling of Plants and DNA Extractions
In both years, maize was sampled at six-leaf and flowering stages, as described (Renoud et al., 2020). In 2014, all plots were studied. The six-leaf stage was sampled on May 25 (FC) and 26 (C and L), shortly after fertilizer application. On each plot, six plants were randomly chosen; their entire root system was dug up and shaken vigorously to dislodge loosely adhering soil. At sites FC and C, one pooled sample (i.e., six-root system) was obtained per plot, which made five pooled samples per treatment. At site L, each root system was treated individually to consider variations from one plant to the other, which made 6 root system × 5 plots = 30 samples per treatment. The sampling at flowering stage was done on July 8 (FC and C) and 9 (L), and six plants were sampled per plot and treated individually, which made 30 samples per treatment.
The moderate levels of plant-to-plant variation in 2014 prompted us to reduce samplings to four root systems per plot in 2015. In 2015, six-leaf maize was sampled on May 27 (C), June 5 (FC), and June 8 (L), and only non-fertilized plots were studied because fertilizers had not been applied yet. The flowering stage sampling was done on July 15 (C), 16 (FC), and 17 (L), and all plots were studied. At each sampling, the four root systems sampled per plot were treated individually, which made 20 samples per treatment.
Each root system sample was flash-frozen on the field, using liquid nitrogen, and lyophilized at the laboratory (24 h at –50°C), as described (Renoud et al., 2020). Briefly, roots and their adhering soil (i.e., rhizosphere; approximately 1 mm from root surface) were separated using brushes, and root-adhering soil was stored at –80°C. DNA was extracted from the latter using the FastDNA SPIN kit (BIO 101 Inc., Carlsbad, CA, United States). A total of 500-mg (for the 2014 pooled samples from FC and C) or 300-mg samples (for the other samples) were transferred in Lysing Matrix E tubes, as well as 5 μL of the internal standard APA9 (at 109 copies/mL, with primers AV1f/AV1r) in order to normalize the efficiency of DNA extraction between rhizosphere samples (Park and Crowley, 2005; Couillerot et al., 2010). After 1-h incubation at 4°C, DNA was extracted and eluted in 50 mL of sterile ultrapure water, according to the manufacturer’s instructions, and DNA concentration assessed using Picogreen (Thermo Fisher Scientific).
Quantitative Polymerase Chain Reaction Analysis of Microbial Functional Groups
The number of genes nifH, acdS, and phlD in the rhizosphere was assessed by qPCR using, respectively, primers polF/polR (Poly et al., 2001; Gaby and Buckley, 2017), acdSF5/acdSR8 (Bouffaud et al., 2018), and B2BF/B2BR3 (Almario et al., 2013). The nifH primers polF/polR have been advocated for combined qPCR and diversity analyses (Bouffaud et al., 2016; Gaby and Buckley, 2017), whereas the acdS primers acdSF5/acdSR8 have been validated for analysis of true acdS genes (i.e., without amplifying related D-cystein desulfhydrase genes coding for other types of PLP-dependent enzymes; Bouffaud et al., 2018). Methods are described in Supplementary File 1 (see also Renoud et al., 2020 for some of the genes).
Sequencing Analysis of nifH, acdS, and All Rhizobacteria
Sequencing was carried out using maize at the six-leaf stage (i.e., prior to N fertilizer applications) in 2015, as described (Renoud et al., 2020). Briefly, equimolar composite samples of four rhizosphere DNA extracts (from four plants) were used per plot, that is, 5 plots × 3 fields = 15 samples per treatment. Illumina MiSeq sequencing (paired-end reads; 2 × 300 bp for nifH and rrs, 2 × 125 bp for acdS) was performed by MR DNA laboratory (Shallowater, TX, United States).1
nifH, acdS, and rrs sequencings were done using, respectively, primers polF/polR, acdSF5/acdSR8, and 515/806 targeting the V4 variable region (Yang et al., 2016; Renoud et al., 2020). All forward primers carried a barcode. The 30-cycle PCR (five cycles implemented on PCR products) was performed using the HotStarTaq Plus Master Mix Kit (Qiagen, Valencia, CA, United States) with the following conditions: 94°C for 3 min, followed by 28 cycles of 94°C for 30 s, 53°C for 40 s, and 72°C for 1 min, and a final elongation step at 72°C for 5 min. PCR products were checked in 2% (wt/vol) agarose gel to verify amplification success and relative band intensity. For each gene, the amplicons of the 15 samples were multiplexed (in equal proportions based on their molecular weight and DNA concentrations) and subsequently purified using calibrated Ampure XP beads prior to preparing a DNA library following Illumina TruSeq DNA library preparation protocol. Reads have been deposited in the European Bioinformatics Institute (EBI) database under accession numbers PRJEB14346 (nifH), PRJEB14343 (acdS), PRJEB14347 (rrs).
Sequence data were processed using the analysis pipeline of MR DNA (Renoud et al., 2020). Briefly, sequences were depleted of barcodes, the sequences < 150 bp or with ambiguous base calls were removed, the remaining sequences denoised, operational taxonomic units (OTUs; arbitrarily defined at 3% divergence threshold for the three genes) generated, and chimeras removed. Final OTUs from the nifH and rrs sequencing were taxonomically classified using BLASTn against a curated database derived from Greengenes (DeSantis et al., 2006), RDPII,2 and NCBI.3 Final OTUs of the acdS sequencing were classified using an in-house curated acdS database (Bouffaud et al., 2018). Datasets without singletons (here, singleton sequences are those found a single time among the 30 sequenced samples from inoculated or control treatments) were used to generate rarefaction curves and diversity indices of Shannon (H) and Simpson (calculated using sequencing subsample data for which each sample had the same number of sequences). Some of the sequences were used previously to describe microbial diversity of non-inoculated maize (Renoud et al., 2020).
Statistical Analyses
Quantitative PCR data were compared by two-factor analysis of variance (i.e., inoculation × fertilizer treatment) and Fisher least significant difference tests, using log-transformed data.
Comparisons of bacterial diversity were carried out by between-class analysis (BCA) (Dolédec and Chessel, 1987) with the ADE4 (Chessel et al., 2004; Dray et al., 2007) and ggplot2 packages for R. BCA is a robust alternative to linear discriminant analysis (Huberty, 1994) when the number of samples is small compared with the number of predictors. When the number of samples is low, and particularly when it is lower than the number of predictors, discriminant analysis cannot be used. In this case, BCA can be very useful and provides illustrative graphical displays of between-group differences (Thioulouse et al., 2012). The significance of BCA results was assessed using a Monte Carlo test with 10,000 permutations (null hypothesis: absence of difference between groups). Non-metric multidimensional scaling (NMDS) was also performed, using the Bray–Curtis distance and the vegan package for R.
Two analyses were carried out to assess the impact of inoculation on particular genera. First, the genera contributing most to treatment differentiation (independently of their abundance) were identified by BCA; the position in the heatmap indicates the statistical importance of these taxa in positioning samples on the BCA axis with and without inoculation, these taxa being classified according to their importance in contributing to the construction of the BCA axis. As a complementary approach, the genus composition of the bacterial community and functional groups were characterized to consider the relative abundance of the most prevalent taxa. Unless otherwise stated, statistical analyses were performed using R version 3.3.2 at p < 0.05.4
Results
Effects of Inoculation on Maize Under Field Conditions
The three field trials have been described before (Rozier et al., 2017). The seed inoculant A. lipoferum CRT1 was under the detection threshold (of 4.0 × 103 cell equivalents per gram of rhizosphere soil) at the stages sampled in the current work, that is, when maize had produced six leaves and at flowering time, in all three field sites studied and in both years. Previous results from these three fields indicated that seed inoculation had resulted in significant changes in (i) maize morphological properties at the six-leaf stage (which, in 2015, took place mainly at site L; Table 1), (ii) plant photosynthetic efficiency except at site L in 2015, (iii) metabolome (studied only in 2015) of maize roots at sites L and C as well as shoots at sites L and FC (i.e., contents of 13 metabolites in roots and 28 metabolites in leaves were significantly affected, with glutamine content modified both in roots and shoot at site FC), and/or (iv) yield at site L (only in 2015, with a significant positive effect), site FC (with a positive effect in 2014 and a negative effect in 2015), and site C (with a significant negative effect in 2014 and a trend for a 16% yield increase in 2015 but that was not statistically significant at p < 0.05) (Rozier et al., 2017). Therefore, inoculation effects were statistically significant but fluctuated according to field and year, which provided suitable experimental conditions in this work to assess field-level variability of inoculation impact on the rhizosphere microbiome.
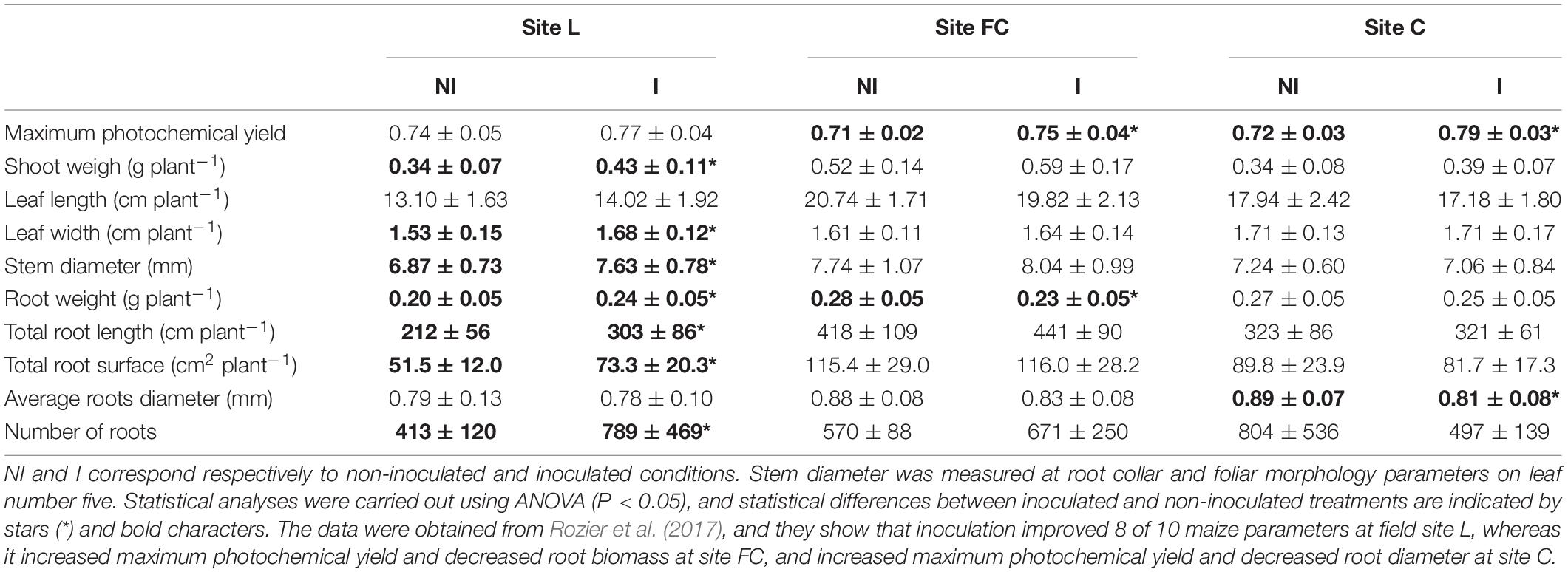
Table 1. Effect of Azospirillum lipoferum CRT1 on root and shoot parameters of maize at 6 leaves in 2015 (i.e., the sampling date at which metabarcoding was implemented) in field sites L, FC, and C.
