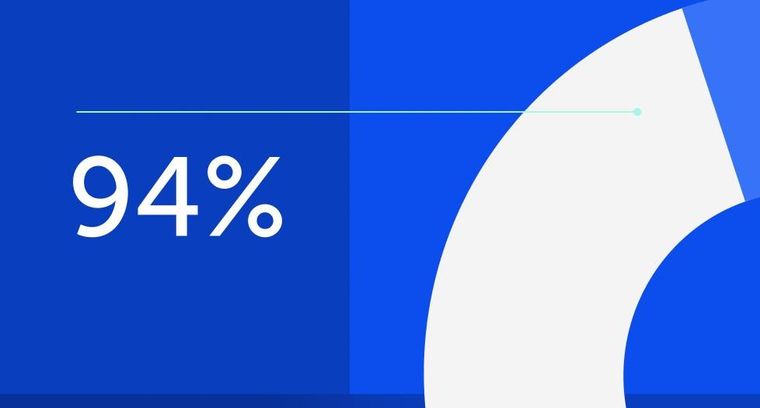
94% of researchers rate our articles as excellent or good
Learn more about the work of our research integrity team to safeguard the quality of each article we publish.
Find out more
MINI REVIEW article
Front. Microbiol., 11 November 2021
Sec. Microbiotechnology
Volume 12 - 2021 | https://doi.org/10.3389/fmicb.2021.759975
The use of traditional chemical insecticides for pest control often leads to environmental pollution and a decrease in biodiversity. Recently, insect sex pheromones were applied for sustainable biocontrol of pests in fields, due to their limited adverse impacts on biodiversity and food safety compared to that of other conventional insecticides. However, the structures of insect pheromones are complex, and their chemical synthesis is not commercially feasible. As yeasts have been widely used for fatty acid-derived pheromone production in the past few years, using engineered yeasts may be promising and sustainable for the low-cost production of fatty acid-derived pheromones. The primary fatty acids produced by Saccharomyces cerevisiae and other yeasts are C16 and C18, and it is also possible to rewire/reprogram the metabolic flux for other fatty acids or fatty acid derivatives. This review summarizes the fatty acid biosynthetic pathway in S. cerevisiae and recent progress in yeast engineering in terms of metabolic engineering and synthetic biology strategies to produce insect pheromones. In the future, insect pheromones produced by yeasts might provide an eco-friendly pest control method in agricultural fields.
Pheromones are the chemicals used by individuals to communicate with members of the same species (Karlson and Luscher, 1959). Many insect pheromones are fatty acid-derived molecules, which play an essential role in the insect life cycle, such as attraction, aggression, aphrodisiacs, anti-aphrodisiacs, aggregation, kin recognition, and alarm signaling (Yew and Chung, 2015). Insect pheromones are trace chemicals usually secreted by female insects to attract males of the same species. Most insect pheromones studies focus on moth and a few other Lepidopteran sex pheromones. Based on their chemical structures, moth sex pheromones are classified into three types, the Type-I (75%), the Type-II (15%), and the miscellaneous groups (10%) (Ando et al., 2004). Type-I sex pheromones are alcohols, aldehydes, and acetates with 10–18 carbon chains, and are produced by most moth species. Type-II sex pheromones comprise odd polyunsaturated hydrocarbons (C17-C23) with two or three double bonds at positions three, six, or nine, in addition to their corresponding epoxide derivatives (Matsumoto, 2010; Sun et al., 2019; Yang et al., 2020). The miscellaneous groups of sex pheromones comprise secondary alcohols with short-chain fatty acids (C7 and C9) and can be further classified as Type-0, while those with methyl-branched compounds are classified as Type-III (Ando and Yamamoto, 2020).
Pest control is a global challenge associated with food supplies. One of the most potent green strategies for pest biocontrol involves mating disruption in insects by releasing pheromones into the crop field (Reddy and Guerrero, 2010; Benelli et al., 2019). Sex pheromones have been widely used for insect control and monitoring in agriculture, horticulture, and forestry (Witzgall et al., 2010). Using pheromones for pest control is highly efficient, non-toxic, not harmful to the beneficial insect species, and does not pollute the environment, which satisfies the requirement of food and environmental security. The sex pheromone components are already being chemically synthesized, nevertheless, their chemical synthesis requires expensive substrates and catalysts and generates hazardous wastes (Herbert et al., 2013; Turczel et al., 2018). In this study, we review Type-I insect sex pheromones and their biosynthetic pathways, since most sex pheromones are assigned to this class. We have also summarized the biosynthesis of sex pheromones using engineered yeasts and discussed the potential application of yeasts for insect sex pheromone production.
Moths and other Lepidopteran insects mainly produce Type-I sex pheromones; these are produced in the sex pheromone gland (PG), and released when needed (Tillman et al., 1999; Ando et al., 2004). In fact, most female moths release pheromones at relatively low level, while some species release periodically when matched pheromones are available (Raina et al., 2000; Jurenka, 2017).
Since most moth pheromones have a straight-chain carbon backbone, their biosynthetic pathways are common in different moth species (Figure 1). The first step of sex pheromone biosynthesis is the synthesis of fatty acids, which starts with acetyl-CoA and is catalyzed by acetyl-CoA carboxylase and fatty acid synthase (FAS). A series of saturated fatty acid precursors are used for pheromone biosynthesis. The secondary step is desaturation, which involves highly specific desaturases to generate one double bond or several double bonds at different positions in the fatty acids. The third step involves the reduction of fatty acids by fatty acid reductases (FARs) to convert fatty acyl to fatty alcohol. The succeeding steps are associated with the functional group modification catalyzed by fatty alcohol oxidases and fatty acetyltransferases to form aldehyde and acetate ester groups (Tehlivets et al., 2007; Wang M. et al., 2020). Here, we highlight some of the key enzymes involved in the desaturation, reduction, and functional group modification steps in the biosynthesis of representative pheromones (Supplementary Figure 1).
Figure 1. Biosynthetic pathways of sex pheromones in several lepidopteran species (including Bombyx mori, Spodoptera litura, Streltzoviella insularis, Antheraea pernyi, Thysanoplusia intermixta, Heliothis subflexa, H. virescens, Grapholita molesta, and G. dimorpha). Pheromone synthesis starts with a ubiquitous fatty acid synthesis, followed by desaturation of fatty acyl-CoA precursors, then reduction and functional group modification steps to produce species-specific alcohol (blue), aldehyde (green), or acetate ester (pink), that can be used as pheromone blends; the structure of each pheromone molecule is also shown. The general biosynthesis processes and enzymes involved are also marked in this figure.
