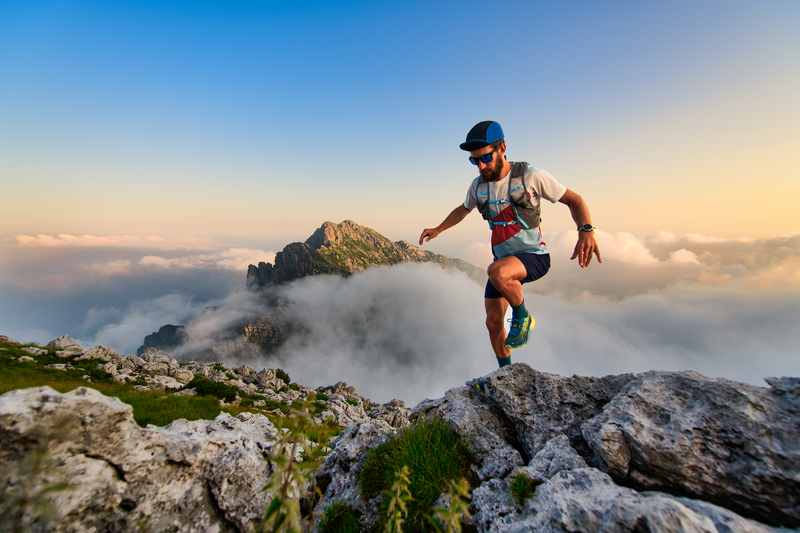
94% of researchers rate our articles as excellent or good
Learn more about the work of our research integrity team to safeguard the quality of each article we publish.
Find out more
ORIGINAL RESEARCH article
Front. Microbiol. , 17 December 2021
Sec. Antimicrobials, Resistance and Chemotherapy
Volume 12 - 2021 | https://doi.org/10.3389/fmicb.2021.732932
This article is part of the Research Topic Molecular Characterization of Clinically Important Gram-Negative Bacteria Recovered from the Environment: Antimicrobial Resistance, Virulence and Epidemiology View all 8 articles
In this work, we characterized a novel chromosome-encoded AmpC β-lactamase gene, blaPRC–1, in an isolate of a newly classified Pseudomonas species designated Pseudomonas wenzhouensis A20, which was isolated from sewage discharged from an animal farm in Wenzhou, China. Susceptibility testing, molecular cloning, and enzyme kinetic parameter analysis were performed to determine the function and enzymatic properties of the β-lactamase. Sequencing and comparative genomic analysis were conducted to clarify the phylogenetic relationship and genetic context of the blaPRC–1 gene. PRC-1 is a 379-amino acid AmpC β-lactamase with a molecular weight of 41.48 kDa and a predicted pI of 6.44, sharing the highest amino acid identity (57.7%) with the functionally characterized AmpC β-lactamase PDC-211 (ARX71249). blaPRC–1 confers resistance to many β-lactam antibiotics, including penicillins (penicillin G, amoxicillin, and amoxicillin-clavulanic acid) and cephalosporins (cefazolin, ceftriaxone, and cefotaxime). The kinetic properties of PRC-1 were compatible with those of a typical class C β-lactamase showing hydrolytic activities against β-lactam antibiotics, and the hydrolytic activity was strongly inhibited by avibactam. The genetic context of blaPRC–1 was relatively conserved, and no mobile genetic element was predicted in its surrounding region. Identification of a novel β-lactamase gene in an unusual environmental bacterium reveals that there might be numerous unknown resistance mechanisms in bacterial populations, which may pose potential risks to human health due to universal horizontal gene transfer between microorganisms. It is therefore of great value to carry out extensive research on the mechanism of antibiotic resistance.
The genus Pseudomonas, first described by Professor Mikula in 1894, is one of the most common bacteria in the world and has been found in various natural environments, human clinical specimens, and infected plants (Peix et al., 2009). Pseudomonas is a diverse and complex genus with the largest number of known species (Lalucat et al., 2020). At present, 396 species and 21 subspecies of Pseudomonas are included in the List of Prokaryotic Names with Standing in Nomenclature (Parte, 2014). The genus Pseudomonas mainly includes species that are pathogenic to animals and humans (Pseudomonas aeruginosa), insects (Pseudomonas entomophila), and plants (Pseudomonas syringae); species that are plant commensals (Pseudomonas stutzeri and Pseudomonas fluorescens); and species used in bioremediation (Pseudomonas putida) (De Smet et al., 2017). Extensively studied and economically important species are the human opportunistic pathogen P. aeruginosa and the plant pathogen P. syringae (Silby et al., 2011). P. aeruginosa is the most common cause of infection among non-fermenting gram-negative bacteria, which mainly affects patients with weakened immune function (Behzadi et al., 2021).
