- 1Servicio de Microbiología del Complejo Hospitalario Universitario de A Coruña (CHUAC), Instituto de Investigación Biomédica de A Coruña (INIBIC), A Coruña, Spain
- 2Microbiome and Health, Faculty of Science, University of A Coruña, A Coruña, Spain
- 3School of Chemistry and Molecular Biosciences, University of Queensland, Brisbane, QLD, Australia
- 4Centro de Investigaciones Científicas Avanzadas (CICA) y Departamento de Química, Facultad de Ciencias, Agrupación Estratégica CICA-INIBIC, Universidad de A Coruña, A Coruña, Spain
Acinetobacter baumannii is a multidrug-resistant pathogen that represents a serious threat to global health. A. baumannii possesses a wide range of virulence factors that contribute to the bacterial pathogenicity. Among them, the siderophore acinetobactin is one of the most important, being essential for the development of the infection. In this study we performed an in-depth analysis of the acinetobactin cluster in the strain A. baumannii ATCC 17978. For this purpose, nineteen individual isogenic mutant strains were generated, and further phenotypical analysis were performed. Individual mutants lacking the biosynthetic genes entA, basG, basC, basD, and basB showed a significant loss in virulence, due to the disruption in the acinetobactin production. Similarly, the gene bauA, coding for the acinetobactin receptor, was also found to be crucial for the bacterial pathogenesis. In addition, the analysis of the ΔbasJ/ΔfbsB double mutant strain demonstrated the high level of genetic redundancy between siderophores where the role of specific genes of the acinetobactin cluster can be fulfilled by their fimsbactin redundant genes. Overall, this study highlights the essential role of entA, basG, basC, basD, basB and bauA in the pathogenicity of A. baumannii and provides potential therapeutic targets for the design of new antivirulence agents against this microorganism.
Introduction
Acinetobacter baumannii is one of the most common nosocomial pathogens responsible for a wide range of concerning diseases, such as pneumonia, bacteremia or secondary meningitis (Wong et al., 2017). The rise of healthcare-associated infections caused by multidrug resistant strains of A. baumannii, together with the scarce development of new antimicrobials in the last decades, represents an important health threat (De Oliveira et al., 2020). In fact, A. baumannii, is one of the Gram-negative ESKAPE pathogens identified by the World Health Organization (WHO) as critical priority for antibiotic discovery (World Health Organization [WHO], 2017).
Although the main characteristic of this pathogen is its ability to acquire new antimicrobial resistance, it shows several mechanisms involved in virulence, persistence and stress adaptation that enhance its pathogenicity (Harding et al., 2018). Within this context, many researchers have focused their efforts in developing alternatives to conventional antibiotics, such as antivirulence agents, that can work alone or together with antibiotics to overcome A. baumannii infections (Dickey et al., 2017).
Iron is an essential micronutrient for bacteria to infect and multiply in tissues and body fluids of the host, playing an important role in pathogenesis. The mechanisms of bacterial iron acquisition include: (i) expression of transporters involved in the uptake of ferrous iron, such as the Feo system; (ii) extraction of heme-iron from hemoproteins; (iii) capture of iron from transferrin and lactoferrin; or (iv) synthesis of siderophores (Sheldon et al., 2016). Siderophores are high-affinity iron-chelating molecules synthetized by microorganisms to scavenge extracellular ferric iron from the environment. Baumanoferrin, fimsbactin and preacinetobactin-acinetobactin (referred to as acinetobactin) are the most common siderophore systems detected in A. baumannii (Yamamoto et al., 1994; Proschak et al., 2013; Penwell et al., 2015). The most extensively studied is acinetobactin which is considered the major siderophore of A. baumannii and it is highly conserved among all A. baumannii strains (Antunes et al., 2011). All the genes required for the synthesis (basA-J), efflux (barA/B) and uptake (bauA-F) of acinetobactin are located in a 26.5-kb chromosomal region (Supplementary Figure 1A) (Mihara et al., 2004), with the exception of the entA homolog gene, found elsewhere in the chromosome (Penwell et al., 2012). Its biosynthesis follows the logic of a non-ribosomal peptide synthetase (NRPS) assembly system, where three precursors are bound in equimolar quantities into the preacinetobactin molecule: N-hydroxyhistamine, L-threonine and 2,3-dihydroxybenzoic acid (DHBA) (Figure 1A) (Yamamoto et al., 1994; Song and Kim, 2020). Once the preacinetobactin synthesis is completed, the siderophore is secreted to the extracellular space (Figure 1B) where two reactions can occur: (i) preacinetobactin stabilization by chelation of ferric iron or (ii) non-enzymatically and irreversibly isomerization to acinetobactin at pH > 7 (Shapiro and Wencewicz, 2016; Moynié et al., 2018). The fimsbactins A-F siderophores are present in a small fraction of the A. baumannii isolates (Antunes et al., 2011; Proschak et al., 2013). These siderophores, also derived from a NRPS assembly system, are structurally related to acinetobactin by the presence of catecholate, phenolate oxazoline, and hydroxamate metal-binding motifs (Proschak et al., 2013). Moreover, the cluster fbsA-Q, coding for the fimsbactins, consists of 18 genes with high functional similarity to those present in the acinetobactin cluster indicating redundancy between both siderophores pathways (Supplementary Figure 1B) (Dorsey et al., 2004; Mihara et al., 2004; Proschak et al., 2013). Although the expression of fimsbactins was shown to be enough to support the growth of A. baumannii in serum, these siderophores are not required for survival during bacteremia (Sheldon and Skaar, 2020).
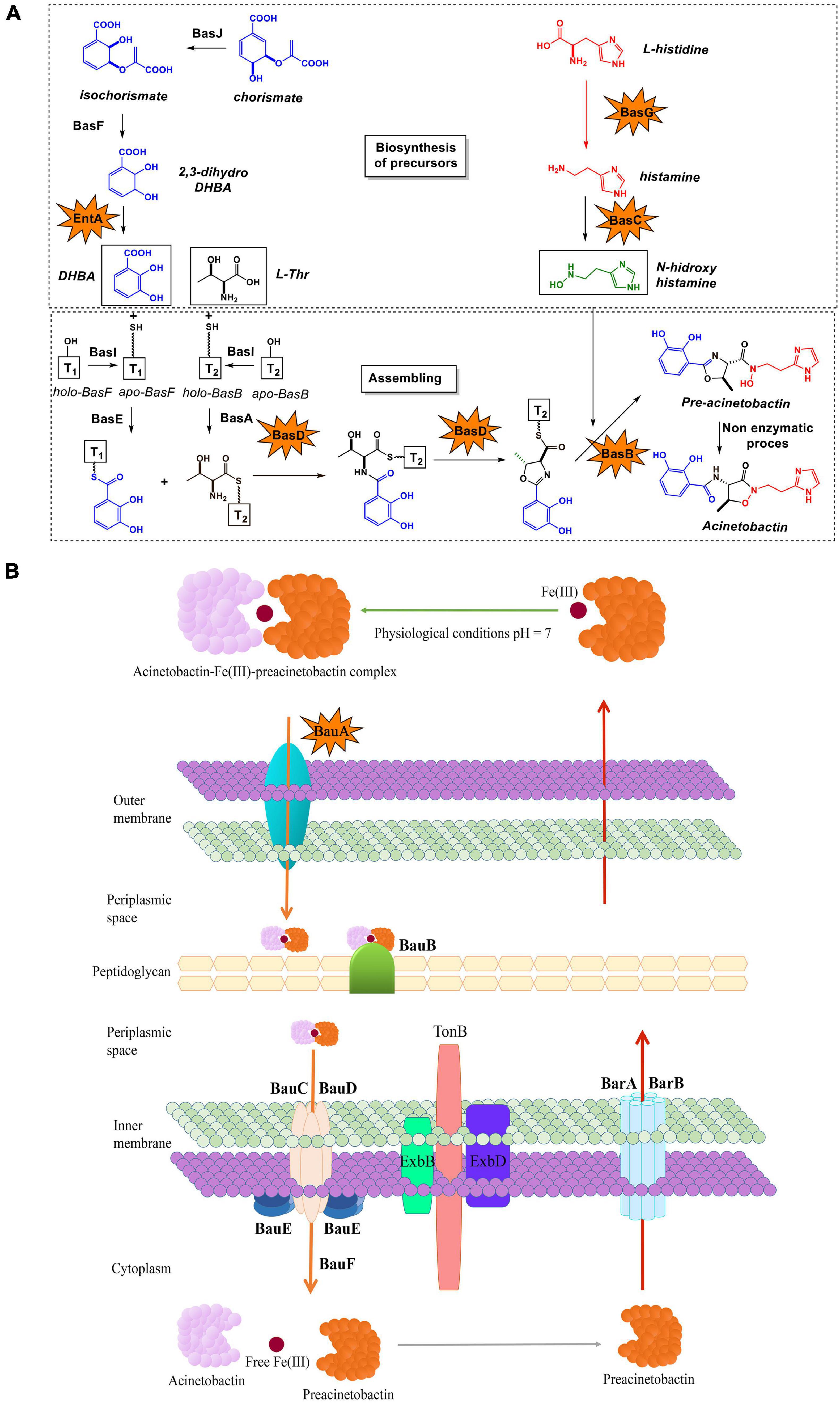
Figure 1. Proposed (A) biosynthetic pathway and (B) transport mechanism of acinetobactin in A. baumannii. The six proteins found to be essential for the development of the bacteremia infection are marked with stars.
Indeed, acinetobactin has been shown to be essential for the virulence of A. baumannii during greater wax moth (Galleria mellonella) and murine bacteremia and pneumonia infections (Gaddy et al., 2012; Penwell et al., 2012; Martínez-Guitián et al., 2020; Sheldon and Skaar, 2020). However, no studies have explored the contribution of the entire collection of acinetobactin genes to the infectious process.
