- 1Department of Plant Pathology, University of Florida, Gainesville, FL, United States
- 2Laboratory of Environmental Microbiology, Embrapa Environment, Brazilian Agricultural Research Corporation, Brasília, Brazil
- 3Institute of Ecology and Earth Sciences, Faculty of Science and Technology, University of Tartu, Tartu, Estonia
- 4Food Systems Institute, University of Florida, Gainesville, FL, United States
Drought stress is an alarming constraint to plant growth, development, and productivity worldwide. However, plant-associated bacteria, fungi, and viruses can enhance stress resistance and cope with the negative impacts of drought through the induction of various mechanisms, which involve plant biochemical and physiological changes. These mechanisms include osmotic adjustment, antioxidant enzyme enhancement, modification in phytohormonal levels, biofilm production, increased water and nutrient uptake as well as increased gas exchange and water use efficiency. Production of microbial volatile organic compounds (mVOCs) and induction of stress-responsive genes by microbes also play a crucial role in the acquisition of drought tolerance. This review offers a unique exploration of the role of plant-associated microorganisms—plant growth promoting rhizobacteria and mycorrhizae, viruses, and their interactions—in the plant microbiome (or phytobiome) as a whole and their modes of action that mitigate plant drought stress.
Introduction
Currently more than 700 million people in the world are severely affected by food insecurity according to the Food Security Information Network and Global Network Against Crises (2020), and this rate is likely to increase if the issues causing food insecurity are not addressed. Especially under climate change, drought is one of the major abiotic stresses threatening agricultural production worldwide (Lesk et al., 2016). Drought alone causes higher annual crop yield losses than all plant pathogens combined (Naylor and Coleman-Derr, 2018). In the last 35 years drought stress has reduced yields globally in staple crops such as wheat, maize, and chickpeas by as much as 21, 40, and 60%, respectively (Daryanto et al., 2016; Khan et al., 2016).
Drought affects plant–water potential and turgor, interfering with plant functions and changing plant physiological and morphological traits (Rahdari and Hoseini, 2012). Under water deficit conditions, many enzymes that participate in photosynthesis, such as ribulose-1, 5-bisphosphate carboxylase/oxygenase (Rubisco), phosphoenolpyruvate carboxylase (PEPCase), NADP-malic enzyme (NADP-ME), fructose-1,6-bisphosphatase (FBPase) and pyruvate orthophosphate dikinase (PPDK), have reduced activity (Ashraf and Harris, 2013; Hemantaranjan, 2018). Consequently, drought causes a reduction in the CO2 assimilation rate, malfunctioning of the primary photosynthetic reaction, and disruption of pigments, thus inhibiting photosynthetic activity in plants (Kerry et al., 2018). Other enzymes related to nutrient uptake, translocation and metabolism of nutrients are also affected by drought. One such enzyme is nitrate reductase (NR), resulting in lower uptake of nitrate from the soil (Kapoor et al., 2020). Reduction in the transpiration rate due to a water deficit also decreases plants’ absorption of nutrients and their efficiency of nutrient utilization. Thus, nutrient diffusion and mass flow of water-soluble nutrients such as nitrate, sulfate, Ca, Mg, and Si are decreased (Selvakumar et al., 2012). Additionally, drought induces free radicals that affect antioxidant defenses and reactive oxygen species (ROS), such as superoxide radicals, hydrogen peroxide, and hydroxyl radicals, resulting in oxidative stress. At high concentrations, ROS can cause plant damage, such as initiating lipid peroxidation, membrane deterioration, and degrading proteins, lipids, and nucleic acids (da Silva et al., 2013).
To cope with drought stress, compounds such as sugars, amino acids, polyols, alkaloids, and ions which contribute to photosynthesis and cell osmolarity, as well as delaying leaf senescence and enhancing root growth—are upregulated during stress (Fang and Xiong, 2015). Furthermore, synthesis of xyloglucan, expansin, pectins, lignin, and suberin was shown to increase under drought, which helps to strengthen the cell wall and maintain cell turgor (Le Gall et al., 2015). Tripathi et al. (2016) identified 163 metabolites in roots that significantly change in abundance during water stress.
Water-saving irrigation and the development of drought-tolerant cultivars through conventional plant breeding techniques and genetic engineering have been used to address drought (Eisenstein, 2013). Irrigation, however, is technical and cannot always be adopted by growers, and drought-tolerant cultivars are not always prefered by growers and consumers. Alternatively, plant-associated microbes and viruses can enhance crop productivity under climate change conditions, providing a sustainable approach to stress resistance (Xu et al., 2008; Martins et al., 2014, 2018; Nadeem et al., 2014). While many studies have elucidated how plant selection for a particular microbiome assemblage can be an important strategy for addressing biotic stressors (Mendes et al., 2011; Carríon et al., 2019), studies are just beginning to address the role of microbial communities in abiotic stress alleviation (de Vries et al., 2020). Perturbations to plant physiology caused by drought can impact the composition and metabolic activity of the plant microbiome, with consequences for both host and microbial fitness (Yang et al., 2009; Fuchslueger et al., 2016).
Drought also drives microbial responses, including biofilm formation (Khan and Bano, 2019), osmo protection (production of osmolytes) (Vurukonda et al., 2016) and morphological changes (Naylor et al., 2017; Francisco et al., 2019). Overall, drought impacts soil heterogeneity, limits nutrient mobility and access, and increases soil oxygen, often producing a substantial decrease in microbial biomass (Naylor and Coleman-Derr, 2018; Jansson and Hofmockel, 2020). Monoderm bacterial lineages such as Actinobacteria, Chloroflexi, and Firmicutes are well known to be more tolerant to desiccation due to their thicker cell walls, and their enrichment during periods of drought is driven by plant metabolism (Xu and Coleman-Derr, 2019). Drought-induced plant compounds—including sugar, amino acids, and especially G3P—are used in bacterial metabolism, and act as precursors to peptidoglycan biosynthesis in cell wall formation, enhancing the ability of monoderm taxa to improve host fitness (Xu et al., 2018; Castro et al., 2019). Semiarid environments, such as the caatinga biome, are characterized by uneven distribution of rain throughout the year (Alvares et al., 2013). Recent research has revealed the potential of bacteria associated with caatinga native plants as inoculants for plant growth promotion under drought conditions (Bonatelli et al., 2021). The adaptations of the bacteria in caatinga might involve tolerance to high temperatures, desiccation tolerance genes, pigment production for protection against UV radiation, production of thermostable enzymes, and production of intracellular osmolytes (Kavamura et al., 2013).
Exploring microbiome functions to understand how ecological processes operate during long-term drought systems provides a promising strategy to identify a subset of specific microbial taxa and functions to improve plant drought tolerance. However, whole microbiome community profiling and core microbiome assessments (composition and function), and characterizations of plant root exudates under moisture limitations, will be needed to understand relationships among environmental variables. Identifying the microbiome functions that enable plants to regulate drought stress is an important step for the development of ecological systems that will be sustainable in our future world climate. The most studied plant associated organisms are plant-growth promoting rhizobacteria (PGPR) (Ngumbi and Kloepper, 2016; Martins et al., 2018b; Ullah et al., 2019), mycorrhizal fungi (Quiroga et al., 2017; Zhang et al., 2017; Diagne et al., 2020), and viruses (Xu et al., 2008; Dastogeer et al., 2018). In this review, we focused on these three drought stress metigators (bacteria, fungi, and viruses) and explained the modes of action through which they help plants mitigate drought stress (Figure 1).
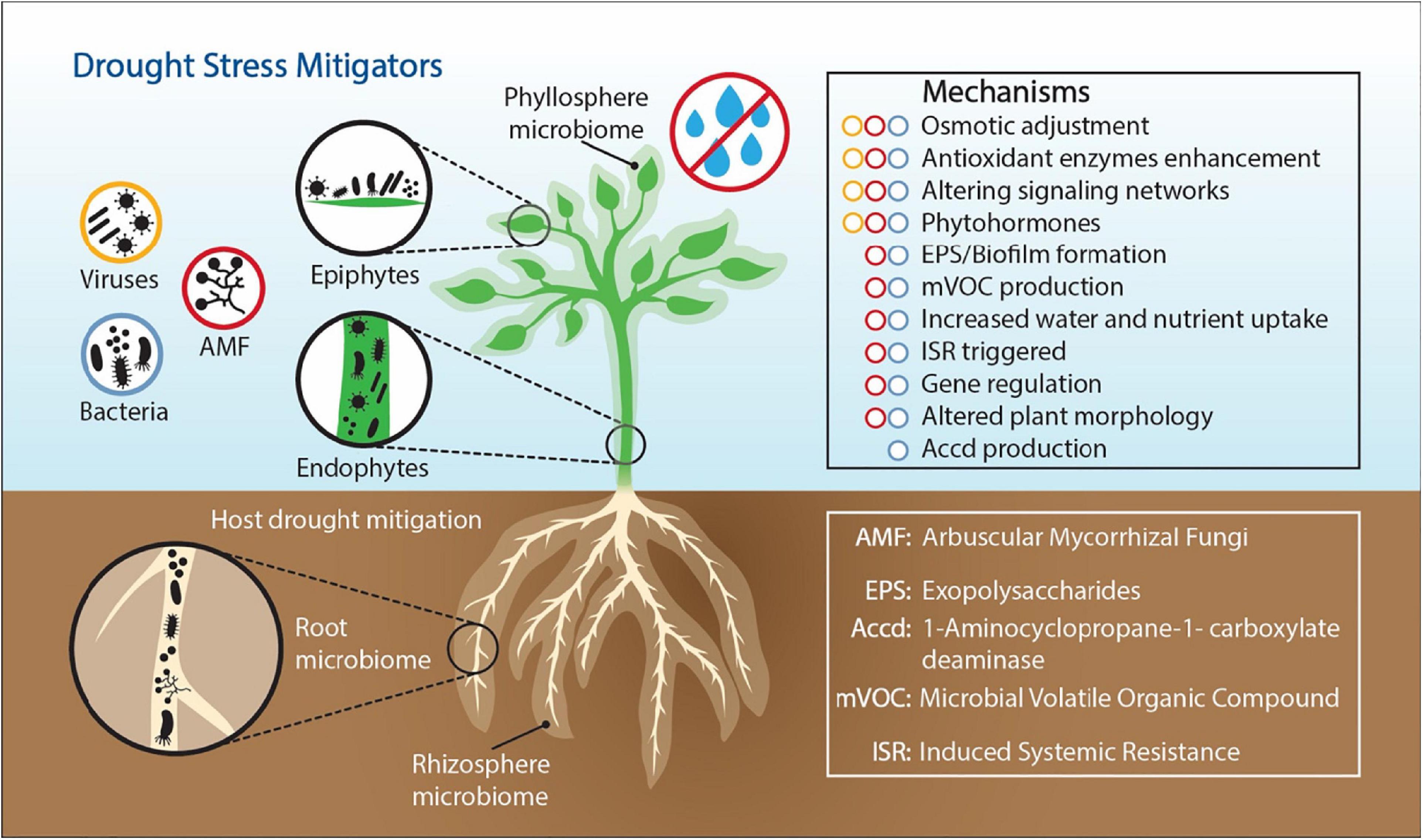
Figure 1. Drought stress alleviating mechanisms employed by plant-associated bacteria, fungi, and viruses. Bacteria, fungi, and viruses are members of the plant microbiome living in different plant compartments, on plant surfaces (epiphytes) and inside plant tissues (endophytes).
Bacteria-Mediated Drought Tolerance
Mutualistic associations between plants and bacterial communities in the soil are very common (Naylor and Coleman-Derr, 2018) and involve rhizobacteria, bacteria present in the rhizosphere (soils in close enough proximity to the root to be influenced by root exudate release), and bacterial endophytes in the root interior (Berg et al., 2014). It has been reported that soil contains around 109–1011 bacterial cells per gram of soil, which often not only exceeds the number of plant host cells but also the number of people existing on Earth (Berg et al., 2016). Plant-associated bacteria (PAB) can also be found aboveground in the phyllosphere, on tissues such as buds, flowers, and leaves, and within plant tissues (endophytes), as well as on pathogen survival structures (e.g., sclerotia), in which case they are termed hyperparasites (Martins et al., 2015). PAB can enhance the tolerance and resistance of plants to biotic and abiotic stresses such as pests, drought, salinity, and pH imbalances (Martins et al., 2013, 2018b; Goswami and Deka, 2020). They can be used as biocontrol agents against plant pathogens and induce production of antimicrobial compounds, such as phytohormones and siderophores, modifying the plant immune response for optimal growth (Kim et al., 2012).
Plant growth-promoting rhizobacteria, commonly known as rhizobacterial drought-tolerance enhancers (Beneduzi et al., 2012; Timmusk et al., 2013), have various mechanisms to cope with the impact of drought on plants as well as on soil. The rhizobacteria-induced drought endurance and resilience (RIDER) process involves several physiological and biochemical changes in the plant. The mechanisms by which RIDER influences plants include: (1) changes in phytohormonal activity; (2) production of aminocyclopropane-1-carboxylate deaminase deaminase (ACCd) to reduce the ethylene level in roots; (3) accumulation of osmolytes that impart drought tolerance in plants; (4) bacterial exopolysaccharide (EPS) production; (5) microbial volatiles organic compounds (mVOCs) production; (6) antioxidant defense (Porcel et al., 2014; Kumar and Verma, 2018); and (7) induction of stress responsive genes by PGPR.
Mechanism of Action of Plant-Growth Promoting Rhizobacteria in Ameliorating Drought Stress in Plants
Change in the Phytohormonal Activity
Phytohormones, such as indole acetic acid (IAA), gibberellins, ethylene, abscisic acid (ABA), and cytokinin, in addition to playing a crucial role in plant growth and development, can help plants cope with abiotic stresses (Cassán et al., 2014; Porcel et al., 2014). PGPR have been reported to promote plant growth under drought conditions by manipulating plant hormones, such as cytokinins (Liu et al., 2013), ABA (Egamberdieva et al., 2017), and IAA (Jochum et al., 2019; Namwongsa et al., 2019), and decreasing ethylene production (Belimov et al., 2015).
Auxin helps ameliorate drought stress in an indirect way, by increasing root growth and/or modification of root architecture and/or root hairs (Contesto et al., 2010; Cassán et al., 2014), which favor water and nutrient uptake from the soil (Egamberdieva et al., 2017). For auxin biosynthesis, tryptophan-dependent pathways are commonly found in microbes. They produce indole-3-acetamide (IAM), indole-3-acetaldoxime (IAQx), tryptamine (TAM), and indole-3-pyruvic acid (IPA) (Su et al., 2011). Numerous reports have supported the role of IAA in triggering drought-responsible signaling pathways upon exposure to drought stress. For example, in the case of Sorghum bicolor, inoculation with three chromium-reducing thermo-tolerant PGPR (e.g., Bacillus cereus TCR17, Providencia rettgeri TCR21, and Myroides odoratimimus TCR22) improved plant growth and physiology by increasing the biosynthesis of IAA, producing plant beneficial metabolites (Bruno et al., 2020). Similarly, tomato inoculation with the PGPR Azospirillum brasilense caused an increase in nitric oxide content, a small diffusible gas acting as a signal molecule in IAA pathway, which caused a root morphology change in tomato (Molina-Favero et al., 2008). Hussain et al. (2014) found evidence of improved growth, biomass, and drought tolerance index in wheat seedlings inoculated with consortia of Rhizobium leguminosarum (LR-30), Mesorhizobium ciceri (CR-30 and CR-39), and Rhizobium phaseoli (MR-2). These modifications were correlated with enhanced auxin synthesis in the rhizobacteria-treated plants. Raheem et al. (2018) demonstrated the production of the auxins indole-3-acetic acid (IAA), indole-3-lactic acid (ILA), and indole-3-carboxylic acid (ICA) in wheat plants inoculated with Bacillus amyloliquefaciens S-134. These auxins triggered root growth in wheat and, thus, helped plants cope with water stress conditions. Likewise, inoculation of wheat seedlings with Azospirillum helped them cope with osmotic stress due to morphological modifications in coleoptile xylem architecture. These morphological modifications were associated with an upregulation of the indole-3-pyruvate decarboxylase gene, which enhanced the IAA synthesis in Azospirillum (Pereyra et al., 2012). A summary of phytohormonal activity associated with water stress tolerance when treated with PGPR is presented in Table 1.
Under drought stress conditions, ABA modifies root architecture to enhance water and nutrient acquisition (Sah et al., 2016; Egamberdieva et al., 2017). It also sustains the hydraulic conductivity of plant roots and shoots to better exploit soil moisture and maintain cell turgor potential. Consequently, it leads to better drought tolerance through upregulation of antioxidant activities and the accumulation of compatible osmolytes, which further maintain the relative water content (RWC).
Bresson et al. (2013) reported that Phyllobacterium brassicacearum strain STM196, isolated from the rhizosphere of Brassica napus, enhanced osmotic stress tolerance in inoculated Arabidopsis plants by elevating ABA content, leading to decreased leaf transpiration. Guajardo et al. (2016) demonstrated that ABA treatment increased antioxidant enzyme activities, which lessened the oxidative stress-induced damage and subsequently improved drought tolerance.