In Lepidoptera and other insects, a variety of acyl-CoA desaturases catalyze the desaturation of fatty acyl intermediates, and they often localize at endoplasmic reticulum membrane (Figure 1; Hagström et al., 2013a). Especially, Δ11 desaturases (Z11 desaturase, E11 desaturase) generate a double bond at the C11 position. In the female silkmoth Bombyx mori, the first double bond is introduced at C16:CoA fatty acid by Z11 desaturase to form Z11-16:CoA (Matsumoto et al., 2007). The desaturation reaction is common in Heliothis subflexa, Spodoptera litura, and Antheraea pernyi (Choi et al., 2002; Lin et al., 2018). In H. subflexa, desaturation of C18:CoA is catalyzed by Z11 desaturase to produce Z11-C18:CoA (Choi et al., 2002). Both Z11 desaturase (converts C16:CoA to Z11-C16:CoA) and E11 desaturase (converts C14:CoA to E11-C14:CoA) have been identified in S. litura (Lin et al., 2018). Several other desaturases that catalyze double bond generation in saturated fatty acyl have been identified. For instance, in Streltzoviella insularis, Δ5 desaturases (Z5 and E5) catalyze the conversion of C16:CoA to (E/Z)5-C16:CoA, and Z9 desaturases catalyze the conversion of C16:CoA to Z9-C16:CoA (Yang et al., 2020). In A. pernyi, E6 desaturase catalyzes C16:CoA to E6-C16:CoA (Wang et al., 2010a). In Grapholita molesta and Grapholita dimorpha, Δ10 desaturase (Z10 and E10) desaturates C14:CoA to form (E/Z)10-C14:CoA (Jung and Kim, 2014). Several desaturases exhibit the specificity for mono-unsaturated substrates. In S. litura, Z9 desaturase introduces a second double bond in E11-C14:CoA to form Z9, E11-C14:CoA, while E12 desaturase converts Z9-C14:CoA to Z9, E12-C14:CoA (Lin et al., 2018). In A. pernyi, E6 desaturase converts C16:CoA to E6-C16:CoA, and Z11 desaturase converts E6-C16:CoA to E6, Z11-C16:CoA (Wang et al., 2010a). Likewise, E5, Z7-C12:CoA is produced by the desaturation of Z7-C12:CoA by E5 desaturase in Thysanoplusia intermixta (Ono et al., 2002). Many moth and other Lepidopteran pheromone intermediates can be desaturated by applying different combinations, temporal orders, and stereospecificity of diverse desaturases.
Fatty acid reductases catalyze the reduction of fatty-acyl pheromone precursors to their corresponding alcohols. In moth, many types of FARs have been identified and their substrate specificity has been found to produce species-specific pheromones (Figure 1). Some FARs exhibit broad substrate preferences. For example, the first pheromone-gland fatty acyl reductase (pgFAR) isolated from the B. mori shows its activity on a broad range of saturated and monounsaturated C14- and C18-acyl precursors (Moto et al., 2003). In three species of Yponomeuta, a single pgFAR has been found to efficiently reduce saturated and unsaturated C14- and C16-acyl precursors (Liénard et al., 2010). The pgFARs have been found to possess a general selectivity for C8-C16 fatty acyl precursors in four closely related heliothine moths (Hagström et al., 2012). Some FARs also reduce specific pheromone intermediates. In two sex pheromone races of Ostrinia nubilalis, the allelic variation in the gene coding for pgFAR has been observed to cause distinct substrate stereoselectivity (E11-C14:acid and Z11-C14:acid), which contributes to the intraspecific reproductive isolation in moths (Lassance et al., 2010). In Spodoptera exigua, a highly selective pgFAR I has been reported, which catalyzes the reduction of Z11-C16:acyl to produce moth pheromone signals (Antony et al., 2016). The substrate selectivity of pgFARs plays an essential role in generating species-specific signals.
Fatty acid reductases catalyze the conversion of the functional groups of fatty-acyl pheromone precursors to the hydroxyl group. Likewise, aldehyde-producing oxidases, acetyltransferases, and other enzymes can also modify the pheromones in many moths (Figure 1). Some oxidases transform fatty alcohols into aldehydes. The aldehydic sex pheromone components in Heliothis zea are catalyzed by cuticular alcohol oxidases, and the oxidases are also responsible for the conversion of the primary fatty alcohols to fatty aldehydes (Dou et al., 2020). In H. subflexa and H. virescens, the major pheromone component is Z11-hexadecenal (Z11-C16:Ald), which is produced by the oxidation of the precursor Z11-hexadecenol (Z11-C16:OH) (Choi et al., 2002). The sex pheromone component of Amyelois transitella, Z11, Z13-C16:Ald, is probably produced from Z11, Z13-C16:OH in the PG by oxidation (Wang et al., 2010b). In some moth species of Choristoneura fumiferana (Roscoe et al., 2016), S. litura (Lin et al., 2018), and S. insularis (Yang et al., 2020), the acetyl transferases catalyze the conversion of fatty alcohols to acetate ester pheromones.
Thus, diverse Type-I pheromones are generated by the co-catalyzation of differing combinations, temporal orders, and substrate specificities of desaturases, reductases, oxidases, and modification enzymes (Matsumoto, 2010).
As a model species, S. cerevisiae has been used to produce a variety of different fatty acids or fatty acid-derived products (Wei et al., 2019; Gao et al., 2020; Guan et al., 2020). Most Lepidopteran sex pheromones are fatty acid alcohols, aldehydes, or fatty alcohol acetates with chain lengths of 10-18 carbons. S. cerevisiae cells mainly produce C16 and C18 fatty acids (Tehlivets et al., 2007; Wei et al., 2017b), which are suitable as potential chassis for the microbial synthesis of insect sex pheromones (Supplementary Figure 1).
