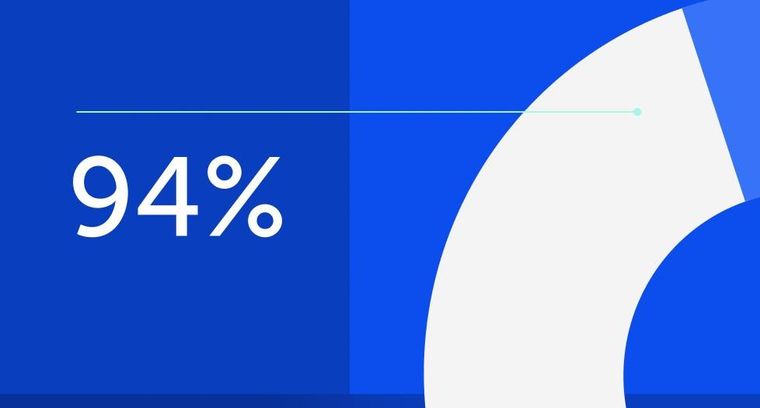
94% of researchers rate our articles as excellent or good
Learn more about the work of our research integrity team to safeguard the quality of each article we publish.
Find out more
ORIGINAL RESEARCH article
Front. Microbiol., 29 November 2021
Sec. Virology
Volume 12 - 2021 | https://doi.org/10.3389/fmicb.2021.746110
Dengue is a serious public health concern worldwide, with ∼3 billion people at risk of contracting dengue virus (DENV) infections, with some suffering severe consequences of disease and leading to death. Currently, there is no broad use vaccine or drug available for the prevention or treatment of dengue, which leaves only anti-mosquito strategies to combat the dengue menace. The present study is an extension of our earlier study aimed at determining the in vitro and in vivo protective effects of a plant-derived phytopharmaceutical drug for the treatment of dengue. In our previous report, we had identified a methanolic extract of aerial parts of Cissampelos pareira to exhibit in vitro and in vivo anti-dengue activity against all the four DENV serotypes. The dried aerial parts of C. pareira supplied by local vendors were often found to be mixed with aerial parts of another plant of the same Menispermaceae family, Cocculus hirsutus, which shares common homology with C. pareira. In the current study, we have found C. hirsutus to have more potent anti-dengue activity as compared with C. pareira. The stem part of C. hirsutus was found to be more potent (∼25 times) than the aerial part (stem and leaf) irrespective of the extraction solvent used, viz., denatured spirit, hydro-alcohol (50:50), and aqueous. Moreover, the anti-dengue activity of stem extract in all the solvents was comparable. Hence, an aqueous extract of the stem of C. hirsutus (AQCH) was selected due to greater regulatory compliance. Five chemical markers, viz., Sinococuline, 20-Hydroxyecdysone, Makisterone-A, Magnoflorine, and Coniferyl alcohol, were identified in fingerprinting analysis. In a test of primary dengue infection in the AG129 mice model, AQCH extract at 25 mg/kg body weight exhibited protection when administered four and three times a day. The AQCH was also protective in the secondary DENV-infected AG129 mice model at 25 mg/kg/dose when administered four and three times a day. Additionally, the AQCH extract reduced serum viremia and small intestinal pathologies, viz., viral load, pro-inflammatory cytokines, and vascular leakage. Based on these findings, we have undertaken the potential preclinical development of C. hirsutus-based phytopharmaceutical, which could be studied further for its clinical development for treating dengue.
Dengue is a mosquito-borne disease caused by infection of one or more of the four antigenically distinct dengue virus (DENV) serotypes. The DENV is a positive single-stranded RNA virus, which belongs to the genus Flavivirus of the Flaviviridae family. Dengue infection has the potential of causing a pandemic and outbreaks in many parts of the world1. Climate change, population growth, increased international travel, rapid urbanization, and ineffectual vector control strategies have led to an expansion of the dengue disease footprint worldwide. At this time, dengue is endemic in more than 100 countries in South-East Asia, Eastern Mediterranean, Western Pacific, Americas, and Africa (Gubler, 2011; Struchiner et al., 2015; Wilder-Smith et al., 2019).
A recent study has revealed that around 390 million dengue infection cases take place per year, out of which 96 million cases show mild to severe clinical manifestations of disease (Bhatt et al., 2013). Another study revealed that around 3.9 billion people are at risk of contracting dengue disease, making it a serious global health concern (Brady et al., 2012). Asia alone is saddled with 75% of the global dengue burden (Bhatt et al., 2013), and India is hyperendemic for dengue, with a whopping 34% contribution to the global burden (Chakravarti et al., 2012; Bhatt et al., 2013; Wilder-Smith and Rupali, 2019; Wilder-Smith et al., 2019). It has been estimated that 49% of India’s population has already been infected with the DENV, although the prevalence varies among different regions (Murhekar et al., 2019). According to a Global Burden of Disease study, dengue alone inflicts a total of ∼58 million symptomatic dengue virus infections worldwide, which estimates USD 8–9 billion annual economic burden for dengue illness globally as per 2013 prices (Shepard et al., 2016; Stanaway et al., 2016).
Dengue is transmitted through the bite of infected female mosquitoes, primarily Aedes aegypti. The disease manifests in febrile illness, and symptoms persist for 2–7 days post 4–10 days of incubation after the mosquito bite (WHO, 2009; Wilder-Smith et al., 2019). Symptomatic dengue infection can range from mild dengue fever (DF) to severe life-threatening dengue hemorrhagic fever (DHF) and dengue shock syndrome (DSS). DF is considered to be a mild disease associated with fever along with other symptoms such as frontal headache, retro-orbital pain, muscle and joint pain, nausea, vomiting, swollen glands, or rash. Severe dengue is more threatening and involves plasma leakage, respiratory distress, fluid accumulation, severe bleeding, and organ deterioration (WHO, 2009; Muller et al., 2017; Wilder-Smith et al., 2019).
There is no proven treatment available for dengue, and patients are provided only supportive medical care, especially fluid management (WHO, 2009). DENV has four antigenically distinct serotypes, all of which co-circulate in more than 140 countries of the world (Shepard et al., 2016). The recent launch of a dengue vaccine, Dengvaxia, by Sanofi Pasteur is of limited use due to its possible disease enhancement potential in seronegative Vaccinees (Aguiar et al., 2016; Thomas and Yoon, 2019). The other two promising vaccine candidates, developed by the National Institutes of Health/Butantan/Merck and Takeda, are in the advanced phase of clinical trials (Perkel, 2016; Biswal et al., 2020; Deng et al., 2020).
Several chemical entities have been tested for anti-DENV activities, but none have shown promise to date (Beesetti et al., 2016; Dighe et al., 2019; Lim, 2019). Several repurposed drugs, such as chloroquine (Tricou et al., 2010), celgosivir (Low et al., 2014), lovastatin (Whitehorn et al., 2016), balapiravir (Nguyen et al., 2013), and prednisolone (Tam et al., 2012), have also been evaluated for their efficacy in the clinical trials. All these trials failed to meet the efficacy endpoints. No conventional drug discovery effort has yet produced any effective and safe drug for the treatment of dengue (Dighe et al., 2019). Plant-based drug discovery has also been a focus of drug development against dengue. Azadirachta indica (Parida et al., 2002), Hippophae rhamnoide (Jain et al., 2008), Carica papaya (Kasture et al., 2016), Cissampelos pareira (Sood et al., 2015), etc. are some of the plants that are traditionally used in different regions of India by tribals, traditional healers, and local people for treating symptomatically dengue-like infections (Singh and Rawat, 2017). These herbal formulations, if validated and proved for their efficacy scientifically, can provide a safe and effective treatment for dengue.
In our previous study, we identified Cissampelos pareira plant for its anti-dengue activity against all four DENV serotypes (Sood et al., 2015). The dried aerial parts of C. pareira obtained from a local vendor are frequently found to be mixed with an identical plant Cocculus hirsutus, as both are twining woody climbing plants belonging to the same order Ranunculales and family, i.e., Menispermaceae. Thus, evaluation of the anti-dengue activity of C. hirsutus was taken up. In the current study, we have focused on investigating the effects of C. hirsutus in in vitro and in vivo tests of protection against dengue.
We found C. hirsutus to have more potent anti-dengue activity as compared with C. pareira. This traditional plant has been used as a detoxifier, aphrodisiac, antipyretic, cardiotonic, etc., reflecting its wide medicinal utility (Marya and Bothara, 2011). Prior reports have also suggested its use for the treatment of chronic rheumatism, syphilitic cachexia, skin diseases, constipation, kidney problems, etc. (Marya and Bothara, 2011). In an animal toxicity study, aqueous extract of aerial parts of C. hirsutus was found to be safe, and acute toxicity was established to be higher than 3,000 mg/kg body weight (Ganapaty et al., 2002).
We report for the first time the potential of C. hirsutus-based drug for the treatment of dengue. We have investigated the efficacy of C. hirsutus extracts using different parts of plant and solvents under varied drying conditions. The anti-dengue activity was established through a flow-cytometry-based neutralization test (FNT), which is more quantitative and robust as compared with plaque-based virus neutralization assays (PRNT) (Kraus et al., 2007). Purified aqueous extract of the stem of C. hirsutus (AQCH) was found to possess the highest pan-DENV inhibitory activity. Through chemical fingerprinting analysis, five marker compounds, Sinococuline, Magnoflorine, 20-Hydroxyecdysone, Makisterone-A, and Coniferyl alcohol, were identified to be present consistently in different batches of AQCH. Only Sinococuline has been found to exhibit potent pan-anti-dengue activity. Furthermore, AQCH phytopharmaceutical substance was found to be protective in the primary and secondary DENV infection in AG129 mice. Importantly, AQCH phytopharmaceutical reduced serum viremia and small intestinal pathologies, viz., viral load, pro-inflammatory cytokines, and vascular leakage. These results have provided the optimism to advance the evaluation of AQCH as a potential phytopharmaceutical for the treatment of dengue through appropriate animal toxicity, human safety, pharmacologic, and efficacy trials.