Aminocyclopropane-1-Carboxylate Deaminase Production
Among the many PGPR products that confer plant drought tolerance, the most studied is the enzyme 1-aminocyclopropane-1-carboxylate deaminase (ACCd) (Danish and Zafar-ul-Hye, 2019; Zafar-ul-Hye et al., 2019; Danish et al., 2020). Under limited water supply, plants increase their production of stress generating ethylene (ET), which is inhibitory for plant growth (e.g., hindering seed germination and root development). Severe drought stress stimulates “1-aminocyclopropane-1-carboxylic acid” (ACC), an ET precursor that increases ethylene accumulation in plants (Gamalero and Glick, 2015). Interestingly, certain PGPR strains possess enzyme ACC deaminase (Glick et al., 2007), which can cleave the plant ethylene precursor ACC to ammonia and α-ketobutyrate, instead of converting it to ET. Therefore, ACC deaminase reduces the formation of endogenous ethylene and promotes plant growth (Chandra et al., 2019). Saleem et al. (2018) found that inoculation with ACCd producing Enterobacter sp. and Bacillus sp. increased drought tolerance in velvet bean (Mucuna pruriens). Niu et al. (2018) suggested that ACC deaminase and EPS-producing bacteria associated with foxtail millet (Setaria italica) could alleviate drought stress in plants, as indicated by improved seed germination and seedling growth. Maize inoculation with Enterobacter cloacae and timber waste biochar (TWBC) was found to be effective in increasing yield under drought stress, due to the potential of higher ACC deaminase synthesis, better nutrient solubilization and higher IAA production (Danish et al., 2020). Likewise, B. amyloliquefaciens and Agrobacterium fabrum promoted considerably higher yields in wheat when the plants were submitted to drought, whether inoculated individually or together. These bacteria produce ACC deaminase that catabolizes the ethylene produced in response to drought stress (Zafar-ul-Hye et al., 2019). Furthermore, the co-inoculation in Cicer arietinum varieties with ACC deaminase producing Bacillus isolate 23-B and Pseudomonas 6-P with M. ciceri improved germination, root length, shoot length, and fresh weight of chickpea seedlings under osmotic potential up to 0.4 MPa (Sharma et al., 2013).
Osmotic Adjustment
Plants increase the production of osmotically active molecules/ions, such as soluble sugars, proline, glycine, trehalose, betaine, organic acids, calcium, potassium, and chloride ions, as an acclimatization response to water deficit conditions (Huang et al., 2014). Proline, one of the most important osmolytes that accumulates in plants under drought stress, results in osmotic adjustment, free radical scavenging, and stabilization of subcellular structures in plant cells to overcome the detrimental effects of drought (Ngumbi and Kloepper, 2016). Some root zone bacteria release proline, which helps plants increase drought tolerance. For example, cucumber plants treated with a mixture of three PGPR strains (Bacillus cereus AR156, Bacillus subtilis SM21, and Serratia sp. XY21) increased leaf proline content three to four-fold, which protected the cucumber plants from over-dehydration (Wang et al., 2012). Proline content was higher under water stress when chickpea varieties were co-inoculated with Bacillus isolate 23-B and M. ciceri (Sharma et al., 2013). Similarly, increased plant drought tolerance was observed in tomato plants treated with phosphate solubilizing bacteria (PSB) Bacillus polymyxa, due to an increase in proline secretion (Shintu and Jayaram, 2015). Drought tolerance due to elevated proline content has been reported across several crops, such as maize (Naseem and Bano, 2014), sorghum (Grover et al., 2014), potato plants (Gururani et al., 2013), and mung bean (Sarma and Saikia, 2014), as well as Arabidopsis (Cohen et al., 2015). An accumulation of choline and glycine betaine (GB) due to the presence of PGPR strains Klebsiella variicola F2 (KJ465989), Raoultella planticola YL2 (KJ465991), and Pseudomonas fluorescens YX2 (KJ465990) also resulted in improved plant growth under drought stress (Gou et al., 2015).
Another plant drought stress tolerance mechanism provided by bacteria is the accumulation of soluble sugars due to starch hydrolysis as osmolytes. Bano et al. (2013) showed that maize seedlings inoculated with Azospirillum lipoferum had increased soluble sugar content and free amino acids, indicating starch hydrolysis, subsequently providing sugar for osmotic adjustment against drought. Trehalose, a xero protectant produced by bacteria under very dry conditions, works as an osmo protectant by stabilizing dehydrated enzymes and membranes (Yang et al., 2009). It also functions as a signaling molecule protecting the integrity of the cell membrane through the expression of the trehalose-6-phosphate synthase gene (Vílchez et al., 2016). A summary of the PGPR induced osmolytic activity in conferring drought tolerance is presented in Table 2.
Exopolysaccharides as Drought Stress Mitigators
Exopolysaccharide, components of bacterial biofilms, provide protection against desiccation during drought stress by enhancing water retention (which may exceed 70 g water per gram of polysaccharide) and by regulating organic carbon sources diffusion (Flemming and Wingender, 2010; Chandra et al., 2021). PGPR form hydrophilic biofilm around plant roots that functions as an additional sheath to protect the roots from desiccation as well as amending the soil structure and its aggregation properties (Rolli et al., 2015). EPS released into soil as capsular and slime materials act as protective capsules around soil aggregates due to the formation of cation bridges, hydrogen bonding, Van der Waals forces, and anion adsorption mechanisms. For instance, seed treatment with B. amyloliquefaciens ALB629, known to produce biofilm and 5-fold more EPS compared to its absence, led to drought stress protection in common bean seedlings (Martins et al., 2018a). Additionally, maize seeds treated with EPS-producing bacterial strains Proteus penneri, Pseudomonas aeruginosa, and Alcaligenes faecalis, along with their respective EPS, improved soil moisture content, plant biomass, root and shoot length, and leaf area (Naseem and Bano, 2014). Yadav et al. (2018), after treating okra with rhizobia, found drought mitigation due to improved soil aggregation in the rhizosphere via sufficient EPS production. Likewise, sunflower plants treated with EPS-producing Rhizobium had an increase in water and nitrogen uptake, attributed to the increased root-adhering soil (RAS)/root tissue (RT) ratio and macroporosity (Vurukonda et al., 2016).
Role of Microbial Volatile Organic Compounds in Inducing Drought Tolerance
Microbial volatile organic compounds in bacteria are generally produced as metabolic end products of anaerobic fermentation processes and extracellular degradation of complex organic molecules (Choudhary et al., 2017). PGPR that produce mVOCs have been shown to increase seedling emergence, root branching, photosynthesis, iron uptake, plant growth, crop yield, plant disease control (Martins et al., 2019), plant parasite control (Terra et al., 2018), as well as drought tolerance. These stress-induced volatiles play a crucial role in developing priming and systemic responses in the plant producing the volatiles and in neighboring plants, making the measurement of volatiles a promising rapid non-invasive technique to assess crop drought stress and its mitigation during stress development (Niinemets, 2010; Timmusk et al., 2014).
Bacterial mVOCs mediated drought tolerance was reported by Asari et al. (2016) using Arabidopsis plants in which B. amyloliquefaciens and B. subtilis produced the mVOCs 3-hydroxy-2-butanone (acetoin) and 2R-, 3R-butanediol. These mVOCs increased growth in Arabidopsis by monopolizing gene expression involved in the maintenance of cell wall structure and influencing numerous phytohormone-signaling pathways that include ethylene, jasmonates, and salicylic acid. Timmusk et al. (2014) showed that priming treatment with Bacillus thuringiensis AZP2 in wheat seedlings resulted in enhanced plant biomass and five-fold higher plant survival under severe drought due to emissions of stress-related volatiles. Application of 2R, 3R-butanediol, a volatile metabolite produced by Pseudomonas chlororaphis O6, stimulated plant survival under drought stress by influencing stomatal aperture closure as well as inducing nitric oxide (NO) signaling in Arabidopsis (Cho et al., 2013). Additionally, certain bacterial mVOCs are involved in biofilm formation, which contains exopolysaccharides as major constituents, and these polysaccharides maintain soil moisture content and increase drought tolerance in plants (Naseem and Bano, 2014).
Antioxidant Metabolism
Water deficit conditions induce the generation of ROS in plants, including superoxide anion radicals (O2–), hydrogen peroxide (H2O2), hydroxyl radicals (OH), singlet oxygen (O12), and alkoxy radicals (RO). These ROS react with proteins, lipids, and deoxyribonucleic acid, causing oxidative damage and impairing the normal redox regulatory state of plant cells (Laxa et al., 2019). In such conditions, plants develop antioxidant defense systems including both enzymatic and non-enzymatic components that work to prevent ROS accumulation and protect plants from oxidative damage occurring during drought stress. Enzymatic components include catalase (CAT), superoxide dismutase (SOD), ascorbate peroxidase (APX), and glutathione reductase (GR), while non-enzymatic components contain glutathione, cysteine, and ascorbic acid (Kaushal and Wani, 2016). The presence of beneficial bacteria in soil can induce drought tolerance by modulating the antioxidant system. Experiments investigating bacterial-mediated tolerance have measured activities of antioxidant enzymes to assess the involvement of the scavenging system during drought stress. Specifically, these studies have investigated if the treatment of plants with PGPR led to a higher antioxidant enzyme activity. Moreno-Galván et al. (2020a) for instance, showed a drought tolerance in Guinea grass (Megathyrsus maximus) due to reduced GR activity. Likewise, the Bacillus–maize interactions that led to an antioxidant response to bacterial inoculation under drought stress are dependent on a decrease in APX and GR activities (Moreno-Galván et al., 2020b). Armada et al. (2016) determined that inoculation of autochthonous PGPR B. thuringiensis in Lavandula dentata and Salvia officinalis promoted growth and drought avoidance by depressing stomatal conductance as well as decreasing GR and APX activity. Similarly, Gururani et al. (2013) observed a 1.8-fold increase in levels of ROS-scavenging enzymes, such as CAT, APX, and SOD, in potato plants inoculated with Bacillus pumilus and Bacillus firmus. Drought tolerance driven by increased levels of CAT has also been observed in other plants, including cucumber, maize, and wheat (Sandhya et al., 2010; Wang et al., 2012). These studies provide evidence for a beneficial effect of PGPR application in conferring drought tolerance to plants by altering the antioxidant activity under drought stress conditions. A summary of antioxidant activity associated with water stress tolerance when treated with PGPR is presented in Table 3.
Induction of Stress Responsive Genes by Plant-Growth Promoting Rhizobacteria
Stress tolerance can be enhanced by treating plants with several PGPR strains which up-regulate stress tolerance inducing genes. Vaishnav and Choudhary (2019) showed that Pseudomonas simiae AU promotes growth and protects soybean plants from drought stress damage, chiefly manifested as changes in the gene expression profiles of transcription factors (DREB/EREB), osmo protectants (P5CS, GOLS), and water transporters (PIP, TIP). Arabidopsis treated with P. polymyxa CR1 induced upregulation of two drought-responsive genes (DRGs), Response to Desiccation RD29A and RD29B (Liu et al., 2020). Additionally, Ghosh et al. (2017) found up-regulation in proline biosynthesis genes in Arabidopsis thaliana when inoculated with Pseudomonas putida GAP-P45 e.g., ornithine-Δ-aminotransferase (OAT), Δ1-pyrroline-5-carboxylate synthetase1 (P5CS1), Δ1-pyrroline-5-carboxylate reductase (P5CR), as well as proline catabolism, e.g., proline dehydrogenase1 (PDH1) and Δ1-pyrroline-5-carboxylate dehydrogenase (P5CDH). These changes in proline metabolic gene expression profile were positively correlated with morpho-physiological evidence of water-stress mitigation in those plants brought about by GAP-P45 inoculation under osmotic stress conditions. Likewise, the gene expression profile of pepper plants inoculated with Bacillus licheniformis K11 under drought stress showed higher levels of dehydrin-like protein (Cadhn), vacuolar ATPase (VA), plant small heat shock proteins (sHSP), and pathogenesis-related proteins (CaPR-10), compared to drought stress without inoculation and non-water-treated pepper (Lim and Kim, 2013). A summary of the plant genes associated with water stress response when treated with PGPR is presented in Table 4.
Screening of Drought Tolerant Microbes
Water restrictors or osmotic inducers such as polyethylene glycol (PEG) are commonly used additives in media to induce water stress. PEG is an impermeable, long-chain neutral polymer which is highly soluble in water. It produces stress in cultured cells similar to drought stress observed in cells of intact plants (Bandeppa et al., 2015; Vurukonda et al., 2016; Ali et al., 2017; Karvembu et al., 2021). These compounds reduce the osmotic potential of the culture medium, reduce water absorption and are not metabolized by plants. However, it is necessary to consider the ideal concentrations of the inducers: the ideal concentration is one which is high enough to meet the goals of the experiment and at the same time, low enough that none of the cultured organisms completely stop growing (Placide et al., 2012).
In most of the experiments, trypticase soy broth (TSB) of differing water potential prepared by adding appropriate concentrations of PEG 6000 (the number indicates the approximate molecular weight) is used to screen drought tolerant bacterial isolates (Fitriani Wangsa Putrie et al., 2013; Ali et al., 2014; Niu et al., 2018). Similarly, Martins et al. (2018) tested bacterial growth under low water activity by growing bacterial isolates in NA-broth medium altered with 2.5% (w/v) glycerol. Ansari and Ahmad (2019) determined tolerance of rhizobacteria to water stress by growing cultures supplemented with 30, 45, and 60% PEG 6000. Although the theory of classical biological control methods discusses growing bacteria in nutrient-poor media (Cook and Baker, 1983), to the best of our knowledge no studies have been conducted so far to screen drought-tolerant microbes in nutrient depleted media.
Effect of Drought Tolerant Bacteria on Plant Health and Yield
In addition to helping plants confer drought tolerance by various mechanisms, PGPR is also reported to improve plant health and yield, which will reduce productivity losses in drought-prone areas. Raheem et al. (2018) showed that inoculation of wheat with mixed culture combinations of B. thuringiensis S-26, D-2, B. amyloliquefaciens S-134, B. simplex D-11, or M. pluranimalium S-29, B. simplex D-1, B. muralis D-5, P. stutzeri S-80 resulted in greater numbers of tillers and spikelets. Increased root length, shoot length, dry weight and relative water content were found in mung bean plants (Vigna radiata) treated with P. aeruginosa strain GGRJ21 (Sarma and Saikia, 2014). Likewise, Grover et al. (2014) found that treatment of Sorghum bicolor with Bacillus spp. strains KB122, KB129, KB133, and KB142 resulted in increased shoot length, root dry biomass, relative water content, sugar, chlorophyll, soil moisture content, and proline content, thereby improving sorghum seedling growth and health under drought stress conditions. Higher values of net photosynthesis (Pn), transpiration (E), and gs were observed in grapevine rootstocks K5BB plantlets inoculated with Pseudomonas spp. or Delftia spp. in comparison to uninoculated control plants (Rolli et al., 2015). Naveed et al. (2014) reported that Burkholderia phytofirmans inoculation of maize cultivar Mazurka improved photosynthesis (net-rate of CO2 assimilation under light saturation) (75%), Chl content (22%), and efficiency of PSII (10%) as compared to the control. Similarly, PGPR strains affects plant physiology by increasing RWC, Chl content, Fv/Fm and reducing leaf MDA content, thus enhancing plant growth under stress situations. Wang et al. (2016) reported that cucumber plants inoculated with a consortium of three PGPR strains called BBS (Bacillus cereus AR156, Bacillus subtilis SM21, and Serratia spp. XY21) reduced both MDA content as well as relative electrical conductivity and increased Chl content (Chla, band a+b increased by 25.9, 31.5, and 27.4%, respectively) in leaves over control during drought.
Studies have found that combinations of rhizobia and other PGPR improves plant health and growth under stress conditions. Co-inoculation of common bean (Phaseolus vulgaris) with Rhizobium tropici- CIAT 899, Phaseolus polymyxa-DSM36, and Phaseolus polymyxa-Loutit strains resulted in greater growth compared to inoculation with Rhizobium alone. Furthermore, co-inoculation exhibited greater nodulation (number and biomass) and nitrogen content compared to drought stressed plants inoculated with only Rhizobium (Figueiredo et al., 2008). Hussain et al. (2014) discovered that IAA produced by the consortia of R. leguminosarum (LR-30), M. ciceri (CR-30 and CR-39), and R. phaseoli (MR-2) improved the growth, biomass and drought tolerance index in wheat.
Mycorrhizal Fungi-Mediated Drought Tolerance
Mycorrhizal symbiosis can be defined as the establishment of an intimate, mostly mutualistic, association between mycorrhizal fungi and plant roots (Bonfante and Genre, 2010; Smith and Read, 2010). Among the mycorrhizal fungi, arbuscular mycorrhizal fungi (AMF), belonging to the phylum Glomeromycota, are the most abundant, commonly present in agricultural soils worldwide and thought to colonize the roots of approx. 80% of land plants (Brundrett and Tedersoo, 2018; Chen et al., 2018), including most crops (Martín-Robles et al., 2018). In this symbiosis, the plant and fungus recognize each other through interacting molecular signals (Hause and Schaarschmidt, 2009; Bonfante and Genre, 2015). In particular, strigolactones exuded by plant roots stimulate branching and pre-symbiotic hypha metabolism and are considered one of the most significant components in the establishment of the association (Bonfante and Genre, 2010). This intimate association is thought to be key in the evolution of plants; ancient arbuscular-like mycorrhizal associations have been hypothesized to be responsible for the conquering of land by the first terrestrial plants (Feijen et al., 2018). This evolutionary achievement is directly related to the ability of mycorrhizal fungi to provide plants with mineral nutrients and water, along with increasing plant resistance to abiotic and biotic stresses, allowing plants to survive, develop, and diversify (Brundrett, 2002; Strullu-Derrien et al., 2018).