Acetyl-CoA is the precursor for fatty acid biosynthesis. In mitochondria, acetyl-CoA mainly enters the TCA cycle to be oxidized and release energy. In the cytoplasm, pyruvate is converted to acetyl-CoA under the catalysis of pyruvate decarboxylase, acetaldehyde dehydrogenase (ALD), and two acetyl-CoA synthetases (ACS1 and ACS2) (Krivoruchko et al., 2015; Zhang et al., 2018; Wang M. et al., 2020). The carboxyl group is transferred to acetyl-CoA to yield malonyl-CoA catalyzed by acetyl-CoA carboxylase (ACC1). Then, acetyl-CoA and malonyl-CoA are catalyzed by FAS for fatty acid synthesis and elongation (Tehlivets et al., 2007). In S. cerevisiae, cytosolic FAS is capable of synthesizing fatty acids up to C20 in vitro. The elongation enzymes (ELO) can increase the length of the fatty acids up to C26 in yeasts (Rössler et al., 2003). Normally, ELO1 extends C12-C16 fatty acyl-CoAs to C16-C18 fatty acids, ELO2 extends palmitoyl-CoA and stearoyl-CoA up to C22 fatty acids, and ELO3 catalyzes the synthesis of C20-C26 fatty acids from C18-CoA (Oh et al., 1997; Rössler et al., 2003; Wenning et al., 2017). Approximately 70-80% of yeast fatty acids are monounsaturated, and the desaturation reactions are catalyzed by OLE1, the endoplasmic reticulum membrane-bound Δ9 fatty acid desaturase (Martin et al., 2007). Metabolic engineering strategies for the biosynthesis of fatty acids mainly include increasing the content of fatty acid precursors and cofactors, eliminating the competing pathways, and regulating the activity of fatty acid synthases and elongation enzymes (Supplementary Figure 1).
Cytosolic acetyl-CoA is the primary substrate for de novo FAS biosynthesis in yeast. Deletion of alcohol dehydrogenase (ADH) genes could reduce ethanol formation from acetaldehyde, and the overexpression of a heterologous mutant acetyl-CoA synthase from Salmonella enterica (SeAcsL641P) combined with the overexpression of ALD (more specifically ALD6) could increase the flux toward acetyl-CoA-derived products (Shiba et al., 2007). Alternatively, engineering the cytosolic pyruvate dehydrogenase (PDH) bypass pathway (Kozak et al., 2014), ATP-citrate lyase (ACL) pathway, and the phosphoketolase pathway could further enhance acetyl-CoA biosynthesis (de Jong et al., 2014). Expression of an ATP-independent PDH complex from Enterococcus faecalis and ACL could increase the pool of cytosolic acetyl-CoA in S. cerevisiae (Supplementary Figure 1; Kozak et al., 2014; Feng et al., 2015).
Malonyl-CoA is the rate-limiting compound in de novo fatty acid biosynthesis in yeast. Overexpression of ACC1 gene in S. cerevisiae was found to increase the levels of fatty acids (Shin et al., 2012). Plasmid-based overexpression of endogenous ACC1 increased the total fatty acid production from 42.7 mg/L to 63.2 mg/L (∼1.48-fold) (Runguphan and Keasling, 2014). The activity of ACC1 is negatively regulated by snf1-dependent phosphorylation, and the introduction of mutations in two phosphorylation sites of ACC1 (ACC1S659A, S1157A) increased ACC1 activity and total fatty acid content (Shi et al., 2014). When the third mutation was introduced in ACC1S659A, S1157A, the resulting strain with ACC1S686A, S659A, S1157A could produce a higher amount malonyl-CoA, and the titer of 3-hydroxypropionic acid — a malonyl-CoA-derived compound — improved by 1.5-fold (Chen et al., 2018).
The cofactor NADPH is required in the cell for de novo fatty acid synthesis in yeast. A recent metabolic flux analysis indicated that 60% of all NADPH is consumed for glutamate biosynthesis by the NADP+-dependent glutamate dehydrogenases GDH1 and GDH3 (d’Espaux et al., 2017). Deletion of the GDH1 gene in yeast resulted in a 2.7-fold improvement in fatty alcohol production (d’Espaux et al., 2017). Xylulose-5-phosphate, which is produced by the pentose phosphate (PP) pathway, acts as the precursor of the phosphoketolase (PHK) pathway. The combination of PHK and PP pathways in S. cerevisiae led to increased cytosolic NADPH levels and subsequently improved production of fatty acid ethyl esters (de Jong et al., 2014). Downregulation of PGI1 (encoding glucose-6-phosphate isomerase 1) and enhancing PP pathway flux by overexpression of ZWF1 (encoding glucose-6-phosphate dehydrogenase), GND1 (encoding 6-phosphogluconate dehydrogenase, decarboxylating 1), TKL1 (encoding transketolase-1), and TAL1 (encoding transaldolase) could increase NADPH supply and significantly improve the production of free fatty acids (FFAs) (Yu et al., 2018).
Another effective way to increase fatty acids production involves eliminating the competing metabolic pathways, such as β-oxidation and triacylglycerol production (Wei et al., 2017a, 2018). The β-oxidation pathway is often stalled by deletion of FAA (encoding acyl-CoA synthetase), PXA (encoding the peroxisomal long-chain acyl-CoA transporter complex), and/or POX1 (encoding fatty acyl-CoA oxidase, catalyzing the first step of β-oxidation). Disruption of β-oxidation by knockouts in FAA2, PXA1, and POX1 genes increased intracellular fatty acid levels by 55% compared to that in the control strain BY4741 (Leber et al., 2015). The disruption of FAA1 in S. cerevisiae B-1 strain resulted in a two-fold increase in fatty acid secretion level (Michinaka et al., 2003), while a double deletion of FAA1 and FAA4 in yeast significantly increased the production of FFAs (Scharnewski et al., 2008; Li et al., 2014; Runguphan and Keasling, 2014; Zhou et al., 2016). Simultaneous deletion of FAA1, FAA4, and POX1 further increased the production of fatty acids by 31% compared to strain with only FAA1 and FAA4 deletion (Li et al., 2014). A S. cerevisiae strain with multi-gene deletions (faa1Δfaa4Δfat1Δfaa2Δpxa1Δpox1Δ) was able to produce 1.3 g/L extracellular FFAs, which is higher than 490 mg/L FFAs production in a strain with triple deletions of faa1Δfaa4Δfat1Δ (Leber et al., 2015).