This study involved experiments on AG129 mice, which were performed at the International Centre for Genetic Engineering and Biotechnology, New Delhi (ICGEB/IAEC/08/2016/RGP-15), in compliance with the Committee for the Purpose of Control and Supervision of Experiments on Animals guidelines issued by the Government of India.
The Vero cell line was purchased from the American Type Cell Culture, VA, United States. This monkey kidney cell line was maintained using Dulbecco’s Modified Eagle medium (DMEM) supplemented with 10% ΔFBS in a 10% CO2 humidified incubator at 37°C. World Health Organization reference DENV strains DENV-1 (WP 74), DENV-2 (S16803), DENV-3 (CH53489), and DENV-4 (TVP-360) were received from Dr. Aravinda de Silva’s lab, University of North Carolina, United States. Anti-dengue 2H2 antibody was produced in-house from its hybridoma.
Organic solvents used in the plant extraction and high-performance liquid chromatography (HPLC) analysis were procured from E. Merck Ltd., Mumbai, India, either of analytical or HPLC grade. Before use, solvents were also filtered through a 0.45-μm membrane filter (Millipore, Billerica, MA, United States). The HPLC column RP18e Purospher-STAR (Hibar) (250 × 4.6 mm; 5μm) was used for chemical fingerprinting (E. Merck) and Eclipse 5 μm; 9.4 × 250 mm was used for purification of marker compounds (Agilent). The chemicals and reagents used for standardization and quality control were procured from Sigma-Aldrich, United States. Water for extraction and HPLC analysis was obtained from a high-purity Milli-Q Advantage A10 water purification system (Millipore, Molsheim, France).
The botanical raw material (BRM), i.e., aerial or stem parts of C. pareira and C. hirsutus, were collected by the botanist from Mandawara block, Lalitpur district, Uttar Pradesh, India. Identification of the collected BRM was performed at the Plant Science Division of Council of Scientific and Industrial Research—Indian Institute of Integrative Medicine (CSIR-IIIM), Jammu, India. Duly identified herbarium specimens of C. pareira (accession no. RRLH-23148) and C. hirsutus (accession no. RRLH-23152) were submitted to internationally recognized Janaki Ammal Herbarium at CSIR-IIIM, Jammu. Furthermore, for some studies, BRM of C. hirsutus collected from the same area was procured from the local vendor at Lalitpur. After critical macroscopic and microscopic examination, the botanical identity of the procured BRM samples of C. hirsutus was confirmed at the Plant Science Division of CSIR-IIIM. The duly identified samples of the procured BRM, i.e., aerial (accession nos. CDR-4037 and CDR-4038) and stem (accession nos. CDR-4061, CDR-4064, CDR-4065, and CDR-4078) parts of C. hirsutus, have been submitted to the Crude Drug Repository at CSIR-IIIM, Jammu.
BRM was dried in the shade and pulverized. The grounded plant masses of C. pareira and/or C. hirsutus were separately charged into an extractor at ambient temperature. The extracting solvent used were: (1) Hydro-alcohol, 50:50, (2) Denatured Spirit, and (3) Water. The extraction solvent was added to the pulverized BRM, and the mixture was heated to reflux temperature for about 3 h. The extracted mass was filtered, collected, and stored in a container. The extraction process was repeated from the residue plant mass two more times. All the three filtered extracts were combined, concentrated, and further dried either using rotor vapor, tray under vacuum, or spray drying. The yield of dry extract (drug substance) obtained was 5–14%, depending on the part of the plant used and the extracting solvent.
The phytopharmaceutical drug-based AQCH tablets 100/300/500 mg has been developed as an immediate-release formulation. The dry granulation method has been selected as the manufacturing process based on the hygroscopic nature of AQCH. The stability of AQCH tablets was extensively evaluated, and the drug product was found to be satisfactory for tested up to 6 months in accelerated conditions [40 ± 2°C/75 ± 5% relative humidity (RH) and for tested up to 18 months in long-term conditions (30 ± 2°C/65 ± 5% RH)] (Supplementary Table 3).
Vero cells were seeded in a 96-well plate (∼25,000 cells/well) in 200-μl DMEM + 10% ΔFBS and incubated for 24 h in an incubator adjusted at 37°C and 10% CO2. Next day, cells were infected with DENV-1, -2, -3, and -4 at a multiplicity of infection of 0.1 (5,000 fluorescence-activated cell sorting infectious unit; FIU) in 100-μl DMEM + 0.5% ΔFBS. After a 2-h incubation of Vero cells with the virus at 37°C and 10% CO2, virus infection media was aspirated, and a suitable range of extract/drug concentration to be tested was added to the wells in 200-μl DMEM + 0.5% ΔFBS in duplicates. Cells were incubated further for another 46 h in an incubator at 37°C and 10% CO2. Wells infected with the virus without any subsequent treatment served as virus controls, whereas wells with no infection and no treatment served as cell controls. These experimental controls were utilized for relative virus infection calculations and antibody background signal adjustments, respectively. Post-incubation, cells were trypsinized, fixed using 4% paraformaldehyde, and stained with anti-prM Alexa-488-labeled 2H2 antibody as reported elsewhere (Kraus et al., 2007; Shukla et al., 2020b). Stained cells were assessed for virus infection using a flow cytometer (BD FACS Verse/Lyric), and data were analyzed through Flowjo software. Extract/drug concentration resulting in a 50% reduction in the number of DENV infected cells with reference to the virus control, which represents 100% infection, was defined as the 50% inhibitory concentration (IC50) of the herbal extract/drug tested. All the extracts/drug/compounds prepared during this study were analyzed using this flow cytometry-based virus inhibition assay for assessing their anti-dengue potential.
The in vitro cell cytotoxic index (CC50) of AQCH was evaluated through 3-(4, 5-dimethylthiazolyl-2)-2,5-diphenyltetrazolium bromide (MTT) assay. Vero cells were seeded as described in the flow-cytometry-based virus inhibition assay. Post-24-h incubation at 37°C and 10% CO2, overlay media was removed, and 200 μl of a suitable concentration range of AQCH prepared in dilution media (DMEM + 0.5% ΔFBS) was added to the wells in duplicates; cells incubated with dilution media alone were kept as cell control and processed in parallel. Cells were incubated further for another 46 h in an incubator at 37°C, 10% CO2. Post-incubation, 10 μl of 5 mg/ml MTT reagent (procured from Sigma Aldrich, United States) prepared in PBS was added and further incubated for 2 h at 37°C, 10% CO2. Upon the formation of formazan crystals, the overlay was removed, and 100 μl of dimethyl sulfoxide was added. After the dissolution of crystals in dimethyl sulfoxide, absorbance was taken at 570 nm. The % cell cytotoxicity was calculated for each AQCH concentration with respect to cell control. The concentration of AQCH at which 50% cell cytotoxicity was observed is reported as CC50.
All nuclear magnetic resonance (NMR) spectral data were recorded on a Bruker 400-MHz spectrometer. Chemical shifts (δ) were referenced internally to the residual solvent peak (CD3OD: 1H δ 3.30, 13C δ 49.0 ppm; CDCl3: 1H 7.26, 13C 77.0 ppm), and the reference point was tetramethylsilane (δH and δC: 0.00 ppm). High-resolution electrospray ionization mass spectrometry spectra were recorded on an Agilent 1100 LC-Q-TOF mass spectrometer and HRMS-6540-UHD machines. HPLC purifications were performed on Thermo Scientific Dionex UltiMate 3000 HPLC system with ultraviolet (UV) detector. Column chromatography was performed using silica gel (60–120 and 230–400 mesh); fractions were monitored by thin-layer chromatography (TLC) using pre-coated silica gel plates 60 F254 (Merck). Spots were visualized by UV light or by spraying with H2SO4-MeOH, anisaldehyde-H2SO4 reagents.