Central to the mutualistic nutritional role of this symbiosis is the development of arbuscules within the root cortex cells, a specialized intracellular tree-like structure, where nutrient exchange occurs (Bonfante and Genre, 2010; Smith and Read, 2010). While the AMF deliver inorganic phosphorus, nitrogen, and other benefits, the plant provides photosynthesized carbon and a secure habitat for the fungus (Brundrett, 2002; Smith and Read, 2010). One key aspect of this symbiotic nutritional pathway is that plants can reach inaccessible nutrient and water pools, as the AMF extraradical mycelia explore areas beyond the reach of fine roots and plant hairs (Smith and Read, 2010; Ferrol et al., 2019). A direct effect of this enhanced nutrient and water provision is an increase in plant fitness, i.e., growth and reproduction (Smith and Read, 2008). In agricultural terms, this translates into an overall increase of 16% grain yield (Zhang et al., 2019), in line with previous estimations of a 23, 20, and 9.5% increase in yield for tomato, wheat, and potato, respectively (Pellegrino et al., 2015; Hijri, 2016; Ziane et al., 2017). AMF can directly impact plant performance by altering the plant–water relationship, and increasing plant productivity under drought stress (Augé, 2001; Rapparini and Peñuelas, 2014). Consequently, AMF is a promising agricultural tool with the potential to decrease the use of agrochemicals, increase soil functionality, and contribute to sustainable agricultural management (Thirkell et al., 2017; Zhang et al., 2019). Despite this potential and the great amount of research devoted to understanding the diverse and complex mechanisms that regulate the AMF amelioration effects on plant water stress (Rapparini and Peñuelas, 2014; Ma et al., 2020), many key mechanistic aspects are still not understood.
Mechanism of Action of Mycorrhiza in Conferring Drought Tolerance in Plants
To ameliorate the adverse effects of drought stress, AMF can directly influence plant physiology, biochemistry and genetic regulation, enhancing two main adaptive strategies: enhancing plant tolerance to drought (withstanding drought with low water potential) or avoiding drought stress by maintaining plant water status (Augé, 2001; Fang and Xiong, 2015; Ma et al., 2020). In these two strategies, AMF have several mechanisms to mediate plant responses to drought stress: (1) the uptake and transfer of water and nutrients, (2) enhanced osmotic adjustment, (3) protection against oxidative stress, (4) increased gas exchange and water use efficiency, (5) gene regulation, and (6) modification of root morphology and soil. While these roles are not completely independent, we still lack a complete understanding of the interactions among these different mechanisms (Figure 2).
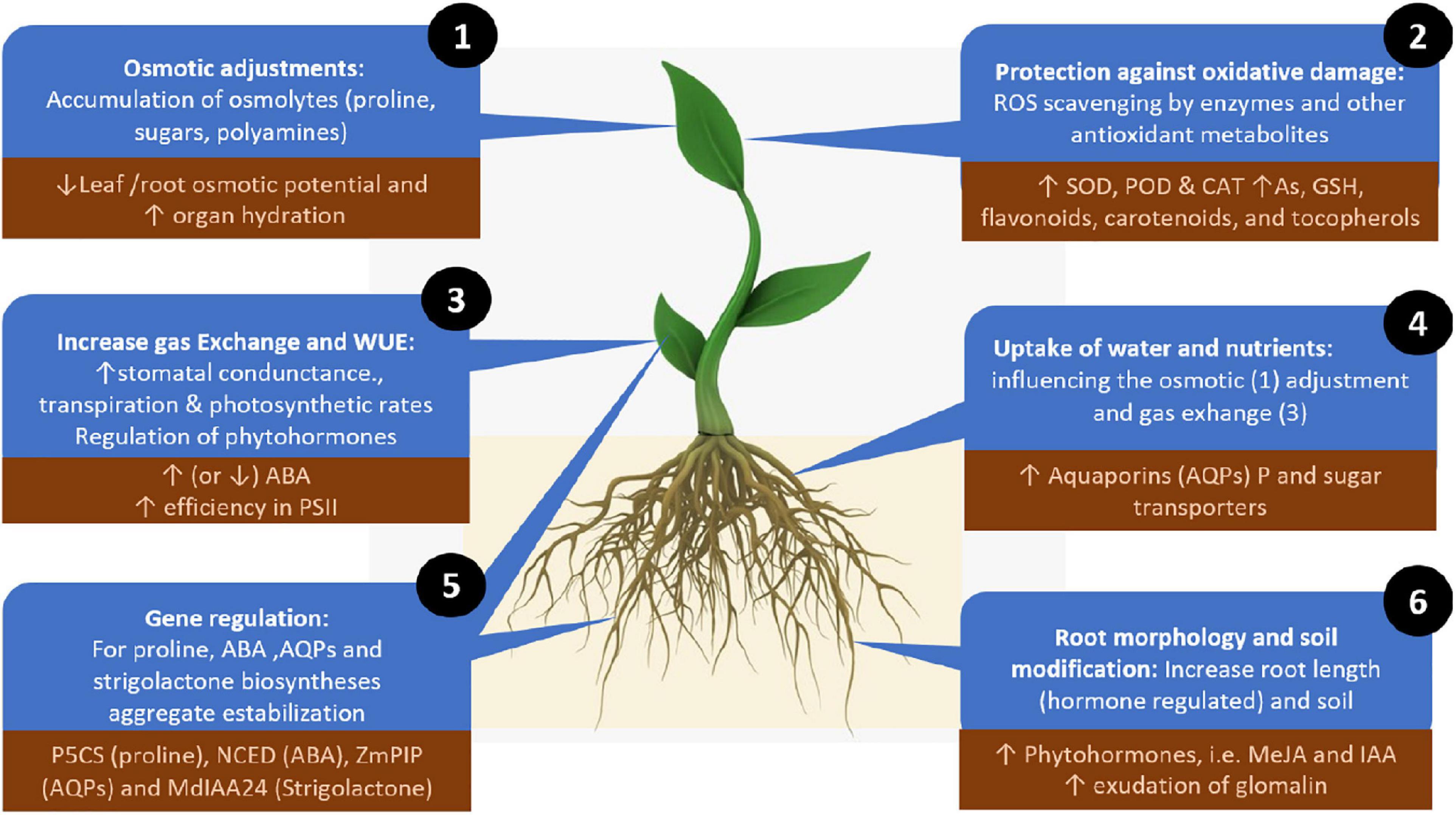
Figure 2. Mechanisms for alleviating drought stress employed by plant-associated arbuscular mycorrhizal fungi. Numbers depict the mechanisms in the order described in the text (order does not indicate relevance). Up or down arrows indicate increases or decreases in the regulator elements involved in each mechanism. The dark brown squared text boxes indicate specific and representative effects, molecules or genes involved in each mechanism. ROS, reactive oxygen species; AQPs, aquaporins; MeJA, methyl jasmonate; IAA, indole acetic acid; SOD, superoxide dismutase; POD, peroxidase; CAT, catalase; As, ascorbate; GSH, glutathione; ABA, abscisic acid; PSII, photosystem II.
Enhanced Osmotic Adjustment
Under drought stress, plants indirectly increase their water potential (decrease stomatal conductance), hampering the water flow from soil to the plant’s roots (Table 5). One direct mechanism is that AMF colonization can improve osmotic balance through the accumulation of inorganic osmolytes like K+, Ca2+, and Mg2+ (Ruiz-Lozano, 2003). However, AMF root colonization also induces biochemical changes that contribute to increased secretion of osmolytes, such as proline, polyamines (PAs), and sugars (Rapparini and Peñuelas, 2014). Each osmolyte can have different mechanisms to enhance plant drought tolerance. For example, PAs, while maintaining cellular pH and ion homeostasis, they can also scavenge ROS, stabilize the plant cell’s lipid bilayer surface and nucleic acids, and even modify stress-responsive gene expression (Talaat and Shawky, 2015; Zhang et al., 2020).
Particularly, proline is known to protect plant tissues against water stress by acting as an osmo solute and hydrophobic protectant. Under drought conditions, AMF induced proline accumulation has been seen in many species including cypress (Cupressus arizonica) (Aalipour et al., 2020), walnut (Juglans regia) (Behrooz et al., 2019), trifoliate orange (Poncirus trifoliata) (Wu et al., 2017), tobacco (Nicotiana tabacum) (Liu et al., 2020), and olive (Olea europaea) (Ouledali et al., 2018). Overall, the accumulation of proline is divergent with the degree of osmotic alterations due to AMF symbiosis and osmoregulation. AM plants accumulated higher levels of proline when exposed to drought stress compared to non-AM plants. However, some studies have also found higher leaf proline concentration in non-AMF added plants than in AMF added plants under water-stress conditions, e.g., Hazzoumi et al. (2015). This has been ascribed to less injury by water deficit, suggesting that AMF might help plants face drought by other means (Rapparini and Peñuelas, 2014). Similarly, carbohydrates have been associated with osmo-protection under drought conditions, particularly non-structural carbohydrates (e.g., sucrose, glucose and fructose) derived from AM enhanced photosynthesis (Augé, 2001). This has been observed, for instance, in trifoliate orange (Wu et al., 2017), macadamia nut (Yooyongwech et al., 2013), lettuce (Baslam and Goicoechea, 2012), and maize (Hu et al., 2020). The regulation of carbohydrates and proline does not seem to be independent: Wu et al. (2017) found that under drought stress leaf concentrations of fructose, glucose, and sucrose were higher, while in proline they were lower. This, along with evidence of stronger regulation of sucrose- and proline-metabolized enzyme activities under drought stress, indicates a potential strong effect enhancing osmotic plant adjustment to drought (Wu et al., 2017).
Protection Against Oxidative Stress
Drought can induce the production of excess ROS necessary the antioxidant defense system, which leads to an imbalance in cellular redox, ultimately damaging cell DNA, lipids, and proteins (Wu et al., 2014b). The antioxidant system includes enzymes (mainly SOD, POD, and CAT) and non-enzymatic molecules [e.g., ascorbate (As), glutathione (GHS), flavonoids, carotenoids, and tocopherols] (Ma et al., 2020). SOD, POD, and CAT are considered the most important members of the active oxygen scavenging enzyme system. Studies have found increased activity of SOD, POD, and CAT enzymes in plants inoculated with AMF under drought conditions (e.g., Essahibi et al., 2018; Liu et al., 2020). While the scavenging ROS effect of non-enzymatic antioxidants has been less studied, some research indicates indirect effects in reducing oxidative stress by AMF activated carotenoids, GHS and α-tocopherol (reviewed by Wu et al., 2014b; Bahadur et al., 2019).
Polyamines are implicated in growth regulation, embryogenesis, cell division, morphogenesis, and enhancing antioxidant defense systems (Altamura et al., 1991; Kumar et al., 1997; Seo et al., 2019; Vuosku et al., 2019). PAs are often composed of cadaverine (Cad), putrescine (Put), spermine (Spm), and spermidine (Spd). Inconsistency in PA accrual patterns has been observed in AM plants in response to water stress. The inoculation of water-stressed trifoliate orange with Funneliformis mosseae (Zhang et al., 2020) resulted in increased Put concentrations, which influenced the ROS-scavenging antioxidant mechanism (Talaat and Shawky, 2013), and decreased in Spd and Spm contents, which may be a nitrogen reserve that can be used to meet plant energy demands soon after recovering from stress (Sharma et al., 2021). In contrast to that, Hu and Chen (2020) reported significant Put content decrease in water stressed AM seedlings. The cumulative pattern of PAs may depend on the type of host plant, the AMF partners, the degree and duration of stress, and, most importantly, the developmental stages and tissues of the plants studied.
Recent studies revealed fatty acid (FA)- and ABA-related metabolites that initiated the protective responses of antioxidant systems. For example, an AMF-induced increase in the content of unsaturated FAs was found to alleviate oxidative damage in trifoliate orange (Wu et al., 2019) and maize (Hu et al., 2020), and AMF-enhanced ABA concentration was also found to stimulate antioxidant systems in black locust (Lou et al., 2021).
Increased Gas Exchange and Water Use Efficiency
Arbuscular mycorrhizal fungi colonization triggers plant physiological responses aboveground, including a generalized enhancement of the rates of gas exchange (stomatal conductance, transpiration, and photosynthetic rates) along with the management of plant water status (e.g., leaf water potential and relative water content) (Augé, 2001; Bárzana et al., 2012). These effects have not been shown to necessarily depend on key metabolism activities, e.g., growth or nutrition (Augé, 2001; Ruiz-Lozano, 2003). Indeed, plant water-use efficiency (WUE), the use of water in plant metabolic activities in relation to its loss in transpiration, has been shown to be highly variable in response in AM vs non-mycorrhizal plants (Augé, 2001). These have been associated with other mechanisms that are linked to water deficit, such as the management of excessive radiation via the enhanced efficiency of photosystem II by AM symbiosis (Ruíz-Sánchez et al., 2011).
Other mechanisms involved in AMF induced plant response to drought include phytohormones, where ABA plays a major role in regulating the expression of aquaporins (AQPs; membrane transporters), increasing water uptake and transport, and root hydraulic conductance (Zargar et al., 2017). Multiple studies have reported the induction of high levels of ABA by AMF root colonization under water stress (Meixner et al., 2005; Aroca et al., 2008; Ludwig-Müller, 2010; Asensio et al., 2012; Ouledali et al., 2019), but lower levels of ABA in mycorrhizal plants responding to drought have been also found (Chitarra et al., 2016; Diagne et al., 2020), indicating that the exact mechanisms are complex and remain elusive.
Nutrients and Water Uptake via AMF
Plant nutritional status can strongly influence the effect of drought stress in plants; indeed, the nutrient uptake by AM fungi alleviates the adverse effects of water stress on plants. Generally, mycorrhiza increased phosphorus (P) uptake under water deficiency (Augé, 2001; Püschel et al., 2021), and in return, better P nutrition enhanced by AMF may improve hydraulic conductivity and help plants to rapidly recover from water stress (Bryla and Duniway, 1997). It has been suggested that higher P content enhances photosynthetic capacity, which can lead to high stomatal conductance and transpiration (Koide, 2010). Compared to P, fewer studies have focused on the importance of AMF in nitrogen nutrition under drought stress conditions. Tobar et al. (1994) found that nitrate is made available to mycorrhizal roots directly by external mycelium under water stress. Although the influence of nitrogen supply on drought tolerance has been well documented in plants (e.g., Ding et al., 2018), the role of nitrogen contributed by AMF in hydration is still unknown.
The direct water uptake of plants via AMF under drought conditions has been questioned due to previous equivocal evidence (Smith and Read, 2010). AM fungal hyphae can penetrate soil pores inaccessible to root hairs and contribute to the redistribution of water from wetter to drier soil patches via AM fungal hyphae (Querejeta et al., 2012). This process (hydraulic lift) has been proposed to keep minimum soil moisture in surface horizons during drought, enabling both the availability of nutrients and the survival of roots and AM fungal hyphae (Smith et al., 2010). Further, the uptake and transfer of water from the soil to the plant via AMF have been experimentally demonstrated from soils with low water potential (Augé, 2001; Ruiz-Lozano, 2003). However, we know little about the relevance of this pathway, although some results are very promising. For instance, Ruth et al. (2011), using a split plant-hyphal chamber with water content sensors, demonstrated that Rhizophagus intraradices contributed 20% to the total water uptake, including indirectly through water effluxed from hyphae and taken up by roots. In turn, more recent greenhouse studies with trifoliate orange (Poncirus trifoliata) under drought vs control treatments highlights the effective role of extraradical hyphae in water absorption under drought conditions: Zou et al. (2019) observed hyphal water absorption greatly increased by 1.4 times, while Zhang F. et al. (2018) noticed elevated hyphal water absorption rate by 2.3–6.6 times.
Gene Regulation
As previously mentioned, the expression of drought-responsive plant genes causes the regulation of physiological responses of mycorrhizal plants to drought stress. Wu et al. (2017) reported reduced P5CS activity in AMF seedlings under drought conditions, indicating that decrease in proline accumulation in AMF seedlings is potentially associated with an AMF-modulated decrease of glutamate synthetic pathways of proline. However, inoculation with Bradyrhizobium japonicum in Glycine max and Lactuca sativa plants clearly indicates that induction of P5CS gene cannot be regarded as a AMF-induced mechanism in alleviating water stress (Porcel et al., 2004). Further studies are needed to understand these discrepancies in the regulation of P5CS gene in AM plants under drought. Studies have found drought treatment significantly increased the expression of NCED genes (Aroca et al., 2008; Merlaen et al., 2019), which encode key enzymes in ABA biosynthesis. AMF-regulated root FA desaturase genes, PtFAD genes, were also observed in P. trifoliate under water deficiency. Similarly, AQP genes have also been described (Porcel et al., 2006; Quiroga et al., 2017). AQPs are important components of the cell transport system. It is believed that AQPs are usually associated with the symbiotic exchange process, determining the transport characteristics of plants and fungi, exhibiting diverse responses to mycorrhization (Maurel and Plassard, 2011, Jia-Dong et al., 2019). Indeed, Li et al. (2013) were the first ones to identify two functional AQP genes from the AMF R. intraradices, in both root cortical cells containing arbuscules and extraradical mycelia under induced osmotic stress, which highlights and confirms the fundamental and direct role of AMF in plant drought tolerance. A further step would promote mycorrhization to circumvent drought conditions in crop production. For example, more recent research has also identified a plant gene MdIAA24, which positively regulated the mycorrhization of apples by controlling the biosynthesis of strigolactone. Overexpression of this gene was associated with a general AM-induced drought-tolerant response with increased relative water content, greater osmotic adjustment, and improved gas exchange capacity among other responses (Huang et al., 2021). In turn, certain.
Antioxidant genes of AMF have shown to contribute to the mitigation of oxidative stress under drought stress (Zou et al., 2021). Together with the antioxidant systems in plants, the network of antioxidant systems in AMF are active scavengers of ROS. Similarly, a suggested mechanism by Zou et al. (2019) to maximize the plant resistance to water stress, was a complex up and down regulation of specific AQPs gene expression, although research in other species and in more natural conditions is needed to demonstrate where specific AQPs gene expression patterns are universal.