Overexpression of the FAS complex by replacing the native FAS1 and FAS2 promoters with the strong constitutive PTEF1 promoter could increase total fatty acid production as well as lipid content (Runguphan and Keasling, 2014). In fact, plasmid-based overexpression of the E. coli acyl-ACP thioesterase (‘TesA) in S. cerevisiae led to the production of 5 mg/L of FFAs, eight-times that produced by the background strain (0.6 mg/L) (Runguphan and Keasling, 2014). Overexpression of ‘TesA in S. cerevisiae WRY1 strain (all fatty acid biosynthesis genes under PTEF1 promoter) improved FFAs production levels to 52 mg/L (Runguphan and Keasling, 2014). Likewise, expressing FAS from Rhodospuridium toruloides (RtFAS) in yeast increased the total lipid as well as FFA content (Zhou et al., 2016). In another report, a combination strategy, involving blockage of fatty acid activation and degradation, introducing an optimized acetyl-CoA pathway, expressing a more efficient FAS, and overexpressing the endogenous acetyl-CoA carboxylase increased FFA titer to 10.4 g/L (Zhou et al., 2016).
The length and saturation of fatty acids in S. cerevisiae can be modulated. When a thioesterase from Acinetobacter baylyi (’AcTesA) was embedded into reaction compartments of fungal FASs, it led to 5-13 times more production of extracellular short/medium-chain fatty acids compared to that in the wild-type strains (Zhu et al., 2017). In one study, S. cerevisiae was successfully engineered to produce very-long-chain fatty acids (C16-C18 and C22-C24 VLCFAs) and derived chemicals by incorporating a heterologous FAS I system from Mycobacterium vaccae. By the introduction of endogenous yeast fatty acid elongation system, C22-C26 fatty acids could be selectively produced (Yu et al., 2017). By tailoring the bacterial carboxylic acid reductase from Mycobacterium marinum (MmCAR) via directed evolution and rational design, introduction of the MmCAR variants and metabolic engineering strategies successfully led to the establishment of a S. cerevisiae platform with the capability of medium-chain fatty alcohol production (Hu et al., 2020). Furthermore, modulation of ACC1 and ELO1 expression led to increased titers of C18:0 and C18:1 (Bergenholm et al., 2018).
Compared with conventional insecticides, insect sex pheromones are specific for pest management and are thus environmentally friendly. Some insect sex pheromone biosynthetic enzymes and pathways have been identified and functionally analyzed, providing the possibility to produce pheromones by synthetic biology-based strategies. A pheromone-gland-specific FAR gene (encoding FAR) of the silkmoth was expressed in S. cerevisiae, and the resulting yeast strain could produce E10, Z12-C16:OH, which induced typical mating behavior in male B. mori (Moto et al., 2003). Co-expression of a Δ11 fatty acyl-CoA desaturase gene and a reductase gene of Agrotis segetum in S. cerevisiae led to the production of a set of long-chain fatty acids and alcohols that do not occur naturally in yeast, and the titer of Z11-C16:OH was 19.5 μg/L. Moreover, the oxidized extracts from the yeast cells were found to induce specific electrophysiological activity in male antennae of H. virescens (Hagström et al., 2013b).
Insect pheromones of Z11-C16:OH and Z9-tetradecenol (Z9-C14:OH) have been produced by engineered oleaginous yeast, Yarrowia lipolytica. The combined activity of a desaturase from A. transitella (AtrΔ11) and a reductase from Helicoverpa armigera (HarFAR) resulted in the production of 1.7 mg/L Z11-C16:OH (Table 1; Holkenbrink et al., 2020). Likewise, the combination expression of a desaturase from Drosophila melanogaster (DmeΔ9), reductase HarFAR, and acetyltransferase ATF1 of S. cerevisiae led to the production of 7.3 mg/L Z9-C14:OAc, which is the main sex pheromone component of the fall armyworm Spodoptera frugiperda. (Table 1; Holkenbrink et al., 2020). Several strategies, including preventing endogenous fatty alcohol degradation, inhibiting acyl-CoA degradation, reducing the flux toward storage lipids, and increasing the supply of tetradecanoyl-CoA precursor, have been applied to improve pheromones production; consequently, the engineered Y. lipolytica strains could produce 73.6 mg/L of Z9-C14:OH (15-fold increase in titer over the background strain) and 2.57 g/L of Z11-C16:OH (Table 1; Holkenbrink et al., 2020). Introduction of a point mutation into the α-subunit of FAS (FAS2I1220F), and overexpression of an optimal combination of a fatty acyl-CoA desaturase (FAD; Lbo_PPTQ) from Lobesia botrana, FAR (HarFAR) from H. armigera and the gene encoding native FAS1 led to a final Z11-14:OH titer of 188.1 mg/L in fed-batch fermentation (Table 1; Petkevicius et al., 2021). In another study, expression of the gene encoding FAR, BlapFAR4 from Bombus lapidarius or BlucFAR1 from Bombus lucorum in Y. lipolytica, led to the production of bumblebee pheromones consisting of long-chain fatty alcohols. The titer of saturated fatty alcohols with C18-C24 was 166.6 mg/L, while the titer of C16 FA-OHs (C16:0-OH and C16:1-OH) was 14.6 mg/L (Table 1; Hambalko et al., 2020). However, in S. cerevisiae, expression of BlucFAR1 produced only a small amount of FA-OHs (6.9 mg/L), while the expression of BlapFAR4 led to the production of 79 mg/L of C16-OHs (Table 1; Tupec et al., 2019). The difference in titers and chain length of FA-OH products in engineered Y. lipolytica and S. cerevisiae may be due to the variations in substrate availability, hinting at the selection of different yeast chassis cells for the biosynthesis of different sex pheromones based on yeast fatty acid profiles (Wei et al., 2017b). As Y. lipolytica is an oleaginous yeast and robust to diverse substrates, Y. lipolytica has more potential to be an ideal insect pheromone production cell factory (Dobrowolski et al., 2019; Vasconcelos et al., 2019).
As cell factories, S. cerevisiae and other yeasts mainly produce C16 and C18 fatty acids (Wei et al., 2017b; Wang J. et al., 2020), that are suitable for the biosynthesis of C16 and C18 pheromones. Currently, various metabolic engineering strategies, such as increasing substrate precursors and cofactors, eliminating competing pathways, and modulation of FAS and other keystone enzymes in sex pheromone biosynthetic pathways, have been developed to improve the production of fatty acids and their derivatives in yeasts. Engineered yeasts can produce a high level of Lepidopteran and a few other insect sex pheromones. Strategies to increase pheromone production using synthetic biology approaches require designing optimal pheromone biosynthetic pathway, and protein engineering of key enzymes with high activities. It is also necessary to identify more suitable keystone genes for insect pheromone production based on omics technologies.