Impact of Inoculation on Numbers of nifH, acdS, and phlD Rhizobacteria in Each Field
At the six-leaf stage in 2014, differences in the size of the nifH group between inoculated and non-inoculated maize were not significant at sites FC and C. At site L, however, inoculation resulted in statistically higher number of nifH bacteria in non-fertilized plots (nutriment stress) but lower number in fertilized plots (Table 2). At the six-leaf stage in 2015, the number of nifH bacteria (studied in non-fertilized plots) was statistically lower at site C upon inoculation, but no difference was found at the two other sites. At flowering stage in 2014, the size of the nifH group was statistically higher for inoculated than non-inoculated maize at sites L (with or without X-level fertilization) and FC (with XS-level fertilization) but not at site C. At flowering stage in 2015, a significant increase in the number of nifH bacteria following inoculation was found again, at sites FC (this time in both fertilization treatments) and C (in non-fertilized plots) (Table 2).
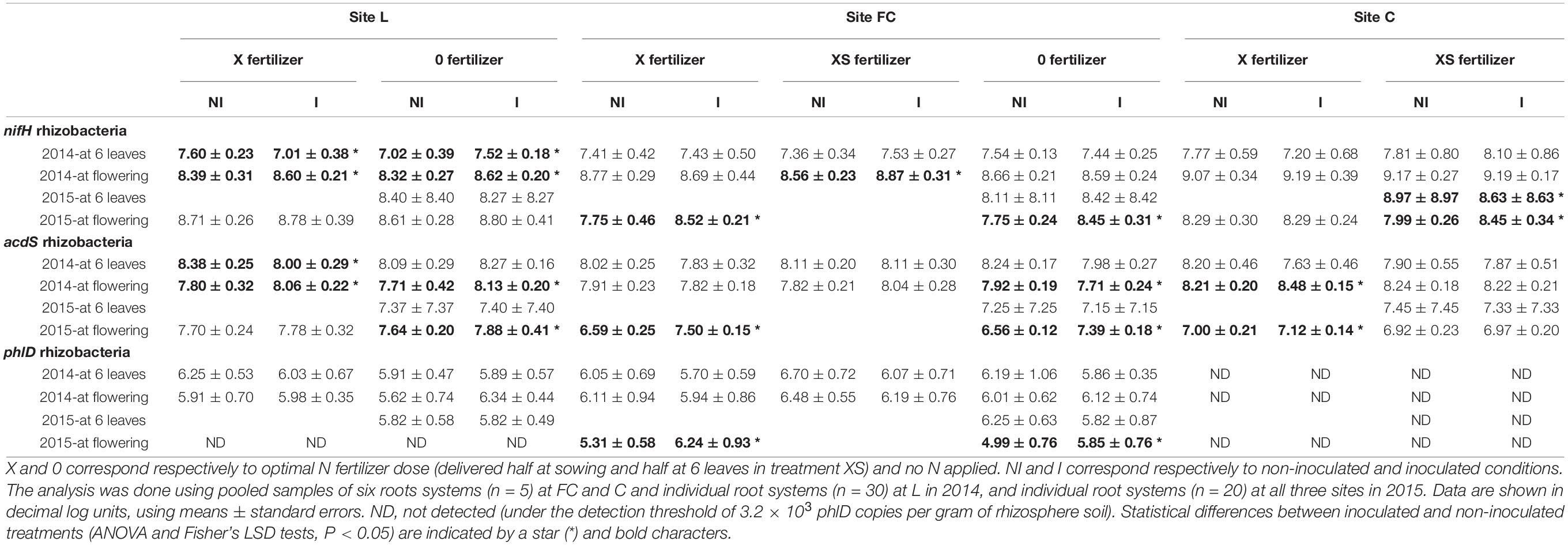
Table 2. Effect of seed inoculation with Azospirillum lipoferum CRT1 on the size of the nifH, acdS, and phlD functional groups in the maize rhizosphere at 6-leaf stage and flowering at three field sites L, FC, and C in 2014 and 2015.
At the six-leaf stage in 2014, the size of the acdS group was statistically higher for inoculated than non-inoculated maize at site L in fertilized plots, but not in non-fertilized plots or at the other two sites (Table 2). There was no difference at the six-leaf stage in 2015. At flowering stage in 2014, a significant increase in the number of acdS bacteria following inoculation was found at sites L (in both fertilization treatments) and FC (with XS-level fertilization), whereas a decrease was observed at site FC (in non-fertilized plots). At flowering stage in 2015, the size of the acdS group was statistically higher for inoculated than non-inoculated maize at sites L (in non-fertilized plots), FC (in both fertilization treatments), and C (in fertilized plots).
At the six-leaf stage, there was no difference in the size of the phlD group between inoculated and non-inoculated maize, regardless of the site or the year (Table 2). At flowering stage in 2014, a significant increase in the number of phlD bacteria following inoculation was found at site L in the absence of fertilization, but there was no difference at the other site studied (FC). At flowering stage in 2015, the size of the phlD group was statistically higher with inoculated than non-inoculated maize at site FC, in both fertilization treatments.
In summary, when considering the four samplings carried out over the 2 years of the study, statistically significant differences in the size of functional groups resulting from inoculation were found in 8 of 19 comparisons at site L (4 of 7 for nifH, 4 of 7 for acdS, 0 of 5 for phlD), 8 of 27 comparisons at FC (3 of 9 for nifH, 3 of 9 for acdS, 2 of 9 for phlD), and 4 of 14 comparisons at C (2 of 7 for nifH, 2 of 7 for acdS), depending on year, maize growth stage, and restricted N fertilization level. Within a given field site, some but not all of the differences observed for various functional groups took place for the same comparisons, that is, at the same combinations of sampling date × N fertilization level.
Impact of Inoculation on Diversity of nifH, acdS, and All Rhizobacteria in Each Field
nifH sequencing of six-leaf maize rhizosphere (prior to N fertilizer applications) in 2015 gave 681,088 sequences, corresponding to 28,475 OTUs. The rarefaction curves reached a plateau (Supplementary Figure 1A), indicating that most of the nifH diversity had been recovered. Subsampling was done with 10,775 sequences per sample. There was no significant difference when comparing the resulting diversity indices between inoculated and non-inoculated maize, regardless of the index (Shannon or Simpson) and field site (Figure 1).
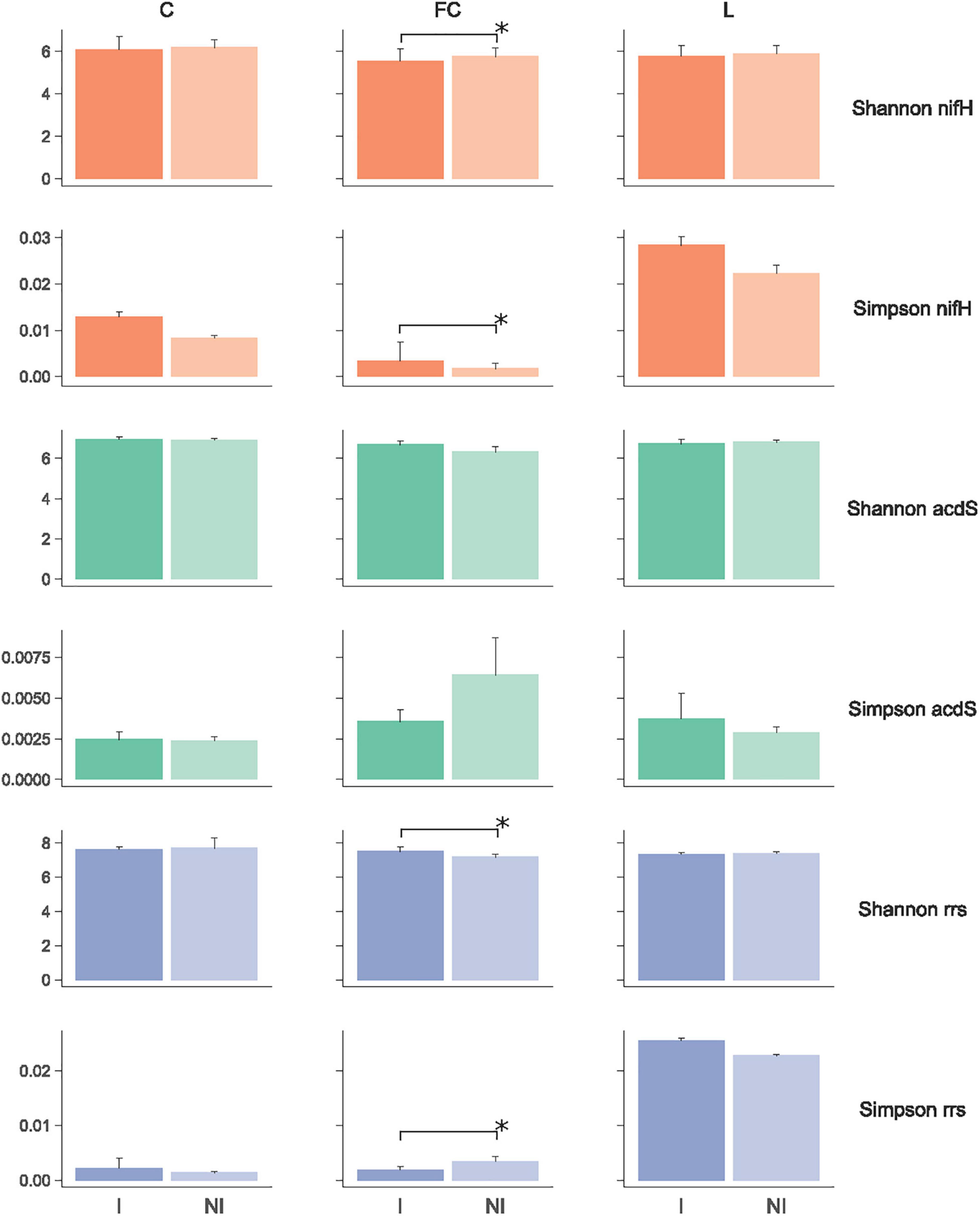
Figure 1. Diversity indices of nifH and acdS functional groups and the entire (rrs) bacterial community at sites L, FC, and C. NI and I correspond, respectively, to non-inoculated and inoculated conditions. Data are shown using means ± standard deviations (n = 5). Statistical analyses were carried out by Wilcoxon test between inoculated and non-inoculated conditions, and differences are shown with an asterisk (p < 0.05).
acdS sequencing resulted in 2,883,839 sequences, which gave 31,220 OTUs. Rarefaction analysis showed that the curves reached a plateau (Supplementary Figure 1B). Subsampling was implemented with 68,376 sequences per sample. At site FC, inoculated maize gave a significantly higher Shannon index (6.69 vs. 6.32; p = 0.032) but a lower Simpson index (3.6 × 10–3 vs. 6.4 × 10–3; p = 0.032) in comparison with non-inoculated maize. Inoculation had no effect on diversity level at the other sites (Figure 1).
rrs sequencing was also performed to determine whether inoculation effects could also take place at the scale of the whole rhizobacterial community. A total of 3,048,495 reads were obtained, corresponding to 38,419 OTUs. Rarefaction analysis indicated that curves reached a plateau (Supplementary Figure 1C). Subsampling was done with 51,696 sequences per sample. As for acdS, inoculated maize at site FC led for rrs data to a significantly higher Shannon index (7.52 vs. 7.19; p = 0.032) but a lower Simpson index (1.9 × 10–3 vs. 3.4 × 10–3; p = 0.046) in comparison with non-inoculated maize. Inoculation had no effect on diversity level at the other sites (Figure 1).