Modification of Root Morphology and Soil
Several studies have highlighted that AMF colonization can modulate root morphological adaptations to improve the drought tolerance of plants. These adaptations have been correlated with changes in phytohormones regulating different metabolic pathways (Wu et al., 2012, 2016; Liu et al., 2016). For instance, Wu et al. (2016) analyzed the changes in root morphology of trifoliate orange (P. trifoliata) seedlings treated with AMF and found a higher total root length and volume under drought stress conditions, correlated with an increase in indole-3-acetic acid, and methyl jasmonate. In the example of Wu et al. (2016), plant tolerance to drought was primarily associated with larger exploration of soil volume by roots, which allowed the plants to absorb more water and nutrients from the soil, as well as the additional AMF radical hyphae.
In addition to wider soil exploration by hyphae, AMF can induce changes in soil structure and organic matter content, which has been related to soil water retention capacity and hydraulic conductivity (Wu et al., 2014a; Bitterlich et al., 2018). Extraradical hyphae produce exudates and degradation by-products, which enhance the formation of soil aggregates, and thus modify soil aggregation, bulk density, and pore size distribution (Rillig and Mummey, 2006; Wilson et al., 2009). Some of these exudates are fungal-derived carbon compounds, e.g., glomalin, that directly contribute to soil organic matter accumulation, promoting soil organic matter storage through aggregate stabilization (Rillig et al., 2001; Wilson et al., 2009; Parihar et al., 2020). Relative to other indirect mechanisms of AMF induced drought tolerance, soil modification, despite its potential relevance, has received little attention (Querejeta, 2017). Most studies are developed in controlled conditions comparing plants with similar size, age, nutritional status, with and without AMF. Therefore, our knowledge lags about the effects of AMF community changes under more natural conditions, in which there are multiple stressors and the potential interaction of multiple microbial organisms.
Free-Living Symbiotic Fungi Effects on Ameliorating Drought Stress: Trichoderma
Besides the arbuscular mycorrhizal fungi, other fungal root endophytes, such as some strains in the genus Trichoderma, have parallel mutualistic roles compared to mycorrhizal fungi, enhancing plant growth and resistance to abiotic and biotic stresses (Harman and Uphoff, 2019). One main difference compared to AMF is that Trichoderma is relatively easy to culture, which can explain the successful earlier application of Trichoderma in agriculture (Field et al., 2021; Khan et al., 2021). Plant beneficial fungal strains of Trichoderma (hereafter Trichoderma) have been considered a free-living opportunistic avirulent plant symbiont (Harman et al., 2004). Among its symbiotic activities, which have historically attracted more scientific attention, are its role protecting plants against several fungal pathogens, and as a stress tolerance inducer, with important implications for sustainable crop production (Lorito et al., 2010; Harman and Uphoff, 2019). The defenseive abilities of Trichoderma, as an antagonist of fungal pathogens and even nematodes, have different mechanisms: the competition for space and nutrients with other fungi, production of antifungal metabolites (antibiosis), and very effective mycoparasitism. Amelioration of drought effects on plants by Trichoderma are thought to be a consequence of its abilities to reprogram plant gene expression through the putative activation of a limited number of general plant pathways (Shoresh et al., 2010).
Mechanisms of Ameliorating Drought Stress of Trichoderma
Plants treated with Trichoderma respond to drought stress by modulating physiological and biochemical parameters, which ultimately leads to the restoration of cellular homeostasis, detoxification of toxins and recovery of growth. Scudeletti et al. (2021) demonstrated that the inoculation of sugarcane plants with Trichoderma asperellum changed antioxidant metabolism, increasing superoxide dismutase and peroxidase enzyme activity, as well as the proline concentration and sugar portioning, and enhanced root and stalk development, thus helping plants to alleviate the negative effects of drought stress. Gusain et al. (2014) have observed enhanced drought tolerance in rice due to Trichoderma harzianum T35 colonization, and they indicated that T. harzianum promoted activity of antioxidant enzymes, SOD, CAT, and APX, thereby preventing oxidative damage to rice through quick elimination of ROS. Mastouri et al. (2012) observed that enhanced tolerance of water stress in tomato caused by T. harzianum T22 was due to the ability of plants to remove damaging ROS, which was accompanied by an increase in the activity of antioxidant enzymes. Mona et al. (2017) concluded that T. harzianum was useful in mitigating drought stress by modulation of plant secondary metabolites. The fungi improved the osmolyte proline concentration in plant tissue and increased synthesis of growth hormones, thus protecting membranes from ROS and enhancing the root system so that it can access more nutrients.
Virus-Mediated Drought Tolerance
Viruses are submicroscopic infectious agents that are absolutely dependent on host living cells for their multiplication. In most cases, viruses have been discovered and studied in connection with the diseases they cause. Thus, they typically are regarded as pathogenic parasites, but there is increasing evidence that some viruses impart beneficial properties to their hosts. The examples range from obligate mutualistic symbiotic relationships in which viruses confer functions essential for the survival of their hosts and, therefore, the survival of the viruses themselves, to benefits that improve host performance under certain conditions (reviewed in Roossinck, 2011).
Remarkably, a number of examples illustrating beneficial effects of viruses on their hosts include cases in which virus infection promotes heat or drought tolerance in the host. For instance, virus infection of a fungal endophyte, Curvularia protuberata, was shown to mediate the ability of the fungus to confer heat tolerance to its host, a tropical panic grass, Dichanthelium lanuginosum, and, thus, survival of the host in geothermal soils in Yellowstone National Park, United States (Márquez et al., 2007). Fungal isolates cured of their symbiont, a double-stranded RNA mycovirus, Curvularia thermal tolerance virus (CThTV), lost the ability to confer thermal tolerance, while tolerance was restored upon virus reintroduction. Xu et al. (2008) showed that virus infection can improve drought tolerance in various plants. Infection of Nicotiana benthamiana plants with any of four RNA viruses—Brome mosaic virus (BMV), Cucumber mosaic virus(CMV), Tobacco mosaic virus, or Tobacco rattle virus—delayed the onset of drought symptoms when plants were kept under water-deficit conditions, compared to uninfected control plants. A similar effect was found in BMV-infected rice plants and in nine other plant species infected with CMV, including economically important crops such as tomato (Solanum lycopersium), watermelon (Cucumis lanatus), zucchini (Cucurbita pepo), cucumber (Cucumis sativus), pepper (Capsicum annum), and beet (Beta vulgaris).
Mechanisms That Mediate the Effect of Virus Infection on Plant Drought Tolerance
Regulation of Osmoprotectants, Antioxidants, and Phytohormone Signaling
Transcriptome analyses of C. protuberata responses to CThTV infection and heat stress demonstrated that genes encoding for key enzymes in the biosynthesis and turnover of osmoprotectants such as trehalose, glycine, betaine, and taurine, as well as in the production of melanin, a pigment known to be involved in the mediation of abiotic stress in fungi, were highly induced in CThTV-caring isolates compared to the virus-free fungus in response to heat stress (Morsy et al., 2010). It is likely that such virus-induced changes in the fungus transcriptome contributed to the survival of D. lanuginosum, the third partner in this three-way mutualistic symbiosis found by Márquez et al. (2007) as discussed above. However, further studies will be needed to fully elucidate the virus role. Increased accumulation of several osmoprotectants, including trehalose and other sugars, as well as antioxidants, was found in metabolite profiling in CMV-infected beet and BMV-infected rice plants, which showed improved drought tolerance (Xu et al., 2008). Yet, some metabolite changes were dependent on the pathosystem. Interestingly, the overall number of metabolites affected by water withholding was less in virus-infected than in mock-inoculated plants, suggesting that the infected plants had less sensitivity to drought. A more recent study demonstrated that, in the case of CMV, drought tolerance of A. thaliana plants was conferred by the viral protein 2b, which interferes with plant RNA silencing pathways and also with signaling mediated by abscisic acid, the abiotic stress response hormone (Westwood et al., 2013). Water stress tolerance in N. benthamiana plants also increased when infected by Yellowtail flower mild mottle virus, manifested as a significant delay in shoot tip wilting (Dastogeer et al., 2018). The virus effect on plant gene expression and production of osmolytes and antioxidants under water-deficit conditions was similar to that of two ascomycete fungal endophytes, isolated from wild Australian Nicotiana species growing in a drought-prone area, which were tested along with the virus. These findings suggested that there is some similarity in the responses of fungus- and virus-infected plants to drought stress.
Plant Virus Evolution Under Drought Conditions Results in Increased Plant Tolerance to Drought
Changes in the environment and stress, including drought, greatly affect plant–virus interactions (Bergès et al., 2018). It seems plausible that viruses, which have an intrinsic ability to evolve rapidly, can provide new functions to hosts that enable adaptation to changing environmental conditions. Indeed, a recent study by González et al. (2021) showed that plant virus evolution under drought conditions modulated virus-plant co-adaptation, resulting in increased plant tolerance to drought. In this work, an RNA virus, Turnip mosaic virus (TuMV), evolved in well-watered and drought conditions by passaging in four accessions of A. thaliana differing in their responses to TuMV infection. Virus variants that evolved in water-deficit conditions conferred a significantly higher drought tolerance to the host plants, compared to those that evolved under the standard watering conditions. Most mutations were concentrated in the region of the virus genome that encodes VPg, a multifunctional protein involved in several aspects of virus–host interactions. The analyses of transcriptomic changes and hormonal levels in plants in response to virus infection and drought revealed that greater resistance to drought conferred by drought-evolved viruses was correlated with complex changes in gene expression and reorganization of hormone signaling. Interestingly, though, such responses were dependent on the plant accession, indicating that plant adaptation to stress involves multiple pathways. Altogether, findings from this intriguing study of experimental virus evolution under severe drought conditions provides evidence of a rapid transition from viral parasitism to mutualism taking place under stress.
Systems Analysis to Understand the Role of the Microbiome in Drought Tolerance
A full understanding of microbiome effects on drought will support the development of more effective microbial assemblages in agriculture. It is likely that assemblages of species will be more effective in supporting drought tolerance than single species, if assemblages that support each other’s effects can be identified. Network analysis is a useful tool for identifying candidate assemblages of microbes supporting plant health, based on associations in experimental samples (Poudel et al., 2016; Garrett et al., 2018). Microbiome networks typically characterize taxa in networks in which links indicate positive (or in some cases negative) associations between taxa. Evaluating these networks can help to identify not only those microbial taxa that have positive associations with drought tolerance, but also other taxa that have positive associations with beneficial taxa, or negative associations with taxa that have negative associations with beneficial taxa (as in, “the enemy of my enemy is my friend”). Network analysis can be used to identify taxa with potentially important roles as hubs or connectors. An inherent challenge in the study of microbiome networks based on observed associations is that these associations commonly reflect factors other than biological interactions, such as shared environmental requirements, or are artifacts based on the use of relative abundance rather than absolute abundance. Network analyses can identify candidate assemblages for further empirical testing, an essential next step, to evaluate the practical economic benefits the assemblages may confer.
Machine learning offers another approach to understanding the relationships among microbiomes and drought tolerance (e.g., Poudel et al., 2019; Topçuoğlu et al., 2020). The ability of machine learning to evaluate the potential of large numbers of predictor variables is useful for integrating both the frequencies of microbial taxa and other system traits such as edaphic factors and plant phenotypic traits including transcriptomic data. An inherent limitation is the potential, when studying large numbers of taxa and transcriptomic data, that a large proportion of taxon frequencies and other potential predictors are weakly predictive of plant responses, as discussed by Efron (2020) in a recent paper about machine learning. Deep learning approaches based on neural networks are another exciting possibility as data sets expand and may include plant phenotypic traits such as plant images. Deep learning approaches have the advantage of often producing better predictions than other machine learning methods, but use of multiple machine learning tools can provide complementary perspectives.
Another methodological advance for understanding microbiomes and drought tolerance will be exploration of the use of hypergraphs to describe the microbiome and transcriptome networks for hosts and microbiomes. While typical microbiome networks are constructed based on pair-wise associations, hypergraphs include the option of links between more than two nodes. Hypergraphs have the potential to add an important new perspective on microbiome interactions.
There are also intriguing possibilities for the use of exponential random graph models (ERGMs) in the study of microbiome networks. ERGMs are often used in social network studies to evaluate network structures, such as the probability that specific types of nodes are linked (Snijders, 2002). ERGMs also have potential for evaluating the probability that particular types of taxa are associated, the network motifs describing their associations, and how these structures change from one environment to another, or from drought-tolerant to non-drought-tolerant scenarios. This type of ERGM analysis may prove useful for identifying taxa that exhibit network roles and patterns similar to other taxa known to contribute to drought tolerance. These similar patterns may be indicative of the importance of these taxa: potentially they have similar beneficial roles, or are competitors of beneficial species, etc.
Combining these tools in ensemble models, and evaluating their results side by side, will provide important perspectives on microbial assemblages to support drought tolerance. The hypotheses about key taxon combinations generated by systems analysis will support the next steps for empirical testing.
Conclusion and Future Prospects
Plant microbiomes have the potential to confer tolerance to abiotic and biotic stresses, including drought. The application of single microbes, consortia of several microbial isolates or the manipulation of the crop microbiome represent promising strategies for addressing many of the challenges of drought to agricultural productivity. Multiple traits of microbes should be considered to identify more efficient inoculants for future use in agriculture. As previously proposed for biotic stress tolerance (Mendes et al., 2013), we suggest the assembly of a “minimal microbiome,” comprised by a minimal set of microbial traits needed to provide a specific ecosystem service, in this case tolerance to drought stress. One way to do this is through microbiome engineering (Kaushal and Wani, 2016). To make progress on this, it is essential to understand the chemical signaling and molecular mechanisms through which PGPR and microbiomes affect plants and interact with them during stress. In addition, drought-tolerant bacteria and microbiomes associated with crop species that are naturally adapted to drought should be given more emphasis. Some of these microbes produce non-reproductive structures, such as endospore in the phylum Firmicutes, which can survive under drought conditions for a long period of time, making them good candidates for formulation to be applied in dry areas.
Viruses, though regarded as pathogenic parasites, interestingly can confer drought tolerance to host plants. However, a very limited number of known mechanisms for viruses to provide drought tolerance are known compared to PGPR and AMF. It is also important to unravel the interactions among these organisms as they aid plants exposed to drought stress, and to understand the chemical signaling and molecular mechanisms through which they interact with plants during stress.
Author Contributions
MP wrote the first draft and revised the manuscript into its final format. SM presented the original idea, worked with MP on the first draft, worked on Figure 1 with MP, and edited the final version. RM and LC mainly contributed to the discussion of the role of the whole microbial community in mitigating drought stress, CB and YM contributed to the discussion of arbuscular mycorrhizae and Tricoderma, including Figure 2 and Table 5. KG contributed the discussion of systems analysis to understand microbial roles in drought tolerance. SF contributed to the discussion of virus-mediated drought tolerance. All authors contributed to the writing and edited the final versions and approved the submitted version.
Funding
This work was supported by the USDA National Institute of Food and Agriculture, Hatch project 1024881. CB and YM were financed by the Estonian Ministry of Education and Research (PRG1065) and by the European Union through the European Regional Development Fund (Centre of Excellence EcolChange).
Conflict of Interest
RM and LC are employed by the Brazilian Agricultural Research Corporation.
The remaining authors declare that the research was conducted in the absence of any commercial or financial relationships that could be construed as a potential conflict of interest.
Publisher’s Note
All claims expressed in this article are solely those of the authors and do not necessarily represent those of their affiliated organizations, or those of the publisher, the editors and the reviewers. Any product that may be evaluated in this article, or claim that may be made by its manufacturer, is not guaranteed or endorsed by the publisher.
References
Aalipour, H., Nikbakht, A., Etemadi, N., Rejali, F., and Soleimani, M. (2020). Biochemical response and interactions between arbuscular mycorrhizal fungi and plant growth promoting rhizobacteria during establishment and stimulating growth of Arizona cypress (Cupressus arizonica G.) under drought stress. Sci. Hortic. 261:108923. doi: 10.1016/j.scienta.2019.108923
Ahmed, A., Abdelmalik, A., Alsharani, T., Al-Qarawi, B. A. Q., and Aref, I. (2020). Response of growth and drought tolerance of Acacia seyal Del. seedlings to arbuscular mycorrhizal fungi. Plant Soil Environ. 66, 264–271. doi: 10.17221/206/2020-PSE
Ali, L., Khalid, M., Asghar, H. N., and Asgher, M. (2017). Scrutinizing of rhizobacterial isolates for improving drought resilience in maize (Zea mays). Int. J. of Agric. Biol. 19, 1054–1064. doi: 10.17957/IJAB/15.0387
Ali, S. Z., Sandhya, V., and Rao, L. V. (2014). Isolation and characterization of drought-tolerant ACC deaminase and exopolysaccharide-producing fluorescent Pseudomonassp. Ann. Microbiol. 64, 493–502. doi: 10.1007/s13213-013-0680-3
Altamura, M. M., Torrigiani, P., Capitani, F., Scaramagli, S., and Bagni, N. (1991). De novo root formation in tobacco thin layers affected by inhibition of polyamine biosynthesis. J Exp Bot 42, 1575–1582. doi: 10.1093/jxb/42.12.1575
Alvares, C. A., Stape, J. L., Sentelhas, P. C., de Moraes Gonçalves, J. L., and Sparovek, G. (2013). Köppen’s climate classification map for Brazil. Meteorol. Z. 22, 711–728. doi: 10.1127/0941-2948/2013/0507
Ansari, F. A., and Ahmad, I. (2019). Isolation, functional characterization and efficacy of biofim forming rhizobacteria under abiotic stress conditions. Anton. Leeuw. Int. J. G. 112, 1827–1839. doi: 10.1007/s10482-019-01306-3
Ansary, M. H., Rahmani, H. A., Ardakani, M. R., Paknejad, F., Habibi, D., and Mafakheri, S. (2012). Effect of Pseudomonas fluorescent on proline and phytohormonal status of maize (Zea mays L.) under water deficit stress. Ann. Biol. Res. 3, 1054–1062.