β-lactamase is an enzyme that can hydrolyze the β-lactam ring and inactivate antibiotics before binding to penicillin-binding proteins (Li et al., 2007). The AmpC enzyme is a type of β-lactamase mediated by chromosomes or plasmids in most Enterobacteriaceae and non-fermenting species, such as P. aeruginosa. AmpC belongs to Class C β-lactamase in the Ambler molecular structure classification and group I in the Bush-Jacoby-Medeiros functional classification of β-lactamases (Bush et al., 1995). AmpC β-lactamases are able to confer resistance to most penicillins and cephalosporins and cannot be inhibited by normal β-lactamase inhibitors, such as clavulanic acid and tazobactam (Bush et al., 1995; Livermore, 1995), but it can be strongly inhibited by avibactam (Docquier and Mangani, 2018). AmpC β-lactamases are usually not expressed or are underexpressed in E. coli because of a weak promoter and a transcriptional attenuator preceding the ampC gene; however, due to mutations in β-lactamases or induction by specific β-lactams, these enzymes can be expressed at high levels and consequently increase the minimum inhibitory concentrations (MICs) of β-lactams (Fisher and Mobashery, 2014; Juan et al., 2017). This effect is attributed to specific spontaneous mutations in the promoter or attenuation consensus sequence of the ampC gene (Haenni et al., 2014). It is worth noting that the diversity caused by mutations and the overexpression caused by antimicrobial induction of these enzymes are evolving to confer resistance to various β-lactam antibiotics. Therefore, the development of new antibiotics and novel antibacterial therapeutic strategies is urgently needed (Bonomo, 2017).
In this study, based on the complete genome sequencing of an isolate of a newly classified Pseudomonas species, Pseudomonas wenzhouensis A20, we characterized a novel chromosome-encoded AmpC β-lactamase, designated PRC-1, and analyzed the kinetic parameters of the β-lactamase. Discovering a novel resistance gene in an environmental bacterium provides valuable information for the treatment of infectious diseases.
The strain P. wenzhouensis A20 carrying the novel β-lactamase gene blaPRC–1 was isolated from sewage discharged from an animal farm in Wenzhou, China. It was initially identified using a bioMérieux VITEK 2 Compact Instrument (BioMérieux, Marcy L’etoile, France). Further species identification was carried out by 16S ribosomal RNA (rRNA) gene sequencing and finally verified using average nucleotide identity (ANI) (Lachance et al., 2020). The bacterial strains and plasmids used in this work are listed in Table 1.
Minimum inhibitory concentrations were determined with the standard agar dilution method on Mueller-Hinton (MH) agar according to the Clinical and Laboratory Standards Institute guidelines (CLSI, 2020). The MICs of β-lactam inhibitors were determined by broth microdilution in cation-adjusted Mueller-Hinton broth (CAMHB). The MIC was interpreted as the β-lactam concentration at which bacterial growth was no longer observed after 20 h of incubation at 37°C. The MICs of ampicillin-avibactam and ampicillin-tazobactam were determined at a constant concentration of 4 mg/L avibactam and tazobactam, and in combination with a series of increasing concentrations of ampicillin, while for amoxicillin-clavulanic acid, a constant concentration ratio (2:1, amoxicillin:clavulanic acid) was applied. MICs for the tested antibiotics were interpreted according to the guidelines of the CLSI (2020); however, for some antibiotics without CLSI interpretation criteria for P. aeruginosa, breakpoints for Enterobacteriaceae in the CLSI guidelines were used as a reference. E. coli ATCC 25922 was used as a quality control strain. Values are the means of three independent measures.