Herein, we have performed an in-depth analysis of the role of the acinetobactin cluster in the virulence of A. baumannii which allowed us to identify potential targets for the design of new antimicrobials against this pathogen.
Materials and Methods
Bacterial Strains and Culture Conditions
All A. baumannii and Escherichia coli strains used in this study are listed in Table 1. Bacteria were grown routinely at 37°C in solid and liquid Luria-Bertani (LB) medium and stored at −80°C in LB broth containing 20% glycerol. When appropriate, media was supplemented with 50 μg/mL of kanamycin (Kan).
Construction of Isogenic Mutant Derivative Strains
All mutants were generated using the suicide vector pMo130 (Genbank: EU862243) as previously described (Álvarez-Fraga et al., 2016). Briefly, a PCR was performed to amplify both upstream and downstream regions flanking each gene of interest and cloned into the pMo130 vector. The plasmid constructions were electroporated into the wild-type strain A. baumannii ATCC 17978. Recombinant colonies representing the first crossover event were selected as previously described (Hamad et al., 2009). The second crossover event leading to gene knockout was confirmed by PCR followed by sequencing. All the primers used for the mutant construction are listed in the Supplementary Table 1.
Growth Rate Analysis Under Normal and Iron-Limiting Conditions
Growth rates were assessed by measuring the optical density (OD) of the A. baumannii ATCC 17978 parental strain and the mutant derivative strains in Mueller Hinton II (MH) medium, in the presence (iron-limiting conditions) or absence (normal conditions) of 0.2 mM of the iron chelator 2,2′-bipyridyl (BIP), as previously described (Álvarez-Fraga et al., 2018). Growth was monitored at OD600 every 20 min until the late-log phase in 48-well plates using the Epoch 2 Microplate Spectrophotometer (BioTek Instruments, United States). The maximum specific growth rate (μmax) and the lag time (λ) parameters were calculated using the single Gompertz growth curve model (Tjørve and Tjørve, 2017). The maximum specific growth rate parameter represents the slope of the tangent at the inflection point. The lag time parameter represents x intercept of the μmax tangent and shows the time (h) to enter exponential phase. Three independent biological replicates were carried out. Statistical analysis was performed using an unpaired, two tailed student’s t-test.
Murine Sepsis Model
A murine sepsis model was used to evaluate the virulence of the A. baumannii ATCC 17978 parental strain and the isogenic mutant derivative strains as previously described (Martínez-Guitián et al., 2019). Briefly, groups of 10 female BALB/c mice were inoculated intraperitoneally with approximately 7.5 × 107 colony forming units per mouse of exponentially grown cells and death was assessed during 168 h at 8-h intervals. The survival curves were plotted using the Kaplan-Meier method and analysed using the log-rank (Mantel-Cox) test. All experiments were carried out with the approval of and in accordance with the regulatory guidelines and standards established by the Animal Ethics Committee (Hospital Universitario A Coruña, Spain, project code P102).
Chemical Analysis of the Siderophore Content of Acinetobacter baumannii Wild-Type and Mutant Strains
The siderophore content of A. baumannii wild-type and the mutant strains was analyzed by using our SPE-HLB/HPLC-HRMS methodology (Espada et al., 2011) adapted for the isolation of acinetobactin (Balado et al., 2015) and detecting the presence of iron(III) chelating compounds using the Chrome Azurol-S Liquid (CAS) assay. Briefly, bacteria were grown at 37°C in M9 minimal media supplemented with 0.2% casamino acids and 0.4% glucose until an OD600 = 1.0. Subsequently, the bacterial suspensions were pelleted, filtered and the resultant cell-free supernatants were freeze-dried to obtain 2.5 g of a residue. One gram of this material was dissolved in milli-Q water (1 mL), loaded in an OASIS HLB cartridge (6 g, 35 cm3, Waters), which was previously conditioned and equilibrated with 120 mL of acetonitrile (solvent B) and water (solvent A), each containing 0.1% TFA (v/v), and fractionated with 1:0, 9:1, 8:2, 7:3, and 0:1 of A:B (v/v) to give ABLH1-5 fractions, respectively. CAS-positive fractions were further analyzed by HPLC (Thermo Scientific) coupled to a PDA detector, monitoring the absorbance at λ = 254, 280 and 313 nm, and to a MSQ plus mass spectrometer in full positive ion mode. The analysis was carried out using a Discovery HS-F5 column (100 × 4.6 m, 5 μm), with a flow of 1 mL/min and the following gradient conditions: acetonitrile (solvent B) and water (solvent A), each containing 0.1% TFA (v/v), 40 min from 10 to 50% of B, 5 min from 50 to 100% of B, a 5 min of an isocratic step at 100% of B, 5 min from 100 to 10% of B and final 5 min of an isocratic step at 10% of B. HPLC/HRMS analysis of the ABLH3 fraction showed the presence of a chromatographic peak with a rt = 11.75 min, which presented a [M + H]+ adduct in its corresponding (+)-HR-ESIMS at m/z 347.1344 that agreed to that of acinetobactin (calcd. for C16H19N4O5, m/z 347.1350). In parallel, fimsbactins A and F were detected in the chromatographic peak with a rt = 19.4 min of ABLH5 by displaying the [M + H]+ adducts at m/z 575.1956 (calcd. for C26H31N4O11, 575.1989) and m/z 439.1803 (calcd. for C19H27N4O8, 439.1829) in the (+)-HR-ESIMS, respectively. Analogs of fimsbactins A and F, where the oxazoline ring is opened, were also identified in the chromatographic peak with a rt = 13.4 min by showing the [M + H]+ adducts at m/z 593.2063 (calcd. for C26H33N4O12, 575.2089) and m/z 457.1909 (calcd. for C19H29N4O8, 457.1229) in their (+)-HR-ESIMS. These analogs were formed from fimsbactins A and F due to the acidic conditions used for separation. Indeed, these compounds were not obtained when the same SPE-HLB/HPLC-MS methodology was used avoiding acidic conditions.
Results
Specific Genes of the Acinetobactin Cluster Are Relevant for the Growth of Acinetobacter baumannii ATCC 17978 Under Iron-Limiting Conditions
To determine the contribution of each gene of the acinetobactin cluster to the growth of A. baumannii ATCC 17978, a total of 19 individual isogenic mutant strains were generated (Figure 1, Table 1, Supplementary Figure 1, and Supplementary Table 2) and growth curves were performed in absence (normal conditions) and presence (iron-limiting conditions) of the iron (III) 2,2′- bipyridyl (BIP). The eleven mutant strains lacking the genes involved in the biosynthesis of acinetobactin were classified in three different groups: (i) genes involved in the synthesis of the DHBA precursor (basJ, basF, and entA), (ii) genes involved in the synthesis of the N-hydroxyhistamine precursor (basG and basC) and (iii) genes involved in the modification and assembly of the acinetobactin precursors into the final molecule (basI, basH, basD, basB, basA, and basE) (Figure 1A). The growth curves under iron-limiting conditions of the strains lacking the genes involved in the DHBA synthesis revealed that the ΔentA mutant was impaired for growth, with a lower maximum specific growth rate (μmax) (0.24, P = 0.0008) and a higher lag time (λ) (4.2, P = 0.0129) than the parental strain (μmax = 0.74 and λ = 1.36). The deletion of basF also resulted in a slightly decrease of μmax (0.49, P = 0.0119) and λ (0.81, P = 0.0423) (Figure 2A and Table 2). The mutant strains ΔbasG and ΔbasC, lacking genes involved in the synthesis of the N-hydroxyhistamine precursor, displayed a significant reduction in the μmax (0.48, P = 0.0084 and 0.43, P = 0.0061; respectively) and an increase in the λ (3.7, P = 0.0002 and 3.9, P = 0.0001; respectively) when compared to the wild-type strain (Figure 2B and Table 2). Among the mutants belonging to the third group, ΔbasD, ΔbasB, and ΔbasA grew poorly under iron-limitation conditions, showing significant lower μmax (0.49, P = 0.01; 0.46, P = 0.0072 and 0.30, P = 0.0012; respectively). The ΔbasD and ΔbasB mutants also shown higher lag times (λ = 3.99, P = 0.0002 and λ = 4.86, P < 0.0001; respectively) compared to the wild-type strain (Figure 2C and Table 2). No significant differences in growth kinetics were observed in the other isogenic mutant strains [P (λ) = 0.16–0.82, P (μmax) = 0.09–0.74] compared to the wild-type strain ATCC 17978 (Figure 2). Under normal conditions, the eleven isogenic mutant strains showed similar growth abilities compared to the wild-type strain ATCC 17978 (Supplementary Figure 2A).