For the HPLC fingerprinting, 100 mg of AQCH (FCH 1909006) was transferred in a 20-ml volumetric flask, added approximately 10 ml of diluent and sonicated/shaken/stirred for 5–10 min to dissolve and made up the volume with diluent and mixed and filtered through a 0.45-m filter for HPLC fingerprinting. It was performed on RP18e Purospher-STAR (Hibar) (250 × 4.6 mm; 5μm) column. The mobile phase containing a buffer (0.1% formic acid in water) and acetonitrile was used at the flow rate of 0.65 ml/min at a column temperature of 30°C at 254-nm wavelength. The volume of injection was 5 μl, and the total run time of the assay was 75 min. A gradient program was used as follows: 0–15 min, 00–05% B; 15–40 min, 05–20% B; 40–55 min, 20–30% B; 55–65 min, 30–60% B; 65–68 min, 60–00% B and 68–75 min, 00% B. For the isolation, a portion of AQCH (500 gm) was suspended in distilled water and partitioned between ethyl acetate (A) and H2O (B). The aqueous layer (B) was basified with NH4OH solution (pH 9) and then extracted with chloroform, which resulted in a chloroform layer (4.0 g, C) and aqueous layer (D). The CHCl3 layer (C) was further purified through repeated column chromatography in neutral alumina eluted with a gradient of CHCl3-MeOH (100:0–0:100) to obtain Sinococuline (1) as a major constituent along with Magnoflorine (2). The aqueous layer (D) was lyophilized (480.5 g) and suspended in methanol. The methanol soluble portion (400.0 g) was purified by column chromatography (silica gel, 100–200 mesh), eluted with a gradient of CHCl3-MeOH (100:0–0:100, 500-ml collected volumes of each fraction), and concentrated, giving 50 fractions (Fr.1–Fr.50), and their composition was monitored by TLC, with those showing similar TLC profiles grouped into six major fractions (Fr-1a to Fr-6a). Fraction Fr-2a afforded two UV active compounds as crystals. These crystals containing two UV active compounds were further subjected to semi-preparative C18 reversed-phase HPLC [Eclipse 5 μm; 9.4 × 250 mm; 3 ml/min; gradient of water (B)/acetonitrile (A) over 32 min; 100–80% B (5 min), 80–60% B (5 min), 60–50% B (5 min), 50–40% B (5 min), 40–20% B (5 min), 20–45% B (3 min), 45–70% B (2 min), and 70–100% B (2 min); column oven temperature 25°C] to give 20-Hydroxyecdysone (3) and Makisterone-A (4). The EtOAc soluble fraction (A) was subjected to column chromatography (silica gel, 100–200 mesh), eluted with a gradient of CHCl3-MeOH (100:0–0:100, 250-ml collected volumes of each fraction) and concentrated, giving 30 fractions (Fr.51–Fr.80). Coniferyl alcohol (5) was obtained from fractions Fr. 66–Fr. 70 in pure. All the isolated compounds 1–5 were identified by detailed spectral analysis using one- and two-dimensional NMR and HRESI-MS data (Supplementary Figure 1) and compared with the reported spectral data (Ishii et al., 1984; Itokawa et al., 1987; Nakasone et al., 1996; Snogan et al., 2007; Taha-Salaime et al., 2019).
AQCH was formulated into tablets of different strengths (100, 300, and 500 mg) using the approved excipients. The accelerated and long-term stability of AQCH and AQCH tablets was assessed by exposing them to different conditions (30 ± 2°C/65 ± 5% RH and 40 ± 2°C/75 ± 5% RH). The in vitro anti-dengue activity and the content of a selected marker compound were evaluated for the stored samples at different time points (1, 2, 3, and 6 months).
A monoclonal anti-flavivirus antibody, 4G2, was produced in-house using hybridoma procured from the American Type Cell Culture. DENV-2 strain S221 used for in vivo studies was obtained from Global Vaccines Inc., NC, United States, and propagated using DMEM adapted C6/36 cell line for preparing virus stocks. The titer of these stocks was estimated using Vero cells in FIU per milliliter, as described elsewhere (Lambeth et al., 2005). AG129 mice were purchased from B&K Universal, United Kingdom, and bred in-house at the International Centre for Genetic Engineering and Biotechnology, New Delhi, for all animal experiments.
Primary DENV infection was established in a type-I and type-II interferon (IFN) receptor knockout, AG129 mice (Shresta et al., 2006; Sarathy et al., 2015) by challenging them with a lethal dose (1 × 105 FIU; intravenous) of DENV-2 S221. Mice were orally gavaged with AQCH, 33 and 100 mg/kg/day (prepared in 0.1% w/v methylcellulose), for 5 days using twice a day (BID), three times a day (TID), and four times a day (QID) dosing regimens starting 30 min post-infection as reported elsewhere (Watanabe et al., 2012). The level of protection conferred by AQCH was monitored through mortality and morbidity scores for 15 days post-lethal challenge. The morbidity score was assessed based on a five-point system: 0.5, mild ruffled fur; 1.0, ruffled fur; 1.5, compromised eyes; 2, compromised eyes with hunched back; 2.5, loose stools; 3.0, limited movement; 3.5, no movement/hind leg paralysis; 4.0, euthanized if the cumulative score was 4; 5.0, assigned to the animal that was dead as reported previously (Ramasamy et al., 2018; Shukla et al., 2020c). The level of protection was scored through % survival for 15 days post-lethal challenge.
The secondary dengue AG129 mouse model was established through intravenous inoculation of immune complexes (IC) of sublethal dose of DENV-2 S221 (2 × 104 FIU) made with neutralizing concentration of 4G2 mAb (10 μg) reported elsewhere (Watanabe et al., 2015; Shukla et al., 2020c, b). IC challenged mice (n = 6) were orally gavaged with different doses of AQCH, 33 and 100 mg/kg/day (prepared in 0.1% w/v methylcellulose), in the BID, TID, and QID dosing regimens for 5 days post-ICs inoculation. The level of protection conferred by AQCH was examined in a similar manner as in the primary dengue AG129 model.
Two groups of IC injected AG129 mice (n = 6) were orally fed with AQCH at 8.25 and 25 mg/kg/dose QID (equivalent to 33 and 100 mg/kg/day, respectively) for 4 days. Serum was collected from each group (n = 3) on day 4 for assessing viral RNA equivalents through real-time quantitative polymerase chain reaction, and post-sera collection vascular leakage was quantified in these mice using Evans blue dye, as detailed in the Supplementary Protocol 1. The remaining three mice from each group were killed on day 4 and small intestines harvested for assessing viral load and levels of tumor necrosis factor-alpha (TNF-α) and interleukin-6 (IL-6) cytokines as elaborated in the Supplementary Material.
Statistical differences between two groups of treated animals were calculated through two-way analysis of variance (with Bonferroni’s correction for multiple comparisons). The Kaplan–Meier survival curves were analyzed by the log-rank test for significance. p-values of ≤0.05 and <0.001 were considered significant and very significant, respectively. All statistical calculations were performed using GraphPad Prism (v8.0) software.
Guided by the Indian Ayurveda literature and our in vitro and in vivo bioassays, we had earlier identified and established that methanolic extract of the aerial parts of C. pareira possesses pan-anti-dengue activity (Sood et al., 2015). C. pareira belongs to the family Menispermaceae, which is historically known to be rich in a variety of alkaloids (Barbosa-Filho et al., 2000). In our previous study (Sood et al., 2015), a total of 19 plants were evaluated for their anti-dengue activity, two of which C. pareira and Tinospora cordifolia belonged to the family Menispermaceae, and both of them were found to possess anti-dengue activity. However, C. pareira, which belongs to the Cocculeae tribe of Menispermaceae, exhibited significantly stronger anti-dengue activity than T. cordifolia, which belongs to the Tinosporeae tribe.
In the present study, we have investigated the anti-dengue activity of plant extracts from aerial parts and stem from C. hirsutus, which has a morphologic resemblance to C. pareira but was found to be microscopically altered in their anatomical characters (Supplementary Table 1). We initiated work on C. hirsutus because of the finding that it is often present with C. pareira in dried collections made from a local vendor.
With methanolic extract of aerial parts of C. pareira as our benchmark, we prepared three batches each of methanolic extract of aerial parts of C. pareira and C. hirsutus in a manner similar to that stated in Sood et al. (2015). In this study, we evaluated all these six methanolic extracts for anti-dengue activity in an in vitro FNT instead of plaque-based bioassay used in Sood et al. (2015). The FNT and plaque-based bioassays are principally similar. However, FNT-based evaluation is advantageous because it is high-throughput and is more stringent, as it uses a higher dose of DENV for the evaluation of the anti-dengue activity. In the FNT assay used in the current study, the Vero cells were infected with 0.1 multiplicity of infection of each DENV serotypes, and post-infection the cells were incubated in media containing extract at various concentrations for 46 h. Post-incubation cells were fixed, permeabilized, and stained with Alexa fluor-labeled anti-dengue mAb, 2H2, reactive to all the four DENV serotypes, which were read in a flow cytometer to determine the percentage of DENV infected cells. This was used to calculate the extract concentration at which 50% of the DENV infection was inhibited (IC50). Upon parallel evaluation of all the six extracts in FNT, it was observed that all the three batches of methanolic aerial C. hirsutus extracts possessed significantly stronger anti-dengue activity against all the four DENV serotypes as compared with methanolic aerial C. pareira extracts (Supplementary Figure 1).
Upon finding C. hirsutus possessing more potent anti-dengue activity as compared with C. pareira, we explored individual preparation of extracts of both aerial (Figure 1A; dashed curves) and stem portions (Figure 1A; solid curves) of C. hirsutus in various solvents such as denatured spirit, hydro-alcohol (50:50), and water. These extracts were evaluated against all the four DENV serotypes by FNT, and their IC50 values were compared (Figure 1B). It was observed that the stem portion of C. hirsutus was significantly more potent than the aerial part, irrespective of the solvent used. Thus, aqueous extract of stem of C. hirsutus, hereafter referred to as AQCH, was advanced further due to regulatory compliance factors.
Figure 1. Evaluation of extracts of aerial and stem parts of C. hirsutus prepared using various extraction solvents: extracts of aerial and stem only parts of C. hirsutus were prepared in different solvents, viz., denatured spirit, hydro-alcohol (50:50), and aqueous. Anti-dengue activity of each of these extracts at various concentrations was evaluated against DENV-1 (magenta curve), DENV-2 (green curve), DENV-3 (blue curve), and DENV-4 (black curve) by flow-cytometry-based virus inhibition assay. (A) % Dengue virus (DENV) infection relative to virus control achieved is represented graphically for denatured spirit (left panel), hydro-alcohol, 50:50 (middle panel), and aqueous (right panel) aerial (dashed curves) and stem (solid curves) extracts. (B) Concentration of extract (μg/ml) that resulted in 50% inhibition of viral infection as compared with virus control [represented by horizontal dotted line in panel (A)], calculated as IC50 using Graphpad Prism, is shown in table for all extracts.