Armada, E., Probanza, A., Roldán, A., and Azcón, R. (2016). Native plant growth promoting bacteria Bacillus thuringiensis and mixed or individual mycorrhizal species improved drought tolerance and oxidative metabolism in Lavandula dentata plants. J. Plant Physiol. 192, 1–12. doi: 10.1016/j.jplph.2015.11.007
Aroca, R., Vernieri, P., and Ruiz-Lozano, J. M. (2008). Mycorrhizal and non-mycorrhizal Lactuca sativa plants exhibit contrasting responses to exogenous ABA during drought stress and recovery. J. Exp. Bot. 59, 2029–2041. doi: 10.1093/jxb/ern057
Asari, S., Matzén, S., Petersen, M. A., Bejai, S., and Meijer, J. (2016). Multiple effects of Bacillus amyloliquefaciensvolatile compounds: plant growth promotion and growth inhibition of phytopathogens. FEMS Microbiol. Ecol. 92:fiw070. doi: 10.1093/femsec/fiw070
Asensio, D., Rapparini, F., and Peñuelas, J. (2012). AM fungi root colonization increases the production of essential isoprenoids vs. nonessential isoprenoids especially under drought stress conditions or after jasmonic acid application. Phytochemistry 77, 149–161. doi: 10.1016/j.phytochem.2011.12.012
Asghari, B., Khademian, R., and Sedaghati, B. (2020). Plant growth promoting rhizobacteria (PGPR) confer drought resistance and stimulate biosynthesis of secondary metabolites in pennyroyal (Mentha pulegium L.) under water shortage condition. Sci. Hortic. 263:109132. doi: 10.1016/j.scienta.2019.109132
Ashraf, M., and Harris, P. J. C. (2013). Photosynthesis under stressful environments: an overview. Photosynthetica 51, 163–190. doi: 10.1007/s11099-013-0021-6
Augé, R. M. (2001). Water relations, drought and vesicular-arbuscular mycorrhizal symbiosis. Mycorrhiza 11, 3–42. doi: 10.1007/s005720100097
Bahadur, A., Batool, A., Nasir, F., Jiang, S., Mingsen, Q., Zhang, Q., et al. (2019). Mechanistic insights into arbuscular mycorrhizal fungi-mediated drought stress tolerance in plants. Int. J. Mol. 20:4199. doi: 10.3390/ijms20174199
Bandeppa, Paul, S., and Kandpal, B. K. (2015). Evaluation of osmo tolerant rhizobacteria for alleviation of water deficit stress in mustard. Green Farming Int. J. 6, 590–593.
Bano, Q., Ilyas, N., Bano, A., Zafar, N., Akram, A., and Hassan, F. (2013). Effect of Azospirillum inoculation on maize (Zea mays L.) under drought stress. Pak. J. Bot. 45, 13–20.
Bárzana, G., Aroca, R., Paz, J. A., Chaumont, F., Martinez-Ballesta, M. C., and Carvajal, M. (2012). Arbuscular mycorrhizal symbiosis increases relative apoplastic water flow in roots of the host plant under both well-watered and drought stress conditions. Ann. Bot. 109, 1009–1017. doi: 10.1093/aob/mcs007
Baslam, M., and Goicoechea, N. (2012). Water deficit improved the capacity of arbuscular mycorrhizal fungi (AMF) for inducing the accumulation of antioxidant compounds in lettuce leaves. Mycorrhiza 22, 347–359. doi: 10.1007/s00572-011-0408-9
Behrooz, A., Vahdati, K., Rejali, F., Lotfi, M., Sarikhani, S., and Leslie, C. (2019). Arbuscular mycorrhiza and plant growth-promoting bacteria alleviate drought stress in walnut. HortScience 54, 1087–1092. doi: 10.21273/HORTSCI13961-19
Belimov, A. A., Dodd, I. C., Safronova, V. I., Shaposhnikov, A. I., Azarova, T. S., Makarova, N., et al. (2015). Rhizobacteria that produce auxins and contain 1-amino-cyclopropane-1-carboxylic acid deaminase decrease amino acid concentrations in the rhizosphere and improve growth and yield of well-watered and water-limited potato (Solanum tuberosum). Ann. Appl. Biol. 167, 11–25. doi: 10.1111/aab.12203
Beneduzi, A., Ambrosini, A., and Passaglia, L. M. P. (2012). Plant growth-promoting rhizobacteria (PGPR): their potential as antagonists and biocontrol agents. Genet. Mol. Biol. 35, 1044–1051. doi: 10.1590/s1415-47572012000600020
Berg, G., Grube, M., Schloter, M., and Smalla, K. (2014). Unraveling the plant microbiome: looking back and future perspectives. Front. Microbiol. 5:148. doi: 10.3389/fmicb.2014.00148
Berg, G., Rybakova, D., Grube, M., and Köberl, M. (2016). The plant microbiome explored: implications for experimental botany. J. Exp. Bot. 67, 995–1002. doi: 10.1093/jxb/erv466
Bergès, S. E., Vile, D., Vazquez-Rovere, C., Blanc, S., Yvon, M., Bédiée, A., et al. (2018). Interactions between drought and plant genotype change epidemiological traits of Cauliflower mosaic virus. Front. Plant Sci. 9:703. doi: 10.3389/fpls.2018.00703
Bitterlich, M., Franken, P., and Graefe, J. (2018). Arbuscular mycorrhiza improves substrate hydraulic conductivity in the plant available moisture range under root growth exclusion. Front. Plant Sci. 9:301. doi: 10.3389/fpls.2018.00301
Bonatelli, M. L., Lacerda-Júnior, G. V., dos Reis Junior, F. B., Fernandes-Júnior, P. I., Melo, I. S., and Quecine, M. C. (2021). Beneficial plant-associated microorganisms from semiarid regions and seasonally dry environments: a review. Front. Microbiol. 11:553223. doi: 10.3389/fmicb.2020.553223
Bonfante, P., and Genre, A. (2015). Arbuscular mycorrhizal dialogues: do you speak ‘plantish’ or ‘fungish’? Trends Plant Sci. 20, 150–154. doi: 10.1016/j.tplants.2014.12.002
Bonfante, P., and Genre, A. (2010). Mechanisms underlying beneficial plant–fungus interactions in mycorrhizal symbiosis. Nat. Commun. 1, 1–11. doi: 10.1038/ncomms1046
Bresson, J., Varoquaux, F., Bontpart, T., Touraine, B., and Vile, D. (2013). The PGPR strain Phyllobacterium brassicacearum STM196 induces a reproductive delay and physiological changes that result in improved drought tolerance in Arabidopsis. New Phytol. 200, 558–569. doi: 10.1111/nph.12383
Brundrett, M. C. (2002). Coevolution of roots and mycorrhizas of land plants. New Phytol. 154, 275–304. doi: 10.1046/j.1469-8137.2002.00397.x
Brundrett, M. C., and Tedersoo, L. (2018). Evolutionary history of mycorrhizal symbioses and global host plant diversity. New Phytol. 220, 1108–1115. doi: 10.1111/nph.14976
Bruno, L. B., Karthik, C., Ma, Y., Kadirvelu, K., Freitas, H., and Rajkumar, M. (2020). Amelioration of chromium and heat stresses in Sorghum bicolor by Cr6+ reducing- thermotolerant plant growth promoting bacteria. Chemosphere 244:125521. doi: 10.1016/j.chemosphere.2019.125521
Bryla, D. R., and Duniway, J. M. (1997). Growth, phosphorus uptake, and water relations of safflower and wheat infected with an arbuscular mycorrhizal fungus. New Phytol. 136, 581–590. doi: 10.1046/j.1469-8137.1997.00780.x
Cabral, C., Ravnskov, S., Tringovska, I., and Wollenweber, B. (2016). Arbuscular mycorrhizal fungi modify nutrient allocation and composition in wheat (Triticum aestivum L.) subjected to heat-stress. Plant Soil 408, 385–399. doi: 10.1007/s11104-016-2942-x
Calvo-Polanco, M., Sánchez-Romera, B., Aroca, R., Asins, M. J., Declerck, S., Dodd, I. C., et al. (2016). Exploring the use of recombinant inbred lines in combination with beneficial microbial inoculants (AM fungus and PGPR) to improve drought stress tolerance in tomato. EEB 131, 47–57. doi: 10.1016/j.envexpbot.2016.06.015
Carríon, V. J., Perez-Jaramillo, J., Cordovez, V., Tracanna, V., de Hollander, M., Ruiz-Buck, D., et al. (2019). Pathogen-induced activation of disease-suppressive functions in the endophytic root microbiome. Science 366, 606–612. doi: 10.1126/science
Cassán, F., Vanderleyden, J., and Spaepen, S. (2014). Physiological and agronomical aspects of phytohormone production by model plant-growth-promoting rhizobacteria (PGPR) belonging to the genus Azospirillum. J. Plant Growth Regul. 33, 440–459. doi: 10.1007/s00344-013-9362-4
Castro, S. P., Cleland, E. E., Wagner, R., Al Sawad, R., and Lipson, D. A. (2019). Soil microbial responses to drought and exotic plants shift carbon metabolism. ISME J. 13, 1776–1787. doi: 10.1038/s41396-019-0389-9
Chandra, D., Srivastava, R., Gupta, V. V. S. R., Franco, C. M. M., and Sharma, A. K. (2019). Evaluation of ACC-deaminase-producing rhizobacteria to alleviate water-stress impacts in wheat (Triticum aestivum L.) plants. Can. J. Microbiol. 65, 387–403. doi: 10.1139/cjm-2018-0636
Chandra, P., Wunnava, A., Verma, P., Chandra, A., and Sharma, R. K. (2021). Strategies to mitigate the adverse effect of drought stress on crop plants—influences of soil bacteria: a review. Pedosphere 31, 496–509. doi: 10.1016/S1002-0160(20)60092-3
Chen, M., Arato, M., Borghi, L., Nouri, E., and Reinhardt, D. (2018). Beneficial services of arbuscular mycorrhizal fungi – from ecology to application. Front. Plant Sci. 9:1270. doi: 10.3389/fpls.2018.01270
Chen, W., Meng, P., Feng, H., and Wang, C. (2020). Effects of arbuscular mycorrhizal fungi on growth and physiological performance of Catalpa bungei CA Mey. under drought stress. Forests 11:1117. doi: 10.3390/f11101117
Chiappero, J., del Rosario Cappellari, L., Alderete, L. G. S., Palermo, T. B., and Banchio, E. (2019). Plant growth promoting rhizobacteria improve the antioxidant status in Mentha piperita grown under drought stress leading to an enhancement of plant growth and total phenolic content. Ind. Crops Prod. 139:111553. doi: 10.1016/j.indcrop.2019.111553
Chitarra, W., Pagliarani, C., Maserti, B., Lumini, E., Siciliano, I., Cascone, P., et al. (2016). Insights on the impact of arbuscular mycorrhizal symbiosis on tomato tolerance to water stress. Plant Physiol. 171, 1009–1023. doi: 10.1104/pp.16.00307
Cho, S. M., Kang, B. R., and Kim, Y. C. (2013). Transcriptome analysis of induced systemic drought tolerance elicited by Pseudomonas chlororaphis O6 in Arabidopsis thaliana. Plant Pathol. J. 29, 209–220. doi: 10.5423/PPJ.SI.07.2012.0103
Choudhary, D. K., Sharma, A. K., Agarwal, P., Varma, A., and Tuteja, N. (2017). “Characterization of bacterial volatiles and their impact on plant health under abiotic stress,” in Volatiles and Food Security, eds D. Choudhary, A. Sharma, P. Agarwal, A. Varma, and N. Tuteja (Singapore: Springer), doi: 10.1007/978-981-10-5553-9
Cohen, A. C., Bottini, R., Pontin, M., Berli, F. J., Moreno, D., and Boccanlandro, H. (2015). Azospirillum brasilense ameliorates the response of Arabidopsis thaliana to drought mainly via enhancement of ABA levels. Physiol. Plant. 153, 79–90. doi: 10.1111/ppl.12221
Contesto, C., Milesi, S., Mantelin, S., Zancarini, A., Desbrosses, G., Varoquaux, F., et al. (2010). The auxin-signaling pathway is required for the lateral root response of Arabidopsis to the rhizobacterium Phyllobacterium brassicacearum. Planta 232, 1455–1470. doi: 10.1007/s00425-010-1264-0
Cook, R. J., and Baker, K. F. (1983). The Nature And Practice Of Biological Control Of Plant Pathogens. St. Paul, Min: American Phytopathological Society.
da Silva, E. C., de Albuquerque, M. B., de Azevedo Neto, A. D., and da Silva Junior, C. D. (2013). “Drought and its consequences to plants–from individual to ecosystem,” in Responses of Organisms to Water Stress, ed. Ş Akıncı (London: InTech), doi: 10.5772/53833
Danish, S., and Zafar-ul-Hye, M. (2019). Co-application of ACC-deaminase producing PGPR and timber-waste biochar improves pigments formation, growth and yield of wheat under drought stress. Sci. Rep. 9:5999. doi: 10.1038/s41598-019-42374-9
Danish, S., Zafar-ul-Hye, M., Fahad, S., Saud, S., Brtnicky, M., Hammerschmidt, T., et al. (2020). Drought stress alleviation by ACC deaminase producing Achromobacter xylosoxidans and Enterobacter cloacae, with and without timber waste biochar in maize. Sustainability 12:6286. doi: 10.3390/SU12156286
Daryanto, S., Wang, L., and Jacinthe, P. A. (2016). Global synthesis of drought effects on maize and wheat production. PLoS One 11:e0156362. doi: 10.1371/journal.pone.0156362
Dastogeer, K. M. G., Li, H., Sivasithamparam, K., Jones, M. G. K., and Wylie, S. J. (2018). Fungal endophytes and a virus confer drought tolerance to Nicotiana benthamiana plants through modulating osmolytes, antioxidant enzymes and expression of host drought responsive genes. Environ. Exp. Bot. 149, 95–108. doi: 10.1016/j.envexpbot.2018.02.009
de Vries, F. T., Griffiths, R. I., Knight, C. G., Nicolitch, O., and Williams, A. (2020). Harnessing rhizosphere microbiomes for drought-resilient crop production. Science 368, 270–274. doi: 10.1126/science.aaz5192
Diagne, N., Ngom, M., Djighaly, P. I., Fall, D., Hocher, V., and Svistoonoff, S. (2020). Roles of arbuscular mycorrhizal fungi on plant growth and performance: importance in biotic and abiotic stressed regulation. Diversity 12:370. doi: 10.3390/d12100370
Ding, L., Lu, Z., Gao, L., Guo, S., and Shen, Q. (2018). Is nitrogen a key determinant of water transport and photosynthesis in higher plants upon drought stress? Front. Plant. Sci. 9:1143. doi: 10.3389/fpls.2018.01143
Efron, B. (2020). Prediction, estimation, and attribution. JASA 115, 636–655. doi: 10.1080/01621459.2020.1762613
Egamberdieva, D., Wirth, S. J., Alqarawi, A. A., Abd-Allah, E. F., and Hashem, A. (2017). Phytohormones and beneficial microbes: essential components for plants to balance stress and fitness. Front. Microbiol. 8:2104. doi: 10.3389/fmicb.2017.02104
Eisenstein, M. (2013). Plant breeding: discovery in a dry spell. Nature 501, S7–S9. doi: 10.1038/501S7a
Essahibi, A., Benhiba, L., Babram, M. A., Ghoulam, C., and Qaddoury, A. (2018). Influence of arbuscular mycorrhizal fungi on the functional mechanisms associated with drought tolerance in carob (Ceratonia siliqua L.). Trees 32, 87–97. doi: 10.1007/s00468-017-1613-8
Fang, Y., and Xiong, L. (2015). General mechanisms of drought response and their application in drought resistance improvement in plants. Cell. Mol. Life Sci. 72, 673–689. doi: 10.1007/s00018-014-1767-0
Feijen, F. A. A., Vos, R. A., Nuytinck, J., and Merckx, V. S. F. T. (2018). Evolutionary dynamics of mycorrhizal symbiosis in land plant diversification. Sci. Rep. 8:10698. doi: 10.1038/s41598-018-28920-x
Ferrol, N., Azcon-Aguilar, C., and Pérez-Tienda, J. (2019). Arbuscular mycorrhizas as key players in sustainable plant phosphorus acquisition: an overview on the mechanisms involved. Plant Sci. 280, 441–447. doi: 10.1016/j.plantsci.2018.11.011
Field, K. J., Daniell, T., Johnson, D., and Helgason, T. (2021). Mycorrhizal mediation of sustainable development goals. Plants People Planet 3, 430–432. doi: 10.1002/ppp3.10223
Figueiredo, M. V., Burity, H. A., Martinez, C. R., and Chanway, C. P. (2008). Alleviation of drought stress in the common bean (Phaseolus vulgaris L.) by co-inoculation with Paenibacillus polymyxa and Rhizobium tropici. Appl. Soil Ecol. 40, 182–188. doi: 10.1016/j.apsoil.2008.04.005
Fitriani Wangsa Putrie, R., Tri Wahyudi, A., AsihNawangsih, A., and Husen, E. (2013). Screening of rhizobacteria for plant growth promotion and their tolerance to drought stress. Microbiol. Indones. 7, 2087–8587. doi: 10.5454/mi.7.3.2
Flemming, H. C., and Wingender, J. (2010). The biofilm matrix. Nat. Rev. Microbiol. 8, 623–633. doi: 10.1038/nrmicro2415
Food Security Information Network and Global Network Against Crises (2020). Available online at: https://www.wfp.org/publications/2020-global-report-food-crises (accessed September 30, 2021).