In summary, inoculation resulted in statistically significant differences in diversity indices for two of three metabarcoding comparisons at site FC (i.e., for acdS and rrs) and none of the six other metabarcoding comparisons (at sites L and C).
Impact of Inoculation on Taxa Composition in Each Field
NMDS evidenced that the main differences in the nifH, acdS, and rrs datasets of six-leaf maize were due to field site particularities, with inoculation impact apparent mainly at site FC based on rrs data (Supplementary Figure 2C). To unmask the overriding effects of field conditions, the impact of inoculation was assessed by BCA. Indeed, BCA of nifH sequences from 2015’s six-leaf maize indicated that the composition of the diazotroph community differed statistically at site L (p = 0.008), at site FC (p = 0.007), and at site C (p = 0.006) following inoculation with A. lipoferum CRT1 (Figures 2A–C). Similarly, BCA of acdS sequences from 2015’s six-leaf maize showed that the composition of ACC-deaminating rhizobacteria differed following inoculation with A. lipoferum CRT1, at sites L (p = 0.012), FC (p = 0.005), and C (p = 0.013) (Figures 3A–C). Finally, BCA of rrs sequences from 2015’s six-leaf maize indicated that the composition of rhizobacteria differed following inoculation with A. lipoferum CRT1, at sites L (p = 0.005), FC (p = 0.006), and C (p = 0.005) (Figures 4A–C).
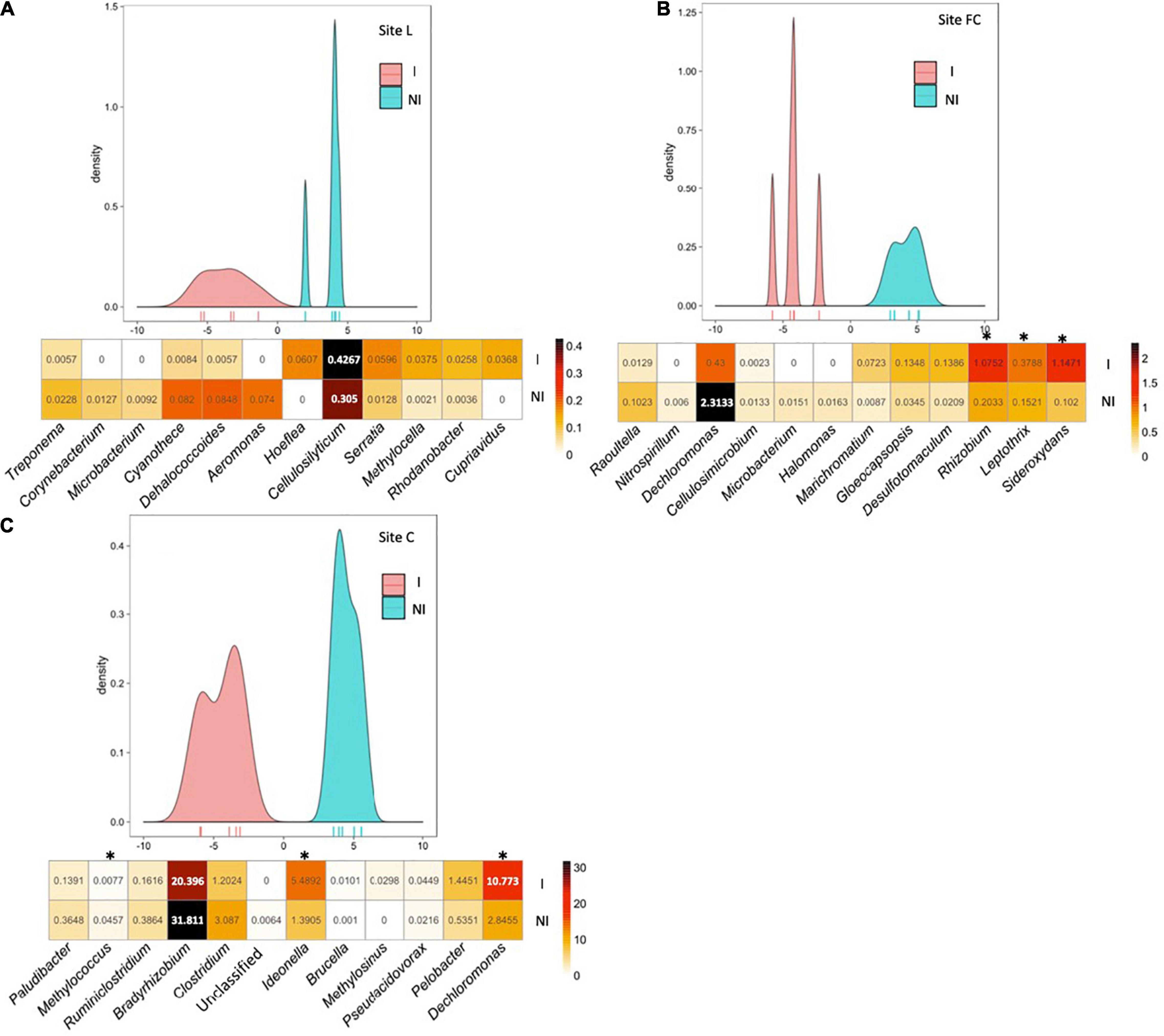
Figure 2. Between-class analysis of the effect of seed inoculation with A. lipoferum CRT1 on the composition of the nifH functional group in the maize rhizosphere at the six-leaf stage at three field sites L (A), FC (B), and C (C), in 2015. NI and I correspond, respectively, to non-inoculated and inoculated conditions. For each site, the BCA graph is completed (below) by the distribution of the bacterial OTUs according to their contribution to treatment differentiation; BCA is supported statistically by a Monte Carlo test at sites L (p = 0.008), FC (p = 0.007), and C (p = 0.006), and the heatmap below indicates the average relative proportions for the 12 bacterial genera most impacted by inoculation in maize rhizosphere. Six bacterial genera most commonly associated with inoculation are indicated at the left, as well as six bacterial genera most often associated with the non-inoculated control (right). Statistical differences between inoculated and non-inoculated treatments are indicated by stars (*) (Wilcoxon test, p < 0.05). Heatmap unit is a percentage of the average relative proportion of taxa.
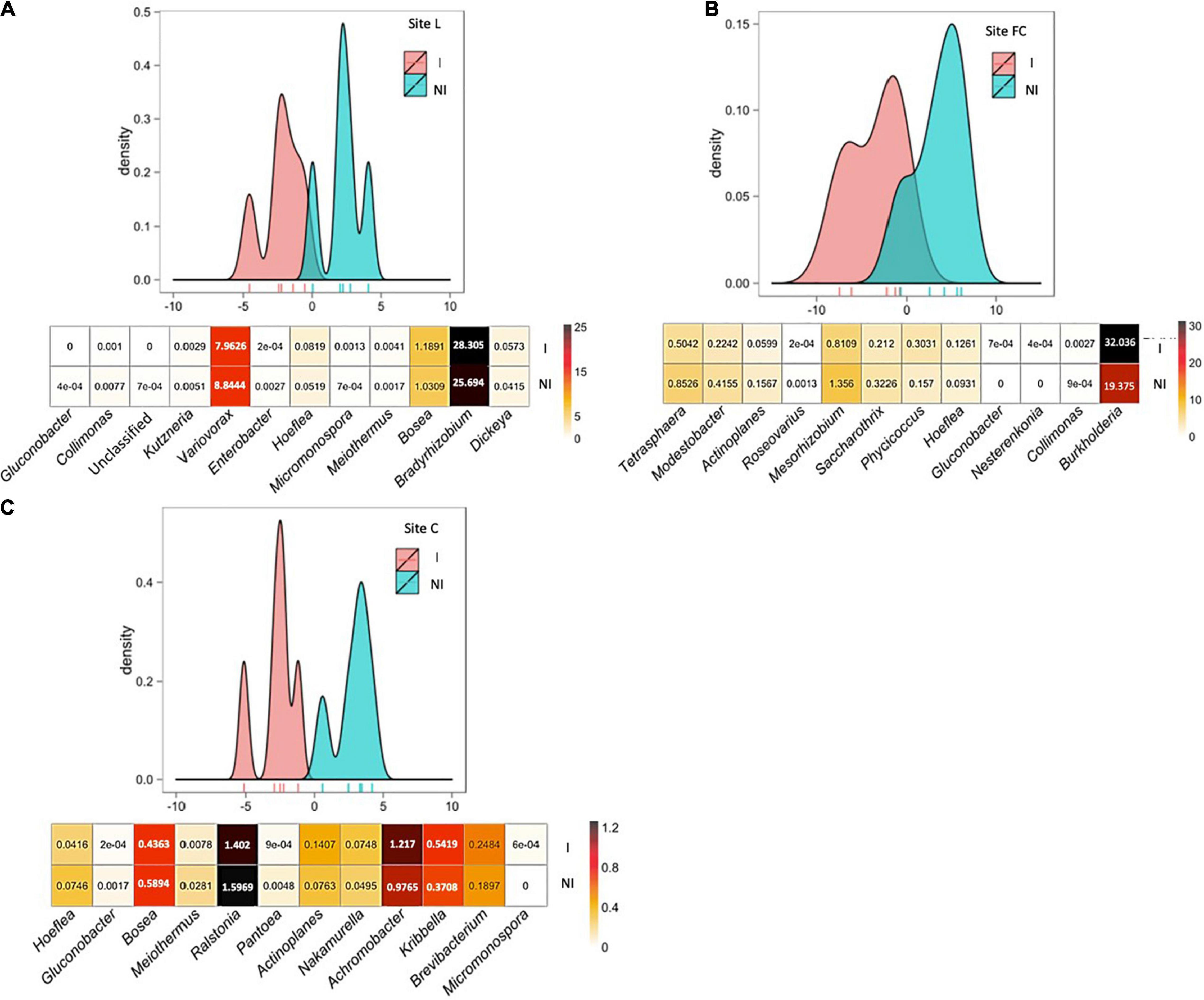
Figure 3. Between-class analysis of the effect of seed inoculation with A. lipoferum CRT1 on the composition of the acdS functional group in the maize rhizosphere at the six-leaf stage at three field sites L (A), FC (B), and C (C), in 2015. NI and I correspond, respectively, to non-inoculated and inoculated conditions. For each site, the BCA graph is completed (below) by the distribution of the bacterial OTUs according to their contribution to treatment differentiation; BCA is statistically supported by a Monte Carlo test at sites L (p = 0.012), FC (p = 0.005), and C (p = 0.013), and the heatmap below indicates the average relative proportions for the 12 bacterial genera most impacted by inoculation in maize rhizosphere. Six bacterial genera most commonly associated with inoculation are indicated at the left, as well as six bacterial genera most often associated with the non-inoculated control (right). Differences between inoculated and non-inoculated treatments were not statistically significant (Wilcoxon test, p < 0.05). Heatmap unit is a percentage of the average relative proportion of taxa.