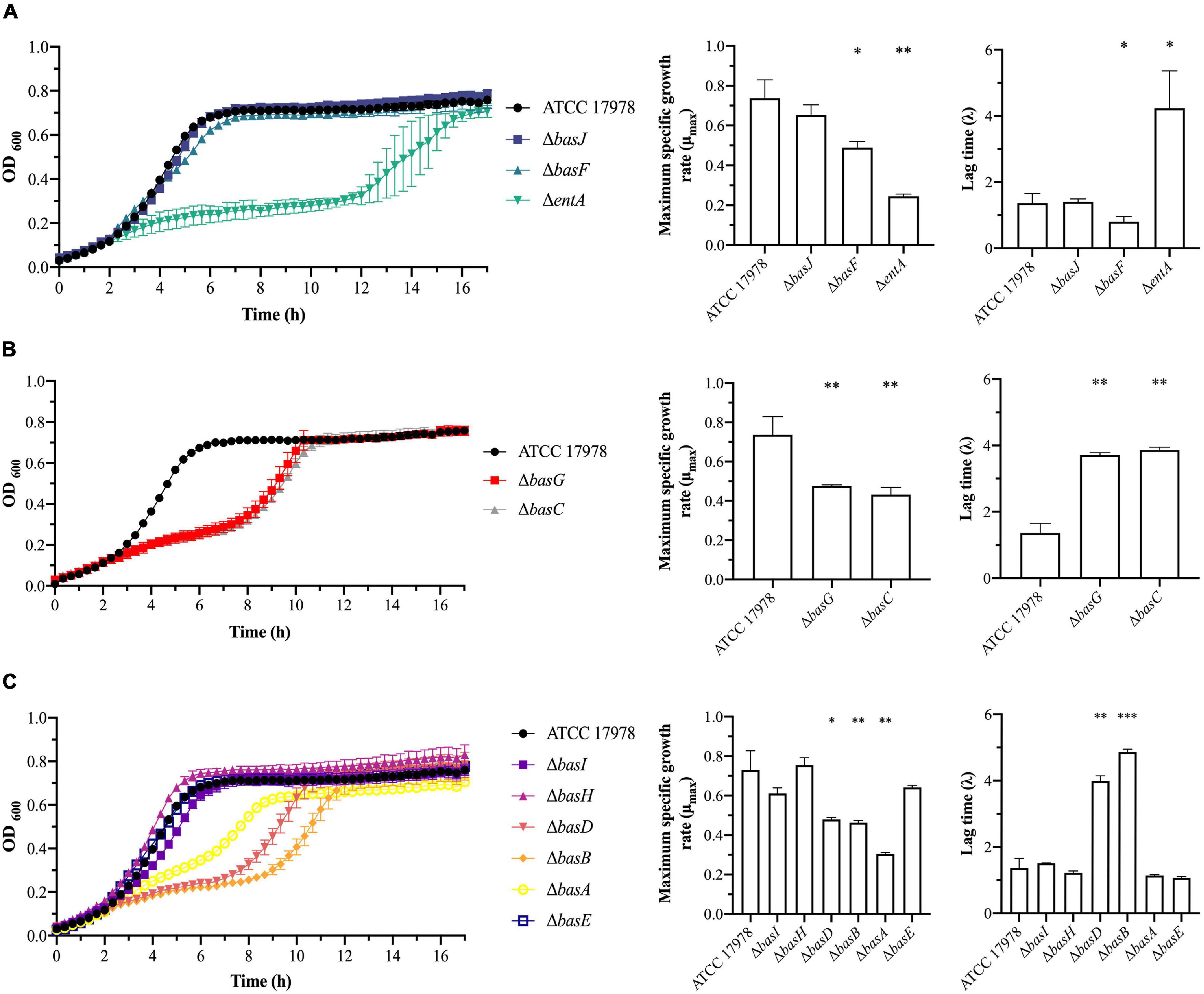
Figure 2. Growth curves and growth kinetics of A. baumannii ATCC 17978 and its isogenic mutant derivative lacking (A) genes involved in the synthesis of the DHBA precursor, (B) genes involved in the synthesis of the N-hydroxyhistamine precursor and (C) genes involved in the modification and assembly of the acinetobactin precursors into the final molecule. The growth curves were performed under iron-limiting conditions. Three independent biological replicates were performed. Unpaired student t test was used for the statistical analysis of the growth kinetics (∗P < 0.05; ∗∗P < 0.01; ∗∗∗P < 0.0001).
In parallel, we performed growth curves with the eight isogenic mutant derivative strains, lacking each of the influx and efflux related genes, under both normal and iron-limiting conditions. As it was observed in the mutant strains related to the acinetobactin biosynthesis, no significant differences were detected between the isogenic mutant strains and the wild-type strain under normal growth conditions (Supplementary Figure 2B). Nevertheless, under iron-limited conditions, the deletion of the genes barA, barB (efflux), and bauC (uptake) resulted in a significant growth inhibition shown as a reduction in the μmax (0.34, P = 0.0018; 0.30, P = 0.0013 and 0.55, P = 0.0241; respectively) and an increase in the λ (3.99, P = 0.0001; 4.11, P < 0.0001 and 2.3, P < 0.0051; respectively) (Figure 3 and Table 2). We also observed a partial reduction of the growth of ΔbauD (μmax = 0.56, P = 0.0320), ΔbauE (μmax = 0.49, P = 0.0128) and ΔbauB (λ = 1.91, P < 0.0321) mutant strains growth. No significant differences in growth kinetics were observed in the other isogenic mutant strains [P (λ) = 0.22–0.51, P (μmax) = 0.06–0.86] (Figure 3).
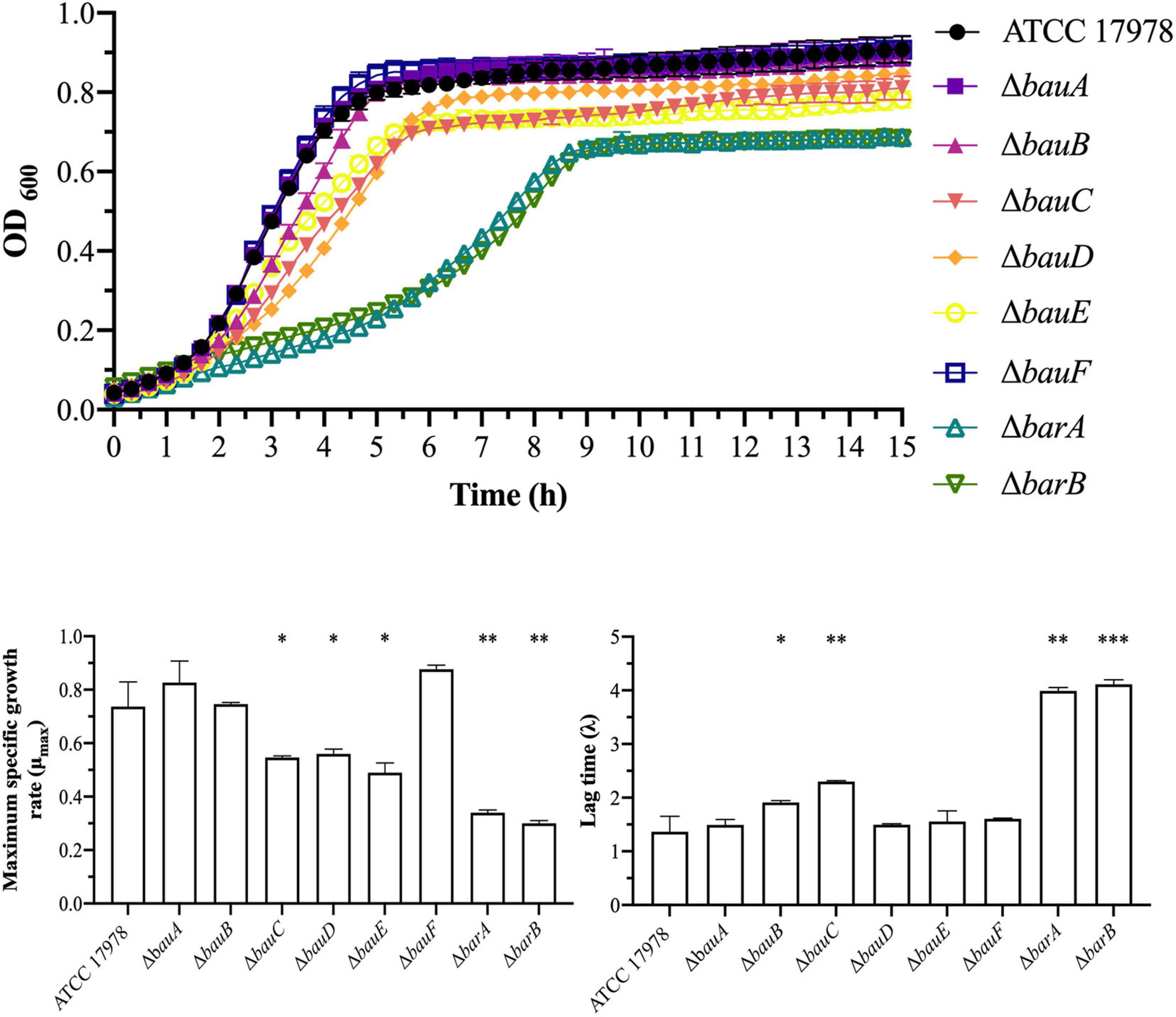
Figure 3. Growth curves and growth kinetics of A. baumannii ATCC 17978 and the isogenic mutant derivative strains lacking genes involved in the transport of acinetobactin. The growth curves were performed under iron-limiting conditions. Three independent biological replicates were performed. Unpaired student t test was used for the statistical analysis of the growth kinetics (∗P < 0.05; ∗∗P < 0.01; ∗∗∗P < 0.0001).
Six Genes of the Acinetobactin Cluster Are Essential for the Virulence in vivo
It has been previously showed that acinetobactin plays an important role in the virulence of A. baumannii (Gaddy et al., 2012; Penwell et al., 2012; Martínez-Guitián et al., 2020; Sheldon and Skaar, 2020). However, it remains unclear which genes of the acinetobactin cluster are essential for the development of the infection. To elucidate this, a murine sepsis model was performed with the wild-type and the 19 mutant derivative strains. Among the mutant strains lacking genes involved in the biosynthesis of acinetobactin, the mice infected with ΔentA (90% survival, P = 0.0009), ΔbasG (90% survival, P = 0.0004), ΔbasC (90% survival, P = 0.0009),ΔbasD (90% survival, P = 0.0004), and ΔbasB (100% survival, P < 0.0001) mutant strains showed survival rates significantly higher compared with those of the mice infected with the parental strain (10% survival) (Figures 4A–C and Table 2). No significant differences in mice survival were observed in the other isogenic mutant strains (P = 0.3068–0.9716) (Figures 4A–C).