High-performance liquid chromatography (HPLC) was performed on the AQCH batch (ID: KL/DBE/002/18) post-confirmation of its pan-anti-dengue activity by FNT (Figure 2A) for its chemical profiling. Additionally, the extent of possible in vitro cytotoxicity caused to Vero cells by AQCH was also evaluated by MTT assay, and the CC50 was determined to be ∼90 μg/ml (Figure 2A). The HPLC chromatogram obtained is shown in Figure 2B. This was followed by isolation of five marker compounds using repeated chromatographic methods and characterized using advanced one- and two-dimensional NMR spectroscopic and mass analysis. Marker compounds were identified to be Sinococuline (1), Magnoflorine (2), 20-Hydroxyecdysone (3), Makisterone-A (4), and Coniferyl alcohol (5) (Figure 2C). The physicochemical data of all the five identified marker compounds are given in Supplementary Table 2.
Figure 2. Chemical fingerprinting of AQCH: (A) AQCH batch KL/DBE/002/18 was prepared, and its anti-dengue activity against DENV-1 (magenta curve), DENV-2 (green curve), DENV-3 (blue curve), and DENV-4 (black curve) was confirmed by flow-cytometry-based virus inhibition assay as represented by graph of % DENV infection on left y-axis and extract concentration. Extent of cell cytotoxicity caused by AQCH (represented by red curve) was also measured by MTT assay that is reflected on right y-axis of graph as % cell cytotoxicity for given extract concentrations on x-axis. CC50 and IC50 values corresponding to concentration of AQCH that is toxic for 50% of cells and at which 50% of DENV infection is inhibited as compared with virus control, respectively, has been represented by a dotted horizontal line; a table of IC50 and CC50 values has been provided as an inset. (B) High-performance liquid chromatography (HPLC) chemical fingerprinting profile of AQCH with peaks corresponding to five identified marker compounds annotated. (C) Chemical structure of five marker compounds (1–5).
The robustness and consistency of AQCH were monitored by the preparation of various batches of AQCH extract utilizing one of the three drying methods—rotar vapor drying, vacuum tray drying, and spray drying. Irrespective of the method used for drying, the in vitro anti-dengue activity of all the extract batches prepared was comparable (Supplementary Figure 2A). The HPLC chromatograms of three batches corresponding to the three drying methods were observed to be overlapping (Supplementary Figure 2B), with a high degree of consistency in retention times of the five marker compounds (Supplementary Figure 2C). This indicates that the AQCH extract preparation method is consistent and robust, and the choice of drying method does not have any implication on its chemical profiling and biological activity. Thus, spray drying was considered as the method of choice, as it resulted in the formation of free-flowing finer extract in a shorter span of time, which is industrially more compatible. Furthermore, the identified compound markers were assessed for their pan anti-dengue inhibitory potency by FNT (Figure 3), and only Sinococuline was found to have anti-dengue inhibitory activity against all four DENV serotypes (Figure 3A). The other chemical entities characterized in the drug substance, Magnoflorine, 20-Hydroxyecdysone, Makisterone-A, and Coniferyl alcohol, showed almost negligible anti-dengue activity (Figures 3B–E).
Figure 3. In vitro flow-cytometry-based dengue virus (DENV) inhibition analysis of all AQCH marker compounds. Five makers compounds (A–E) isolated from AQCH were tested for their anti-dengue inhibitory potency against DENV-1 (magenta curve), DENV-2 (green curve), DENV-3 (blue curve), and DENV-4 (black curve) serotypes using in vitro flow-cytometry-based virus inhibition assay. X-axis denotes compound concentrations (μg/ml) in each panel, and y-axis represents percent virus inhibition against virus control (no compound was added). Horizontal dotted line of each panels shows 50% inhibition of viral infection as compared with virus control, and IC50 was calculated for each of compounds using Graphpad Prism.
To assess the in vivo AQCH efficacy, we tested AQCH in dengue-sensitive AG129 mouse model. AG129 mice are IFN type I and II receptor-deficient mice that allow viral infection to propagate and develop dengue viral symptoms. To develop the DENV disease symptoms in AG129 mice, we optimized the mouse-adapted DENV-2 strain S221 dose, which could develop dengue severe disease symptoms in the absence of any antiviral treatment. Briefly, AG129 mice (n = 6) were challenged with a lethal dose (1.0 × 105 FIU) of DENV-2 S221, which killed all the mice by days 6–7. We utilized this primary DENV infection model for the assessment of AQCH efficacy in vivo. In this experiment, we tested AQCH in three different dosing regimens, i.e., BID (orange curves), TID (purple curves), and QID (blue curve) in Figure 4, at two different doses of 100 mg/kg/day (solid curves) and 33 mg/kg/day (dashed curves) (Figure 4A). It was observed that BID and TID regimens too conferred similar protection, 100% survival at 100 and ∼33% at 33 mg/kg/day, as fed QID for 5 days post-infection. Thus, there was no significant difference in the survival scores of mice between different dosing regimens at either of the two AQCH doses (p > 0.9999). However, the morbidity and weight loss scores of mice were moderately higher under the BID regimen (p = 0.0019) than TID (p = 0.0295) and QID for each of the two doses (Figures 4C,D). Outcomes with control mice groups were observed as expected as 0% survival in the unfed infected group “V” (green curve) and 100% survival in AQCH-fed uninfected group “Only AQCH” (black curve). Among the two doses, significantly lower protection and higher morbidity were observed at 33 mg/kg/day AQCH treatment in the BID and TID dosing regimens (p = 0.01).
Figure 4. Effect of AQCH dose and dosing regimen against lethal primary DENV infection. (A) Schematic representation of study design. Groups of AG129 mice (n = 6) inoculated with lethal dose of mouse-adapted DENV-2 S221 virus (105 FIU/Mouse); thereafter, mice were orally gavage with two doses of AQCH, viz., 100 mg/kg/day (solid curves) and 33 mg/kg/day (dashed curves) in three different dosing regimen, i.e., twice a day (BID; orange curves), thrice a day (TID; purple curves), and four times a day (QID; blue curves) for 5 days and mice were monitored for another 10 days, for their survival (B), morbidity (C), and body weight change (D). Uninfected (neither infected with virus nor fed with AQCH; represented by magenta), virus-infected, V (infected with virus but not fed with AQCH; represented by green curves), and Only AQCH (not infected with virus but fed with AQCH at 25 mg/kg QID; black curves) groups of AG129 mice served as experimental controls. Not significant (ns) and single star (*) of right side of survival graph denotes their statistical differences between same dosing schedule of different doses.
Secondary dengue infection is one of the worst scenarios in dengue infection and develops very severe dengue disease through antibody-dependent enhancement (ADE) pathway (Halstead et al., 2010; Guzman et al., 2013). Thus, we investigated the therapeutic potential of AQCH against severe DENV infection using the secondary dengue AG129 mouse model. The IC of DENV-2 S221 were made in vitro by mixing 100% neutralizing concentration of monoclonal antibody 4G2 (10 μg). The 4G2 mAb potentially binds the fusion loop of all four DENV serotypes and tends to enhance DENV infection by following Fcγ receptor-mediated intrinsic ADE, which is manifested as severe dengue infections (DHF/DSS) as evidenced elsewhere (Halstead et al., 2010; Shukla et al., 2020a). We used this ADE model to evaluate AQCH efficacy in vivo. The IC inoculated mice were fed orally with previously primary dengue disease model tested doses of AQCH, viz., 33 (dashed curves) or 100 mg/kg/day (solid curves), using BID (orange curves), TID (purple curves), and QID (blue curves) dosing regimens for 5 days post-IC inoculation (Figure 5). The outcomes with control groups, viz., “Un-infected” (magenta curve), “V” (green curve), “Only AQCH” (black curve), and “IC” (gray curves), were as expected. Unlike the case in the primary dengue AG129 model, the efficacy of AQCH was not found to be similar across the three evaluated dosing regimens. QID was the most effective dosing regimen followed by TID and BID as 100%, 50%, and 0% mice survival rates were observed, respectively, with AQCH treatment at 100 mg/kg/day dose (Figure 5B). Since protection was not observed at 100 mg/kg/day AQCH dose in BID regimen, a lower AQCH dose of 33 mg/kg/day was evaluated only in TID and QID regimens, where it exhibited ∼33% and 50% protection, respectively (Figure 5B), which suggests that BID and TID dosing regimens are not appropriate regimens for treating severely infected AG129 mice. Also, morbidity scores and body weight changes correlated well across BID, TID, and QID regimens at 100 mg/kg/day with the observed survival efficacies (Figures 5C,D).
Figure 5. Effect of AQCH dose and dosing regimen against secondary DENV infection: (A) schematic representation elaborating that AG129 mice (n = 6) were injected with sub-lethal dose of DENV-2 S221 alone (marked as V; green curve) or as DENV-2 S221-4G2 IC (IC). IC injected AG129 mice were orally fed with either AQCH at indicated higher (solid curve) and lower (dashed curve) doses or only methylcellulose (marked as IC; gray curve) for 5 days in BID (orange), TID (purple), and QID (blue) dosing regimens. Uninfected (magenta curves) and Only AQCH (black curves) groups of AG129 mice were used as experimental controls. (B–D) represents their survival curves, morbidity score, and % body weight changes, respectively, which were observed during entire study duration of 15 days. Statistical differences (ns; not significant, and *; less significant) of survival between different dosing schedules are represented on the right side of graph.