The cost of chemically synthesized pheromones is high, therefore, mating disruption applications currently primarily target higher-value crops (Ioriatti and Lucchi, 2016). Biosynthesis of insect sex pheromone using engineered microbial strains is one of the most promising environmentally friendly strategies for large-scale commercial production of pheromones at a relatively low cost. An increasing number of FADs and FARs and other functional modification enzymes involved in the biosynthesis of Lepidopteran sex pheromones have been successfully identified and functionally characterized. However, characterizing more efficient insect sex pheromone enzymes and adaption of insect sex pheromone pathways to yeast cell factories still need further studies.
As cell factories, S. cerevisiae and other yeasts produce fatty acids that are appropriate for C16 and C18 pheromone biosynthesis. Various metabolic engineering strategies, such as increasing levels of the biosynthetic precursors and cofactor, eliminating competing pathways, and regulating the activity of FAS and elongation enzymes, have been developed in yeast to increase the production of fatty acids and to modify chain length and saturation. With such synthetic biology strategies, engineering of S. cerevisiae, Y. lipolytica or other yeasts to produce Lepidopteran sex pheromones has achieved considerable success. With further development and application of advanced yeast tools, such as high throughput screening strategy (Tan et al., 2020), metabolic mass transfer strategy (Xue et al., 2021), and efficient gene editing tools for non-conventional oleaginous yeasts (Shan et al., 2021), large-scale commercial production of sex pheromones in engineered yeasts with a high titer, rate and yield would be possible, and will also facilitate moth-mating disruption using biosynthetic sex pheromones in a cost-efficient manner. In the future, the application of yeast-based sex pheromones will lead to eco-friendly agriculture with a green pest control strategy.
YW conceived the study. XZ and YW drafted the manuscript and designed the whole study. XZ, QM, and YW prepared the figures. BJ, XX, and LQ revised and polished the manuscript. All authors read, revised, and approved the manuscript.
This work was supported by the National Natural Science Foundation of China (Nos. 31800079 and 32111530179).
The authors declare that the research was conducted in the absence of any commercial or financial relationships that could be construed as a potential conflict of interest.
All claims expressed in this article are solely those of the authors and do not necessarily represent those of their affiliated organizations, or those of the publisher, the editors and the reviewers. Any product that may be evaluated in this article, or claim that may be made by its manufacturer, is not guaranteed or endorsed by the publisher.
We would like to thank TopEdit (www.topeditsci.com) for the English language editing of this manuscript.
The Supplementary Material for this article can be found online at: https://www.frontiersin.org/articles/10.3389/fmicb.2021.759975/full#supplementary-material
Ando, T., Inomata, S., and Yamamoto, M. (2004). Lepidopteran sex pheromones. Top. Curr. Chem. 239, 51–96. doi: 10.1007/b9544
Ando, T., and Yamamoto, M. (2020). Semiochemicals containing lepidopteran sex pheromones: wonderland for a natural product chemist. J. Pestic. Sci. 45, 191–205. doi: 10.1584/jpestics.D20-046
Antony, B., Ding, B. J., Moto, K., Aldosari, S. A., and Aldawood, A. S. (2016). Two fatty acyl reductases involved in moth pheromone biosynthesis. Sci. Rep. 6:29927. doi: 10.1038/srep29927
Benelli, G., Lucchi, A., Thomson, D., and Ioriatti, C. (2019). Sex pheromone aerosol devices for mating disruption: challenges for a brighter future. Insects 10:308. doi: 10.3390/insects10100308
Bergenholm, D., Gossing, M., Wei, Y., Siewers, V., and Nielsen, J. (2018). Modulation of saturation and chain length of fatty acids in Saccharomyces cerevisiae for production of cocoa butter-like lipids. Biotechnol. Bioeng. 115, 932–942. doi: 10.1002/bit.26518
Chen, X., Yang, X., Shen, Y., Hou, J., and Bao, X. (2018). Screening phosphorylation site mutations in yeast acetyl-CoA carboxylase using malonyl-CoA sensor to improve malonyl-CoA-derived product. Front. Microbiol. 9:47. doi: 10.3389/fmicb.2018.00047
Choi, M. Y., Han, K. S., Boo, K. S., and Jurenka, R. A. (2002). Pheromone biosynthetic pathways in the moths Helicoverpa zea and Helicoverpa assulta. Insect Biochem. Mol. Biol. 32, 1353–1359. doi: 10.1016/s0965-1748(02)00055-3
de Jong, B. W., Shi, S., Siewers, V., and Nielsen, J. (2014). Improved production of fatty acid ethyl esters in Saccharomyces cerevisiae through up-regulation of the ethanol degradation pathway and expression of the heterologous phosphoketolase pathway. Microb. Cell Fact. 13:39. doi: 10.1186/1475-2859-13-39
d’Espaux, L., Ghosh, A., Runguphan, W., Wehrs, M., Xu, F., Konzock, O., et al. (2017). Engineering high-level production of fatty alcohols by Saccharomyces cerevisiae from lignocellulosic feedstocks. Metab. Eng. 42, 115–125. doi: 10.1016/j.ymben.2017.06.004
Dobrowolski, A., Drzymała, K., Rzechonek, D. A., Mituła, P., and Mirończuk, A. M. (2019). Lipid production from waste materials in seawater-based medium by the yeast Yarrowia lipolytica. Front. Microbiol. 10:547. doi: 10.3389/fmicb.2019.00547