A Generay Genomic DNA Miniprep Kit (Shanghai Generay Biotech Co., Ltd., Shanghai, China) was used to extract the total bacterial DNA of P. wenzhouensis A20. Genomic DNA was sequenced by both the Illumina HiSeq-2500 and PacBio RS II platforms by Shanghai Personal Biotechnology Co., Ltd. (Shanghai, China). The PacBio long reads were initially assembled by Canu v1.8 (Koren et al., 2017), and hybrid assembly was subsequently conducted using Unicycler v0.4.8 (Wick et al., 2017), with the contigs generated by Canu and all the sequenced reads (including short and long reads) serving as an input. The cyclization of the whole-genome assembly was confirmed through the built-in tools of Unicycler. BWA v0.7.12 (Li and Durbin, 2009) and Genome Analysis Toolkit (McKenna et al., 2010) were used for short read alignment to the draft of the whole-genome assembly to improve assembly quality. Open reading frames (ORFs) were predicted using Prokka v1.14.6 (Seemann, 2014) with default parameters and annotated by the BLAST program with an e-value threshold of 1e-5 against the non-redundant protein sequence (NR) database of the National Center for Biotechnology Information (NCBI) and the UniProt/Swiss-Prot database. Resistance genes were identified using a combination of the ResFinder database (Zankari et al., 2012) and the Comprehensive Antibiotic Resistance Database (CARD) (McArthur et al., 2013). Mobile genetic elements (MGEs) were detected using ISFinder (Siguier et al., 2006) and INTEGRALL (Moura et al., 2009) with default parameters. FastANI v1.31 (Jain et al., 2018) was used to calculate the ANI. ProtParam1 was used to predict the molecular weight and pI value of PRC-1. The putative signal peptide cleavage site of PRC-1 was predicted by SignalP 5.0 (Almagro Armenteros et al., 2019). Circular maps of the genome and other relatives were drawn using CGView Server (Petkau et al., 2010). Multiple sequence alignments of PRC-1 and other PDC family β-lactamases were performed using MAFFT v7.475 (Katoh and Standley, 2013). A neighbor-joining phylogenetic tree including PRC-1 and other proteins sharing ≥85% amino acid sequence similarity was reconstructed using MEGAX (Kumar et al., 2018). The resulting tree was visualized using the online tool iTol (Letunic and Bork, 2007). Other bioinformatics tools in this study were applied with Python and Biopython scripts (Cock et al., 2009).
The nucleotide sequence of the blaPRC–1 gene with the upstream promoter region was amplified using PrimeSTAR HS DNA Polymerase (Takara Bio Inc., Dalian, China), and P. wenzhouensis A20 genomic DNA was used as the template. The primers with restriction endonuclease sites (BamHI and HindIII for the forward and reverse primers, respectively) are listed in Supplementary Table 1. The PCR product and the cloning vector pUCP24 were digested with both BamHI and HindIII (Takara Bio Inc.). The resulting DNA fragments were ligated with T4 DNA ligase (Takara Bio Inc.), and the ligated product was transformed into E. coli DH5α with the calcium chloride method. The transformants were selected on LB agar plates supplemented with gentamicin (40 μg/mL). Single colonies were inoculated into LB medium supplemented with the same antibiotics and cultured overnight. Plasmids were extracted from the cultures using a Plasmid Mini Extraction Kit (Generay Biotech Co., Ltd.), and the inserts were verified by PCR and further by DNA sequencing (TsingKe, Shanghai, China). The same method was used to clone the ORF of blaPRC–1 without the signal sequence into the pCold I vector (the primers are listed in Supplementary Table 1), and the recombinant plasmid (pCold I-blaPRC–1) was transformed into competent E. coli BL21 cells. The transformants were selected on LB agar plates supplemented with 100 μg/mL ampicillin. For the expression of PRC-1, the recombinant strain (pCold I-blaPRC–1/BL21) was cultured overnight in LB medium, diluted 100-fold in fresh medium, and then incubated at 16°C and 250 rpm for 2–3 h, which was followed by the addition of 0.5 mM isopropyl-β-d-thiogalactopyranoside (IPTG, Sigma Chemicals Co., St. Louis, MO, United States) when the OD600 reached 0.6 and further incubation for 21 h at 16°C (Choi and Geletu, 2018). His-tagged PRC-1 protein was purified by nickel affinity chromatography with BeyoGold His-tag purification resin (Beyotime, Shanghai, China) and then digested with Enterokinase (GenScript, Nanjing, China) for 40 h at 37°C to remove the His-tag. The protein was identified by SDS-PAGE using a 12% acrylamide separation gel and Coomassie blue G-250 staining.