Francisco, C. S., Ma, X., Zwyssig, M. M., McDonald, B. A., and Palma-Guerrero, J. (2019). Morphological changes in response to environmental stresses in the fungal plant pathogen Zymoseptoria tritici. Sci. Rep. 9:9642. doi: 10.1038/s41598-019-45994-3
Fuchslueger, L., Bahn, M., Hasibeder, R., Kienzl, S., Fritz, K., Schmitt, M., et al. (2016). Drought history affects grassland plant and microbial carbon turnover during and after a subsequent drought event. Ecology. 104, 1453–1465. doi: 10.1111/1365-2745.12593
Gamalero, E., and Glick, B. R. (2015). Bacterial modulation of plant ethylene levels. Plant Physiol. 169, 13–22. doi: 10.1104/pp.15.00284
Garrett, K. A., Alcalá-Briseño, R. I., Andersen, K. F., Buddenhagen, C. E., Choudhury, R. A., Fulton, J. C., et al. (2018). Network analysis: a systems framework to address grand challenges in plant pathology. Annu. Rev. Phytopathol. 56, 559–580. doi: 10.1146/annurev-phyto-080516-035326
Ghosh, D., Sen, S., and Mohapatra, S. (2017). Modulation of proline metabolic gene expression in Arabidopsis thaliana under water-stressed conditions by a drought-mitigating Pseudomonas putida strain. Ann. Microbiol. 67, 655–668. doi: 10.1007/s13213-017-1294-y
Glick, B. R., Cheng, Z., Czarny, J., and Duan, J. (2007). Promotion of plant growth by ACC deaminase-producing soil bacteria. Eur. J. Plant Pathol. 119, 329–339. doi: 10.1007/s10658-007-9162-4
Gong, M., You, X., and Zhang, Q. (2015). Effects of Glomus intraradices on the growth and reactive oxygen metabolism of foxtail millet under drought. Ann. Microbiol. 65, 595–602. doi: 10.1007/s13213-014-0895-y
González, R., Butković, A., Escaray, F. J., Martínez-Latorre, J., Melero, Í, Pérez-Parets, E., et al. (2021). Plant virus evolution under strong drought conditions results in a transition from parasitism to mutualism. Proc. Natl. Acad. Sci. U.S.A. 118:e2020990118. doi: 10.1073/pnas.2020990118
Goswami, M., and Deka, S. (2020). Plant growth-promoting rhizobacteria—alleviators of abiotic stresses in soil: a review. Pedosphere 30, 40–61. doi: 10.1016/S1002-0160(19)60839-8
Gou, W., Tian, L., Ruan, Z., Zheng, P., Chen, F., Zhang, L., et al. (2015). Accumulation of choline and glycine betaine and drought stress tolerance induced in maize (Zea mays) by three plant growth promoting rhizobacteria (PGPR) strains. Pak. J. Bot. 47, 581–586.
Grover, M., Madhubala, R., Ali, S. Z., Yadav, S. K., and Venkateswarlu, B. (2014). Influence of Bacillus spp. strains on seedling growth and physiological parameters of sorghum under moisture stress conditions. J. Basic Microbiol. 54, 951–961. doi: 10.1002/jobm.201300250
Guajardo, E., Correa, J. A., and Contreras-Porcia, L. (2016). Role of abscisic acid (ABA) in activating antioxidant tolerance responses to desiccation stress in intertidal seaweed species. Planta 243, 767–781. doi: 10.1007/s00425-015-2438-6
Gururani, M. A., Upadhyaya, C. P., Baskar, V., Venkatesh, J., Nookaraju, A., and Park, S. W. (2013). Plant growth-promoting rhizobacteria enhance abiotic stress tolerance in Solanum tuberosum through inducing changes in the expression of ROS-scavenging enzymes and improved photosynthetic performance. J. Plant Growth Regul. 32, 245–258. doi: 10.1007/s00344-012-9292-6
Gusain, Y. S., Singh, U. S., and Sharma, A. K. (2014). Enhance activity of stress related enzymes in rice (Oryza sativa L.) induced by plant growth promoting fungi under drought stress. Afr. J. Agric. Res. 9, 1430–1434.
Gusain, Y. S., Singh, U. S., and Sharma, A. K. (2015). Bacterial mediated amelioration of drought stress in drought tolerant and susceptible cultivars of rice (Oryza sativa L.). Afr. 14, 764–773. doi: 10.5897/AJB2015.14405
Harman, G. E., and Uphoff, N. (2019). Symbiotic root-endophytic soil microbes improve crop productivity and provide environmental benefits. Scientifica 2019:9106395. doi: 10.1155/2019/9106395
Harman, G. E., Howell, C. R., Viterbo, A., Chet, I., and Lorito, M. (2004). Trichoderma species—opportunistic avirulent plant symbionts. Nat. Rev. Microbiol. 2, 43–56. doi: 10.1038/nrmicro797
Harris-Valle, C., Esqueda, M., Gutiérrez, A., Castellanos, A. E., Gardea, A. A., and Berbara, R. (2018). Physiological response of Cucurbita pepo var. pepo mycorrhized by Sonoran desert native arbuscular fungi to drought and salinity stresses. Braz. J. Microbiol. 49, 45–53. doi: 10.1016/j.bjm.2017.04.005
Hause, B., and Schaarschmidt, S. (2009). The role of jasmonates in mutualistic symbioses between plants and soil-born microorganisms. Phytochemistrys 70, 1589–1599. doi: 10.1016/j.phytochem.2009.07.003
Hazzoumi, Z., Moustakime, Y., Elharchli, E. H., and Joutei, K. A. (2015). Effect of arbuscular mycorrhizal fungi (AMF) and water stress on growth, phenolic compounds, glandular hairs, and yield of essential oil in basil (Ocimum gratissimum L). Chem. Biol. Technol. Agric. 2:10. doi: 10.1186/s40538-015-0035-3
Hemantaranjan, A. (2018). Molecular Physiology of Abiotic Stresses in Plant Productivity. Jothpur: Scientific Publishers.
Hijri, M. (2016). Analysis of a large dataset of mycorrhiza inoculation field trials on potato shows highly significant increases in yield. Mycorrhiza 26, 209–214. doi: 10.1007/s00572-015-0661-4
Hu, Y., and Chen, B. (2020). Arbuscular mycorrhiza induced putrescine degradation into γ-aminobutyric acid, malic acid accumulation, and improvement of nitrogen assimilation in roots of water-stressed maize plants. Mycorrhiza 30, 329–339. doi: 10.1007/s00572-020-00952-0
Hu, Y., Xie, W., and Chen, B. (2020). Arbuscular mycorrhiza improved drought tolerance of maize seedlings by altering photosystem II efficiency and the levels of key metabolites. Chem. Biol.Technol. 7, 1–14. doi: 10.1186/s40538-020-00186-4
Huang, B., Da Costa, M., and Jiang, Y. (2014). Research advances in mechanisms of turfgrass tolerance to abiotic stresses: from physiology to molecular biology. CRC Crit. Rev. Plant Sci. 33, 141–189. doi: 10.1080/07352689.2014.870411
Huang, D., Ma, M., Wang, Q., Zhang, M., Jing, G., Li, C., et al. (2020). Arbuscular mycorrhizal fungi enhanced drought resistance in apple by regulating genes in the MAPK pathway. Plant Physiol. Biochem. 149, 245–255. doi: 10.1016/j.plaphy.2020.02.020
Huang, D., Wang, Q., Jing, G., Ma, M., Li, C., and Ma, F. (2021). Overexpression of MdIAA24 improves apple drought resistance by positively regulating strigolactone biosynthesis and mycorrhization. Tree Physiol. 41, 134–146. doi: 10.1093/treephys/tpaa109
Hussain, M. B., Zahir, Z. A., Asghar, H. N., and Asgher, M. (2014). Can catalase and exopolysaccharides producing rhizobia ameliorate drought stress in wheat? Int. J. Agric. Biol. 16, 3–13.
Jansson, J. K., and Hofmockel, K. S. (2020). Soil microbiomes and climate change. Nat. Rev. Microbiol. 18, 35–46. doi: 10.1038/s41579-019-0265-7
Jia-Dong, H., Tao, D., Hui-Hui, W., Ying-Ning, Z., Qiang-Sheng, W., and Kamil, K. (2019). Mycorrhizas induce diverse responses of root TIP aquaporin gene expression to drought stress in trifoliate orange. Sci. Hortic. 243, 64–69. doi: 10.1016/j.scienta.2018.08.010
Jochum, M. D., McWilliams, K. L., Borrego, E. J., Kolomiets, M. V., Niu, G., Pierson, E. A., et al. (2019). Bioprospecting plant growth-promoting rhizobacteria that mitigate drought stress in grasses. Front. Microbiol. 10:2106. doi: 10.3389/fmicb.2019.02106
Kapoor, D., Bhardwaj, S., Landi, M., Sharma, A., Ramakrishnan, M., and Sharma, A. (2020). The impact of drought in plant metabolism: how to exploit tolerance mechanisms to increase crop production. Appl. Sci. 10:16. doi: 10.3390/app10165692
Karvembu, P., Gomathi, V., Anandham, R., and Mary, J. K. (2021). Isolation, screening and identification of moisture stress tolerant Rhizobacteria from xerophyte Prosopis juliflora (SW). J. Pharmacogn. Phytochem. 9, 605–609. doi: 10.22271/phyto.2020.v9.i6i.12981
Kasim, W. A., Osman, M. E., Omar, M. N., Abd El-Daim, I. A., Bejai, S., and Meijer, J. (2013). Control of drought stress in wheat using plant-growth-promoting bacteria. J. Plant Growth Regul. 32, 122–130. doi: 10.1007/s00344-012-9283-7
Kaushal, M., and Wani, S. P. (2016). Plant-growth-promoting rhizobacteria: drought stress alleviators to ameliorate crop production in drylands. Ann. Microbiol. 66, 35–42. doi: 10.1007/s13213-015-1112-3
Kavamura, V. N., Taketani, R. G., Lançoni, M. D., Andreote, F. D., Mendes, R., and Soares de Melo, I. (2013). Water regime influences bulk soil and rhizosphere of Cereus jamacaru bacterial communities in the Brazilian caatinga biome. PLoS One 8:e73606. doi: 10.1371/journal.pone.0073606
Kerry, R. G., Patra, S., Gouda, S., Patra, J. K., and Das, G. (2018). “Microbes and their role in drought tolerance of agricultural food crops,” in Microbial Biotechnology, eds J. Patra, G. Das, and H. S. Shin (Singapore: Springer), doi: 10.1007/978-981-10-7140-9_12
Khan, M. R., Parveen, G., Zaid, A., Wani, S. H., and Jogaiah, S. (2021). “Potential of Trichoderma species in alleviating the adverse effects of biotic and abiotic stresses in plants,” in Biocontrol Agents and Secondary Metabolites, ed. S. Jogaiah (Sawston: Woodhead Publishing), 85–112.
Khan, N., and Bano, A. (2019). Exopolysaccharide producing rhizobacteria and their impact on growth and drought tolerance of wheat grown under rainfed conditions. PLoS One 14:e0222302. doi: 10.1371/journal.pone.0222302
Khan, N., Bano, A., and Babar, M. A. (2019a). Metabolic and physiological changes induced by plant growth regulators and plant growth promoting rhizobacteria and their impact on drought tolerance in Cicer arietinum L. PLoS One 14:e0213040. doi: 10.1371/journal.pone.0213040
Khan, N., Bano, A., Rahman, M. A., Guo, J., Kang, Z., and Babar, M. A. (2019b). Comparative physiological and metabolic analysis reveals a complex mechanism involved in drought tolerance in chickpea (Cicer arietinum L.) induced by PGPR and PGRs. Sci. Rep. 9, 1–19. doi: 10.1038/s41598-019-38702-8
Khan, Z., Rho, H., Firrincieli, A., Hung, S. H., Luna, V., Masciarelli, O., et al. (2016). Growth enhancement and drought tolerance of hybrid poplar upon inoculation with endophyte consortia. Curr. Plant Biol. 6, 38–47. doi: 10.1016/j.cpb.2016.08.001
Kim, Y. C., Glick, B. R., Bashan, Y., and Ryu, C. M. (2012). “Enhancement of plant drought tolerance by microbes,” in Plant Responses to Drought Stress, ed. R. Aroca (Heidelberg: Springer), 383–413.
Koide, R. T. (2010). “Mycorrhizal symbiosis and plant reproduction,” in Arbuscular Mycorrhizas: Physiology and Function, eds H. Koltai and Y. Kapulnik (Dordecht: Springer), 297–320.
Kumar, A., Altabella, T., Taylor, M. A., and Tiburcio, A. F. (1997). Recent advances in polyamine research. Trends Plant Sci. 2, 124–130. doi: 10.1016/S1360-1385(97)01013-3
Kumar, A., and Verma, J. P. (2018). Does plant—microbe interaction confer stress tolerance in plants: a review? Microbiol. Res. 207, 41–52. doi: 10.1016/j.micres.2017.11.004
Laxa, M., Liebthal, M., Telman, W., Chibani, K., and Dietz, K. J. (2019). The role of the plant antioxidant system in drought tolerance. Antioxidants 8:94. doi: 10.3390/antiox8040094
Le Gall, H., Philippe, F., Domon, J. M., Gillet, F., Pelloux, J., and Rayon, C. (2015). Cell wall metabolism in response to abiotic stress. Plants 4, 112–166. doi: 10.3390/plants4010112
Lesk, C., Rowhani, P., and Ramankutty, N. (2016). Influence of extreme weather disasters on global crop production. Nature 529, 84–87. doi: 10.14288/1.0376077
Li, T., Hu, Y.-J., Hao, Z.-P., Hong, L., Wang, Y.-S., and Chen, B.-D. (2013). First cloning and characterization of two functional aquaporin genes from an arbuscular mycorrhizal fungus Glomus intraradices. New Phytol. 197, 617–630. doi: 10.1111/nph.12011
Li, T., Sun, Y., Ruan, Y., Xu, L., Hu, Y., Hao, Z., et al. (2016). Potential role of D-myo-inositol-3-phosphate synthase and 14-3-3 genes in the crosstalk between Zea mays and Rhizophagus intraradices under drought stress. Mycorrhiza 26, 879–893. doi: 10.1007/s00572-016-0723-2
Lim, J.-H., and Kim, S.-D. (2013). Induction of drought stress resistance by multi-functional pgpr bacillus licheniformis K11 in pepper. Plant Phytol. 29, 201–208.