Dou, X., Zhang, A., and Jurenka, R. (2020). Functional identification of fatty acyl reductases in female pheromone gland and tarsi of the corn earworm, Helicoverpa zea. Insect Biochem. Mol. Biol. 116:103260. doi: 10.1016/j.ibmb.2019.103260
Feng, X., Lian, J., and Zhao, H. (2015). Metabolic engineering of Saccharomyces cerevisiae to improve 1-hexadecanol production. Metab. Eng. 27, 10–19. doi: 10.1016/j.ymben.2014.10.001
Gao, Q., Wang, L., Zhang, M., Wei, Y., and Lin, W. (2020). Recent Advances on Feasible Strategies for Monoterpenoid Production in Saccharomyces cerevisiae. Front. Bioeng. Biotechnol. 8:609800. doi: 10.3389/fbioe.2020.609800
Guan, R., Wang, M., Guan, Z., Jin, C.-Y., Lin, W., Ji, X., et al. (2020). Metabolic engineering for glycyrrhetinic acid production in Saccharomyces cerevisiae. Front. Bioeng. Biotechnol. 8:588255. doi: 10.3389/fbioe.2020.588255
Hagström, A. K., Liénard, M. A., Groot, A. T., Hedenström, E., and Löfstedt, C. (2012). Semi-selective fatty acyl reductases from four heliothine moths influence the specific pheromone composition. PLoS One 7:e37230. doi: 10.1371/journal.pone.0037230
Hagström, Å. K., Walther, A., Wendland, J., and Löfstedt, C. (2013a). Subcellular localization of the fatty acyl reductase involved in pheromone biosynthesis in the tobacco budworm, Heliothis virescens (Noctuidae: lepidoptera). Insect Biochem. Mol. Biol. 43, 510–521. doi: 10.1016/j.ibmb.2013.03.006
Hagström, Å. K., Wang, H. L., Liénard, M. A., Lassance, J. M., Johansson, T., and Löfstedt, C. (2013b). A moth pheromone brewery: production of (Z)-11-hexadecenol by heterologous co-expression of two biosynthetic genes from a noctuid moth in a yeast cell factory. Microb. Cell Fact. 12:125. doi: 10.1186/1475-2859-12-125
Hambalko, J., Gajdoš, P., Nicaud, J. M., Ledesma-Amaro, R., Tupec, M., Pichová, I., et al. (2020). Production of long chain fatty alcohols found in bumblebee pheromones by Yarrowia lipolytica. Front. Bioeng. Biotechnol. 8:593419. doi: 10.3389/fbioe.2020.593419
Herbert, M. B., Marx, V. M., Pederson, R. L., and Grubbs, R. H. (2013). Concise syntheses of insect pheromones using Z-selective cross metathesis. Angew. Chem. Int. Ed. Engl. 52, 310–314. doi: 10.1002/anie.201206079
Holkenbrink, C., Ding, B. J., Wang, H. L., Dam, M. I., Petkevicius, K., Kildegaard, K. R., et al. (2020). Production of moth sex pheromones for pest control by yeast fermentation. Metab. Eng. 62, 312–321. doi: 10.1016/j.ymben.2020.10.001
Hu, Y., Zhu, Z., Gradischnig, D., Winkler, M., Nielsen, J., and Siewers, V. (2020). Engineering carboxylic acid reductase for selective synthesis of medium-chain fatty alcohols in yeast. Proc. Natl. Acad. Sci. U. S. A. 117, 22974–22983. doi: 10.1073/pnas.2010521117
Ioriatti, C., and Lucchi, A. (2016). Semiochemical strategies for tortricid moth control in apple orchards and vineyards in Italy. J. Chem. Ecol. 42, 571–583. doi: 10.1007/s10886-016-0722-y
Jung, C. R., and Kim, Y. (2014). Comparative transcriptome analysis of sex pheromone glands of two sympatric lepidopteran congener species. Genomics 103, 308–315. doi: 10.1016/j.ygeno.2014.02.009
Jurenka, R. (2017). Regulation of pheromone biosynthesis in moths. Curr. Opin. Insect Sci. 24, 29–35. doi: 10.1016/j.cois.2017.09.002
Karlson, P., and Luscher, M. (1959). Pheromones’: a new term for a class of biologically active substances. Nature 183, 55–56. doi: 10.1038/183055a0
Kozak, B. U., Van Rossum, H. M., Luttik, M. A., Akeroyd, M., Benjamin, K. R., Wu, L., et al. (2014). Engineering acetyl coenzyme A supply: functional expression of a bacterial pyruvate dehydrogenase complex in the cytosol of Saccharomyces cerevisiae. mBio 5, e01696–14. doi: 10.1128/mBio.01696-14
Krivoruchko, A., Zhang, Y., Siewers, V., Chen, Y., and Nielsen, J. (2015). Microbial acetyl-CoA metabolism and metabolic engineering. Metab. Eng. 28, 28–42. doi: 10.1016/j.ymben.2014.11.009
Lassance, J. M., Groot, A. T., Liénard, M. A., Antony, B., Borgwardt, C., Andersson, F., et al. (2010). Allelic variation in a fatty-acyl reductase gene causes divergence in moth sex pheromones. Nature 466, 486–489. doi: 10.1038/nature09058
Leber, C., Polson, B., Fernandez-Moya, R., and Da Silva, N. A. (2015). Overproduction and secretion of free fatty acids through disrupted neutral lipid recycle in Saccharomyces cerevisiae. Metab. Eng. 28, 54–62. doi: 10.1016/j.ymben.2014.11.006
Li, X., Guo, D., Cheng, Y., Zhu, F., Deng, Z., and Liu, T. (2014). Overproduction of fatty acids in engineered Saccharomyces cerevisiae. Biotechnol. Bioeng. 111, 1841–1852. doi: 10.1002/bit.25239
Liénard, M. A., Hagström, A. K., Lassance, J. M., and Löfstedt, C. (2010). Evolution of multicomponent pheromone signals in small ermine moths involves a single fatty-acyl reductase gene. Proc. Natl. Acad. Sci. U. S. A. 107, 10955–10960. doi: 10.1073/pnas.1000823107
Lin, X., Wang, B., and Du, Y. (2018). Key genes of the sex pheromone biosynthesis pathway in female moths are required for pheromone quality and possibly mediate olfactory plasticity in conspecific male moths in Spodoptera litura. Insect Mol. Biol. 27, 8–21. doi: 10.1111/imb.12335
Martin, C. E., Oh, C. S., and Jiang, Y. (2007). Regulation of long chain unsaturated fatty acid synthesis in yeast. Biochim. Biophys. Acta 1771, 271–285. doi: 10.1016/j.bbalip.2006.06.010
Matsumoto, S. (2010). Molecular mechanisms underlying sex pheromone production in moths. Biosci. Biotechnol. Biochem. 74, 223–231. doi: 10.1271/bbb.90756