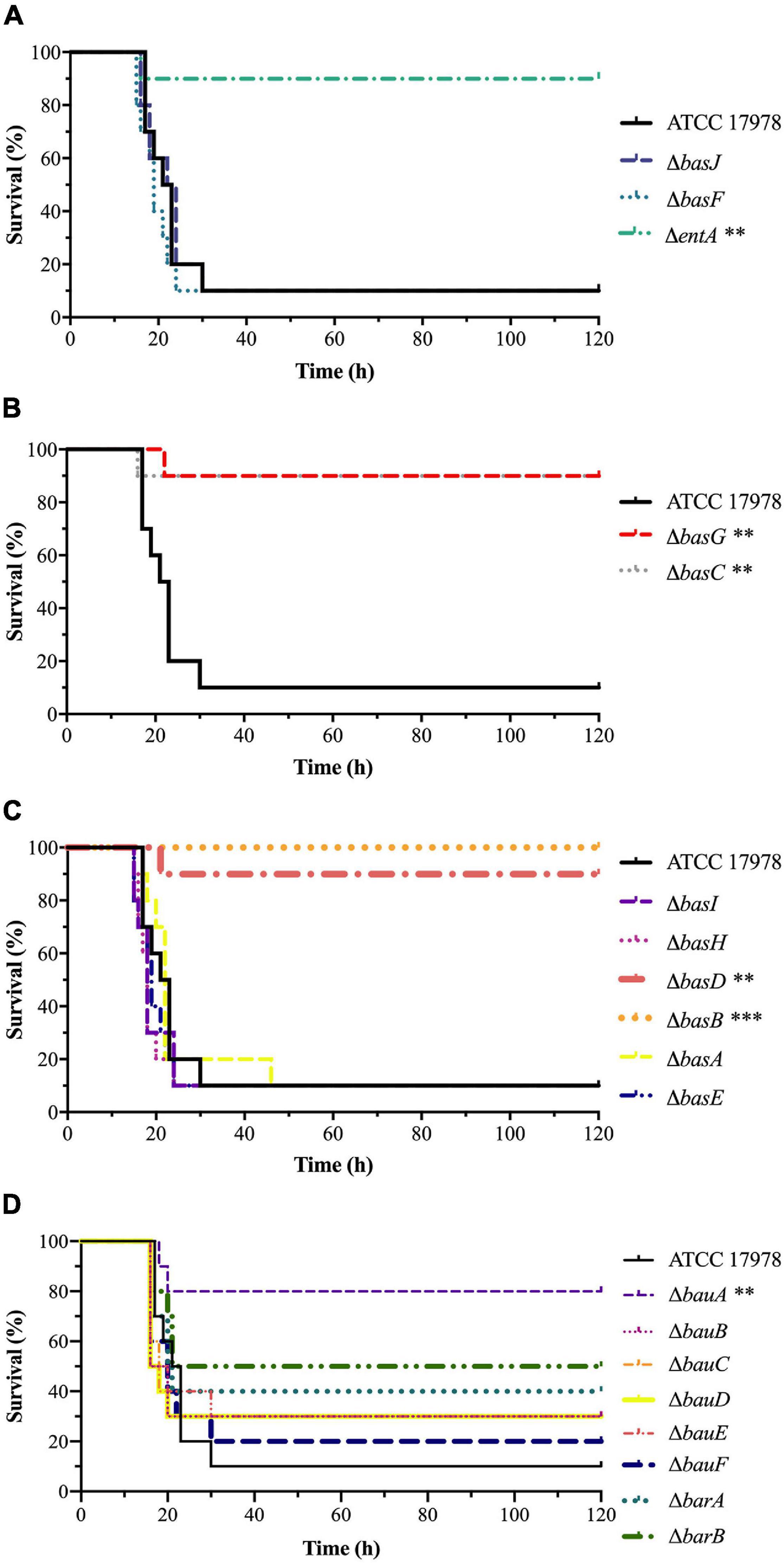
Figure 4. Sepsis infection in mice. Survival of BALB/c mice (n = 10 per group) after bacteremia infection with A. baumannii ATCC 17978 and the isogenic mutant derivative strains lacking (A) genes involved in the synthesis of the DHBA precursor, (B) genes involved in the synthesis of the N-hydroxyhistamine precursor, (C) genes involved in the modification and assembly of the acinetobactin precursors into the final molecule and (D) genes involved in the acinetobactin transport The log-rank (Mantel-Cox) test was used for statistical analysis (∗∗P < 0.01, ∗∗∗P < 0.0001).
Among the mutant strains lacking genes involved in the transport of acinetobactin, only mice infected with the ΔbauA strain showed a significant increase in the survival rate (80% survival, P = 0.0039) compared with those mice infected with the parental strain (10% survival) (Figure 4D and Table 2). No significant differences in mice survival were observed in the other isogenic mutant strains (P = 0.1926–0.9484) (Figure 4D).
Analysis of the Siderophore-Content of Acinetobacter baumannii Wild-Type and Mutant Strains
To confirm whether the deletion of specific genes in A. baumannii ATCC 17978 caused a disruption in the biosynthesis or transport of acinetobactin, we analysed the presence of this siderophore in eight isogenic mutant strains (Table 2): ΔbasB, ΔbasG, ΔbasC, ΔbasD, and ΔbasJ, related to the biosynthesis; ΔbauA and ΔbauB, related to the influx and ΔbarB, related to the efflux of acinetobactin. We employed a bio-guided fractionation based on the SPE-HLB/HPLC-MS methodology described by Balado et al. (2015), using the colorimetric CAS liquid assay for the detection of iron(III)-chelating compounds (Supplementary Figure 3A). Thus, the cell-free supernatants of interest were freeze-dried and fractionated by solid-phase extraction (SPE) using hydrophilic-lipophilic balance (HLB) cartridges (Figure 5A). HPLC/HRMS analysis of the CAS-positive fractions obtained from the wild-type strain allowed us to detect acinetobactin and fimsbactins A and F (Figure 5A). Specifically, acinetobactin was localized in the chromatographic peak with rt = 11.75 min of the fraction ABLH3 eluted from the HLB cartridge with 8:2 of H2O:CH3CN (v/v), each containing 0.1% TFA (v/v), showing a [M + H]+ ion at m/z 347 in its corresponding MS (Figures 5B,C and Supplementary Figure 4). On the other hand, fimsbactin A and F were detected in the chromatographic peak with rt = 19.4 min of ABHL5 eluted with 100% of CH3CN, containing 0.1% TFA, displaying a [M + H]+ ion at m/z 575 and 439, respectively, in their MS (Supplementary Figures 5, 6). Due to the acidic conditions of the methodology, two analogs of fimsbactin A and F, having an opened oxazoline ring, were also found in the chromatographic peak with rt = 13.4 min of this fraction, displaying a [M + H]+ ion at m/z 593 and 457, respectively (Supplementary Figures 5, 7). This was confirmed after the analysis of the analogous fraction (ABLHWA5) obtained under non-acidic conditions (Supplementary Figures 3B, 8).
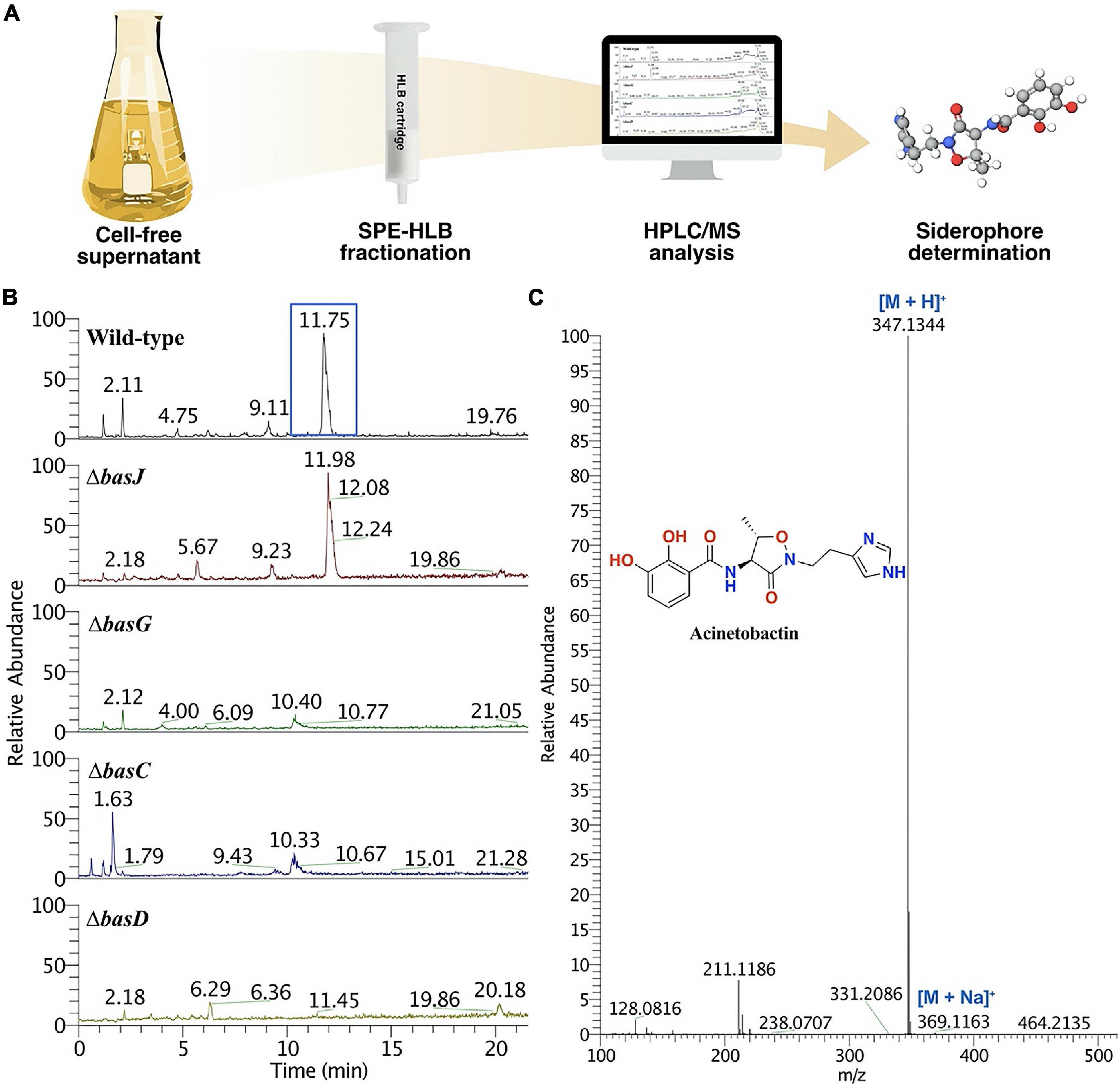
Figure 5. (A) Schematic representation of the siderophore-content isolation SPE-HLB/HPLC-MS methodology carried out in the cell-free supernatant of A. baumannii wild-type and mutant strains. (B) Comparison of the total ion current (TIC) chromatographic profiles of the ABLH3 fraction of A. baumannii wild type, in which acinetobactin was detected at rt = 11.75 min, to that of five mutant strains with depleted genes involved in the biosynthesis of acinetobactin. (C) High resolution mass spectrum of acinetobactin detected in the chromatographic peak at rt = 11.75 min of the ABLH3 fraction obtained from the A. baumannii wild-type strain.