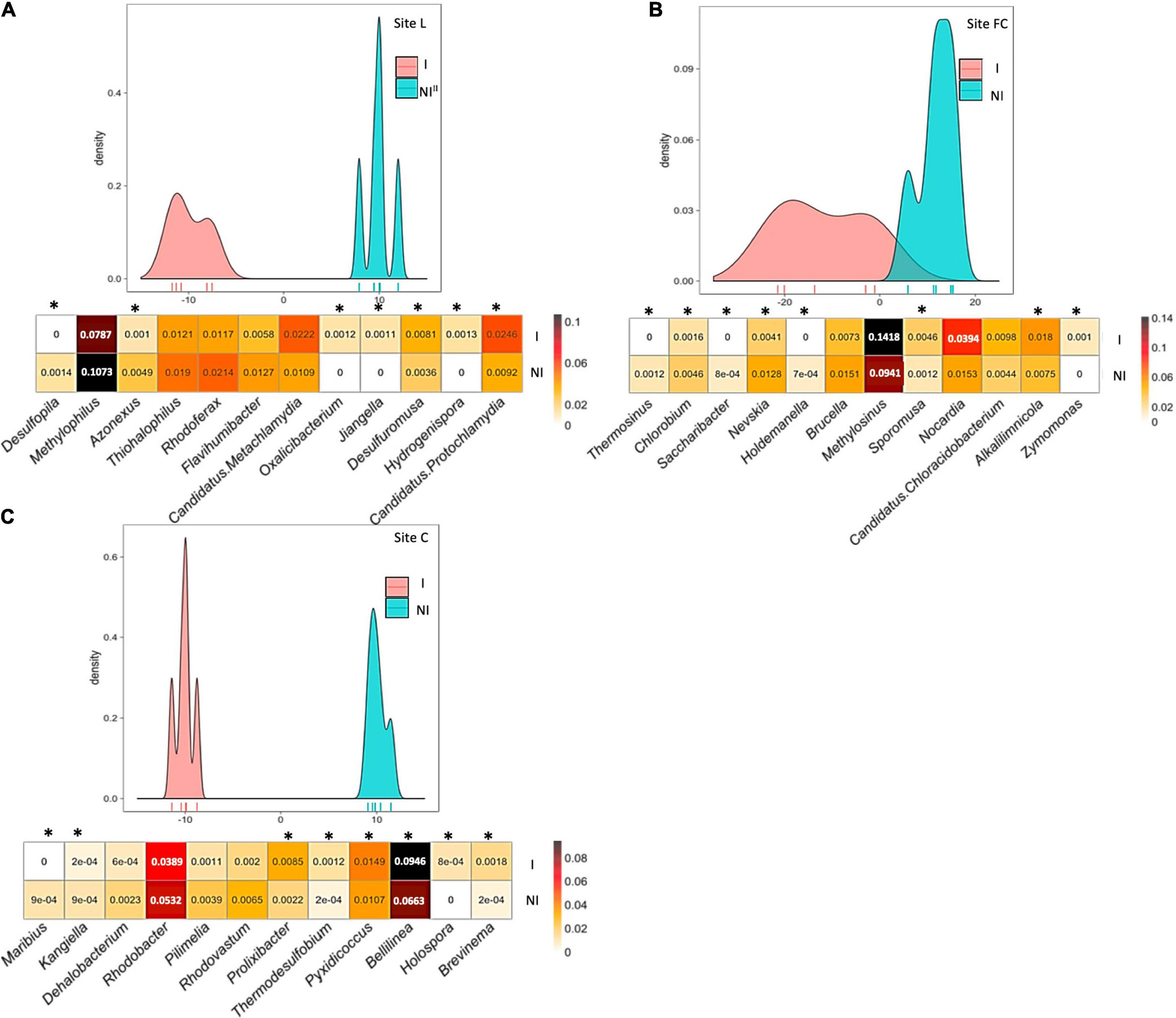
Figure 4. Between-class analysis of the effect of seed inoculation with A. lipoferum CRT1 on the composition of the total bacterial community in the maize rhizosphere at the six-leaf stage at three field sites L (A), FC (B), and C (C), in 2015. NI and I correspond, respectively, to non-inoculated and inoculated conditions. For each site, the BCA graph is completed (below) by the distribution of the bacterial OTUs according to their contribution to treatment differentiation; BCA is statistically supported by a Monte Carlo test at sites L (p = 0.005), FC (p = 0.006), and C (p = 0.005), and the heatmap below indicates the relative proportions for the 12 bacterial genera most impacted by inoculation in maize rhizosphere. Six bacterial genera most commonly associated with inoculation are indicated at the left, as well as six bacterial genera most often associated with the non-inoculated control (right). Statistical differences between inoculated and non-inoculated treatments are indicated by stars (*) (Wilcoxon test, p < 0.05). Heatmap unit is a percentage of the average relative proportion of taxa.
BCA of nifH data showed that the six taxa most often associated with inoculation and the six taxa most commonly associated with the non-inoculated control displayed different rhizosphere levels overall between inoculated and non-inoculated maize. At site L, bacterial genera contributing most to the difference were (by decreasing order of importance) Treponema, Corynebacterium, Microbacterium, Cyanothece, Dehalococcoides, and Aeromonas (genera most commonly associated with inoculated maize), as well as Hoeflea, Cellulosilyticum, Serratia, Methylocella, Rhodanobacter, and Cupriavidus (genera most commonly associated with non-inoculated maize). At site FC, bacterial genera contributing most to the difference were Raoultella, Nitrospirillum, Dechloromonas, Cellulosimicrobium, Microbacterium, and Halomonas (genera most commonly associated with inoculated maize), as well as Marichromatium, Gloeocapsopsis, Desulfomaculum, Rhizobium, Leptothrix, and Sideroxydans (genera most commonly associated with non-inoculated maize). At site C, bacterial genera contributing most to the difference were Paludibacter, Methylococcus, Ruminiclostridium, Bradyrhizobium, Clostridium and an unclassified genus (genera most commonly associated with inoculated maize), as well as Ideonella, Brucella, Methylosinus, Pseudacidovorax, Pelobacter, and Dechloromonas (genera most commonly associated with non-inoculated maize).
BCA of acdS data indicated that the 12 most discriminating taxa displayed different rhizosphere levels overall between inoculated and non-inoculated maize at the sites. At site L, bacterial genera contributing most to the difference were Gluconobacter, Collimonas, an unclassified genus, Kutzneria, Variovorax, and Enterobacter (genera most commonly associated with inoculated maize); as well as Hoeflea, Micromonospora, Meiothermus, Bosea, Bradyrhizobium, and Dickeya (genera most commonly associated with non-inoculated maize). At site FC, bacterial genera contributing most to the difference were Tetrasphaera, Modestobacter, Actinoplanes, Roseovarius, Mesorhizobium, and Saccharothrix (genera most commonly associated with inoculated maize), as well as Phycicoccus, Hoeflea, Gluconobacter, Nesterenkonia, Collimonas, and Burkholderia (genera most commonly associated with non-inoculated maize). At site C, bacterial genera contributing most to the difference were Hoeflea, Gluconobacter, Bosea, Meiothermus, Ralstonia, and Pantoea (genera most commonly associated with inoculated maize), as well as Actinoplanes, Nakamurella, Achromobacter, Kribbella, Brevibacterium, and Micromonospora (genera most commonly associated with non-inoculated maize).
BCA of rrs data revealed that the 12 most discriminating taxa displayed different rhizosphere levels overall between inoculated and non-inoculated maize. At site L, bacterial genera contributing most to the difference were Desulfopila, Methylophilus, Azonexus, Thiohalophilus, Rhodoferax, and Flavihumibacter (genera most commonly associated with inoculated maize), as well as Candidatus Metachlamydia, Oxalicibacterium, Jjiangella, Desulfuromusa, Hydrogenispora, and Candidatus Protochlamydia (genera most commonly associated with non-inoculated maize). At site FC, bacterial genera contributing most to the difference were Thermosinus, Chlorobium, Saccharibacter, Nevskia, Holdemanella, and Brucella (genera most commonly associated with inoculated maize), as well as Methylosinus, Sporomusa, Nocardia, Candidatus Chloracidobacterium, Alkalilimnicola, and Zymomonas (genera most commonly associated with non-inoculated maize). At site C, bacterial genera contributing most to the difference were Maribius, Kangiella, Dehalobacterium, Rhodobacter, Pilimelia, and Rhodovastum (genera most commonly associated with inoculated maize), as well as Prolixibacter, Thermodesulfobium, Pyxidicoccus, Bellilinea, Holospora, and Brevinema (genera most commonly associated with non-inoculated maize).
Despite these rhizomicrobiota modifications, individual comparisons (Wilcoxon test) in the relative abundance of particular genera between inoculated and non-inoculated conditions did not show systematically a statistically significant change, due to low sample number (n = 5), high number of variables (e.g., n = 1,111 genera for the entire community; with the corresponding probability correction effect), and the fact that taxa found in only one of the two treatments were not necessarily found in all five replicates of that treatment. However, the comparison of genus composition data (Supplementary Figure 3) proved useful to emphasize trends among the most prevalent genera in functional groups (but not in the rhizobacterial community as a whole). Among the diazotrophs, there were trends for Azospirillum, Bradyrhizobium, Geobacter (at all three sites), Desulfovibrio (at sites L and FC), Skermanella, Clostridium, and Rhizobium (at site L) being more prevalent in inoculated maize, and for Dechloromonas (at all three sites), Ruminiclostridium (at sites L and FC), Azoarcus, Pseudomonas, Leptothrix, Cellulosilyticum, Ideonella (at site L), and Sideroxydans (at site FC) being less prevalent in inoculated maize. However, contrasted trends were observed for Paenibacillus (prevalence in inoculated maize higher at sites FC and C but lower at site L), Pelosinus (higher at L and C but lower at FC), and Clostridium, Skermanella, and Rhizobium (all three higher at L but lower at, respectively, FC, C, and both FC and C). Among the ACC deaminase producers, there was a trend for Variovorax (at site FC) being more prevalent in inoculated maize, and for Burkholderia, Bradyrhizobium (at site FC; thus, the trend for acdS+ Bradyrhizobium at FC was opposite to the trend for the entire genus; see above), and Methylibium (at site C) being less prevalent in inoculated maize.
In summary, inoculation with A. lipoferum CRT1 resulted in significantly different compositions of the diazotroph community, the ACC-deaminating community, and the global bacterial community, at each of the field sites L, FC, and C. For each of the three communities, the bacterial genera contributing most to the distinction between inoculated and non-inoculated maize differed from one field to the other (except that Microbacterium was more prevalent among diazotrophs in inoculated maize than the control at both sites L and FC), indicating field-specific inoculation effects on indigenous bacteria.
Taxa Indicative of Inoculation Impact Across the Three Fields
Even though the bacterial genera mainly implicated in functional group (and whole community) differences between inoculated maize and the control mostly differed from one field to the other, an attempt was made to identify differentiating taxa (including taxa with lower contributions) that would contribute to treatment differentiation more reproducibly, that is, across all three sites. To this aim, we pooled bacterial composition data from the maize rhizosphere of the three fields to determine which bacterial genera were the most impacted by Azospirillum inoculation at the scale of the three sites studied (Figures 5A–C). With this approach, inoculated and non-inoculated maize could be discriminated by BCA based on nifH (p = 0.001) or rrs data (p = 10–4), but not with acdS data (p = 0.19) despite apparent differences in the genus composition profile at two of three sites (Supplementary Figure 3).