Severe dengue disease is known to be associated with small intestinal pathologies of enhanced viral load, increased levels of pro-inflammatory cytokines, and vascular permeability (Vejchapipat et al., 2006; Watanabe et al., 2018). Thus, it was of interest to determine the impact of AQCH treatment on small intestinal pathology, which could be examined in secondary dengue disease AG129 mice exhibiting ADE-mediated severe dengue.
It has been reported that small intestinal pathology is a better correlate of protection than serum viremia levels in dengue disease (Watanabe et al., 2018). Thus, we evaluated the effect of AQCH treatment on both the serum viremia and small intestinal viral load. Secondary dengue disease AG129 mice (n = 3) were orally fed with AQCH (8.25 and 25 mg/kg/dose, QID) for 4 days. Mice injected with IC showed symptoms of disease onset such as lethargy, whereas the mice treated with 25 mg/kg/dose QID of AQCH showed no disease symptoms (Supplementary Media File). Viremia levels were determined in serum and small intestinal tissue samples collected on day 4. Mice treatment with AQCH significantly reduced (p < 0.0001) the virus load in both the serum (Figure 6A) and small intestine (Figure 6B). However, unlike the observation with small intestinal viral load, reduction in serum viremia was not found to be dose-dependent, as QID treatment with 8.25 mg/kg/dose (blue empty bar) of AQCH led to a higher reduction in serum viremia than 25 mg/kg/dose, blue solid bar (Figure 6A).
Figure 6. Serum and intestinal viral inhibition by AQCH in secondary DENV infection: AG129 mice (n = 3) inoculated with DENV-2 S221-4G2 IC to establish secondary dengue infection were orally fed with either 25 mg/kg QID (blue solid bar) or 8.25 mg/kg QID (blue empty bar) of AQCH. Serum samples were withdrawn on day 4, and mice were killed to collect small intestinal tissue. Serum viremia levels (A) and small intestinal viral load (B) were determined using SYBR green-based real-time PCR. “Only AQCH,” “V,” and “IC” control groups are shown in black, green, and gray bars, respectively. Signals detected in “Only AQCH” group (black bars) considered as real-time quantitative polymerase chain reaction background, as this group was fed with AQCH (25 mg/kg QID) only and not infected with virus. Statistical differences between groups are presented on the top of bar graphs, ns, not significant (p ≥ 0.05), ***very high significant difference (p < 0.0001).
Since vascular leakage is a prominent manifestation of severe dengue infections (DHF/DSS), we assessed intestinal vascular leakage using Evans blue dye, which serves as a marker for albumin extravasation. The leakage was quantified through colorimetric estimation of the dye amount absorbed, which was represented as a fold increase in OD620 per gm of tissue’s wet weight in comparison with the “Un-infected” control. AQCH treatment was observed to significantly (p = 0.0015) reduce the vascular leakage at both the dose levels—25 and 8.25 mg/kg/dose QID (Figure 7A, solid and empty blue bars, respectively). Enhanced vascular leakage was observed in the “IC” mice group (Figure 7A, gray bar), as expected. The visual examination of day 4 small intestines of mice also reflected similar findings with distinctive fluid accumulation in the “IC” group, whereas the small intestines of other groups—“V,” “IC + 25 mg/kg/dose AQCH QID,” and “IC + 8.25 mg/kg/dose AQCH QID”—appeared normal and similar to the “Only AQCH” group (Figure 7B).
Figure 7. Inhibition of intestinal vascular leakage and reduction in cytokine storm by AQCH in secondary dengue virus (DENV) infection. Secondary dengue AG129 mice (n = 6) were treated with AQCH either at 25 mg/kg (solid blue bar) or 8.25 mg/kg (empty blue bar) QID dose for 4 days. (A) On day 4, three AG129 mice of each group were examined for vascular leakage in small intestine by Evans blue staining assay. Data are depicted as fold-change in dye staining with reference to an untreated control that had not received any AQCH treatment. A fold-change of 1 corresponds to basal vascular leakage equivalent to untreated group. Remaining three mice of each group were killed on day 4, perfused them with sterile PBS, and luminal content was flushed again with PBS for identification of visual intestinal vascular leakage, a representative vascular leak from each group is shown in panel (B). Fifty milligrams of small intestines from panel (B) were prepared for determination of TNF-α (C) and IL-6 (D) by enzyme-linked immunosorbent assay, as reported earlier (Shukla et al., 2018). Control groups used in this study were “Only AQCH” group (black bar) and Virus only group (V; green bar); IC group (gray bar) is shown in each of panels. Statistical differences between two groups were calculated through two analysis of variance with Bonferroni correction and shown at top of bar graphs; ns, not significant, *p = 0.01, **p = 0.001, and ***p < 0.0001.
The manifestation of severe dengue infections occurs during the critical phase of the infection, and levels of pro-inflammatory cytokines, such as TNF-α and IL-6, become elevated, which leads to further disease progression and causes DHF/DSS. To assess the anti-inflammatory activity of AQCH, we evaluated the expression levels of pro-inflammatory cytokines, TNF-α (Figure 7C), and IL-6 (Figure 7D), in small intestines of mice. The results obtained were in correlation with the findings of vascular leakage examination. Treatment with AQCH inhibited the secretion of cytokines in the small intestine in a dose-dependent manner. The “IC” group animals showed the highest expression levels of cytokines (TNF-α and IL-6), which was expected due to 4G2-mediated ADE in secondary dengue infection AG129 mice (Figures 7C,D).
With the finding of strong and consistent in vitro and in vivo anti-dengue potency of AQCH, we formulated the drug substance into 100-, 300-, and 500-mg strengths of AQCH tablets (drug product). The tablets were subjected to accelerated and long-term stability studies along with the AQCH drug substance. Samples from the stability study were analyzed for Magnoflorine content and in vitro anti-DENV-2 activity by FNT to evaluate any deterioration or loss in bioactivity upon storage under the conditions stated. It was found that there was neither a significant change in the Magnoflorine content (Supplementary Table 3) nor any loss of anti-DENV-2 activity (Table 1) of AQCH and AQCH tablets of all the three strengths (100, 300, and 500 mg) under the condition tested up to 6 months. The long-term stability study is ongoing, and samples will be evaluated for up to 3 years of storage.
Plants have been known and traditionally used worldwide for their therapeutic potential since time immemorial. Their therapeutic usefulness has been documented all around the globe in various traditional pieces of literature. Hence, these classical troves, which detail the medicinal utility of plants, are an attractive repertoire of knowledge that could be explored through contemporary methods for the development of safe and effective therapies for various diseases. This has ushered the development of many plant-derived molecules, which today are in clinical use globally, morphine being the first FDA-approved plant-derived molecule (Li and Weng, 2017). Additional examples include antimalarials such as Quinine from Cinchona bark used widely. It is also noticed that approximately 25% of the drugs prescribed worldwide are derived from plants, and such active compounds are in use (Sahoo et al., 2009).
Dengue is one of the world’s rapidly spreading arboviral diseases, with the incidence of symptomatic dengue doubling every decade (Stanaway et al., 2016). The highest burden of this disease lies in Southeast Asia, with India being one of the epicenters (Bhatt et al., 2013; Stanaway et al., 2016). According to a study, actual dengue cases in India are ∼282 times higher than that reported annually, having an economic impact of USD ∼1.11 billion (Shepard et al., 2014). Thus, there is a dire need for an effective dengue vaccine and/or drug to fight against dengue. There have been many efforts toward the development of drugs for dengue, including the repurposing of approved drugs (Low et al., 2017; Botta et al., 2018). However, none of the drugs have yet succeeded in proof-of-concept trials, and thus, a dengue antiviral still remains an unmet need.
Our group had previously evaluated 19 medicinal plants for their anti-dengue activity that led to the identification of C. pareira of the Menispermaceae family as the most potent plant (Sood et al., 2015). Our continued exploration has led to the identification of C. hirsutus with significantly higher anti-dengue potential than C. pareira. When the methanolic extracts of aerial parts of C. hirsutus and C. pareira were compared head-on in an in vitro FNT assay, we found that C. hirsutus have significantly more potent anti-dengue activity (Supplementary Figure 1).
We next investigated if methanol extraction could be replaced with milder solvents such as denatured spirit, hydro-alcohol (50:50), or water. In a series of investigations, we prepared the extract of stem and aerial parts of C. hirsutus in various solvents and evaluated the drug substance for their anti-dengue activity (Figure 1). These experiments yielded two important outcomes. First, irrespective of the solvent used, the stem of C. hirsutus was significantly more potent in its anti-dengue activity than the aerial part. Second, the stem extract prepared in water was comparable in its anti-dengue activity to other tested solvents. Thus, aqueous extract of the stem of C. hirsutus (AQCH) was selected as the extract of choice for the preparation of lab-scale and GMP scale drug substances.
After establishing the anti-dengue potential of AQCH through bioassays, we further evaluated the industrial viability of AQCH drug substance production. For this, an AQCH batch was profiled through HPLC, and five marker compounds—Sinococuline, Magnoflorine, 20-Hydroxyecdysone, Makisterone-A, and Coniferyl alcohol—were identified (Figure 2). The chemical profiling data of a bioactive batch of AQCH enabled us to modify our extraction method without compromising the bioactivity or quality of the extract prepared. Thus, various batches of AQCH were prepared using three different drying methods, viz., rotary vapor, vacuum tray, or spray drying. All the AQCH batches were found to exhibit similar anti-dengue activity (Supplementary Figure 2A) irrespective of the drying process, indicating the robustness and consistency of the method of extraction. This was corroborated by their HPLC chemical fingerprinting that yielded similar chromatograms (Supplementary Figures 2B,C). Spray-dried AQCH extracts were utilized for further evaluations due to greater industrial compatibility. Additionally, the identified compound markers were evaluated for their pan-anti-dengue inhibitory potency by flow-cytometry based inhibition assay (Figure 3), and only Sinococuline was found to have anti-dengue inhibitory activity against all four DENV serotypes.