Finally, comparison of the HPLC chromatographic profiles of ABHL3 and ABHL5 fractions from the parental strain and the former selected mutant derivative strains, revealed that those mutants lacking genes involved in the biosynthesis of acinetobactin, except for ΔbasJ, were not able to produce acinetobactin (Figure 5B, Table 2, and Supplementary Figure 9). However, the five mutant strains displayed the presence of fimsbactins A and F in their ABHL5 fractions (Table 2 and Supplementary Figure 10). In parallel, the mutant strains lacking genes involved in the influx and efflux of acinetobactin did not show any difference in comparison to the parental strain in none of the fractions (Supplementary Figures 11–14).
Specific Genes of the Fimsbactin Cluster Could Contribute to the Acinetobactin Biosynthesis
Our results showed that the genes basG and basC, involved in the biosynthesis of the N-hydroxyhistamine precursor, and the genes basD and basB, involved in the assembly of the precursors, are essential for the biosynthesis of acinetobactin and therefore, they are crucial for the virulence of A. baumannii in vivo. However, the deletion of basJ gene which is involved in the biosynthesis of the DHBA precursor did not have any effect in the biosynthesis of acinetobactin and in the virulence of the bacterium. Taking into account that DHBA is also a fimsbactin precursor, our data suggest that in the absence of specific genes of the acinetobactin cluster, A. baumannii ATCC 17978 is able to successfully synthetize acinetobactin using redundant genes from the fimsbactin cluster.
To explore this hypothesis, we performed an in-depth analysis of both clusters using BLAST (see Supplementary Figure 1 for cluster organization and Supplementary Table 2 for gene description). This analysis showed that all the genes (except for entA) belonging to the acinetobactin cluster involved in the DHBA biosynthesis and the NRPS assembly have a potential redundant gene in the fimsbactin cluster (Supplementary Table 3). To further investigate this genetic redundancy, basJ and basF genes were selected and two double mutant strains were generated lacking both redundant genes of the acinetobactin and the fimsbactin clusters (ΔbasJ/ΔfbsB and ΔbasF/ΔfbsC) (Table 1).
Growth curves under iron-limiting conditions showed a significant decrease in the growth abilities of both ΔbasJ/ΔfbsB (μmax = 0.24, P = 0.001 and λ = 8.94, P = 0.0009) and ΔbasF/ΔfbsC (μmax = 0.38, P = 0.006 and λ = 9.65, P < 0.0001) mutant strains compared to the parental strain ATCC 17978 (μmax = 0.74 and λ = 1.36) (Figure 6A and Table 2). However, no significant differences were detected between the isogenic double mutant strains and the wild-type strain under normal growth conditions (Supplementary Figure 2C).
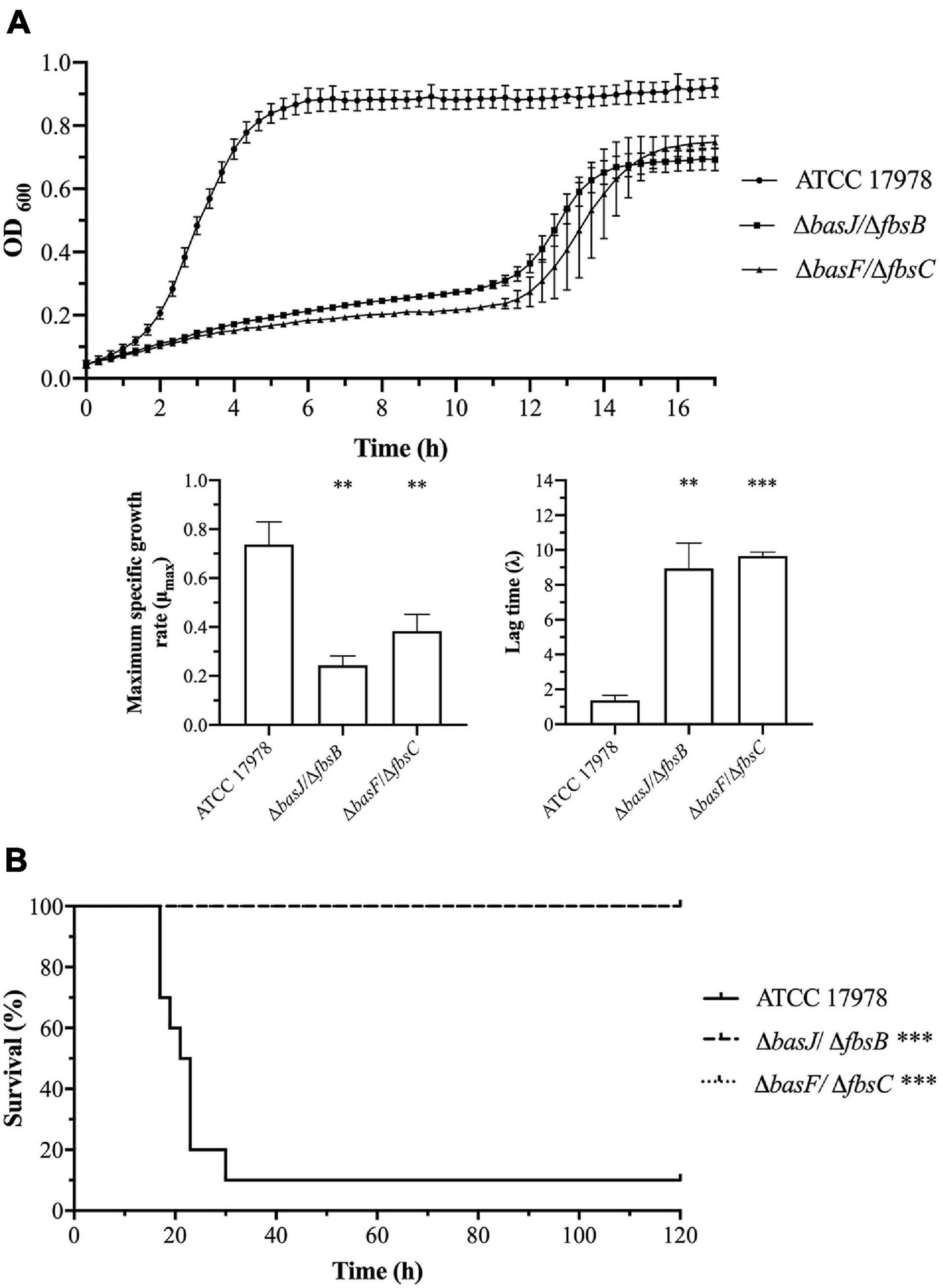
Figure 6. (A) Growth curves and growth kinetics of A. baumannii ATCC 17978 and the ΔbasJ/ΔfbsB and ΔbasF/ΔfbsC double mutant strains. The growth curves were performed under iron-limiting conditions. (B) Sepsis infection in mice. Survival of BALB/c mice (n = 10 per group) after bacteremia infection with A. baumannii ATCC 17978 and the ΔbasJ/ΔfbsB and ΔbasF/ΔfbsC double mutant strains. The log-rank (Mantel-Cox) test was used for statistical analysis (∗∗P < 0.01, ∗∗∗P < 0.0001).
Furthermore, a murine sepsis model was performed with the ATCC 17978 parental strain and the two double isogenic mutant strains. Mice infected with ΔbasJ/ΔfbsB and ΔbasF/ΔfbsC strains displayed a significantly increase of the survival rate (100% survival, P < 0.0001) in relation to those infected with the ATCC 17978 parental strain (10% survival) (Figure 6B and Table 2).
Finally, the siderophore-content of the double mutant ΔbasJ/ΔfbsB was studied using our SPE-HLB/HPLC/MS methodology, revealing complete inhibition of acinetobactin and fimsbactins production by lack of detection of these siderophores in the HPLC/MS analysis (Table 2 and Supplementary Figures 13, 14).