Liu, F. C., Xing, S. J., Ma, H. L., Du, Z. Y., and Ma, B. Y. (2013). Cytokinin-producing, plant growth-promoting rhizobacteria that confer resistance to drought stress in Platycladus orientalis container seedlings. App. Microb. Biotech. 97, 9155–9164. doi: 10.1007/s00253-013-5193-2
Liu, J., Guo, C., Chen, Z.-L., He, J.-D., and Zou, Y.-N. (2016). Mycorrhizal inoculation modulates root morphology and root phytohormone responses in trifoliate orange under drought stress. Emir. Jour. Food Agric. 28, 251–256. doi: 10.9755/ejfa.2015-11-1044
Liu, L., Li, D., Ma, Y., Shen, H., Zhao, S., and Wang, Y. (2020). Combined application of arbuscular mycorrhizal fungi and exogenous melatonin alleviates drought stress and improves plant growth in tobacco seedlings. J. Plant Growth Regul. 40, 1074–1087. doi: 10.1007/s00344-020-10165-6
Lorito, M., Woo, S. L., Harman, G. E., and Monte, E. (2010). Translational research on Trichoderma: from ’omics to the field. Ann. Rev. Phytopathol. 48, 395–417. doi: 10.1146/annurev-phyto-073009-114314
Lou, X., Zhang, X., Zhang, Y., and Tang, M. (2021). The synergy of arbuscular mycorrhizal fungi and exogenous abscisic acid benefits Robinia pseudoacacia L. Growth through altering the distribution of Zn and endogenous abscisic acid. J. Fungus 7:671. doi: 10.3390/jof7080671
Ludwig-Müller, J. (2010). “Hormonal responses in host plants triggered by arbuscular mycorrhizal fungi,” in Arbuscular Mycorrhizas: Physiology And Function, eds H. Koltai and Y. Kapulnik (Dordrecht: Springer), 169–190. doi: 10.1007/978-90-481-9489-6_8
Ma, Y., Dias, M. C., and Freitas, H. (2020). Drought and salinity stress responses and microbe-induced tolerance in plants. Front. Plant Sci. 11:1750. doi: 10.3389/fpls.2020.591911
Márquez, L. M., Redman, R. S., Rodriguez, R. J., and Roossinck, M. J. (2007). A virus in a fungus in a plant: three-way symbiosis required for thermal tolerance. Science 315, 513–515. doi: 10.1126/science.1136237
Martín-Robles, N., Lehmann, A., Seco, E., Aroca, R., Rillig, M. C., and Milla, R. (2018). Impacts of domestication on the arbuscular mycorrhizal symbiosis of 27 crop species. New Phytol. 218, 322–334. doi: 10.1111/nph.14962
Martins, S. A., Schurt, D. A., Seabra, S. S., Martins, S. J., Ramalho, M. A. P., Moreira, F. M. S., et al. (2018). Common bean (Phaseolus vulgaris L.) growth promotion and biocontrol by rhizobacteria under Rhizoctonia solani suppressive and conducive soils. Appl. Soil. Ecol. 127, 129–135. doi: 10.1016/j.apsoil.2018.03.007
Martins, S. J., Faria, A. F., Medeiros, F. H. V., Pedroso, M. P., Cunha, M. G., and Rocha, M. R. (2019). Rhizobacterial volatiles in the control of anthracnose in common bean. Biol. Control 131, 36–42. doi: 10.1016/j.biocontrol.2019.01.003
Martins, S. J., Medeiros, F. H. V., Souza, R. M., and Ferreira Vilela, L. A. (2014). Is curtobacterium wilt biocontrol temperature dependent? Acta Sci. Agron. 36, 409–415. doi: 10.4025/actasciagron
Martins, S. J., Medeiros, F. H. V., Souza, R. M., Rezende, M. L. V., and Ribeiro Junior, P. M. (2013). Biological control of bacterial wilt of common bean by plant growth-promoting rhizobacteria. Biol. Control 66, 65–71. doi: 10.1016/j.biocontrol.2013.03.009
Martins, S. J., Rocha, G. A., de Melo, H. C., de Castro Georg, R., Ulhôa, C. J., and de Campos Dianese, É, et al. (2018b). Plant-associated bacteria mitigate drought stress in soybean. Environ. Sci. Pollut. Res. Int. 25, 13676–13686. doi: 10.1007/s11356-018-1610-5
Martins, S. J., Medeiros, F. H. V., Lakshmanan, V., and Bais, H. P. (2018a). Impact of seed exudates on growth and biofilm formation of Bacillus amyloliquefaciens ALB629 in common bean. Front. Microbiol. 8:2631. doi: 10.3389/fmicb.2017.02631
Martins, S. J., Soares, A. C., Medeiros, F. H. V., Santos, D. B. C., and Pozza, E. A. (2015). Contribution of host and environmental factors to the hyper parasitism of coffee rust under field conditions. Australas. Plant Pathol. 44, 605–610. doi: 10.1007/s13313-015-0375-2
Mastouri, F., Björkman, T., and Harman, G. E. (2012). Trichoderma harzianum enhances antioxidant defense of tomato seedlings and resistance to water deficit. Mol. Plant Microbe Interact. 25, 1264–1271. doi: 10.1094/MPMI-09-11-0240
Maurel, C., and Plassard, C. (2011). Aquaporins: for more than water at the plant–fungus interface? New Phytol. 190, 815–817. doi: 10.1111/j.1469-8137.2011.03731.x
Meixner, C., Ludwig-Müller, J., Miersch, O., Gresshoff, P., Staehelin, C., and Vierheilig, H. (2005). Lack of mycorrhizal autoregulation and phytohormonal changes in the supernodulating soybean mutant nts 1007. Planta 222, 709–715. doi: 10.1007/s00425-005-0003-4
Mendes, R., Garbeva, P., and Raaijmakers, J. M. (2013). The rhizosphere microbiome: significance of plant beneficial, plant pathogenic, and human pathogenic microorganisms. FEMS Microbiol. Rev. 37, 634–663. doi: 10.1111/1574-6976.12028
Mendes, R., Kruijt, M., de Bruijn, I., Dekkers, E., van der Voort, M., and Schneider, J. H. (2011). Deciphering the rhizosphere microbiome for disease-suppressive bacteria. Science 332, 1097–1100. doi: 10.1126/science.1203980
Merlaen, B., De Keyser, E., Ding, L., Leroux, O., Chaumont, F., and Van Labeke, M.-C. (2019). Physiological responses and aquaporin expression upon drought and osmotic stress in a conservative vs prodigal Fragaria x ananassa cultivar. Plant Physiol. Biochem. 145, 95–106. doi: 10.1016/j.plaphy.2019.10.030
Molina-Favero, C., Creus, C. M., Simontacchi, M., Puntarulo, S., and Lamattina, L. (2008). Aerobic nitric oxide production by Azospirillum brasilense Sp245 and its influence on root architecture in tomato. Mol. Plant Microbe Interact. 21, 1001–1009. doi: 10.1094/MPMI-21-7-1001
Mona, S. A., Hashem, A., Abd_Allah, E. F., Alqarawi, A. A., Soliman, D. W. K., Wirth, S., et al. (2017). Increased resistance of drought by Trichoderma harzianum fungal treatment correlates with increased secondary metabolites and proline content. J. Integr. Agric. 16, 1751–1757. doi: 10.1016/S2095-3119(17)61695-2
Moreno-Galván, A. E., Cortés-Patiño, S., Romero-Perdomo, F., Uribe-Vélez, D., Bashan, Y., and Bonilla, R. R. (2020a). Proline accumulation and glutathione reductase activity induced by drought-tolerant rhizobacteria as potential mechanisms to alleviate drought stress in Guinea grass. Appl. Soil Ecol. 147:103367. doi: 10.1016/j.apsoil.2019.103367
Moreno-Galván, A., Romero-Perdomo, F. A., Estrada-Bonilla, G., Meneses, C. H. S. G., and Bonilla, R. R. (2020b). Dry-caribbean Bacillus spp. Strains ameliorate drought stress in maize by a strain-specific antioxidant response modulation. Microorganisms 8:823. doi: 10.3390/microorganisms8060823
Morsy, M. R., Oswald, J., He, J., Tang, Y., and Roossinck, M. J. (2010). Teasing apart a three-way symbiosis: transcriptome analyses of Curvularia protuberata in response to viral infection and heat stress. Biochem. Biophys. Res. Commun. 401, 225–230. doi: 10.1016/j.bbrc.2010.09.034
Nadeem, S. M., Ahmad, M., Zahir, Z. A., Javaid, A., and Ashraf, M. (2014). The role of mycorrhizae and plant growth promoting rhizobacteria (PGPR) in improving crop productivity under stressful environments. Biotechnol. Adv. 32, 429–448. doi: 10.1016/j.biotechadv.2013.12.005
Namwongsa, J., Jogloy, S., Vorasoot, N., Boonlue, S., Riddech, N., and Mongkolthanaruk, W. (2019). Endophytic bacteria improve root traits, biomass and yield of Helianthus tuberosus L. under normal and deficit water conditions. J. Microbiol. Biotechnol. 29, 1777–1789. doi: 10.4014/jmb.1903.03062
Naseem, H., and Bano, A. (2014). Role of plant growth-promoting rhizobacteria and their exopolysaccharide in drought tolerance of maize. J. Plant Interact. 9, 689–701. doi: 10.1080/17429145.2014.902125
Naveed, M., Mitter, B., Reichenauer, T. G., Wieczorek, K., and Sessitsch, A. (2014). Increased drought stress resilience of maize through endophytic colonization by Burkholderia phytofirmans PsJN and Enterobacter sp. FD17. EEB. 97, 30–39. doi: 10.1016/j.envexpbot.2013.09.014
Naylor, D., and Coleman-Derr, D. (2018). Drought stress and root-associated bacterial communities. Front. Plant Sci. 8:2223. doi: 10.3389/fpls.2017.02223
Naylor, D., Degraaf, S., Purdom, E., and Coleman-Derr, D. (2017). Drought and host selection influence bacterial community dynamics in the grass root microbiome. ISME J. 11, 2691–2704. doi: 10.1038/ismej.2017.118
Ngumbi, E., and Kloepper, J. (2016). Bacterial-mediated drought tolerance: current and future prospects. Appl. Soil Ecol. 105, 109–125. doi: 10.1016/j.apsoil.2016.04.009
Niinemets, Ü (2010). Mild versus severe stress and BVOCs: thresholds, priming and consequences. Trends Plant Sci. 15, 145–153. doi: 10.1016/j.tplants.2009.11.008
Niu, X., Song, L., Xiao, Y., and Ge, W. (2018). Drought-tolerant plant growth-promoting rhizobacteria associated with foxtail millet in a semi-arid agroecosystem and their potential in alleviating drought stress. Front. Microbiol. 8:2580. doi: 10.3389/fmicb.2017.02580
Ouledali, S., Ennajeh, M., Ferrandino, A., Khemira, H., Schubert, A., and Secchi, F. (2019). Influence of arbuscular mycorrhizal fungi inoculation on the control of stomata functioning by abscisic acid (ABA) in drought-stressed olive plants. South African J. Bot. 121, 152–158. doi: 10.1016/j.sajb.2018.10.024
Ouledali, S., Ennajeh, M., Zrig, A., Gianinazzi, S., and Khemira, H. (2018). Estimating the contribution of arbuscular mycorrhizal fungi to drought tolerance of potted olive trees (Olea europaea). Acta Physiol. Plant 40:81. doi: 10.1007/s11738-018-2656-1
Parihar, M., Rakshit, A., Meena, V. S., Gupta, V. K., Rana, K., Choudhary, M., et al. (2020). The potential of arbuscular mycorrhizal fungi in C cycling: a review. Arch. Microbiol. 202, 1581–1596. doi: 10.1007/s00203-020-01915-x
Pellegrino, E., Öpik, M., Bonari, E., and Ercoli, L. (2015). Responses of wheat to arbuscular mycorrhizal fungi: a meta-analysis of field studies from 1975 to 2013. Soil Biol Biochem. 84, 210–217. doi: 10.1016/j.soilbio.2015.02.020
Pereyra, M. A., Garcia, P., Colabelli, M. N., Barassi, C. A., and Creus, C. M. (2012). A better water status in wheat seedlings induced by Azospirillum under osmotic stress is related to morphological changes in xylem vessels of the coleoptile. Appl. Soil Ecol. 53, 94–97. doi: 10.1016/j.apsoil.2011.11.007
Placide, R., Christian, C. S., and Rony, S. (2012). Development of in vitro technique to screen for drought tolerant banana varieties by sorbitol induced osmotic stress. Afr. J. Plant Sci. 6, 16–425. doi: 10.5897/ajps12.101
Porcel, R., Aroca, R., Azcón, R., and Ruiz-Lozano, J. M. (2006). PIP aquaporin gene expression in arbuscular mycorrhizal glycine max and lactuca sativa plants in relation to drought stress tolerance. Plant Mol. Biol. 60, 389–404. doi: 10.1007/s11103-005-4210-y
Porcel, R., Azcón, R., and Ruiz-Lozano, J. M. (2004). Evaluation of the role of genes encoding for Δ1-pyrroline-5-carboxylate synthetase (P5CS) during drought stress in arbuscular mycorrhizal Glycine max and Lactuca sativa plants. Physiol. Mol. Plant Pathol. 65, 211–221. doi: 10.1016/j.pmpp.2005.02.003
Porcel, R., Zamarreño, ÁM., García-Mina, J. M., and Aroca, R. (2014). Involvement of plant endogenous ABA in Bacillus megaterium PGPR activity in tomato plants. BMC Plant Biol. 14:36. doi: 10.1186/1471-2229-14-36
Poudel, R., Jumpponen, A., Kennelly, M. M., Rivard, C., Gomez-Montano, L., and Garrett, K. A. (2019). Integration of system phenotypes in microbiome networks to identify candidate synthetic communities: a study of the grafted tomato rhizobiome. bioRxiv [Preprint] doi: 10.1101/2019.12.12.874966
Poudel, R., Jumpponen, A., Schlatter, D. C., Paulitz, T. C., Gardener, B. B., Kinkel, L. L., et al. (2016). Microbiome networks: a systems framework for identifying candidate microbial assemblages for disease management. Phytopathology 106, 1083–1096. doi: 10.1094/PHYTO-02-16-0058-FI
Püschel, D., Bitterlich, M., Rydlová, J., and Jansa, J. (2020). Facilitation of plant water uptake by an arbuscular mycorrhizal fungus: a Gordian knot of roots and hyphae. Mycorrhiza 30, 299–313. doi: 10.1007/s00572-020-00949-9
Püschel, D., Bitterlich, M., Rydlová, J., and Jansa, J. (2021). Drought accentuates the role of mycorrhiza in phosphorus uptake. Soil Biol. Biochem. 157:108243. doi: 10.1016/j.soilbio.2021.108243
Querejeta, J. I. (2017). Soil Water Retention and Availability as Influenced by Mycorrhizal Symbiosis: Consequences for Individual Plants, Communities, and Ecosystems. Johnson NC: Elsevier.
Querejeta, J. I., Egerton-Warburton, L. M., Prieto, I., Vargas, R., and Allen, M. F. (2012). Changes in soil hyphal abundance and viability can alter the patterns of hydraulic redistribution by plant roots. Plant Soil 355, 63–73. doi: 10.1007/s11104-011-1080-8
Quiroga, G., Erice, G., Aroca, R., Chaumont, F., and Ruiz-Lozano, J. M. (2017). Enhanced drought stress tolerance by the arbuscular mycorrhizal symbiosis in a drought-sensitive maize cultivar is related to a broader and differential regulation of host plant aquaporins than in a drought-tolerant cultivar. Front. Plant Sci. 8:1056. doi: 10.3389/fpls.2017.01056
Raheem, A., Shaposhnikov, A., Belimov, A. A., Dodd, I. C., and Ali, B. (2018). Auxin production by rhizobacteria was associated with improved yield of wheat (Triticum aestivum L.) under drought stress. Arch. Agron. Soil Sci. 64, 574–587. doi: 10.1080/03650340.2017.1362105
Rapparini, F., and Peñuelas, J. (2014). “Mycorrhizal fungi to alleviate drought stress on plant growth,” in Use of Microbes for the Alleviation of Soil Stresses, ed. M. Miransari (New York, NY: Springer), 21–42.
Ren, A. T., Zhu, Y., Chen, Y. L., Ren, H. X., Li, J. Y., Abbott, L. K., et al. (2019). Arbuscular mycorrhizal fungus alters root-sourced signal (abscisic acid) for better drought acclimation in Zea mays L. seedlings. EEB 167:103824. doi: 10.1016/j.envexpbot.2019.103824
Rillig, M. C., and Mummey, D. L. (2006). Mycorrhizas and soil structure. New Phytol. 171, 41–53. doi: 10.1111/j.1469-8137.2006.01750.x
Rillig, M., Wright, S., Nichols, K. A., Schmidt, W. F., and Torn, M. S. (2001). Large contribution of arbuscular mycorrhizal fungi to soil carbon pools in tropical forest soils. Plant Soil 233, 166–167. doi: 10.1023/A:1010364221169
Rolli, E., Marasco, R., Vigani, G., Ettoumi, B., Mapelli, F., Deangelis, M. L., et al. (2015). Improved plant resistance to drought is promoted by the root-associated microbiome as a water stress-dependent trait. Environ. Microbiol. 17, 316–331. doi: 10.1111/1462-2920.12439
Roossinck, M. J. (2011). The good viruses: viral mutualistic symbioses. Nat. Rev. Microbiol. 9, 99–108. doi: 10.1038/nrmicro2491
Ruiz-Lozano, J. M. (2003). Arbuscular mycorrhizal symbiosis and alleviation of osmotic stress. New perspectives for molecular studies. Mycorrhiza 13, 309–317. doi: 10.1007/s00572-003-0237-6
Ruiz-Lozano, J. M., Aroca, R., Zamarreño, ÁM., Molina, S., Andreo-Jiménez, B., Porcel, R., et al. (2016). Arbuscular mycorrhizal symbiosis induces strigolactone biosynthesis under drought and improves drought tolerance in lettuce and tomato. Plant Cell Environ. 39, 441–452. doi: 10.1111/pce.12631
Ruíz-Sánchez, M., Armada, E., Muñoz, Y., de Salamone, I. E. G., Aroca, R., Ruiz-Lozano, J. M., et al. (2011). Azospirillum and arbuscular mycorrhizal colonization enhance rice growth and physiological traits under well-watered and drought conditions. J. Plant Physiol. 168, 1031–1037. doi: 10.1016/j.jplph.2010.12.019
Ruth, B., Khalvati, M., and Schmidhalter, U. (2011). Quantification of mycorrhizal water uptake via high-resolution on-line water content sensors. Plant Soil 342, 459–468. doi: 10.1007/s11104-010-0709-3
Sah, S. K., Reddy, K. R., and Li, J. (2016). Abscisic acid and abiotic stress tolerance in crop plants. Front. Plant Sci. 7:571. doi: 10.3389/fpls.2016.00571
Saleem, A. R., Brunetti, C., Khalid, A., Della Rocca, G., Raio, A., Emiliani, G., et al. (2018). Drought response of Mucuna pruriens (L.) DC. inoculated with ACC deaminase and IAA producing rhizobacteria. PLoS One 13:e0191218. doi: 10.1371/journal.pone.0191218
Sandhya, V., Ali, S. Z., Grover, M., Reddy, G., and Venkateswarlu, B. (2010). Effect of plant growth promoting Pseudomonas spp. on compatible solutes, antioxidant status and plant growth of maize under drought stress. Plant Growth Regul. 62, 21–30. doi: 10.1007/s10725-010-9479-4
Sarma, R. K., and Saikia, R. (2014). Alleviation of drought stress in mung bean by strain Pseudomonas aeruginosa GGRJ21. Plant Soil 377, 111–126. doi: 10.1007/s11104-013-1981-9
Scudeletti, D., Crusciol, C. A. C., Bossolani, J. W., Moretti, L. G., Momesso, L., Servaz Tubaña, B., et al. (2021). Trichoderma asperellum inoculation as a tool for attenuating drought stress in sugarcane. Front. Plant Sci. 12:570. doi: 10.3389/fpls.2021.645542
Selvakumar, G., Panneerselvam, P., and Ganeshamurthy, A. N. (2012). “Bacterial mediated alleviation of abiotic stress in crops,” in Bacteria in Agrobiology: Stress Management, ed. D. K. Maheshwari (Heidelberg: Springer), 205–224.