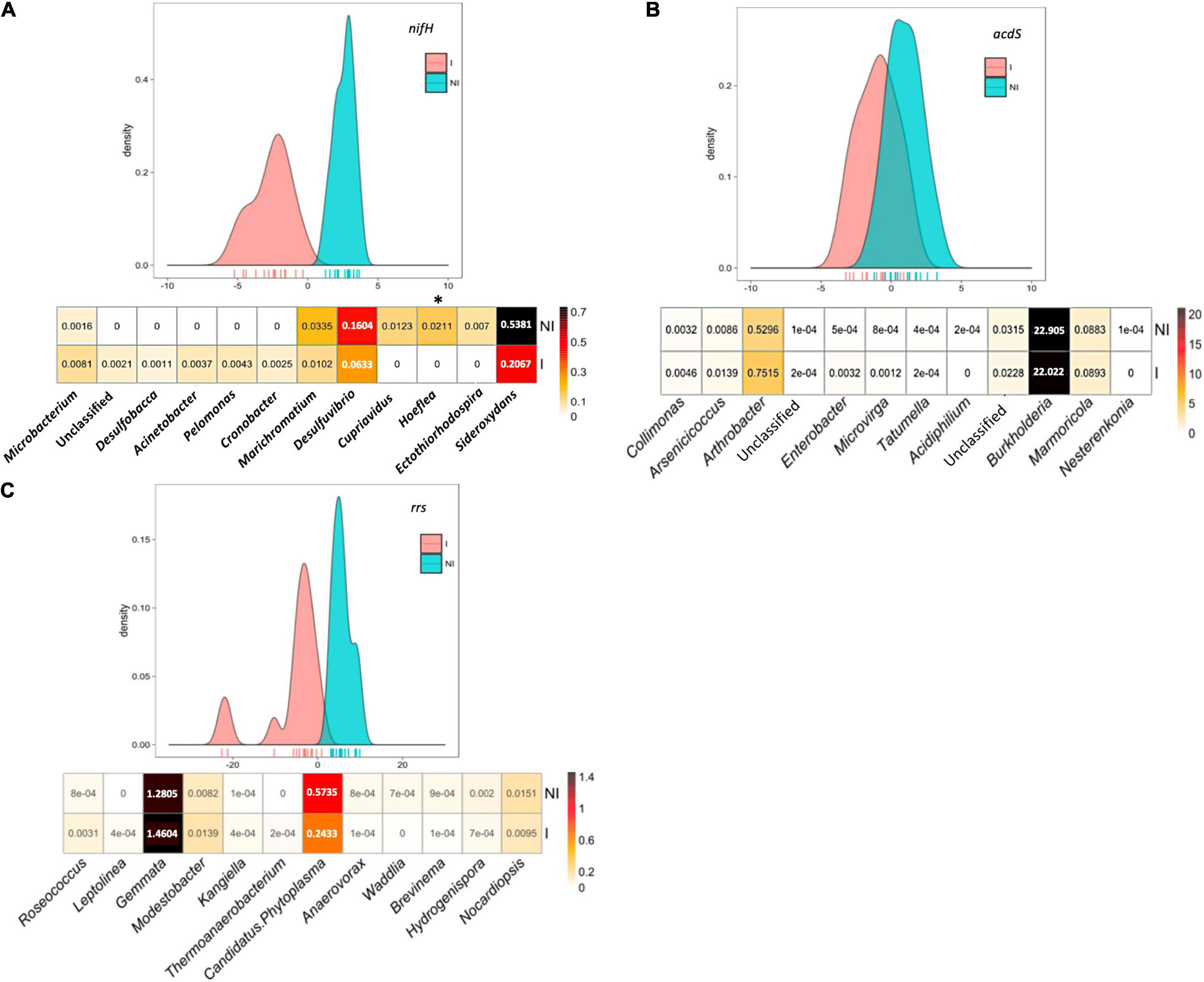
Figure 5. Between-class analysis of the effect of seed inoculation with A. lipoferum CRT1 on the composition of (A) nifH and (B) acdS functional groups, as well as (C) the total bacterial community (rrs) over three sites. Analyses were done on pooled data of bacterial composition of maize rhizosphere of the three sites studied. NI and I correspond, respectively, to non-inoculated and inoculated conditions. For each bacterial group, the BCA graph is completed (below) by the distribution of the bacterial OTUs according to their contribution to treatment differentiation. BCA is statistically supported by a Monte Carlo test based on nifH (p = 0.001) and rrs data (p = 10– 4), but not with acdS data (p = 0.19), and the heatmap below indicates the relative proportions for the 12 bacterial genera most impacted by inoculation in maize rhizosphere. Six bacterial genera most commonly associated with inoculation are indicated at the left, as well as six bacterial genera most often associated with the non-inoculated control (right). Statistical differences between inoculated and non-inoculated treatments are indicated by stars (*) (Wilcoxon test, p < 0.05). Heatmap unit is a percentage of the average relative proportion of taxa.
With nifH data, the 12 bacterial genera contributing most to treatment differentiation were an unclassified genus, Microbacterium, Desulfobacca, Acinetobacter, Pelomonas, and Cronobacter (genera most commonly associated with inoculated maize), as well as Marichromatium, Desulfurivibrio, Cupriavidus, Hoeflea, Ectothiorhodospira, and Sideroxydans (genera most commonly associated with non-inoculated maize). With rrs data, the 12 genera contributing most to treatment differentiation were Roseococcus, Leptolinea, Gemmata, Modestobacter, Kangella, and Thermoanaerobacterium (genera most commonly associated with inoculated maize), as well as Candidatus Phytoplasma, Anaerovorax, Waddlia, Brevinema, Hydrogenispora, and Nocardiopsis (genera most commonly associated with non-inoculated maize).
In summary, in our search for more cosmopolitan taxa indicators of inoculation, the artificial pooling of metabarcoding sequences from the three sites did evidence additional taxa (on top of Microbacterium) among the 12 taxa contributing most to differentiation of inoculated maize from the non-inoculated control when considering the diazotroph community (and the global bacterial community), but not the ACC-deaminating community.
Discussion
In this work, we tested the hypothesis that PGPR inoculation could result in major changes in root-associated functional microbial communities implicated in phytostimulation, using a range of field trials where the seed inoculant A. lipoferum CRT1 had triggered changes in maize metabolome, photosynthetic efficiency, plant development/growth, and/or crop yield (Rozier et al., 2017). Indeed, we observed a modification of strong magnitude in the composition of the diazotroph community and (to a lower degree) of the ACC deaminase community. It is interesting to note that, for both functional groups, these impacts took place irrespective of the morphological or physiological effects evidenced on maize plants at the time of sampling. Rice inoculation with Azospirillum (or Pseudomonas) in a field had little effect on nifH T-RFLP profiles (García de Salamone et al., 2012), whereas maize inoculation with nitrogen-fixing Pseudomonas in pots changed diazotroph community composition based on Illumina MiSeq sequencing of nifH (Ke et al., 2019). The issue was not investigated so far with the ACC deaminase community, as the methodology needed to do so has not been available for long (Bouffaud et al., 2018). The impact of inoculation on functional groups needs to receive further research attention, because it may bring additional insight into PGPR modes of action when the latter entail stimulation of indigenous plant-beneficial microorganisms (Trabelsi and Mhamdi, 2013; Vacheron et al., 2013; Ambrosini et al., 2016; da Costa et al., 2018) and also because it is likely to be more informative than taxonomic studies to describe the breadth of inoculant impacts (Ke et al., 2019).
Here, the significant effects of inoculation on the diversity of the nifH and acdS functional groups were also evidenced with rrs data, that is, when considering inoculation effects at the level of the whole rhizobacterial community. Based on nifH, acdS, and rrs analyses, the genera most impacted by inoculation belonged to Actinobacteria, Cyanobacteria, Firmicutes, or Proteobacteria, indicating a taxonomic shift larger than what was thought so far. Overall, the effects of inoculation were underpinned by changes in the relative abundance of multiple taxa. Some of these changes were small, but others were of larger magnitude (e.g., a decrease from 32 to 19% for Burkholderia; Figure 3). These findings contrast with previous studies showing minor and/or transient ecological impact of Azospirillum inoculants on the resident bacterial community colonizing the rhizosphere (Ambrosini et al., 2016), by molecular fingerprinting in the case of maize (Herschkovitz et al., 2005; Lerner et al., 2006; Matsumura et al., 2015), wheat (Naiman et al., 2009; Baudoin et al., 2010), rice (Pedraza et al., 2009; García de Salamone et al., 2010, 2012; Bao et al., 2013), or other crops (Correa et al., 2007; Felici et al., 2008), as well as by Illumina MiSeq metabarcoding in the case of maize (da Costa et al., 2018). Modest inoculant impacts on the rhizobacterial community were also found with Bacillus on lettuce (by metagenomics; Kröber et al., 2014) and tomato (by pyrosequencing; Qiao et al., 2017), Pseudomonas on lettuce (by DGGE and pyrosequencing; Schreiter et al., 2014), Pseudomonas or Achromobacter on maize (by Illumina MiSeq; da Costa et al., 2018), Stenotrophomonas on maize (by Illumina MiSeq; Kusstatscher et al., 2020), multispecies inoculants on tomato (by Illumina MiSeq; Nuzzo et al., 2020) and wheat (by pyrosequencing; Dal Cortivo et al., 2020), or a multispecies organic amendment on sugarcane (by Illumina MiSeq; Berg et al., 2019), whereas a larger impact was observed by Illumina MiSeq with Pseudomonas on maize (Ke et al., 2019) and different oilseed crops (Jiménez et al., 2020); Pseudomonas, Paenibacillus, or Bacillus on lettuce (Passera et al., 2020); an organic amendment enriched in microorganisms on strawberry (Deng et al., 2019); or a multispecies inoculant on onion (Pellegrini et al., 2021).
The second hypothesis tested was that the effects of PGPR inoculation on functional communities could be field site-specific, which turned out to be the case. Indeed, the most impacted bacterial genera seldom overlapped between sites, and when they did, modifications did not follow the same trend in all sites. Genus overlap (i.e., the same genera impacted at several sites) was more often found in the acdS group, perhaps because there were fewer genera in this group. When we pooled data from all fields, we showed that the acdS group did not differ significantly between inoculated and non-inoculated treatments, in contrary to the nifH group (and the rrs community). This suggests that inoculation effects on acdS rhizobacteria were more site-dependent than those on nifH rhizobacteria and on all rhizobacteria. These findings are likely to have implications in terms of rhizosphere functioning, phytostimulation implementation, and crop behavior, because they mean that inoculants will stimulate indigenous plant-beneficial microorganisms in a field-specific manner, as the natural soil community, the cropping/agronomic history, the pedoclimatic conditions, and/or crop physiology may differ between fields.
Here, inoculation resulted in 16 increases and 4 decreases in gene copy number (for nifH, acdS, or phlD) of the 60 individual comparisons carried out. These significant differences depended on the gene studied, the field, the year, the crop stage, and nitrogen fertilization (nutriment stress or not). Importantly, they often varied between fields when a given gene × nitrogen fertilization combination was compared across the various sampling dates assessed, pointing again to site-specific effects of inoculation on microbial functional groups important for phytostimulation.
As the nifH+ inoculant A. lipoferum CRT1 remained under qPCR detection threshold, it suggests that the augmentation in nifH copy number in CRT1-inoculated maize in certain comparisons was not due to presence of the nifH+ inoculant itself. Lack of inoculant survival does not necessarily mean that inoculation has no effect (Mawarda et al., 2020). Furthermore, inoculation effects on the density of the three functional groups were stronger at flowering stage, even though the inoculant was long below detection limit. Inoculation of Ensifer (previously Sinorhizobium) meliloti resulted also in changes in the number of nifH+ bacteria in maize rhizosphere that were plant phenology-dependent (Babić et al., 2008; Gupta et al., 2012). acdS gene copies were higher in CRT1-inoculated maize at flowering stage, which could have ecological consequences (Glick, 2014) as ethylene is produced from ACC in higher amount at flowering stage than in early growth stages (Bleecker and Kende, 2000).