AQCH drug substance was evaluated for its protective efficacy in vivo in AG129 mice in primary (Figure 4) and secondary dengue infection (Figure 5) settings, which is an established model for the evaluation of antivirals (Watanabe et al., 2018). AG129 mice being deficient in IFN-α/β and γ receptors allow propagation of DENV and development of dengue disease-like symptoms, such as high viremia, vascular leakage, elevated pro-inflammatory cytokines, low platelets, high hematocrit, and death (Johnson and Roehrig, 1999; Chan et al., 2015). In the primary dengue AG129 infection model, mice were challenged with a lethal dose of mouse-adapted DENV-2 strain S221 and subsequently treated with AQCH. However, in the secondary dengue infection model, AG129 mice were inoculated with in vitro made IC of sub-lethal dose of DENV-2 S221 and neutralizing concentration of cross-neutralizing monoclonal antibody, 4G2. 4G2 essentially binds to the fusion-loop of all four DENV serotypes and tends to enhance dengue virus infection. A sub-lethal dose of DENV-2 S221 does not cause any mortality in mice; however, it becomes lethal when complexed with 4G2 mAb due to ADE and mimicking a lethal secondary dengue infection.
In both primary and secondary dengue AG129 infection models, two doses of AQCH (100 and 33 mg/kg/day) were evaluated in each of the three dosing regimens (BID, TID, and QID), where the dose-dependent protection was observed with 100 mg/kg/day being more efficacious than 33 mg/kg/day in all the three dosing regimens in both the models (Figures 4, 5). Evaluation of different dosing regimens for any new anti-dengue drug becomes crucial as the potency can be enhanced with increased dosing frequency. This was well illustrated by Celgosivir, which was found to be less effective at 100 mg/kg/day single dose than at 50, 25, or even 10 mg/kg/day given BID in AG129 mice (Watanabe et al., 2012). In this study also, increased dosing frequency yielded significantly higher survival efficacies. The effect of the dosing regimen was less pronounced in primary AG129 mice (Figure 4); however, it became more discernible in the secondary dengue infection model, where QID feeding of 100 mg/kg/day resulted in the survival of 100% of animals, whereas the BID feeding resulted in the survival of none (Figure 5). Hence, all further in vivo evaluations of AQCH were performed at the most protective dose and a dosing regimen of 25 mg/kg/dose QID (equivalent to 100 mg/kg/day).
The AG129 mice exhibit enhanced serum viremia and acute small intestinal pathologies during secondary DENV infection (Watanabe et al., 2015). Thus, we examined the impact of AQCH drug substance treatment in the secondary dengue AG129 model on serum viremia as well as markers of small intestinal pathology, viz., viral load, vascular leakage, and TNF-α and IL-6 levels. It was observed that AQCH treatment reduced both the serum and small intestinal viral loads. However, unlike small intestinal viremia, the serum viral load reduction was not dose-dependent (Figure 6). AQCH treatment at 8.25 mg/kg/dose QID (33 mg/kg/day) led to a higher reduction of serum viremia than at 100 mg/kg/day, which does not correlate with survival data (Figure 5B). This indicates that the serum viremia levels are not truly correlative of protection in coherence with Watanabe’s observations (Watanabe et al., 2015). Like viremia, cytokine expression levels and extent of vascular leakage in the small intestine too were dose-dependent with AQCH at 8.25 mg/kg/dose QID (33 mg/kg/day), offering lower reduction than 100 mg/kg/day dose (Figure 7). This corroborated well with the lower survival efficacy of secondary dengue AG129 mice upon treatment with 33 mg/kg/day (Figure 5B). These observations indicate that small intestinal pathology indeed correlates well with the protection efficacy of an anti-dengue drug.
Demonstration of potent anti-dengue activity by AQCH in in vitro and in vivo analyses lays the ground for its clinical development. We chose a batch of AQCH extract, FCH1901002, and formulated it into tablets of various strengths (100, 300, and 500 mg), which were found to be stable for more than 6 months upon storage (Table 1). This was also evidenced in in vivo protective efficacy and showed similar protection as AQCH at 25 mg/kg/dose QID (data not shown here). This supports the case for the potential preclinical development of AQCH to proceed further for its clinical development to combat dengue fever.
In conclusion, this is the first study reporting an aqueous extract of the stem of C. hirsutus to possess significant pan-anti-dengue activity; the extraction process is robust and consistent, making this plant industrially viable for further clinical development. At this time, when there is no approved anti-dengue drug available, this phytopharmaceutical formulation can be a breakthrough in providing an efficacious and economical remedy to combat dengue, especially in resource-poor nations.
This work provides the first evidence of the pan-anti-dengue potential of C. hirsutus-based phytopharmaceutical as determined through extensive in vitro and in vivo experiments. We have also characterized five chemical entities in the AQCH phytopharmaceutical substance, which provides means for the standardization of phytopharmaceutical drug substances and phytopharmaceutical products. Based on these findings, a program to develop a safe and effective C. hirsutus-derived phytopharmaceutical product for the treatment of dengue has been initiated.
Although both the in vitro and in vivo models of dengue virus infections used in the current study have been extensively deployed for the development of a potential preclinical AQCH phytopharmaceutical against dengue virus infections, these models may not reflect the true efficacy of AQCH phytopharmaceutical in dengue-infected humans. In the absence of an ideal model system, additional studies need to be performed to establish the anti-dengue potential of AQCH for the treatment of dengue infections in humans.
The raw data supporting the conclusions of this article will be made available by the authors, without undue reservation.
The animal study was reviewed and approved by the Institutional Animal Ethics Committee (IAEC) of International Centre for Genetic Engineering and Biotechnology, New Delhi (ICGEB/IAEC/08/2016/RGP-15) in compliance with the “Committee for the Purpose of Control and Supervision of Experiments on Animals (CPCSEA)” guidelines issued by the Government of India.
NK, UA, and AAL: study conceptualization. SG and PK: plant identification. KN: plant extract preparation. RS and HB: animal experiments. AP, RKR, HB, and RS: virus inhibition assays. RS, AP, and RKR: virus culture and titration. RA: serum and tissue viral estimation. RKR: MTT assay. RKR, TJ, RP, HM, and SM: tablet formulation and stability studies. DS, VS, PG, APG, YSB, and RAV: chemical fingerprinting and marker compound isolation. RS, RKR, AP, RA, HB, RKS, KN, DS, VS, YSB, RAV, TJ, BV, AAL, UA, and NK: analysis and interpretation of data. UA, RKR, RS, and NK: drafting of the manuscript. RS, RKR, AP, RA, HB, RKS, SC, KN, DS, VS, PG, APG, SG, PK, YSB, TJ, BV, RP, HM, SM, RK, RSo, RAV, DSR, AAL, UA, and NK: approval of final manuscript. All authors contributed to the article and approved the submitted version.
This study is part of a collaborative project between Sun Pharmaceutical Industries Ltd., International Centre for Genetic Engineering and Biotechnology, New Delhi, and CSIR-Indian Institute of Integrative Medicine, Jammu. Sun Pharmaceutical Industries Ltd., provided funding support to ICGEB (NK) and IIIM (RAV). NK also received funding support from ICGEB, India.
This study received funding from Sun Pharmaceutical Industries Limited, which was provided for the conduct of work to IIIM, Jammu, and ICGEB, New Delhi. The investigators from the funder had the following involvement with the study–(1) Design, conduct, analysis, and interpretation: UA, RKR, RA, HB, RSo, and AAL; (2) preparation and characterization of the drug substance and drug product: KN, TJ, BV, RP, HM, SM, and RK. RKR, RA, HB, KN, TJ, BV, RP, HM, SM, RK, RS, AAL, and UA were employed by company Sun Pharmaceutical Industries Limited.
The remaining authors declare that the research was conducted in the absence of any commercial or financial relationships that could be construed as a potential conflict of interest.
All claims expressed in this article are solely those of the authors and do not necessarily represent those of their affiliated organizations, or those of the publisher, the editors and the reviewers. Any product that may be evaluated in this article, or claim that may be made by its manufacturer, is not guaranteed or endorsed by the publisher.
We are thankful to Dr. Mohan Prasad, Dr. Azadar Khan, Mr. Narendra Lakkad, Dr. Romi Singh, Dr. Atul Raut, Mr. Gaurav Sahal, Mr. Rakesh Sinha, Mr. Kohinoor Das from Sun Pharmaceutical Industries Ltd., Dr. Dinakar Salunke from International Centre for Genetic Engineering and Biotechnology, New Delhi, and Mohammad Aslam from Department of Biotechnology, Government of India for their support during the entire study period.
The Supplementary Material for this article can be found online at: https://www.frontiersin.org/articles/10.3389/fmicb.2021.746110/full#supplementary-material
Aguiar, M., Stollenwerk, N., and Halstead, S. B. (2016). The risks behind Dengvaxia recommendation. Lancet Infect. Dis. 16, 882–883. doi: 10.1016/S1473-3099(16)30168-2
Barbosa-Filho, J. M., Da-Cunha, E. V. L., and Gray, A. I. (2000). “Alkaloids of the menispermaceae,” in The Alkaloids: Chemistry and Biology, Vol. 54, ed. G. A. Cordell (Cambridge, MA: Academic Press), 1–190.