Discussion
In the last decades, the emergence of A. baumannii multidrug-resistant strains has become a worldwide concerning problem derived from the scarcity of effective therapeutic options against this bacterium. Hence, the World Health Organization (WHO) included A. baumannii as a critical priority pathogen claiming an urgent need of efficient alternatives to the known antibiotics (World Health Organization [WHO], 2017). Within this context, the search and identification of new therapeutic targets in A. baumannii have become a priority.
The pathogenesis success of A. baumannii is partially linked to the synthesis of active siderophores that supply the iron needed for its essential role in crucial metabolic events. Among all the siderophore systems identified, acinetobactin is considered the major siderophore of this bacterium (Yamamoto et al., 1994). Since then, several studies have focused on unraveling its regulation, chelating mechanisms and its role in the virulence of the bacterium (Mihara et al., 2004; Gaddy et al., 2012; Shapiro and Wencewicz, 2016; Sheldon and Skaar, 2020). After confirming that acinetobactin-related metabolism is a crucial virulence factor and that it is highly conserved among A. baumannii strains, the iron(III) uptake system mediated by acinetobactin has been proposed as a potential therapeutic target to combat this multidrug-resistant pathogen (Antunes et al., 2011; Gaddy et al., 2012; Sheldon and Skaar, 2020). However, the contribution of each individual gene involved in the acinetobactin metabolism in the infectious process is still unclear. Thus, we have performed an in-depth analysis of the acinetobactin gene cluster by conducting different phenotypical assays with the well-known strain A. baumannii ATCC 17978 and 19 isogenic mutant derivative strains lacking genes involved in the biosynthesis and transport of acinetobactin.
Three different siderophore-mediated iron uptake systems (acinetobactin, baumanoferrin and fimsbactin) were identified from the reference strain A. baumannii ATCC 17978, which corresponding gene clusters were found upregulated under in vitro iron-limiting conditions and in vivo infection (Eijkelkamp et al., 2011; Murray et al., 2017; Martínez-Guitián et al., 2020). Acinetobactin and fimsbactins are synthesized through non-ribosomal peptide synthetase (NRPS) assembly systems, sharing the DHBA and L-threonine precursors (Proschak et al., 2013; Song and Kim, 2020). This could explain the high level of genetic redundancy between both clusters where most of the genes involved in the biosynthesis of the acinetobactin have a potential redundant gene in the fimsbactin cluster. Hence, although fimsbactins are only present in a small percentage of A. baumannii strains, fimsbactin genes could complement the inactivation of some acinetobactin genes, suggesting a redundancy in both pathways. Within this context, A. baumannii ATCC 17978 confers the perfect background for the present study.
Bioinformatic analysis of the acinetobactin gene cluster showed that basG, basC and entA genes do not have a potential redundant gene. The lack of redundant basG and basC genes in the fimsbactin cluster is easily explained since these genes are involved in the biosynthesis of the precursor of acinetobactin N-hydroxyhistamine (Shapiro and Wencewicz, 2016) and this moiety is not present in the fimsbactins. On the other hand, the entA gene is always located outside of the acinetobactin cluster, varying its location between strains. In A. baumannii ATCC 17978, acinetobactin and fimsbactins share the gene entA, which is located in the fimsbactin cluster (Penwell et al., 2012). The individual deletion of these three genes resulted in a drastic reduction of the virulence when compared to the wild-type strain. Our results agreed with a recent study published by Sheldon and Skaar where they demonstrated that the deletion of gene basG impairs growth on human serum, transferrin or lactoferrin as sole iron sources, and severely attenuates survival of A. baumannii ATCC 17978 in a murine bacteremia model (Sheldon and Skaar, 2020).
Although Dorsey et al. predicted that basC gene had an essential function in the biosynthesis of the acinetobactin on the basis of its involvement in the synthesis of N-hydroxyhistamine (Dorsey et al., 2004), this hypothesis was never investigated until now. Siderophore-content analysis of the cell free supernatants of both ΔbasG and ΔbasC using our SPE-HLB/HPLC-MS methodology showed that the deletion of these genes resulted in the complete inhibition of acinetobactin production. The low virulent phenotype of these two mutants could be related to the lack of the siderophore. As the previous case, it would be expected that the deletion of the entA gene will inhibit the biosynthesis of both acinetobactin and fimsbactin siderophores.
Deletion of basB and basD also led to a significant decrease in virulence characterized by an impaired fitness under iron-limiting conditions and increased mice survival. We have previously reported that basB is an essential gene for the virulence of A. baumannii during pneumonia in mice and for bacteria growth under iron-limiting conditions (Martínez-Guitián et al., 2020). In addition, Gaddy et al. demonstrated that basD gene is essential for the biosynthesis of acinetobactin and for the bacterial growth under iron-depleted conditions in A. baumannii ATCC 19606 (Gaddy et al., 2012). Both genes code for proteins involved in the last steps of the biosynthetic pathway of acinetobactin, where DHBA and L-Threonine precursors are linked (BasD) and the resulting intermediate is bonded to N-hydroxyhistamine (BasB) to give preacinetobactin (Hasan et al., 2015; Shapiro and Wencewicz, 2016; Song and Kim, 2020). Both ΔbasB and ΔbasD mutants were unable to synthetize acinetobactin.
A closer analysis of the basJ gene, showed that its deletion did not have any effect in the biosynthesis of acinetobactin. However, the deletion of both acinetobactin (basJ) and fimsbactin (fbsB) redundant genes resulted in the loss of acinetobactin and fimsbactins production. This fact demonstrates that in absence of the basJ gene, A. baumannii ATCC 17978 can use fbsB to synthesize acinetobactin. This is a clear example of molecular redundancy whereby two genes have the same function or when an alternative pathway fulfills the mission role of an inactivated gene. Pathogens used it to adapt to a continuous changing environment, avoiding the antimicrobial defenses of their hosts (Ghosh and O’Connor, 2017). Based on our results, we predict that the redundancy of ΔbasF,ΔbasI,ΔbasH,ΔbasA, and ΔbasE strains possibly lead to the unchanged ability to synthesize acinetobactin.
Among the genes involved in the transport of acinetobactin, only the gen bauA, coding for the outer-membrane receptor, was found to be essential for the virulence during a murine sepsis model. Previous studies have shown that the gene bauA is essential for the virulence of A. baumannii ATCC 19606 since its deletion led to a decrease in the ability of the bacteria to infect, divide inside body fluids of mice and in fitness under iron-limiting conditions (Gaddy et al., 2012). In fact, BauA was proposed as a good vaccine candidate since mice injected with recombinant BauA were able to produce antibodies against this protein. In addition, passive immunization using serum anti-BauA protected mice from infection (Esmaeilkhani et al., 2016). Our data slightly differ with this study since we have not observed any reduction in the fitness of A. baumannii ATCC 17978 when bauA was deleted. This discrepancy could be explained by the higher susceptibility of the strain ATCC 19606 to chelate iron (III) compared to the ATCC 17978 strain, possibly due to the lack of fimsbactins and baumanoferrin production in the ATCC 19606 strain (Antunes et al., 2011; Proschak et al., 2013; Penwell et al., 2015; Ramirez et al., 2019).
Both BarA and BarB proteins belong to the acinetobactin secretion system (efflux) of the ABC superfamily. Mutant strains lacking the genes involved in the synthesis of these proteins, ΔbarA and ΔbarB, showed a significant decrease in fitness under iron-limiting conditions. Notwithstanding, no statistical differences between the percentage of survival of the mice infected with these mutant strains and the mice infected with the parental strain were observed. SPE-HLB/HPLC-MS analysis of ΔbarB mutant strain cultures showed the presence of acinetobactin in its cell-free supernatant, which indicates that this single mutant strain did not prevent the efflux of acinetobactin outside the bacteria. A previous study carried out by Penwell et al. in A. baumannii ATCC 19606, showed a growth defect under iron-limiting conditions and a 60% decrease of acinetobactin effluxed in the ΔbarA/ΔbarB cell-free supernatant compared with the parental strain (Penwel, 2013). The reduction in the efflux of acinetobactin matches with the partial loss of virulence in the ΔbarA and ΔbarB mutant strains. It is known that A. baumannii possesses a wide variety of transport mechanisms. It is possible that under stress conditions, the bacteria could use non-specific transporter systems to secrete and uptake acinetobactin and do not lose the iron-battle against the host (Iacono et al., 2008; Coyne et al., 2010, 2011; Fernando and Kumar, 2012).
Several researchers have focused their efforts on the development of new inhibitors based on acinetobactin metabolism. Inhibitors of BasE, an enzyme involved in biosynthesis of the acinetobactin, have shown a powerful inhibitory activity (Neres et al., 2013). Analogous of acinetobactin have also shown bacteriostatic activity as they were able to block the transport of the iron-acinetobactin complex inside the bacteria (Bohac et al., 2017; Shapiro and Wencewicz, 2017). In the last years, siderophore conjugates using the “Trojan Horse” antibiotic drug delivery strategy has become more popular for combating this microorganism. In fact, cefiderocol (fetcroja) was the first cathecol-substituted siderophore cephalosporin approved by the FDA and EMA (Ji et al., 2012; Wencewicz and Miller, 2013; Ghosh et al., 2017; Parsels et al., 2021).
In summary, we performed an in-depth analysis of the role of each individual gene of the acinetobactin metabolism in the virulence of A. baumannii ATCC 17978, allowing us to identify six potential targets for the design of new antimicrobials against this microorganism: five of them involved in its biosynthesis (entA, basG, basC, basD, and basB) and one related to its transport (bauA). Due to the similar function and potentially similar structure of the enzymes involved in the biosynthesis of acinetobactin and fimsbactin, inhibitors against the remaining biosynthetic steps could also have the potential to be effective by inactivating both redundant proteins.