Two types of mechanisms could explain the ecological impact of A. lipoferum CRT1: first, a direct effect of the inoculant on indigenous rhizobacteria by antagonism, competition, or cooperation (Pandey and Kumar, 1990; Tapia-Hernández et al., 1990; Tortora et al., 2011; Trabelsi and Mhamdi, 2013; Ambrosini et al., 2016), which could modify community composition. So far, Azospirillum species are mostly described as plant stimulators (Dobbelaere et al., 2003; Bashan and de-Bashan, 2010) by their capacity to produce phytohormones (Karadeniz et al., 2006; Cohen et al., 2015), but they may interact with other plant-beneficial microorganisms (Russo et al., 2005; Combes-Meynet et al., 2011). Here, the lack of significant inoculant survival (strain CRT1 remaining perhaps at very low levels, as a member of the rare biosphere) means that direct inoculant effects could occur only at the very early stages of maize colonization, where an impact on keystone “hub” microorganisms (Agler et al., 2016; Mawarda et al., 2020) might, in turn, have affected the microbiome network. Second, several studies showed that A. lipoferum CRT1 does not need to be well established in the maize rhizosphere to benefit plant growth (Jacoud et al., 1999; Rozier et al., 2017, 2019). In the current work, inoculation had resulted in changes in plant morphology and physiology (photosynthetic efficiency and metabolome), and at the six-leaf stage in 2015, plant growth modifications were observed at site L and plant metabolome changes at all three sites (Rozier et al., 2017). This starter effect of A. lipoferum CRT1 suggests modification of plant physiology from very early development stages of maize (Jacoud et al., 1999; Walker et al., 2011; Rozier et al., 2017, 2019), probably driving root microbiota changes. Indeed, CRT1 inoculation can also result in modification of plant secondary metabolites, especially benzoxazinoids and cinnamic acids (Walker et al., 2011), which are present in root secretions and known to play a major role in plant–microbe interactions (Neal et al., 2012).
Conclusion
In conclusion, the seed inoculant A. lipoferum CRT1 did not manage to establish itself in the maize rhizosphere. However, it had a significant impact on the diversity of key functional groups important for plant performance and of the whole bacterial community in the rhizosphere, pointing to direct and/or plant-mediated effects of the inoculant on resident rhizobacteria. Importantly, we showed that this impact was field site-specific, thereby validating our hypothesis. This study demonstrated that inoculation has the potential to affect rhizosphere microbial functioning and identified community-based ecological mechanisms by which an inoculant could influence plant performance. It also showed the usefulness of considering microbial functional groups to reveal ecological effects on the plant microbiome, and further research efforts are necessary to develop the molecular toolbox needed to monitor a wider range of plant-beneficial functions under optimized or stress conditions.
Data Availability Statement
The datasets presented in this study can be found in online repositories. The names of the repository/repositories and accession number(s) can be found in the article.
Author Contributions
LL, YM-L, CP-C, and DM designed the project. SR, JV, CP-C, LL, YM-L, and DM carried out field work. SR and JV conducted the molecular work. SR and DA implemented bioinformatic analyses. SR, YM-L, and DM analyzed data and prepared the first draft of the manuscript, which was finalized by all authors.
Funding
This work was funded through the ANR project Azodure (ANR-12-AGRO-0008) and the IDEX-Lyon project Phytobiome.
Conflict of Interest
The authors declare that the research was conducted in the absence of any commercial or financial relationships that could be construed as a potential conflict of interest.
Publisher’s Note
All claims expressed in this article are solely those of the authors and do not necessarily represent those of their affiliated organizations, or those of the publisher, the editors and the reviewers. Any product that may be evaluated in this article, or claim that may be made by its manufacturer, is not guaranteed or endorsed by the publisher.
Acknowledgments
We are grateful to Jacqueline Haurat and Hélène Brunet for technical help, farmers for access to fields, Agrauxine for inoculant product, and La Dauphinoise coop for field management.
Supplementary Material
The Supplementary Material for this article can be found online at: https://www.frontiersin.org/articles/10.3389/fmicb.2021.760512/full#supplementary-material
Supplementary Figure 1 | Rarefaction curves for nifH (A), acdS (B), and rrs (C) at field sites L, FC, and C. NI and I correspond, respectively, to non-inoculated and inoculated conditions.
Supplementary Figure 2 | Non-metric multidimensional scaling (NMDS) of nifH (A), acdS (B), and rrs (C) data at field sites L, FC, and C. NI and I correspond, respectively, to non-inoculated and inoculated conditions. NMDS of distance matrices was based on the Bray–Curtis distance and was done using the vegan package in R.
Supplementary Figure 3 | Relative abundance of the most prevalent bacterial genera based on analysis of nifH, acdS and rrs data for field sites L, FC, and C (results from the five replicates were pooled). NI and I correspond, respectively, to non-inoculated and inoculated conditions.
Footnotes
References
Agler, M. T., Ruhe, J., Kroll, S., Morhenn, C., Kim, S.-T., Weigel, D., et al. (2016). Microbial hub taxa link host and abiotic factors to plant microbiome variation. PLoS Biol. 14:e1002352. doi: 10.1371/journal.pbio.1002352
Almario, J., Moënne-Loccoz, Y., and Muller, D. (2013). Monitoring of the relation between 2,4-diacetylphloroglucinol-producing Pseudomonas and Thielaviopsis basicola populations by real-time PCR in tobacco black root-rot suppressive and conducive soils. Soil Biol. Biochem. 57, 144–155. doi: 10.1016/j.soilbio.2012.09.003
Ambrosini, A., de Souza, R., and Passaglia, L. M. (2016). Ecological role of bacterial inoculants and their potential impact on soil microbial diversity. Plant Soil 400, 93–207.
Babić, K. H., Schauss, K., Hai, B., Sikora, S., Redžepović, S., Radl, V., et al. (2008). Influence of different Sinorhizobium meliloti inocula on abundance of genes involved in nitrogen transformations in the rhizosphere of alfalfa (Medicago sativa L.). Environ. Microbiol. 10, 2922–2930. doi: 10.1111/j.1462-2920.2008.01762.x
Bao, Z., Sasaki, K., Okubo, T., Ikeda, S., Anda, M., Hanzawa, E., et al. (2013). Impact of Azospirillum sp. B510 inoculation on rice-associated bacterial communities in a paddy field. Microbes Environ. 28, 487–490. doi: 10.1264/jsme2.me13049
Bashan, Y., and de-Bashan, L. E. (2010). How the plant growth-promoting bacterium Azospirillum promotes plant growth - a critical assessment. Adv. Agron. 108, 77–136. doi: 10.1016/s0065-2113(10)08002-8
Basu, A., Prasad, P., Das, S. N., Kalam, S., Sayyed, R. Z., Reddy, M. S., et al. (2021). Plant growth promoting rhizobacteria (PGPR) as green bioinoculants: recent developments, constraints, and prospects. Sustainability 13:1140. doi: 10.3390/su13031140
Baudoin, E., Lerner, A., Mirza, M. S., El Zemrany, H., Prigent-Combaret, C., Jurkevich, E., et al. (2010). Effects of Azospirillum brasilense with genetically modified auxin biosynthesis gene ipdC upon the diversity of the indigenous microbiota of the wheat rhizosphere. Res. Microbiol. 161, 219–226. doi: 10.1016/j.resmic.2010.01.005
Baudoin, E., Nazaret, S., Mougel, C., Ranjard, L., and Moënne-Loccoz, Y. (2009). Impact of inoculation with the phytostimulatory PGPR Azospirillum lipoferum CRT1 on the genetic structure of the rhizobacterial community of field-grown maize. Soil Biol. Biochem. 41, 409–413. doi: 10.1016/j.soilbio.2008.10.015
Berg, S., Dennis, P. G., Paungfoo-Lonhienne, C., Anderson, J., Robinson, N., Brackin, R., et al. (2019). Effects of commercial microbial biostimulants on soil and root microbial communities and sugarcane yield. Biol. Fertil. Soils 56, 565–580. doi: 10.1007/s00374-019-01412-4
Bertrand, H., Plassard, C., Pinochet, X., Touraine, B., Normand, P., and Cleyet-Marel, J.-C. (2000). Stimulation of the ionic transport system in Brassica napus by a plant growth-promoting rhizobacterium (Achromobacter sp.). Can. J. Microbiol. 46, 229–236. doi: 10.1139/w99-137
Bleecker, A. B., and Kende, H. (2000). Ethylene: a gaseous signal molecule in plants. Annu. Rev. Cell Dev. Biol. 16, 1–18. doi: 10.1146/annurev.cellbio.16.1.1
Bouffaud, M.-L., Renoud, S., Dubost, A., Moënne-Loccoz, Y., and Muller, D. (2018). 1-Aminocyclopropane-1-carboxylate deaminase producers associated to maize and other Poaceae species. Microbiome 6:114. doi: 10.1186/s40168-018-0503-7
Bouffaud, M.-L., Renoud, S., Moënne-Loccoz, Y., and Muller, D. (2016). Is plant evolutionary history impacting recruitment of diazotrophs and nifH expression in the rhizosphere? Sci. Rep. 6:21690. doi: 10.1038/srep21690
Brazelton, J. N., Pfeufer, E. E., Sweat, T. A., McSpadden Gardener, B. B., and Coenen, C. (2008). 2,4-diacetylphloroglucinol alters plant root development. Mol. Plant Microbe Interact. 21, 1349–1358. doi: 10.1094/MPMI-21-10-1349
Brusamarello-Santos, L. C., Gilard, F., Brulé, L., Quilleré, I., Gourion, B., Ratet, P., et al. (2017). Metabolic profiling of two maize (Zea mays L.) inbred lines inoculated with the nitrogen fixing plant-interacting bacteria Herbaspirillum seropedicae and Azospirillum brasilense. PLoS One 12:e0174576. doi: 10.1371/journal.pone.0174576
Cáceres, E. A. (1982). Improved medium for isolation of Azospirillum spp. Appl. Environ. Microbiol. 44, 990–991. doi: 10.1128/aem.44.4.990-991.1982
Cangahuala-Inocente, G. C., Plucani do Amaral, F., Faleiro, A. C., Huergo, L. F., and Maisonnave Arisi, A. C. (2013). Identification of six differentially accumulated proteins of Zea mays seedlings (DKB240 variety) inoculated with Azospirillum brasilense strain FP2. Eur. J. Soil Biol. 58, 45–50. doi: 10.1016/j.ejsobi.2013.06.002
Castro-Sowinski, S., Herschkovitz, Y., Okon, Y., and Jurkevitch, E. (2007). Effects of inoculation with plant growth-promoting rhizobacteria on resident rhizosphere microorganisms. FEMS Microbiol. Lett. 276, 1–11. doi: 10.1111/j.1574-6968.2007.00878.x
Chamam, A., Sanguin, H., Bellvert, F., Meiffren, G., Comte, G., Wisniewski-Dyé, F., et al. (2013). Plant secondary metabolite profiling evidences strain-dependent effect in the Azospirillum–Oryza sativa association. Phytochemistry 87, 65–77. doi: 10.1016/j.phytochem.2012.11.009
Chessel, D., Dufour, A. B., and Thioulouse, J. (2004). The ade4 package - I: one-table methods. R. News 4, 5–10.