Matsumoto, S., Hull, J. J., Ohnishi, A., Moto, K., and Fónagy, A. (2007). Molecular mechanisms underlying sex pheromone production in the silkmoth, Bombyx mori: characterization of the molecular components involved in bombykol biosynthesis. J. Insect Physiol. 53, 752–759. doi: 10.1016/j.jinsphys.2007.02.014
Michinaka, Y., Shimauchi, T., Aki, T., Nakajima, T., Kawamoto, S., Shigeta, S., et al. (2003). Extracellular secretion of free fatty acids by disruption of a fatty acyl-CoA synthetase gene in Saccharomyces cerevisiae. J. Biosci. Bioeng. 95, 435–440. doi: 10.1016/s1389-1723(03)80041-5
Moto, K., Yoshiga, T., Yamamoto, M., Takahashi, S., Okano, K., Ando, T., et al. (2003). Pheromone gland-specific fatty-acyl reductase of the silkmoth, Bombyx mori. Proc. Natl. Acad. Sci. U. S. A. 100, 9156–9161. doi: 10.1073/pnas.1531993100
Oh, C. S., Toke, D. A., Mandala, S., and Martin, C. E. (1997). ELO2 and ELO3, homologues of the Saccharomyces cerevisiae ELO1 gene, function in fatty acid elongation and are required for sphingolipid formation. J. Biol. Chem. 272, 17376–17384. doi: 10.1074/jbc.272.28.17376
Ono, A., Imai, T., Inomata, S., Watanabe, A., and Ando, T. (2002). Biosynthetic pathway for production of a conjugated dienyl sex pheromone of a Plusiinae moth, Thysanoplusia intermixta. Insect Biochem. Mol. Biol. 32, 701–708. doi: 10.1016/s0965-1748(01)00154-0
Petkevicius, K., Koutsoumpeli, E., Betsi, P. C., Ding, B. J., Kildegaard, K. R., Jensen, H., et al. (2021). Biotechnological production of the European corn borer sex pheromone in the yeast Yarrowia lipolytica. Biotechnol. J. 16:e2100004. doi: 10.1002/biot.202100004
Raina, A. K., Wergin, W. P., Murphy, C. A., and Erbe, E. F. (2000). Structural organization of the sex pheromone gland in Helicoverpa zea in relation to pheromone production and release. Arthropod Struct. Dev. 29, 343–353. doi: 10.1016/s1467-8039(01)00014-7
Reddy, G. V., and Guerrero, A. (2010). New pheromones and insect control strategies. Vitam. Horm. 83, 493–519. doi: 10.1016/S0083-6729(10)83020-1
Roscoe, L. E., Silk, P., and Eveleigh, E. S. (2016). Evidence of male hair pencil pheromone in Choristoneura fumiferana (Lepidoptera: tortricidae). J. Insect. Sci. 16:27. doi: 10.1093/jisesa/iew010
Rössler, H., Rieck, C., Delong, T., Hoja, U., and Schweizer, E. (2003). Functional differentiation and selective inactivation of multiple Saccharomyces cerevisiae genes involved in very-long-chain fatty acid synthesis. Mol. Genet. Genomics 269, 290–298. doi: 10.1007/s00438-003-0836-0
Runguphan, W., and Keasling, J. D. (2014). Metabolic engineering of Saccharomyces cerevisiae for production of fatty acid-derived biofuels and chemicals. Metab. Eng. 21, 103–113. doi: 10.1016/j.ymben.2013.07.003
Scharnewski, M., Pongdontri, P., Mora, G., Hoppert, M., and Fulda, M. (2008). Mutants of Saccharomyces cerevisiae deficient in acyl-CoA synthetases secrete fatty acids due to interrupted fatty acid recycling. FEBS J. 275, 2765–2778. doi: 10.1111/j.1742-4658.2008.06417.x
Shan, L., Dai, Z., and Wang, Q. (2021). Advances and opportunities of CRISPR/Cas technology in bioengineering non-conventional yeasts. Front. Bioeng. Biotechnol. 9:765396. doi: 10.3389/fbioe.2021.765396
Shi, S., Chen, Y., Siewers, V., and Nielsen, J. (2014). Improving production of malonyl coenzyme A-derived metabolites by abolishing Snf1-dependent regulation of Acc1. mBio 5, e01130–14. doi: 10.1128/mBio.01130-14
Shiba, Y., Paradise, E. M., Kirby, J., Ro, D. K., and Keasling, J. D. (2007). Engineering of the pyruvate dehydrogenase bypass in Saccharomyces cerevisiae for high-level production of isoprenoids. Metab. Eng. 9, 160–168. doi: 10.1016/j.ymben.2006.10.005
Shin, G. H., Veen, M., Stahl, U., and Lang, C. (2012). Overexpression of genes of the fatty acid biosynthetic pathway leads to accumulation of sterols in Saccharomyces cerevisiae. Yeast 29, 371–383. doi: 10.1002/yea.2916
Sun, L., Wang, Q., Zhang, Y., Tu, X., Yan, Y., Wang, Q., et al. (2019). The sensilla trichodea-biased EoblPBP1 binds sex pheromones and green leaf volatiles in Ectropis obliqua Prout, a geometrid moth pest that uses Type-II sex pheromones. J. Insect Physiol. 116, 17–24. doi: 10.1016/j.jinsphys.2019.04.005
Tan, L., Zheng, Z., Xu, Y., Kong, W., Dai, Z., Qin, X., et al. (2020). Efficient selection scheme for incorporating noncanonical amino acids into proteins in Saccharomyces cerevisiae. Front. Bioeng. Biotechnol. 8:569191. doi: 10.3389/fbioe.2020.569191
Tehlivets, O., Scheuringer, K., and Kohlwein, S. D. (2007). Fatty acid synthesis and elongation in yeast. Biochim. Biophys. Acta 1771, 255–270. doi: 10.1016/j.bbalip.2006.07.004
Tillman, J. A., Seybold, S. J., Jurenka, R. A., and Blomquist, G. J. (1999). Insect pheromones–an overview of biosynthesis and endocrine regulation. Insect Biochem. Mol. Biol. 29, 481–514. doi: 10.1016/s0965-1748(99)00016-8
Tupec, M., Buček, A., Janoušek, V., Vogel, H., Prchalová, D., Kindl, J., et al. (2019). Expansion of the fatty acyl reductase gene family shaped pheromone communication in Hymenoptera. Elife 8:e39231. doi: 10.7554/eLife.39231
Turczel, G., Kovács, E., Merza, G., Coish, P., and Tuba, R. (2018). Synthesis of semiochemicals via olefin metathesis. ACS Sustain. Chem. Eng. 7, 33–48. doi: 10.1021/acs.joc.7b02944
Vasconcelos, B., Teixeira, J. C., Dragone, G., and Teixeira, J. A. (2019). Oleaginous yeasts for sustainable lipid production-from biodiesel to surf boards, a wide range of “green” applications. Appl. Microbiol. Biotechnol. 103, 3651–3667. doi: 10.1007/s00253-019-09742-x