Beesetti, H., Khanna, N., and Swaminathan, S. (2016). Investigational drugs in early development for treating dengue infection. Expert Opin. Investig. Drugs 25, 1059–1069. doi: 10.1080/13543784.2016.1201063
Bhatt, S., Gething, P. W., Brady, O. J., Messina, J. P., Farlow, A. W., Moyes, C. L., et al. (2013). The global distribution and burden of dengue. Nature 496, 504–507. doi: 10.1038/nature12060
Biswal, S., Borja-Tabora, C., Martinez Vargas, L., Velásquez, H., Theresa Alera, M., Sierra, V., et al. (2020). Efficacy of a tetravalent dengue vaccine in healthy children aged 4-16 years: a randomised, placebo-controlled, phase 3 trial. Lancet (London, England) 395, 1423–1433. doi: 10.1016/S0140-6736(20)30414-1
Botta, L., Rivara, M., Zuliani, V., and Radi, M. (2018). Drug repurposing approaches to fight Dengue virus infection and related diseases. Front. Biosci. 23:997–1019. doi: 10.2741/4630
Brady, O. J., Gething, P. W., Bhatt, S., Messina, J. P., Brownstein, J. S., Hoen, A. G., et al. (2012). Refining the global spatial limits of dengue virus transmission by evidence-based consensus. PLoS Negl. Trop. Dis. 6:e1760. doi: 10.1371/journal.pntd.0001760
Chakravarti, A., Arora, R., and Luxemburger, C. (2012). Fifty years of dengue in India. Trans. R. Soc. Trop. Med. Hyg. 106, 273–282. doi: 10.1016/j.trstmh.2011.12.007
Chan, K. W. K., Watanabe, S., Kavishna, R., Alonso, S., and Vasudevan, S. G. (2015). Animal models for studying dengue pathogenesis and therapy. Antiviral Res. 123, 5–14. doi: 10.1016/j.antiviral.2015.08.013
Deng, S.-Q., Yang, X., Wei, Y., Chen, J.-T., Wang, X.-J., and Peng, H.-J. (2020). A review on dengue vaccine development. Vaccines 8:63. doi: 10.3390/vaccines8010063
Dighe, S. N., Ekwudu, O., Dua, K., Chellappan, D. K., Katavic, P. L., and Collet, T. A. (2019). Recent update on anti-dengue drug discovery. Eur. J. Med. Chem. 176, 431–455.
Ganapaty, S., Dash, G. K., Subburaju, T., and Suresh, P. (2002). Diuretic, laxative and toxicity studies of Cocculus hirsutus aerial parts. Fitoterapia 73, 28–31. doi: 10.1016/s0367-326x(01)00345-8
Gubler, D. J. (2011). Dengue, urbanization and globalization: the unholy trinity of the 21(st) century. Trop. Med. Health 39, 3–11. doi: 10.2149/tmh.2011-S05
Guzman, M. G., Alvarez, M., and Halstead, S. B. (2013). Secondary infection as a risk factor for dengue hemorrhagic fever/dengue shock syndrome: an historical perspective and role of antibody-dependent enhancement of infection. Arch. Virol. 158, 1445–1459. doi: 10.1007/s00705-013-1645-3
Halstead, S. B., Mahalingam, S., Marovich, M. A., Ubol, S., and Mosser, D. M. (2010). Intrinsic antibody-dependent enhancement of microbial infection in macrophages: disease regulation by immune complexes. Lancet Infect. Dis. 10, 712–722. doi: 10.1016/S1473-3099(10)70166-3
Ishii, H., Ishikawa, T., Akaike, M., Tohjoh, T., Toyoki, M., Ishikawa, M., et al. (1984). [Studies on the chemical constituents of rutaceous plants. LIX. The chemical constituents of Xanthoxylum nitidum (Roxb.) D.C. (Fagara nitida Roxb.) (1) examination of the alkaloidal fraction of the bark]. Yakugaku Zasshi 104, 1030–1042. doi: 10.1248/yakushi1947.104.10_1030
Itokawa, H., Tsuruoka, S., Takeya, K., Mori, N., Sonobe, T., Kosemura, S., et al. (1987). An antitumor morphinane alkaloid, sinococuline, from Cocculus trilobus. Chem. Pharm. Bull. (Tokyo) 35, 1660–1662. doi: 10.1248/cpb.35.1660
Jain, M., Ganju, L., Katiyal, A., Padwad, Y., Mishra, K. P., Chanda, S., et al. (2008). Effect of Hippophae rhamnoides leaf extract against Dengue virus infection in human blood-derived macrophages. Phytomedicine 15, 793–799. doi: 10.1016/j.phymed.2008.04.017
Johnson, A. J., and Roehrig, J. T. (1999). New mouse model for dengue virus vaccine testing. J. Virol. 73, 783–786. doi: 10.1128/JVI.73.1.783-786.1999
Kasture, P. N., Nagabhushan, K. H., and Kumar, A. (2016). A multi-centric, double-blind, placebo-controlled, randomized, prospective study to evaluate the efficacy and safety of carica papaya leaf extract, as empirical therapy for thrombocytopenia associated with dengue fever. J. Assoc. Phys. India 64, 15–20.
Kraus, A. A., Messer, W., Haymore, L. B., and de Silva, A. M. (2007). Comparison of plaque- and flow cytometry-based methods for measuring dengue virus neutralization. J. Clin. Microbiol. 45, 3777–3780. doi: 10.1128/JCM.00827-07
Lambeth, C. R., White, L. J., Johnston, R. E., and de Silva, A. M. (2005). Flow cytometry-based assay for titrating dengue virus. J. Clin. Microbiol. 43, 3267–3272. doi: 10.1128/JCM.43.7.3267-3272.2005
Li, F.-S., and Weng, J.-K. (2017). Demystifying traditional herbal medicine with modern approach. Nat. Plants 3:17109. doi: 10.1038/nplants.2017.109
Lim, S. P. (2019). Dengue drug discovery: progress, challenges and outlook. Antiviral Res. 163, 156–178. doi: 10.1016/j.antiviral.2018.12.016
Low, J. G. H., Ooi, E. E., and Vasudevan, S. G. (2017). Current status of dengue therapeutics research and development. J. Infect. Dis. 215, S96–S102. doi: 10.1093/infdis/jiw423
Low, J. G., Sung, C., Wijaya, L., Wei, Y., Rathore, A. P. S., Watanabe, S., et al. (2014). Efficacy and safety of celgosivir in patients with dengue fever (CELADEN): a phase 1b, randomised, double-blind, placebo-controlled, proof-of-concept trial. Lancet Infect. Dis. 14, 706–715. doi: 10.1016/S1473-3099(14)70730-3
Marya, B. H., and Bothara, S. B. (2011). Ethnopharmacological properties of Cocculus hirsutus (L.) diels- a review. Int. J. Pharm. Sci. Rev. Res. 7:Article–022.
Muller, D. A., Depelsenaire, A. C. I., and Young, P. R. (2017). Clinical and laboratory diagnosis of dengue virus infection. J. Infect. Dis. 215, S89–S95. doi: 10.1093/infdis/jiw649
Murhekar, M. V., Kamaraj, P., Kumar, M. S., Khan, S. A., Allam, R. R., Barde, P., et al. (2019). Burden of dengue infection in India, 2017: a cross-sectional population based serosurvey. Lancet Glob. Health 7, e1065–e1073. doi: 10.1016/S2214-109X(19)30250-5
Nakasone, Y., Takara, K., Wada, K., Tanaka, J., Yogi, S., and Nakatani, N. (1996). Antioxidative compounds isolated from kokuto, non-centrifugal cane sugar. Biosci. Biotechnol. Biochem. 60, 1714–1716. doi: 10.1271/bbb.60.1714
Nguyen, N. M., Tran, C. N. B., Phung, L. K., Duong, K. T. H., Huynh Hle, A., Farrar, J., et al. (2013). A randomized, double-blind placebo controlled trial of balapiravir, a polymerase inhibitor, in adult dengue patients. J. Infect. Dis. 207, 1442–1450. doi: 10.1093/infdis/jis470
Parida, M. M., Upadhyay, C., Pandya, G., and Jana, A. M. (2002). Inhibitory potential of neem (Azadirachta indica Juss) leaves on dengue virus type-2 replication. J. Ethnopharmacol. 79, 273–278. doi: 10.1016/s0378-8741(01)00395-6
Perkel, J. M. (2016). NIH dengue vaccine leaps into phase 3 studies. Nat. Biotechnol. 34:449. doi: 10.1038/nbt0516-449
Ramasamy, V., Arora, U., Shukla, R., Poddar, A., Shanmugam, R. K., White, L. J., et al. (2018). A tetravalent virus-like particle vaccine designed to display domain III of dengue envelope proteins induces multi-serotype neutralizing antibodies in mice and macaques which confer protection against antibody dependent enhancement in AG129 mice. PLoS Negl. Trop. Dis. 12:e0006191. doi: 10.1371/journal.pntd.0006191
Sahoo, N., Choudhury, K., and Manchikanti, P. (2009). Manufacturing of biodrugs: need for harmonization in regulatory standards. BioDrugs 23, 217–229. doi: 10.2165/11317110-000000000-00000
Sarathy, V. V., White, M., Li, L., Gorder, S. R., Pyles, R. B., Campbell, G. A., et al. (2015). A lethal murine infection model for dengue virus 3 in AG129 mice deficient in type I and II interferon receptors leads to systemic disease. J. Virol. 89, 1254–1266. doi: 10.1128/JVI.01320-14
Shepard, D. S., Halasa, Y. A., Tyagi, B. K., Adhish, S. V., Nandan, D., Karthiga, K. S., et al. (2014). Economic and disease burden of dengue illness in India. Am. J. Trop. Med. Hygiene 91, 1235–1242. doi: 10.4269/ajtmh.14-0002
Shepard, D. S., Undurraga, E. A., Halasa, Y. A., and Stanaway, J. D. (2016). The global economic burden of dengue: a systematic analysis. Lancet Infect. Dis. 16, 935–941. doi: 10.1016/S1473-3099(16)00146-8
Shresta, S., Sharar, K. L., Prigozhin, D. M., Beatty, P. R., and Harris, E. (2006). Murine model for dengue virus-induced lethal disease with increased vascular permeability. J. Virol. 80, 10208–10217.