Cohen, A. C., Bottini, R., Pontin, M., Berli, F. J., Moreno, D., Boccanlandro, H., et al. (2015). Azospirillum brasilense ameliorates the response of Arabidopsis thaliana to drought mainly via enhancement of ABA levels. Physiol. Plant. 153, 79–90. doi: 10.1111/ppl.12221
Combes-Meynet, E., Pothier, J. F., Moënne-Loccoz, Y., and Prigent-Combaret, C. (2011). The Pseudomonas secondary metabolite 2,4-diacetylphloroglucinol is a signal inducing rhizoplane expression of Azospirillum genes involved in plant-growth promotion. Mol. Plant Microbe Interact. 24, 271–284. doi: 10.1094/MPMI-07-10-0148
Correa, O. S., Romero, A. M., Montecchia, M. S., and Soria, M. A. (2007). Tomato genotype and Azospirillum inoculation modulate the changes in bacterial communities associated with roots and leaves. J. Appl. Microbiol. 102, 781–786. doi: 10.1111/j.1365-2672.2006.03122.x
Couillerot, O., Bouffaud, M.-L., Baudoin, E., Muller, D., Caballero-Mellado, J., and Moënne-Loccoz, Y. (2010). Development of a real-time PCR method to quantify the PGPR strain Azospirillum lipoferum CRT1 on maize seedlings. Soil Biol. Biochem. 42, 2298–2305. doi: 10.1016/j.soilbio.2010.09.003
Creus, C. M., Graziano, M., Casanovas, E. M., Pereyra, M. A., Simontacchi, M., Puntarulo, S., et al. (2005). Nitric oxide is involved in the Azospirillum brasilense-induced lateral root formation in tomato. Planta 221, 297–303. doi: 10.1007/s00425-005-1523-7
da Costa, P. B., de Campos, S. B., Albersmeier, A., Dirksen, P., Dresseno, A. L. P., dos Santos, O. J. A. P., et al. (2018). Invasion ecology applied to inoculation of plant growth promoting bacteria through a novel SIMPER-PCA approach. Plant Soil 422, 467–478. doi: 10.1007/s11104-017-3492-6
Dal Cortivo, C., Ferrari, M., Visioli, G., Lauro, M., Fornasier, F., Barion, G., et al. (2020). Effects of seed-applied biofertilizers on rhizosphere biodiversity and growth of common wheat (Triticum aestivum L.) in the field. Front. Plant Sci. 11:72. doi: 10.3389/fpls.2020.00072
Deng, S., Wipf, H. M. L., Pierroz, G., Raab, T. K., Khanna, R., and Coleman-Derr, D. (2019). A plant growth-promoting microbial soil amendment dynamically alters the strawberry root bacterial microbiome. Sci. Rep. 9:17677. doi: 10.1038/s41598-019-53623-2
DeSantis, T. Z., Hugenholtz, P., Larsen, N., Rojas, M., Brodie, E. L., Keller, K., et al. (2006). Greengenes, a chimera-checked 16S rRNA gene database and workbench compatible with ARB. Appl. Environ. Microbiol. 72, 5069–5072. doi: 10.1128/AEM.03006-05
Di Salvo, L. P., Cellucci, G. C., Carlino, M. E., and García de Salamone, I. E. (2018). Plant growth-promoting rhizobacteria inoculation and nitrogen fertilization increase maize (Zea mays L.) grain yield and modified rhizosphere microbial communities. Appl. Soil Ecol. 126, 113–120. doi: 10.1016/j.apsoil.2018.02.010
Dobbelaere, S., Vanderleyden, J., and Okon, Y. (2003). Plant growth-promoting effects of diazotrophs in the rhizosphere. Crit. Rev. Plant Sci. 22, 107–149.
Dolédec, S., and Chessel, D. (1987). Rythmes saisonniers et composantes stationnelles en milieu aquatique. I- Description d’un plan d’observations complet par projection de variables. Acta oecologica. Oecol. Generalis 8, 403–426.
Dray, S., Dufour, A. B., and Chessel, D. (2007). The ade4 package - II: two-table and K-table methods. R. News 7, 47–52.
Drogue, B., Sanguin, H., Chamam, A., Mozar, M., Llauro, C., Panaud, O., et al. (2014a). Plant root transcriptome profiling reveals a strain-dependent response during Azospirillum-rice cooperation. Front. Plant Sci. 5:607. doi: 10.3389/fpls.2014.00607
Drogue, B., Prigent-Combaret, C., Sanguin, H., Borland, S., and Wisniewski-Dyé, F. (2014b). Genome wide profiling of Azospirillum lipoferum 4B gene expression during interaction with rice roots. FEMS Microbiol. Ecol. 87, 543–555. doi: 10.1111/1574-6941.12244
El Zemrany, H., Cortet, J., Lutz, M. P., Chabert, A., Baudoin, E., Haurat, J., et al. (2006). Field survival of the phytostimulator Azospirillum lipoferum CRT1 and functional impact on maize crop, biodegradation of crop residues, and soil faunal indicators in a context of decreasing nitrogen fertilisation. Soil Biol. Biochem. 38, 1712–1726. doi: 10.1016/j.soilbio.2005.11.025
Fages, J., and Mulard, D. (1988). Isolement de bactéries rhizosphériques et effet de leur inoculation en pots chez Zea mays. Agronomie 8, 309–314. doi: 10.1051/agro:19880405
Felici, C., Vettori, L., Giraldi, E., Forino, L. M. C., Toffanin, A., Tagliasacchi, A. M., et al. (2008). Single and co-inoculation of Bacillus subtilis and Azospirillum brasilense on Lycopersicon esculentum: effects on plant growth and rhizosphere microbial community. Appl. Soil Ecol. 40, 260–270. doi: 10.1016/j.apsoil.2008.05.002
Florio, A., Pommier, T., Gervaix, J., Bérard, A., and Le Roux, X. (2017). Soil C and N statuses determine the effect of maize inoculation by plant growth-promoting rhizobacteria on nitrifying and denitrifying communities. Sci. Rep. 7:8411. doi: 10.1038/s41598-017-08589-4
Fuhrman, J. A. (2009). Microbial community structure and its functional implications. Nature 459:193. doi: 10.1038/nature08058
Gabriel, J. L., Zarco-Tejada, P. J., López-Herrera, P. J., Pérez-Martín, E., Alonso-Ayuso, M., and Quemada, M. (2017). Airborne and ground level sensors for monitoring nitrogen status in a maize crop. Biosyst. Eng. 160, 124–133.
Gaby, J. C., and Buckley, D. H. (2017). The use of degenerate primers in qPCR analysis of functional genes can cause dramatic quantification bias as revealed by investigation of nifH primer performance. Microb. Ecol. 74, 701–708. doi: 10.1007/s00248-017-0968-0
García de Salamone, I. E., Di Salvo, L. P., Escobar Ortega, J. S., Boa Sorte, P. M. F., Urquiaga, S., and Teixeira, K. R. S. (2010). Field response of rice paddy crop to Azospirillum inoculation: physiology of rhizosphere bacterial communities and the genetic diversity of endophytic bacteria in different parts of the plants. Plant Soil 336, 351–362. doi: 10.1007/s11104-010-0487-y
García de Salamone, I. E., Funes, J. M., Di Salvo, L. P., Escobar-Ortega, J. S., D’Auria, F., Ferrando, L., et al. (2012). Inoculation of paddy rice with Azospirillum brasilense and Pseudomonas fluorescens: Impact of plant genotypes on rhizosphere microbial communities and field crop production. Appl. Soil Ecol. 61, 196–204. doi: 10.1016/j.apsoil.2011.12.012
Glick, B. R. (2014). Bacteria with ACC deaminase can promote plant growth and help to feed the world. Microbiol. Res. 69, 30–39. doi: 10.1016/j.micres.2013.09.009
Gupta, R., Bru, D., Bisaria, V. S., Philippot, L., and Sharma, S. (2012). Responses of Cajanus cajan and rhizospheric N-cycling communities to bioinoculants. Plant and Soil 358, 143–154. doi: 10.1007/s11104-012-1293-5
Herschkovitz, Y., Lerner, A., Davidov, Y., Okon, Y., and Jurkevitch, E. (2005). Azospirillum brasilense does not affect population structure of specific rhizobacterial communities of inoculated maize (Zea mays). Environmental Microbiology 7, 1847–1852. doi: 10.1111/j.1462-2920.2005.00926.x
Jacoud, C., Job, D., Wadoux, P., and Bally, R. (1999). Initiation of root growth stimulation by Azospirillum lipoferum CRT1 during maize seed germination. Can. J. Microbiol. 45, 339–342. doi: 10.1139/w99-023
Jiménez, J. A., Novinscak, A., and Filion, M. (2020). Inoculation with the plant-growth-promoting rhizobacterium Pseudomonas fluorescens LBUM677 impacts the rhizosphere microbiome of three oilseed crops. Front. Microbiol. 11:2534. doi: 10.3389/fmicb.2020.569366
Karadeniz, A., and Topcuoğlu, Ş, and Inan, S. (2006). Auxin, gibberellin, cytokinin and abscisic acid production in some bacteria. World J. Microbiol. Biotechnol. 22, 1061–1064. doi: 10.1007/s11274-005-4561-1
Ke, X., Feng, S., Wang, J., Lu, W., Zhang, W., Chen, M., et al. (2019). Effect of inoculation with nitrogen-fixing bacterium Pseudomonas stutzeri A1501 on maize plant growth and the microbiome indigenous to the rhizosphere. Syst. Appl. Microbiol. 42, 248–260. doi: 10.1016/j.syapm.2018.10.010
Keel, C., Wirthner, P., Oberhänsli, T., Voisard, C., Haas, D., and Défago, G. (1990). Pseudomonads as antagonists of plant pathogens in the rhizosphere: role of the antibiotic 2,4-diacetylphloroglucinol in the suppression of black root rot of tobacco. Symbiosis (Rehovot) 9, 327–341.