Data Availability Statement
The original contributions presented in the study are included in the article/Supplementary Material, further inquiries can be directed to the corresponding author.
Ethics Statement
The animal study was reviewed and approved by Hospital Universitario A Coruña, Spain, project code P102.
Author Contributions
KC-P, SR-F, NT-T, and LÁ-F performed mutant construction. MM-G, JV-U and KC-P performed phenotypic experiments and animal models. LA performed the analysis of the siderophore-content. AB, MP, CJ, and LÁ-F designed and supervised the experiments and wrote the manuscript. GB and JR revised the manuscript. All authors read and approved the final manuscript.
Funding
This work was funded by Projects PI15/00860 awarded to GB and PI17/01482 to AB and MP, all within in the National Plan for Scientific Research, Development and Technological Innovation 2013–2016 and funded by the ISCIII – General Subdirection of Assessment and Promotion of the Research-European Regional Development Fund (FEDER) “A way of making Europe.” The study was also funded by project IN607A 2016/22 (GAIN- Agencia Gallega de Innovación – Consellería de Economía, Emprego e Industria) awarded to GB. This work was also supported by Planes Nacionales de I + D + i 2008–2011/2013–2016 and Instituto de Salud Carlos III, Subdirección General de Redes y Centros de Investigación Cooperativa, Ministerio de Economía y Competitividad, Spanish Network for Research in Infectious Diseases (REIPI RD16/0016/006) co-financed by European Development Regional Fund “A way to achieve Europe” and operative program Intelligent Growth 2014–2020. This work was also supported by Grant RTI2018-093634-B-C22 (AEI/FEDER, EU) from the State Agency for Research (AEI) of Spain, co-funded by the FEDER Programme from the European Union and Xunta de Galicia for the support of Grant ED431E 2018/03 for CICA-INIBIC strategic and the initiative “Seed Projects 2019–2020.” JV-U was financially supported by the ISCIII project FI18/00315, LÁ-F by the ISCIII project PI14/00059 and the IN606B-2018/011, MM-G was financially supported by the Grant Clara Roy (SEIMC, Spanish Society of Clinical Microbiology and Infectious Diseases), KC-P by IN607A 2016/22 and AECC (Asociación Española Contra el Cáncer) predoctoral fellowship and LA by Xunta de Galicia co-funded with the European Social Fund (FSE) of the European Union (ED481A-2019/081).
Conflict of Interest
The authors declare that the research was conducted in the absence of any commercial or financial relationships that could be construed as a potential conflict of interest.
Publisher’s Note
All claims expressed in this article are solely those of the authors and do not necessarily represent those of their affiliated organizations, or those of the publisher, the editors and the reviewers. Any product that may be evaluated in this article, or claim that may be made by its manufacturer, is not guaranteed or endorsed by the publisher.
Acknowledgments
We thank M. I. Voskuil (Dept. of Immunology and Microbiology, University of Colorado Medical School, CO, United States) for providing pMo130.
Supplementary Material
The Supplementary Material for this article can be found online at: https://www.frontiersin.org/articles/10.3389/fmicb.2021.752070/full#supplementary-material
References
Álvarez-Fraga, L., Pérez, A., Rumbo-Feal, S., Merino, M., Vallejo, J. A., Ohneck, E. J., et al. (2016). Analysis of the role of the LH92_11085 gene of a biofilm hyper-producing Acinetobacter baumannii strain on biofilm formation and attachment to eukaryotic cells. Virulence 7, 443–455. doi: 10.1080/21505594.2016.1145335
Álvarez-Fraga, L., Vázquez-Ucha, J. C., Martínez-Guitián, M., Vallejo, J. A., Bou, G., Beceiro, A., et al. (2018). Pneumonia infection in mice reveals the involvement of the feoA gene in the pathogenesis of Acinetobacter baumannii. Virulence 9, 496–509. doi: 10.1080/21505594.2017.1420451
Antunes, L. C., Imperi, F., Towner, K. J., and Visca, P. (2011). Genome-assisted identification of putative iron-utilization genes in Acinetobacter baumannii and their distribution among a genotypically diverse collection of clinical isolates. Res. Microbiol. 162, 279–284. doi: 10.1016/j.resmic.2010.10.010
Balado, M., Souto, A., Vences, A., Careaga, V. P., Valderrama, K., Segade, Y., et al. (2015). Two Catechol Siderophores, Acinetobactin and Amonabactin, Are Simultaneously Produced by Aeromonas salmonicida subsp. salmonicida Sharing Part of the Biosynthetic Pathway. ACS Chem. Biol. 10, 2850–2860. doi: 10.1021/acschembio.5b00624
Bohac, T. J., Shapiro, J. A., and Wencewicz, T. A. (2017). Rigid oxazole acinetobactin analog blocks siderophore cycling in Acinetobacter baumannii. ACS Infect. Dis. 3, 802–806. doi: 10.1021/acsinfecdis.7b00146
Coyne, S., Courvalin, P., and Périchon, B. (2011). Efflux-mediated antibiotic resistance in Acinetobacter spp. Antimicrob. Agents Chemother. 55, 947–953. doi: 10.1128/AAC.01388-10
Coyne, S., Rosenfeld, N., Lambert, T., Courvalin, P., and Périchon, B. (2010). Overexpression of resistance-nodulation-cell division pump AdeFGH confers multidrug resistance in Acinetobacter baumannii. Antimicrob. Agents Chemother. 54, 4389–4393. doi: 10.1128/AAC.00155-10
De Oliveira, D. M. P., Forde, B. M., Kidd, T. J., Harris, P. N. A., Schembri, M. A., Beatson, S. A., et al. (2020). Antimicrobial resistance in ESKAPE pathogens. Clin. Microbiol. Rev. 33:e00181-19. doi: 10.1128/CMR.00181-19
Dickey, S. W., Cheung, G. Y. C., and Otto, M. (2017). Different drugs for bad bugs: antivirulence strategies in the age of antibiotic resistance. Nat. Rev. Drug Discov. 16, 457–471. doi: 10.1038/nrd.2017.23
Dorsey, C. W., Tomaras, A. P., Connerly, P. L., Tolmasky, M. E., Crosa, J. H., and Actis, L. A. (2004). The siderophore-mediated iron acquisition systems of Acinetobacter baumannii ATCC 19606 and Vibrio anguillarum 775 are structurally and functionally related. Microbiology 150, 3657–3667. doi: 10.1099/mic.0.27371-0
Eijkelkamp, B. A., Hassan, K. A., Paulsen, I. T., and Brown, M. H. (2011). Investigation of the human pathogen Acinetobacter baumannii under iron limiting conditions. BMC Genomics 12:126. doi: 10.1186/1471-2164-12-126
Esmaeilkhani, H., Rasooli, I., Nazarian, S., and Sefid, F. (2016). In vivo validation of the immunogenicity of recombinant Baumannii Acinetobactin Utilization A protein (rBauA). Microb. Pathog. 98, 77–81. doi: 10.1016/j.micpath.2016.06.032
Espada, A., Anta, C., Bragado, A., Rodríguez, J., and Jiménez, C. (2011). An approach to speed up the isolation of hydrophilic metabolites from natural sources at semipreparative level by using a hydrophilic-lipophilic balance/mixed-mode strong cation exchange-high-performance liquid chromatography/mass spectrometry system. J. Chromatogr. A 1218, 1790–1794. doi: 10.1016/j.chroma.2011.01.072
Fernando, D., and Kumar, A. (2012). Growth phase-dependent expression of RND efflux pump- and outer membrane porin-encoding genes in Acinetobacter baumannii ATCC 19606. J. Antimicrob. Chemother. 67, 569–572. doi: 10.1093/jac/dkr519
Gaddy, J. A., Arivett, B. A., Mcconnell, M. J., López-Rojas, R., Pachón, J., and Actis, L. A. (2012). Role of acinetobactin-mediated iron acquisition functions in the interaction of Acinetobacter baumannii strain ATCC 19606T with human lung epithelial cells, Galleria mellonella caterpillars, and mice. Infect. Immun. 80, 1015–1024. doi: 10.1128/IAI.06279-11
Ghosh, M., Miller, P. A., Möllmann, U., Claypool, W. D., Schroeder, V. A., Wolter, W. R., et al. (2017). Targeted antibiotic delivery: selective siderophore conjugation with daptomycin confers potent activity against multidrug resistant Acinetobacter baumannii both in vitro and in vivo. J. Med. Chem. 60, 4577–4583. doi: 10.1021/acs.jmedchem.7b00102
Ghosh, S., and O’Connor, T. J. (2017). Beyond Paralogs: the multiple layers of redundancy in bacterial pathogenesis. Front. Cell Infect. Microbiol. 7:467. doi: 10.3389/fcimb.2017.00467
Hamad, M. A., Zajdowicz, S. L., Holmes, R. K., and Voskuil, M. I. (2009). An allelic exchange system for compliant genetic manipulation of the select agents Burkholderia pseudomallei and Burkholderia mallei. Gene 430, 123–131. doi: 10.1016/j.gene.2008.10.011