Shukla, R., Rajpoot, R. K., Arora, U., Poddar, A., Swaminathan, S., and Khanna, N. (2018). Pichia pastoris-expressed bivalent virus-like particulate vaccine induces domain III-Focused bivalent neutralizing antibodies without antibody-dependent enhancement in vivo. Front. Microbiol. 8:2644. doi: 10.3389/fmicb.2017.02644
Shukla, R., Shanmugam, R. K., Ramasamy, V., Arora, U., Batra, G., Acklin, J. A., et al. (2020b). Zika virus envelope nanoparticle antibodies protect mice without risk of disease enhancement. EBioMedicine 54:102738. doi: 10.1016/j.ebiom.2020.102738
Shukla, R., Beesetti, H., Brown, J. A., Ahuja, R., Ramasamy, V., Shanmugam, R. K., et al. (2020a). Dengue and Zika virus infections are enhanced by live attenuated dengue vaccine but not by recombinant DSV4 vaccine candidate in mouse models. EBioMedicine 60:102991. doi: 10.1016/j.ebiom.2020.102991
Shukla, R., Ramasamy, V., Shanmugam, R. K., Ahuja, R., and Khanna, N. (2020c). Antibody-dependent enhancement: a challenge for developing a safe dengue vaccine. Front. Cell. Infect. Microbiol. 10:572681. doi: 10.3389/fcimb.2020.572681
Singh, P. K., and Rawat, P. (2017). Evolving herbal formulations in management of dengue fever. J. Ayurveda Integr. Med. 8, 207–210. doi: 10.1016/j.jaim.2017.06.005
Snogan, E., Vahirua-Lechat, I., Ho, R., Bertho, G., Girault, J.-P., Ortiga, S., et al. (2007). Ecdysteroids from the medicinal fern Microsorum scolopendria (Burm. f.). Phytochem. Anal. 18, 441–450. doi: 10.1002/pca.1000
Sood, R., Raut, R., Tyagi, P., Pareek, P. K., Barman, T. K., Singhal, S., et al. (2015). Cissampelos pareira Linn: natural source of potent antiviral activity against all four dengue virus serotypes. PLoS Negl. Trop. Dis. 9:e0004255. doi: 10.1371/journal.pntd.0004255
Stanaway, J. D., Shepard, D. S., Undurraga, E. A., Halasa, Y. A., Coffeng, L. E., Brady, O. J., et al. (2016). The global burden of dengue: an analysis from the Global Burden of Disease Study 2013. Lancet Infect. Dis. 16, 712–723. doi: 10.1016/S1473-3099(16)00026-8
Struchiner, C. J., Rocklöv, J., Wilder-Smith, A., and Massad, E. (2015). Increasing dengue incidence in singapore over the past 40 years: population growth, climate and mobility. PLoS One 10:e0136286. doi: 10.1371/journal.pone.0136286
Taha-Salaime, L., Davidovich-Rikanati, R., Sadeh, A., Abu-Nassar, J., Marzouk-Kheredin, S., Yahyaa, Y., et al. (2019). Phytoecdysteroid and clerodane content in three wild Ajuga species in israel. ACS Omega 4, 2369–2376. doi: 10.1021/acsomega.8b03029
Tam, D. T. H., Ngoc, T. V., Tien, N. T. H., Kieu, N. T. T., Thuy, T. T. T., Thanh, L. T. C., et al. (2012). Effects of short-course oral corticosteroid therapy in early dengue infection in Vietnamese patients: a randomized, placebo-controlled trial. Clin. Infect. Dis. Off. Publ. Infect. Dis. Soc. Am. 55, 1216–1224. doi: 10.1093/cid/cis655
Thomas, S. J., and Yoon, I. K. (2019). A review of Dengvaxia®: development to deployment. Hum. Vaccines Immunother. 15, 2295–2314. doi: 10.1080/21645515.2019.1658503
Tricou, V., Minh, N. N., Van, T. P., Lee, S. J., Farrar, J., Wills, B., et al. (2010). A randomized controlled trial of chloroquine for the treatment of dengue in Vietnamese adults. PLoS Negl. Trop. Dis. 4:e785. doi: 10.1371/journal.pntd.0000785
Vejchapipat, P., Theamboonlers, A., Chongsrisawat, V., and Poovorawan, Y. (2006). An evidence of intestinal mucosal injury in dengue infection. Southeast Asian J. Trop. Med. Public Health 37, 79–82.
Watanabe, S., Chan, K. W. K., Wang, J., Rivino, L., Lok, S.-M., and Vasudevan, S. G. (2015). Dengue virus infection with highly neutralizing levels of cross-reactive antibodies causes acute lethal small intestinal pathology without a high level of viremia in mice. J. Virol. 89, 5847–5861. doi: 10.1128/jvi.00216-15
Watanabe, S., Low, J. G.-H., and Vasudevan, S. G. (2018). Preclinical antiviral testing for dengue virus infection in mouse models and its association with clinical studies. ACS Infect. Dis. 4, 1048–1057. doi: 10.1021/acsinfecdis.8b00054
Watanabe, S., Rathore, A. P. S., Sung, C., Lu, F., Khoo, Y. M., Connolly, J., et al. (2012). Dose- and schedule-dependent protective efficacy of celgosivir in a lethal mouse model for dengue virus infection informs dosing regimen for a proof of concept clinical trial. Antiviral Res. 96, 32–35. doi: 10.1016/j.antiviral.2012.07.008
Whitehorn, J., Nguyen, C. V. V., Khanh, L. P., Kien, D. T. H., Quyen, N. T. H., Tran, N. T. T., et al. (2016). Lovastatin for the treatment of adult patients with dengue: a randomized, double-blind, placebo-controlled trial. Clin. Infect. Dis. Off. Publ. Infect. Dis. Soc. Am. 62, 468–476. doi: 10.1093/cid/civ949
WHO (2009). Dengue: Guidelines for Diagnosis, Treatment, Prevention and Control: New Edition. Geneva: WHO Guidelines Approved by the Guidelines Review Committee.
Wilder-Smith, A., and Rupali, P. (2019). Estimating the dengue burden in India. Lancet Glob. Heal. 7, e988–e989. doi: 10.1016/S2214-109X(19)30249-9
Keywords: dengue, phytopharmaceutical, Cissampelos pareira, Cocculus hirsutus, AG129 mice, vascular leakage, TNF-α, IL-6
Citation: Shukla R, Rajpoot RK, Poddar A, Ahuja R, Beesetti H, Shanmugam RK, Chaturvedi S, Nayyar K, Singh D, Singamaneni V, Gupta P, Gupta AP, Gairola S, Kumar P, Bedi YS, Jain T, Vashishta B, Patil R, Madan H, Madan S, Kalra R, Sood R, Vishwakarma RA, Reddy DS, Lal AA, Arora U and Khanna N (2021) Cocculus hirsutus-Derived Phytopharmaceutical Drug Has Potent Anti-dengue Activity. Front. Microbiol. 12:746110. doi: 10.3389/fmicb.2021.746110
Received: 23 July 2021; Accepted: 04 October 2021;
Published: 29 November 2021.
Edited by:
Mohammadjavad Paydar, Université de Montréal, CanadaReviewed by:
Kenny Voon, International Medical University, MalaysiaCopyright © 2021 Shukla, Rajpoot, Poddar, Ahuja, Beesetti, Shanmugam, Chaturvedi, Nayyar, Singh, Singamaneni, Gupta, Gupta, Gairola, Kumar, Bedi, Jain, Vashishta, Patil, Madan, Madan, Kalra, Sood, Vishwakarma, Reddy, Lal, Arora and Khanna. This is an open-access article distributed under the terms of the Creative Commons Attribution License (CC BY). The use, distribution or reproduction in other forums is permitted, provided the original author(s) and the copyright owner(s) are credited and that the original publication in this journal is cited, in accordance with accepted academic practice. No use, distribution or reproduction is permitted which does not comply with these terms.
*Correspondence: Navin Khanna, bmF2aW5raGFubmE1QGdtYWlsLmNvbQ==; bmF2aW5AaWNnZWIucmVzLmlu; Upasana Arora, dXBhc2FuYS5hcm9yYUBzdW5waGFybWEuY29t
†These authors have contributed equally to this work
Disclaimer: All claims expressed in this article are solely those of the authors and do not necessarily represent those of their affiliated organizations, or those of the publisher, the editors and the reviewers. Any product that may be evaluated in this article or claim that may be made by its manufacturer is not guaranteed or endorsed by the publisher.
Research integrity at Frontiers
Learn more about the work of our research integrity team to safeguard the quality of each article we publish.