Seo, S. Y., Kim, Y. J., and Park, K. Y. (2019). Increasing polyamine contents enhances the stress tolerance via reinforcement of antioxidative properties. Front. Plant Sci. 10:1331. doi: 10.3389/fpls.2019.01331
Sharma, K., Gupta, S., Thokchom, S. D., Jangir, P., and Kapoor, R. (2021). Arbuscular mycorrhiza-mediated regulation of polyamines and aquaporins during abiotic stress: deep insights on the recondite players. Front. Plant Sci. 12:1072. doi: 10.3389/fpls.2021.642101
Sharma, P., Khanna, V., and Kumari, P. (2013). Efficacy of aminocyclopropane-1-carboxylic acid (ACC)-deaminase-producing rhizobacteria in ameliorating water stress in chickpea under axenic conditions. Afr. J. Microbiol. Res. 7, 5749–5757. doi: 10.5897/AJMR2013.5918
Shintu, P. V., and Jayaram, K. M. (2015). Phosphate solubilising bacteria (Bacillus polymyxa) -an effective approach to mitigate drought in tomato (Lycopersicon esculentum Mill.). Trop. Plant Res. 2, 17–22.
Shoresh, M., Harman, G. E., and Mastouri, F. (2010). Induced systemic resistance and plant responses to fungal biocontrol agents. Ann. Rev. Phytopathol. 48, 21–43. doi: 10.1146/annurev-phyto-073009-114450
Smith, S. E., Facelli, E., Pope, S., and Andrew Smith, F. (2010). Plant performance in stressful environments: interpreting new and established knowledge of the roles of arbuscular mycorrhizas. Plant Soil 326, 3–20. doi: 10.1007/s11104-009-9981-5
Snijders, T. A. B. (2002). Markov chain monte carlo estimation of exponential random graph models. J. Soc. Struct. 3, 1–40.
Sohrabi, Y., Heidari, G., Weisany, W., Golezani, K. G., and Mohammadi, K. (2012). Changes of antioxidative enzymes, lipid peroxidation and chlorophyll content in chickpea types colonized by different Glomus species under drought stress. Symbiosis 56, 5–18. doi: 10.1007/s13199-012-0152-8
Strullu-Derrien, C., Selosse, M.-A., Kenrick, P., and Martin, F. M. (2018). The origin and evolution of mycorrhizal symbioses: from palaeomycology to phylogenomics. New Phytol. 220, 1012–1030. doi: 10.1111/nph.15076
Su, Y.-H., Liu, Y.-B., and Zhang, X.-S. (2011). Auxin–cytokinin interaction regulates meristem development. Mol. Plant 4, 616–625. doi: 10.1093/mp/ssr007
Sukweenadhi, J., Kim, Y.-J., Choi, E.-S., Koh, S.-C., Lee, S.-W., Kim, Y.-J., et al. (2015). Paenibacillus yonginensis DCY84T induces changes in Arabidopsis thaliana gene expression against aluminum, drought, and salt stress. Microbiol. Res. 172, 7–15. doi: 10.1016/j.micres.2015.01.007
Talaat, N. B., and Shawky, B. T. (2013). Modulation of nutrient acquisition and polyamine pool in salt-stressed wheat (Triticum aestivum L.) plants inoculated with arbuscular mycorrhizal fungi. Acta Physiol. Plant 3, 2601–2610. doi: 10.1007/s11738-013-1295-9
Talaat, N. B., and Shawky, B. T. (2015). “Plant-microbe interaction and salt stress tolerance in plants,” in Managing Salt Tolerance In Plants: Molecular And Genomic Perspectives, eds M. A. Hossain and S. H. Wani (Boca Raton, MA: Press/Taylor & Francis Group), 267–289.
Terra, W. C., Campos, V. P., Martins, S. J., Costa, L. S. A. S., da Silva, J. C. P., Barros, A. F., et al. (2018). Volatile organic molecules from Fusarium oxysporum 21 with nematicidal activity against Meloidogyne incognita. Crop Prot. 106, 125–131. doi: 10.1016/j.cropro.2017.12.022
Thirkell, T. J., Charters, M. D., Elliott, A. J., Sait, S. M., and Field, K. J. (2017). Are mycorrhizal fungi our sustainable saviours? Considerations for achieving food security. J. Ecol. 105, 921–929. doi: 10.1111/1365-2745.12788
Timmusk, S., Abd El-Daim, I. A., Copolovici, L., Tanilas, T., Kännaste, A., Behers, L., et al. (2014). Drought-tolerance of wheat improved by rhizosphere bacteria from harsh environments: enhanced biomass production and reduced emissions of stress volatiles. PLoS One 9:e96086. doi: 10.1371/journal.pone.0096086
Timmusk, S., Timmusk, K., and Behers, L. (2013). Rhizobacterial plant drought stress tolerance enhancement: towards sustainable water resource management and food security. J. Food Secur. 1, 6–9. doi: 10.12691/jfs-1-1-2
Tobar, R., Azcon, R., and Barea, J. M. (1994). Improved nitrogen uptake and transport from 15N-labelled nitrate by external hyphae of arbuscular mycorrhiza under water-stressed conditions. New Phytol. 126, 119–122. doi: 10.1111/j.1469-8137.1994.tb07536.x
Topçuoğlu, B. D., Lesniak, N. A., Ruffin, M. T. IV, Wiens, J., and Schloss, P. D. (2020). A framework for effective application of machine learning to microbiome-based classification problems. mBio 11, 1–19. doi: 10.1128/mBio.00434-20
Tripathi, P., Rabara, R. C., Reese, R. N., Miller, M. A., Rohila, J. S., Subramanian, S., et al. (2016). A toolbox of genes, proteins, metabolites and promoters for improving drought tolerance in soybean includes the metabolite coumestrol and stomatal development genes. BMC Genomics 17:102. doi: 10.1186/s12864-016-2420-0
Ullah, A., Akbar, A., and Luo, Q. (2019). Microbiome diversity in cotton rhizosphere under normal and drought conditions. Microb. Ecol. 77, 429–439. doi: 10.1007/s00248-018-1260-7
Vaishnav, A., and Choudhary, D. K. (2019). Regulation of drought-responsive gene expression in Glycine max L. merrill is mediated through Pseudomonas simiae strain AU. J. Plant Growth Regul. 38, 333–342. doi: 10.1007/s00344-018-9846-3
Vargas, L., Santa Brigida, A. B., Mota Filho, J. P., De Carvalho, T. G., Rojas, C. A., Vaneechoutte, D., et al. (2014). Drought tolerance conferred to sugarcane by association with Gluconacetobacter diazotrophicus: a transcriptomic view of hormone pathways. PLoS One 9:e114744. doi: 10.1371/journal.pone.0114744
Vílchez, J. I., García-Fontana, C., Román-Naranjo, D., González-López, J., and Manzanera, M. (2016). Plant drought tolerance enhancement by trehalose production of desiccation-tolerant microorganisms. Front. Microbiol. 7:1577. doi: 10.3389/fmicb.2016.01577
Vuosku, J., Muilu-Mäkelä, R., Avia, K., Suokas, M., Kestilä, J., Läärä, E., et al. (2019). Thermospermine synthase (ACL5) and diamine oxidase (DAO) expression is needed for zygotic embryogenesis and vascular development in scots pine. Front. Plant Sci. 10:1600. doi: 10.3389/fpls.2019.01600
Vurukonda, S. S. K. P., Vardharajula, S., Shrivastava, M., and SkZ, A. (2016). Multifunctional Pseudomonas putida strain FBKV2 from arid rhizosphere soil and its growth promotional effects on maize under drought stress. Rhizosphere 1, 4–13. doi: 10.1016/j.rhisph.2016.07.005
Wang, C. J., Yang, W., Wang, C., Gu, C., Niu, D. D., Liu, H. X., et al. (2012). Induction of drought tolerance in cucumber plants by a consortium of three plant growth-promoting rhizobacterium strains. PLoS One 7:e52565. doi: 10.1371/journal.pone.0052565
Wang, C., Wang, C., Gao, Y.-L., Wang, Y.-P., and Gao, J.-H. (2016). A consortium of three plant growth-promoting rhizobacterium strains acclimates lycopersicon esculentum and confers a better tolerance to chilling stress. J. Plant Growth Regul. 35, 54–64. doi: 10.1007/s00344-015-9506-9
Westwood, J. H., Mccann, L., Naish, M., Dixon, H., Murphy, A. M., Stancombe, M. A., et al. (2013). A viral RNA silencing suppressor interferes with abscisic acid-mediated signaling and induces drought tolerance in Arabidopsis thaliana. Mol. Plant Pathol. 14, 158–170. doi: 10.1111/j.1364-3703.2012.00840.x
Wilson, G. W. T., Rice, C. W., Rillig, M. C., Springer, A., and Hartnett, D. C. (2009). Soil aggregation and carbon sequestration are tightly correlated with the abundance of arbuscular mycorrhizal fungi: results from long-term field experiments. Ecol. Lett. 12, 452–461. doi: 10.1111/j.1461-0248.2009.01303.x
Wu, H.-H., Zou, Y.-N., Rahman, M. M., Ni, Q.-D., and Wu, Q.-S. (2017). Mycorrhizas alter sucrose and proline metabolism in trifoliate orange exposed to drought stress. Sci. Rep. 7:42389. doi: 10.1038/srep42389
Wu, Q. S., He, J. D., Srivastava, A. K., Zou, Y. N., and Kuča, K. (2019). Mycorrhizas enhance drought tolerance of citrus by altering root fatty acid compositions and their saturation levels. Tree Physiol. 39, 1149–1158. doi: 10.1093/treephys/tpz039
Wu, Q.-S., He, X.-H., Zou, Y.-N., Liu, C. Y., and Xiao, J. (2012). Arbuscular mycorrhizas alter root system architecture of Citrus tangerine through regulating metabolism of endogenous polyamines. Plant. Growth Regul. 68, 27–35. doi: 10.1007/s10725-012-9690-6
Wu, Q.-S., Liu, C.-Y., Zhang, D.-J., Zou, Y. N., He, X.-H., and Wu, Q.-H. (2016). Mycorrhiza alters the profile of root hairs in trifoliate orange. Mycorrhiza 26, 237–247. doi: 10.1007/s00572-015-0666-z
Wu, Q.-S., Zou, Y.-N., and Fathi Abd-Allah, E. (2014b). “Mycorrhizal association and ROS in plants,” in Oxidative Damage to Plants, ed. P. Ahmad (Cambridge, MA: Academic Press), 453–475. doi: 10.1016/B978-0-12-799963-0.00015-0
Wu, Q.-S., Cao, M.-Q., Zou, Y.-N., and He, X. (2014a). Direct and indirect effects of glomalin, mycorrhizal hyphae and roots on aggregate stability in rhizosphere of trifoliate orange. Sci. Rep. 4:5823. doi: 10.1038/srep05823
Xie, W., Hao, Z., Zhou, X., Jiang, X., Xu, L., Wu, S., et al. (2018). Arbuscular mycorrhiza facilitates the accumulation of glycyrrhizin and liquiritin in Glycyrrhiza uralensis under drought stress. Mycorrhiza 28, 285–300. doi: 10.1007/s00572-018-0827-y
Xu, L., and Coleman-Derr, D. (2019). Causes and consequences of a conserved bacterial root microbiome response to drought stress. Curr. Opin. Microbiol. 49, 1–6. doi: 10.1016/j.mib.2019.07.003
Xu, L., Naylor, D., Dong, Z., Simmons, T., Pierroz, G., Hixson, K. K., et al. (2018). Drought delays development of the sorghum root microbiome and enriches for monoderm bacteria (2018). Proc. Natl. Acad. Sci. U.S.A. 115, E4284–E4293. doi: 10.1073/pnas.1717308115
Xu, P., Chen, F., Mannas, J. P., Feldman, T., Sumner, L. W., and Roossinck, M. J. (2008). Virus infection improves drought tolerance. New Phytol. 180, 911–921. doi: 10.1111/j.1469-8137.2008.02627.x
Yadav, S. N., Singh, A. K., Peter, J. K., Masih, H., Benjamin, J. C., Singh, D. K., et al. (2018). Study of exopolysaccharide containing PGPR on growth of okra plant under water stress conditions. Int. J. Curr. Microbiol. Appl. Sci. 7, 3337–3374. doi: 10.20546/ijcmas.2018.711.385
Yang, J., Kloepper, J. W., and Ryu, C. M. (2009). Rhizosphere bacteria help plants tolerate abiotic stress. Trends Plant Sci. 14, 1–4. doi: 10.1016/j.tplants.2008.10.004
Yooyongwech, S., Phaukinsang, N., Cha-um, S., and Supaibulwatana, K. (2013). Arbuscular mycorrhiza improved growth performance in Macadamia tetraphylla L. grown under water deficit stress involves soluble sugar and proline accumulation. Plant Growth Regul. 69, 285–293. doi: 10.1007/s10725-012-9771-6
Zafar-ul-Hye, M., Danish, S., Abbas, M., Ahmad, M., and Munir, T. M. (2019). ACC deaminase producing PGPR Bacillus amyloliquefaciens and Agrobacterium fabrum along with biochar improve wheat productivity under drought stress. Agronomy 9:343. doi: 10.3390/agronomy9070343
Zargar, S. M., Nargar, P., Deshmukh, R., Nazir, M., Wani, A. A., Masoodi, K. Z., et al. (2017). Aquaporins as potential drought tolerance inducing proteins: towards instigating stress tolerance. J. Proteomics 169, 233–238. doi: 10.1016/j.jprot.2017.04.010
Zhang, F., Zou, Y. N., and Wu, Q. S. (2018). Quantitative estimation of water uptake by mycorrhizal extraradical hyphae in citrus under drought stress. Sci. Hortic. 229, 132–136. doi: 10.1016/j.scienta.2017.10.038
Zhang, F., Zou, Y. N., Wu, Q. S., and Kuča, K. (2020). Arbuscular mycorrhizas modulate root polyamine metabolism to enhance drought tolerance of trifoliate orange. EEB 171:103962. doi: 10.1016/j.envexpbot.2019.103926
Zhang, H., Murzello, C., Sun, Y., Kim, M.-S., Xie, X., Jeter, R. M., et al. (2010). Choline and osmotic-stress tolerance induced in Arabidopsis by the soil microbe Bacillus subtilis (GB03). MPMI 23, 1097–1104. doi: 10.1094/MPMI-23-8-1097
Zhang, H., Wei, S., Hu, W., Xiao, L., and Tang, M. (2017). Arbuscular mycorrhizal fungus Rhizophagus irregularis increased potassium content and expression of genes encoding potassium channels in Lycium barbarum. Front. Plant Sci. 8:440. doi: 10.3389/fpls.2017.00440
Zhang, S., Lehmann, A., Zheng, W., You, Z., and Rillig, M. C. (2019). Arbuscular mycorrhizal fungi increase grain yields: a meta-analysis. New Phytol. 222, 543–555. doi: 10.1111/nph.15570
Zhang, T., Hu, Y., Zhang, K., Tian, C., and Guo, J. (2018). Arbuscular mycorrhizal fungi improve plant growth of Ricinus communis by altering photosynthetic properties and increasing pigments under drought and salt stress. Ind. Crops Prod. 117, 13–19. doi: 10.1016/j.indcrop.2018.02.087
Zhang, Y. F., Wang, P., Bi, Q., Zhang, Z. H., and Yang, Y. F. (2016). Effects of arbuscular mycorrhizal fungi on the growth of Leymus chinensis under saline stress of different intensities. Acta Ecol. Sin. 36, 5467–5476. doi: 10.1016/j.scitotenv.2016.10.091
Ziane, H., Meddad-Hamza, A., Beddiar, A., and Gianinazzi, S. (2017). Effects of arbuscular mycorrhizal fungi and fertilization levels on industrial tomato growth and production. Int. J. Agric. Biol. 19, 341–347. doi: 10.17957/IJAB/15.0287
Zou, Y. N., Wu, H. H., Giri, B., Wu, Q. S., and Kuča, K. (2019). Mycorrhizal symbiosis down-regulates or does not change root aquaporin expression in trifoliate orange under drought stress. Plant Physiol. Biochem. 144, 292–299. doi: 10.1016/j.plaphy.2019.10.001
Keywords: microbiome, climate change, food security, plant–microbiome interaction, phytobiome, AMF, PGPR, Arabidopsis
Citation: Poudel M, Mendes R, Costa LAS, Bueno CG, Meng Y, Folimonova SY, Garrett KA and Martins SJ (2021) The Role of Plant-Associated Bacteria, Fungi, and Viruses in Drought Stress Mitigation. Front. Microbiol. 12:743512. doi: 10.3389/fmicb.2021.743512
Received: 18 July 2021; Accepted: 20 September 2021;
Published: 25 October 2021.
Edited by:
Valentina Fiorilli, University of Turin, ItalyReviewed by:
Dilfuza Egamberdieva, Leibniz Center for Agricultural Landscape Research (ZALF), GermanyErika Banchio, National University of Río Cuarto, Argentina
Qiang-Sheng Wu, Yangtze University, China
Copyright © 2021 Poudel, Mendes, Costa, Bueno, Meng, Folimonova, Garrett and Martins. This is an open-access article distributed under the terms of the Creative Commons Attribution License (CC BY). The use, distribution or reproduction in other forums is permitted, provided the original author(s) and the copyright owner(s) are credited and that the original publication in this journal is cited, in accordance with accepted academic practice. No use, distribution or reproduction is permitted which does not comply with these terms.
*Correspondence: Samuel J. Martins, c2oubWFydGluc0B1ZmwuZWR1