Harding, C. M., Hennon, S. W., and Feldman, M. F. (2018). Uncovering the mechanisms of Acinetobacter baumannii virulence. Nat. Rev. Microbiol. 16, 91–102. doi: 10.1038/nrmicro.2017.148
Hasan, T., Choi, C. H., and Oh, M. H. (2015). Genes involved in the biosynthesis and transport of Acinetobactin in Acinetobacter baumannii. Genomics Inform. 13, 2–6. doi: 10.5808/GI.2015.13.1.2
Iacono, M., Villa, L., Fortini, D., Bordoni, R., Imperi, F., Bonnal, R. J., et al. (2008). Whole-genome pyrosequencing of an epidemic multidrug-resistant Acinetobacter baumannii strain belonging to the European clone II group. Antimicrob. Agents Chemother. 52, 2616–2625. doi: 10.1128/AAC.01643-07
Ji, C., Juárez-Hernández, R. E., and Miller, M. J. (2012). Exploiting bacterial iron acquisition: siderophore conjugates. Future Med. Chem. 4, 297–313. doi: 10.4155/fmc.11.191
Martínez-Guitián, M., Vázquez-Ucha, J. C., Álvarez-Fraga, L., Conde-Pérez, K., Lasarte-Monterrubio, C., Vallejo, J. A., et al. (2019). Involvement of HisF in the Persistence of Acinetobacter baumannii during a pneumonia infection. Front. Cell. Infect. Microbiol. 9:310. doi: 10.3389/fcimb.2019.00310
Martínez-Guitián, M., Vázquez-Ucha, J. C., Álvarez-Fraga, L., Conde-Pérez, K., Vallejo, J. A., Perina, A., et al. (2020). Global transcriptomic analysis during murine pneumonia infection unravels new virulence factors in Acinetobacter baumannii. J. Infect. Dis. 223, 1356–1366. doi: 10.1093/infdis/jiaa522
Mihara, K., Tanabe, T., Yamakawa, Y., Funahashi, T., Nakao, H., Narimatsu, S., et al. (2004). Identification and transcriptional organization of a gene cluster involved in biosynthesis and transport of acinetobactin, a siderophore produced by Acinetobacter baumannii ATCC 19606T. Microbiology 150, 2587–2597. doi: 10.1099/mic.0.27141-0
Moynié, L., Serra, I., Scorciapino, M. A., Oueis, E., Page, M. G., Ceccarelli, M., et al. (2018). Preacinetobactin not acinetobactin is essential for iron uptake by the BauA transporter of the pathogen Acinetobacter baumannii. eLife 7:e42270. doi: 10.7554/eLife.42270.027
Murray, G. L., Tsyganov, K., Kostoulias, X. P., Bulach, D. M., Powell, D., Creek, D. J., et al. (2017). Global gene expression profile of Acinetobacter baumannii during bacteremia. J. Infect. Dis. 215, S52–S57. doi: 10.1093/infdis/jiw529
Neres, J., Engelhart, C. A., Drake, E. J., Wilson, D. J., Fu, P., Boshoff, H. I., et al. (2013). Non-nucleoside inhibitors of BasE, an adenylating enzyme in the siderophore biosynthetic pathway of the opportunistic pathogen Acinetobacter baumannii. J. Med. Chem. 56, 2385–2405. doi: 10.1021/jm301709s
Parsels, K. A., Mastro, K. A., Steele, J. M., Thomas, S. J., and Kufel, W. D. (2021). Cefiderocol: a novel siderophore cephalosporin for multidrug-resistant Gram-negative bacterial infections. J. Antimicrob. Chemother. 76, 1379–1391. doi: 10.1093/jac/dkab015
Penwel, W. F. (2013). Iron Acquisition in Acinetobacter baumannii. Ph.D. thesis. Oxford: Miami University.
Penwell, W. F., Arivett, B. A., and Actis, L. A. (2012). The Acinetobacter baumannii entA gene located outside the acinetobactin cluster is critical for siderophore production, iron acquisition and virulence. PLoS One 7:e36493. doi: 10.1371/journal.pone.0036493
Penwell, W. F., Degrace, N., Tentarelli, S., Gauthier, L., Gilbert, C. M., Arivett, B. A., et al. (2015). Discovery and Characterization of New Hydroxamate Siderophores, Baumannoferrin A and B, produced by Acinetobacter baumannii. Chembiochem 16, 1896–1904. doi: 10.1002/cbic.201500147
Proschak, A., Lubuta, P., Grün, P., Löhr, F., Wilharm, G., De Berardinis, V., et al. (2013). Structure and biosynthesis of fimsbactins A-F, siderophores from Acinetobacter baumannii and Acinetobacter baylyi. Chembiochem 14, 633–638. doi: 10.1002/cbic.201200764
Ramirez, M. S., Penwell, W. F., Traglia, G. M., Zimbler, D. L., Gaddy, J. A., Nikolaidis, N., et al. (2019). Identification of potential virulence factors in the model strain Acinetobacter baumannii A118. Front. Microbiol. 10:1599. doi: 10.3389/fmicb.2019.01599
Shapiro, J. A., and Wencewicz, T. A. (2016). Acinetobactin isomerization enables adaptive iron acquisition in Acinetobacter baumannii through pH-triggered siderophore swapping. ACS Infect. Dis. 2, 157–168. doi: 10.1021/acsinfecdis.5b00145
Shapiro, J. A., and Wencewicz, T. A. (2017). Structure-function studies of acinetobactin analogs. Metallomics 9, 463–470. doi: 10.1039/C7MT00064B
Sheldon, J. R., Laakso, H. A., and Heinrichs, D. E. (2016). Iron acquisition strategies of bacterial pathogens. Microbiol. Spectr. 4:2. doi: 10.1128/9781555819286.ch3
Sheldon, J. R., and Skaar, E. P. (2020). Acinetobacter baumannii can use multiple siderophores for iron acquisition, but only acinetobactin is required for virulence. PLoS Pathog. 16:e1008995. doi: 10.1371/journal.ppat.1008995
Song, W. Y., and Kim, H. J. (2020). Current biochemical understanding regarding the metabolism of acinetobactin, the major siderophore of the human pathogen Acinetobacter baumannii, and outlook for discovery of novel anti-infectious agents based thereon. Nat. Prod. Rep. 37, 477–487. doi: 10.1039/C9NP00046A
Tjørve, K. M. C., and Tjørve, E. (2017). The use of Gompertz models in growth analyses, and new Gompertz-model approach: an addition to the Unified-Richards family. PLoS One 12:e0178691. doi: 10.1371/journal.pone.0178691
Wencewicz, T. A., and Miller, M. J. (2013). Biscatecholate-monohydroxamate mixed ligand siderophore-carbacephalosporin conjugates are selective sideromycin antibiotics that target Acinetobacter baumannii. J. Med. Chem. 56, 4044–4052. doi: 10.1021/jm400265k
Wong, D., Nielsen, T. B., Bonomo, R. A., Pantapalangkoor, P., Luna, B., and Spellberg, B. (2017). Clinical and pathophysiological overview of acinetobacter infections: a century of challenges. Clin. Microbiol. Rev. 30, 409–447. doi: 10.1128/CMR.00058-16
World Health Organization [WHO] (2017). Global Priority List of Antibiotic-Resistant Bacteria to Guide Research, Discovery, and Development of New Antibiotics. Available online at: http://www.who.int/medicines/publications/WHO-PPL-Short_Summary_25Feb-ET_NM_WHO.pdf?ua=1 (accessed June 25, 2021).
Keywords: Acinetobacter baumannii, acinetobactin, fimsbactin, iron uptake, siderophore, virulence, mouse sepsis infection
Citation: Conde-Pérez K, Vázquez-Ucha JC, Álvarez-Fraga L, Ageitos L, Rumbo-Feal S, Martínez-Guitián M, Trigo-Tasende N, Rodríguez J, Bou G, Jiménez C, Beceiro A and Poza M (2021) In-Depth Analysis of the Role of the Acinetobactin Cluster in the Virulence of Acinetobacter baumannii. Front. Microbiol. 12:752070. doi: 10.3389/fmicb.2021.752070
Received: 02 August 2021; Accepted: 16 September 2021;
Published: 05 October 2021.
Edited by:
Younes Smani, Institute of Biomedicine of Seville (IBIS), SpainReviewed by:
Oleg Reva, University of Pretoria, South AfricaKarl Hassan, The University of Newcastle, Australia
Copyright © 2021 Conde-Pérez, Vázquez-Ucha, Álvarez-Fraga, Ageitos, Rumbo-Feal, Martínez-Guitián, Trigo-Tasende, Rodríguez, Bou, Jiménez, Beceiro and Poza. This is an open-access article distributed under the terms of the Creative Commons Attribution License (CC BY). The use, distribution or reproduction in other forums is permitted, provided the original author(s) and the copyright owner(s) are credited and that the original publication in this journal is cited, in accordance with accepted academic practice. No use, distribution or reproduction is permitted which does not comply with these terms.
*Correspondence: Laura Álvarez-Fraga, bGF1cmEuYWx2YXJlei5mcmFnYUBzZXJnYXMuZXM=
†ORCID: Kelly Conde-Pérez, orcid.org/0000-0003-1238-2221; Juan C. Vázquez-Ucha, orcid.org/0000-0003-4949-0779; Laura Álvarez-Fraga, orcid.org/0000-0003-3920-5866; Lucía Ageitos, orcid.org/0000-0002-2422-3773; Soraya Rumbo-Feal, orcid.org/0000-0002-1796-1815; Marta Martínez-Guitián, orcid.org/0000-0002-3457-0613; Noelia Trigo-Tasende, orcid.org/0000-0001-5093-8994; Jaime Rodríguez, orcid.org/0000-0001-5348-6970; Germán Bou, orcid.org/0000-0001-8837-0062; Carlos Jiménez, orcid.org/0000-0003-2628-303X; Alejandro Beceiro, orcid.org/0000-0002-6340-7815; Margarita Poza, orcid.org/0000-0001-9423-7268
‡These authors have contributed equally to this work and share first authorship
§These authors have contributed equally to this work and share last authorship