Kröber, M., Wibberg, D., Grosch, R., Eikmeyer, F., Verwaaijen, B., Chowdhury, S. P., et al. (2014). Effect of the strain Bacillus amyloliquefaciens FZB42 on the microbial community in the rhizosphere of lettuce under field conditions analyzed by whole metagenome sequencing. Front. Microbiol. 5:252. doi: 10.3389/fmicb.2014.00252
Kusstatscher, P., Wicaksono, W. A., Thenappan, D. P., Adam, E., Müller, H., and Berg, G. (2020). Microbiome management by biological and chemical treatments in maize is linked to plant health. Microorganisms 8:1506. doi: 10.3390/microorganisms8101506
Lerner, A., Herschkovitz, Y., Baudoin, E., Nazaret, S., Moënne-Loccoz, Y., Okon, Y., et al. (2006). Effect of Azospirillum brasilense inoculation on rhizobacterial communities analyzed by denaturing gradient gel electrophoresis and automated ribosomal intergenic spacer analysis. Soil Biol. Biochem. 38, 1212–1218. doi: 10.1016/j.soilbio.2005.10.007
Matsumura, E. E., Secco, V. A., Moreira, R. S., dos Santos, O. J. A. P., Hungria, M., and de Oliveira, A. L. M. (2015). Composition and activity of endophytic bacterial communities in field-grown maize plants inoculated with Azospirillum brasilense. Ann. Microbiol. 65, 2187–2200. doi: 10.1007/s13213-015-1059-4
Mawarda, P. C., Le Roux, X., van Elsas, J. D., and Salles, J. F. (2020). Deliberate introduction of invisible invaders: a critical appraisal of the impact of microbial inoculants on soil microbial communities. Soil Biol. Biochem. 148:107874. doi: 10.1016/j.soilbio.2020.107874
Molina-Favero, C., Creus, C. M., Simontacchi, M., Puntarulo, S., and Lamattina, L. (2008). Aerobic nitric oxide production by Azospirillum brasilense Sp245 and its influence on root architecture in tomato. Mol. Plant Microbe Interact. 21, 1001–1009. doi: 10.1094/MPMI-21-7-1001
Naiman, A. D., Latrónico, A., and Garcia de Salamone, I. E. (2009). Inoculation of wheat with Azospirillum brasilense and Pseudomonas fluorescens: impact on the production and culturable rhizosphere microflora. Eur. J. Soil Biol. 45, 44–51. doi: 10.1016/j.ejsobi.2008.11.001
Neal, A. L., Ahmad, S., Gordon-Weeks, R., and Ton, J. (2012). Benzoxazinoids in root exudates of maize attract Pseudomonas putida to the rhizosphere. PLoS One 7:e35498. doi: 10.1371/journal.pone.0035498
Nuzzo, A., Satpute, A., Albrecht, U., and Strauss, S. L. (2020). Impact of soil microbial amendments on tomato rhizosphere microbiome and plant growth in field soil. Microb. Ecol. 80, 398–409. doi: 10.1007/s00248-020-01497-7
Okon, Y., and Labandera-Gonzalez, C. A. (1994). Agronomic applications of Azospirillum: an evaluation of 20 years worldwide field inoculation. Soil Biol. Biochem. 26, 1591–1601. doi: 10.1016/0038-0717(94)90311-5
Pandey, A., and Kumar, S. (1990). Inhibitory effects of Azotobacter chroococcum and Azospirillum brasilense on a range of rhizosphere fungi. Indian J. Exp. Biol. 28, 52–54.
Park, J.-W., and Crowley, D. E. (2005). Normalization of soil DNA extraction for accurate quantification of target genes by real-time PCR and DGGE. Biotechniques 38, 579–586. doi: 10.2144/05384ST04
Passera, A., Vacchini, V., Cocetta, G., Arpanahi, A. A., Casati, P., Ferrante, A., et al. (2020). Towards nutrition-sensitive agriculture: an evaluation of biocontrol effects, nutritional value, and ecological impact of bacterial inoculants. Sci. Total Environ. 724:138127. doi: 10.1016/j.scitotenv.2020.138127
Pedraza, R. O., Bellone, C. H., de Bellone, S. C., Sorte, P. M. F. B., and dos Santos Teixeira, K. R. (2009). Azospirillum inoculation and nitrogen fertilization effect on grain yield and on the diversity of endophytic bacteria in the phyllosphere of rice rainfed crop. Eur. J. Soil Biol. 45, 36–43. doi: 10.1016/j.ejsobi.2008.09.007
Pellegrini, M., Spera, D. M., Ercole, C., and Del Gallo, M. (2021). Allium cepa L. inoculation with a consortium of plant growth-promoting bacteria: Effects on plants, soil, and the autochthonous microbial community. Microorganisms 9:639. doi: 10.3390/microorganisms9030639
Pii, Y., Mimmo, T., Tomasi, N., Terzano, R., Cesco, S., and Crecchio, C. (2015). Microbial interactions in the rhizosphere: beneficial influences of plant growth-promoting rhizobacteria on nutrient acquisition process. A review. Biol. Fertil. Soils 51, 403–415. doi: 10.1007/s00374-015-0996-1
Poly, F., Jocteur Monrozier, L., and Bally, R. (2001). Improvement in the RFLP procedure for studying the diversity of nifH genes in communities of nitrogen fixers in soil. Res. Microbiol. 152, 95–103. doi: 10.1016/s0923-2508(00)01172-4
Qiao, J., Yu, X., Liang, X., Liu, Y., Borriss, R., and Liu, Y. (2017). Addition of plant-growth-promoting Bacillus subtilis PTS-394 on tomato rhizosphere has no durable impact on composition of root microbiome. BMC Microbiol. 17:131. doi: 10.1186/s12866-017-1039-x
Raaijmakers, J., Paulitz, T. C., Steinberg, C., Alabouvette, C., and Moënne-Loccoz, Y. (2009). The rhizosphere: a playground and battlefield for soilborne pathogens and beneficial microorganisms. Plant Soil 321, 341–361. doi: 10.1007/s11104-008-9568-6
Reed, S. C., Cleveland, C. C., and Townsend, A. R. (2011). Functional ecology of free-living nitrogen fixation: a contemporary perspective. Annu. Rev. Ecol. Evol. Syst. 42, 489–512. doi: 10.1146/annurev-ecolsys-102710-145034
Renoud, S., Bouffaud, M.-L., Dubost, A., Prigent-Combaret, C., Legendre, L., Moënne-Loccoz, Y., et al. (2020). Co-occurrence of rhizobacteria with nitrogen fixation and/or 1-aminocyclopropane-1-carboxylate deamination abilities in the maize rhizosphere. FEMS Microbiol. Ecol. 96:fiaa0622. doi: 10.1093/femsec/fiaa062
Rozier, C., Erban, A., Hamzaoui, J., Prigent-Combaret, C., Comte, G., Kopka, J., et al. (2016). Xylem sap metabolite profile changes during phytostimulation of maize by the plant growth-promoting rhizobacterium, Azospirillum lipoferum CRT1. Metabolomics (Los Angel) 6, 1–10.
Rozier, C., Gerin, F., Czarnes, S., and Legendre, L. (2019). Biopriming of maize germination by the plant growth-promoting rhizobacterium Azospirillum lipoferum CRT1. J. Plant Physiol. 237, 111–119. doi: 10.1016/j.jplph.2019.04.011
Rozier, C., Hamzaoui, J., Lemoine, D., Czarnes, S., and Legendre, L. (2017). Field-based assessment of the mechanism of maize yield enhancement by Azospirillum lipoferum CRT1. Sci. Rep. 7:7416. doi: 10.1038/s41598-017-07929-8
Russo, A., Felici, C., Toffanin, A., Götz, M., Collados, C., Barea, J. M., et al. (2005). Effect of Azospirillum inoculants on arbuscular mycorrhiza establishment in wheat and maize plants. Biol. Fertil. Soils 41, 301–309. doi: 10.1007/s00374-005-0854-7
Sarabia, M., Cazares, S., González-Rodríguez, A., Mora, F., Carreón-Abud, Y., and Larsen, J. (2018). Plant growth promotion traits of rhizosphere yeasts and their response to soil characteristics and crop cycle in maize agroecosystems. Rhizosphere 6:67–73.
Schimel, J., and Schaeffer, S. M. (2012). Microbial control over carbon cycling in soil. Front. Microbiol. 3:348. doi: 10.3389/fmicb.2012.00348
Schmidt, J. E., and Gaudin, A. C. M. (2018). What is the agronomic potential of biofertilizers for maize? A meta-analysis. FEMS Microbiol. Ecol. 94, fiy094. doi: 10.1093/femsec/fiy094
Schreiter, S., Ding, G. C., Grosch, R., Kropf, S., Antweiler, K., and Smalla, K. (2014). Soil type-dependent effects of a potential biocontrol inoculant on indigenous bacterial communities in the rhizosphere of field-grown lettuce. FEMS Microbiol. Ecol. 90, 718–730. doi: 10.1111/1574-6941.12430
Steenhoudt, O., and Vanderleyden, J. (2000). Azospirillum, a free-living nitrogen-fixing bacterium closely associated with grasses: genetic, biochemical and ecological aspects. FEMS Microbiol. Rev. 24, 487–506. doi: 10.1111/j.1574-6976.2000.tb00552.x
Tapia-Hernández, A., Mascarua-Esparza, M., and Caballero-Mellado, J. (1990). Production of bacteriocins and siderophore-like activity by Azospirillum brasilense. Microbios 64, 73–83.
Thioulouse, J., Prin, Y., and Duponnois, R. (2012). Multivariate analyses in soil microbial ecology: a new paradigm. Environ. Ecol. Stat. 19, 499–520. doi: 10.1007/s10651-012-0198-z
Tortora, M. L., Díaz-Ricci, J. C., and Pedraza, R. O. (2011). Azospirillum brasilense siderophores with antifungal activity against Colletotrichum acutatum. Arch. Microbiol. 193, 275–286. doi: 10.1007/s00203-010-0672-7
Trabelsi, D., and Mhamdi, R. (2013). Microbial inoculants and their impact on soil microbial communities: a review. BioMed Res. Int. 2013:863240. doi: 10.1155/2013/863240
Vacheron, J., Desbrosses, G., Bouffaud, M.-L., Touraine, B., Moënne-Loccoz, Y., Muller, D., et al. (2013). Plant growth-promoting rhizobacteria and root system functioning. Front. Plant Sci. 4:356. doi: 10.3389/fpls.2013.00356
Volpin, H., Burdman, S., Castro-Sowinski, S., Kapulnik, Y., and Okon, Y. (1996). Inoculation with Azospirillum increased exudation of rhizobial nod-gene inducers by alfalfa roots. Mol. Plant Microbe Interact. 9, 388–394. doi: 10.1094/mpmi-9-0388
Walker, V., Bertrand, C., Bellvert, F., Moënne-Loccoz, Y., Bally, R., and Comte, G. (2011). Host plant secondary metabolite profiling shows a complex, strain-dependent response of maize to plant growth-promoting rhizobacteria of the genus Azospirillum. New Phytol. 189, 494–506. doi: 10.1111/j.1469-8137.2010.03484.x
Keywords: microbial functional group, PGPR, inoculation, nitrogen fixers, ACC deaminase producers, 4-diacetylphloroglucinol producers
Citation: Renoud S, Vacheron J, Abrouk D, Prigent-Combaret C, Legendre L, Muller D and Moënne-Loccoz Y (2022) Field Site-Specific Effects of an Azospirillum Seed Inoculant on Key Microbial Functional Groups in the Rhizosphere. Front. Microbiol. 12:760512. doi: 10.3389/fmicb.2021.760512
Received: 18 August 2021; Accepted: 03 December 2021;
Published: 26 January 2022.
Edited by:
Xun Wen Chen, Southern University of Science and Technology, ChinaReviewed by:
Anelise Beneduzi, Livestock and Irrigation, BrazilJosé David Flores Félix, Universidade da Beira Interior, Portugal
Entao Wang, Instituto Politécnico Nacional (IPN), Mexico
Riyazali Zafarali Sayyed, P.S.G.V.P.M’s Arts, Science and Commerce College, India
Copyright © 2022 Renoud, Vacheron, Abrouk, Prigent-Combaret, Legendre, Muller and Moënne-Loccoz. This is an open-access article distributed under the terms of the Creative Commons Attribution License (CC BY). The use, distribution or reproduction in other forums is permitted, provided the original author(s) and the copyright owner(s) are credited and that the original publication in this journal is cited, in accordance with accepted academic practice. No use, distribution or reproduction is permitted which does not comply with these terms.
*Correspondence: Yvan Moënne-Loccoz, eXZhbi5tb2VubmUtbG9jY296QHVuaXYtbHlvbjEuZnI=
†Present address: Sébastien Renoud, BGene, Grenoble, France Jordan Vacheron, Université de Lausanne, Department of Fundamental Microbiology, Lausanne, Switzerland