Wang, H. L., Liénard, M. A., Zhao, C. H., Wang, C. Z., and Löfstedt, C. (2010a). Neofunctionalization in an ancestral insect desaturase lineage led to rare Δ6 pheromone signals in the Chinese tussah silkworm. Insect Biochem. Mol. Biol. 40, 742–751. doi: 10.1016/j.ibmb.2010.07.009
Wang, H. L., Zhao, C. H., Millar, J. G., Cardé, R. T., and Löfstedt, C. (2010b). Biosynthesis of unusual moth pheromone components involves two different pathways in the navel orangeworm, Amyelois transitella. J. Chem. Ecol. 36, 535–547. doi: 10.1007/s10886-010-9777-3
Wang, J., Ledesma-Amaro, R., Wei, Y., Ji, B., and Ji, X.-J. (2020). Metabolic engineering for increased lipid accumulation in Yarrowia lipolytica - A Review. Bioresour. Technol. 313:123707. doi: 10.1016/j.biortech.2020.123707
Wang, M., Wei, Y., Ji, B., and Nielsen, J. (2020). Advances in metabolic engineering of Saccharomyces cerevisiae for cocoa butter equivalent production. Front. Bioeng. Biotechnol. 8:594081. doi: 10.3389/fbioe.2020.594081
Wei, Y., Bergenholm, D., Gossing, M., Siewers, V., and Nielsen, J. (2018). Expression of cocoa genes in Saccharomyces cerevisiae improves cocoa butter production. Microb. Cell Fact. 17:11. doi: 10.1186/s12934-018-0866-2
Wei, Y., Siewers, V., and Nielsen, J. (2017b). Cocoa butter-like lipid production ability of non-oleaginous and oleaginous yeasts under nitrogen-limited culture conditions. Appl. Microbiol. Biotechnol. 101, 3577–3585. doi: 10.1007/s00253-017-8126-7
Wei, Y., Gossing, M., Bergenholm, D., Siewers, V., and Nielsen, J. (2017a). Increasing cocoa butter-like lipid production of Saccharomyces cerevisiae by expression of selected cocoa genes. AMB Express 7, 34–34. doi: 10.1186/s13568-017-0333-1
Wei, Y., Ji, B., Siewers, V., Xu, D., Halkier, B. A., and Nielsen, J. (2019). Identification of genes involved in shea butter biosynthesis from Vitellaria paradoxa fruits through transcriptomics and functional heterologous expression. Appl. Microbiol. Biotechnol. 103, 3727–3736. doi: 10.1007/s00253-019-09720-3
Wenning, L., Yu, T., David, F., Nielsen, J., and Siewers, V. (2017). Establishing very long-chain fatty alcohol and wax ester biosynthesis in Saccharomyces cerevisiae. Biotechnol. Bioeng. 114, 1025–1035. doi: 10.1002/bit.26220
Witzgall, P., Kirsch, P., and Cork, A. (2010). Sex pheromones and their impact on pest management. J. Chem. Ecol. 36, 80–100. doi: 10.1007/s10886-009-9737-y
Xue, H., Sun, W., Wang, Y., and Li, C. (2021). Refining metabolic mass transfer for efficient biosynthesis of plant natural products in yeast. Front. Bioeng. Biotechnol. 9:633741. doi: 10.3389/fbioe.2021.633741
Yang, Y., Tao, J., and Zong, S. (2020). Identification of putative Type-I sex pheromone biosynthesis-related genes expressed in the female pheromone gland of Streltzoviella insularis. PLoS One 15:e0227666. doi: 10.1371/journal.pone.0227666
Yew, J. Y., and Chung, H. (2015). Insect pheromones: an overview of function, form, and discovery. Prog. Lipid. Res. 59, 88–105. doi: 10.1016/j.plipres.2015.06.001
Yu, T., Zhou, Y. J., Huang, M., Liu, Q., Pereira, R., David, F., et al. (2018). Reprogramming yeast metabolism from alcoholic fermentation to lipogenesis. Cell 174, 1549–1558. doi: 10.1016/j.cell.2018.07.013
Yu, T., Zhou, Y. J., Wenning, L., Liu, Q., Krivoruchko, A., Siewers, V., et al. (2017). Metabolic engineering of Saccharomyces cerevisiae for production of very long chain fatty acid-derived chemicals. Nat. Commun. 8:15587. doi: 10.1038/ncomms15587
Zhang, Y., Nielsen, J., and Liu, Z. (2018). Metabolic engineering of Saccharomyces cerevisiae for production of fatty acid-derived hydrocarbons. Biotechnol. Bioeng. 115, 2139–2147. doi: 10.1002/bit.26738
Zhou, Y. J., Buijs, N. A., Zhu, Z., Qin, J., Siewers, V., and Nielsen, J. (2016). Production of fatty acid-derived oleochemicals and biofuels by synthetic yeast cell factories. Nat. Commun. 7:11709. doi: 10.1038/ncomms11709
Keywords: insect sex pheromone, fatty acids, Saccharomyces cerevisiae, metabolic engineering, synthetic biology
Citation: Zhang X, Miao Q, Xu X, Ji B, Qu L and Wei Y (2021) Developments in Fatty Acid-Derived Insect Pheromone Production Using Engineered Yeasts. Front. Microbiol. 12:759975. doi: 10.3389/fmicb.2021.759975
Received: 06 September 2021; Accepted: 26 October 2021;
Published: 11 November 2021.
Edited by:
Yinjie Tang, Washington University in St. Louis, United StatesReviewed by:
Xiaochao Xiong, Washington State University, United StatesCopyright © 2021 Zhang, Miao, Xu, Ji, Qu and Wei. This is an open-access article distributed under the terms of the Creative Commons Attribution License (CC BY). The use, distribution or reproduction in other forums is permitted, provided the original author(s) and the copyright owner(s) are credited and that the original publication in this journal is cited, in accordance with accepted academic practice. No use, distribution or reproduction is permitted which does not comply with these terms.
*Correspondence: Yongjun Wei, eW9uZ2p1bndlaUB6enUuZWR1LmNu
Disclaimer: All claims expressed in this article are solely those of the authors and do not necessarily represent those of their affiliated organizations, or those of the publisher, the editors and the reviewers. Any product that may be evaluated in this article or claim that may be made by its manufacturer is not guaranteed or endorsed by the publisher.
Research integrity at Frontiers
Learn more about the work of our research integrity team to safeguard the quality of each article we publish.