Kinetic parameters of hydrolysis for β-lactams by the novel β-lactamase PRC-1 were determined on a UV-VIS spectrophotometer (U-3900, HITACHI, Japan) at 37°C in 10 mM phosphate buffer (pH 7.4) in a final reaction volume of 200 μl. The steady-state kinetic parameters (kcat and Km) were determined by non-linear regression of the initial reaction rates with the Michaelis-Menten equation in Prism (version 8.0.2) software (GraphPad Software, CA, United States) (Chen et al., 2019). For poor substrates (cefepime, imipenem, and aztreonam), the tested antibiotics were treated as competitive inhibitors of the AmpC enzyme and nitrocefin as a reporter substrate, and the inhibition constants were determined by observing the apparent Km at various concentrations of inhibitor. Data were analyzed to obtain the values of Vmax and Km by GraphPad Prism with the competitive model inhibition/non-linear regression curve fit method. kcat values were determined from the initial rates calculated at saturating substrate concentrations (Faheem et al., 2013). The concentrations of the β-lactamase inhibitors avibactam and clavulanic acid leading to a 50% reduction in hydrolysis of nitrocefin (IC50) were measured after 5 min of preincubation of the enzymes with the inhibitors at 37°C and nitrocefin as the substrate at 100 μM. The IC50 values were determined by non-linear regression analysis (GraphPad Prism, version 8.0.2) using log (inhibitor) vs. response – (three parameters) (Chen et al., 2019). Values are the means of three independent measures.
The chromosome and blaPRC–1 gene sequences of P. wenzhouensis A20 have been deposited in GenBank under accession numbers CP072610 and MW854031, respectively.
The strain P. wenzhouensis A20 was isolated from sewage discharged from an animal farm in Wenzhou, China. 16S rRNA homologous gene analysis demonstrated that P. wenzhouensis A20 showed the closest relationship with the strain Pseudomonas hydrolytica DSWY01 (NR_170428.1), at 98.50% identity and 100% coverage. The taxonomy of the genus Pseudomonas was developed mainly through phenotypic and biochemical properties (Palleroni, 2010), whereas currently, molecular analysis, as a good supplement, is particularly important. According to previous studies on the phylogeny of Pseudomonas based on 16S ribosomal DNA (rDNA) sequences, there was not enough discrimination at the species level (Mulet et al., 2010), and the multilocus sequence analysis (MLSA) approach based on the four housekeeping genes (the 16S rRNA, gyrB, rpoB, and rpoD genes) has subsequently been applied, which provided better results than the former method but could not accurately discriminate the phylogeny of Pseudomonas species (Mulet et al., 2010). At present, the new gold standards for species delineation are digital whole-genome comparisons by using genome-to-genome distance calculations (GGDCs) or ANI (Lalucat et al., 2020). According to ANI, the threshold to classify the species of a certain bacterium is a cutoff score of ≥95% between the unclassified bacterium genome and all the bacterial genomes available in the public databases. Given the large number of genomes (∼26,600) from 303 Pseudomonas species available in the NCBI database, one genome sequence from each of 40 species which showed the highest similarities of 16S rRNA gene sequences with that of A20 was chosen to perform ANI analysis. Among them, the highest ANI value of 94.25% was found between the A20 genome and that of Pseudomonas mendocina EF27 (GCF_008041835.1) (Supplementary Table 3), indicating that strain A20 might represent a novel species of the genus Pseudomonas. Moreover, when submitting the genome sequence to GenBank of NCBI, the ANI results calculated by the scientists there also did not identify a species for A20, which further confirmed it to be a novel species.
The P. wenzhouensis A20 genome consists of a circular chromosome and does not have any plasmid. The chromosome is 4.4 Mb in length with an average GC content of 62.2% and encodes 4,063 ORFs (Table 2). Screening for A20-homologous genomes (>80% nucleotide sequence identity and >30% coverage) in the NCBI nucleotide database showed that most of the close relatives were derived from 20 species. Comparative genomic analysis revealed that the chromosome of P. wenzhouensis A20 shared the highest sequence similarities with those of Pseudomonas sihuiensis KCTC 32246 chromosome (LT629797.1; 80.83% coverage and 89.54% identity), Pseudomonas alcaliphila JAB1 chromosome (CP016162.1; 80.78% coverage and 89.19% identity), and P. mendocina S5 (CP013124.1; 79.02% coverage and 88.59% identity). Moreover, A20 is basically similar to other species in conserved backbone sequences (Figure 1), among them, similar sequences are represented by lines of different shades, whereas the blank regions indicate differences among A20 and other species.
Figure 1. Comparative chromosome map of the P. wenzhouensis A20 and 20 other Pseudomonas strains using A20 as the reference. Circle 1 (from inside to outside) shows the scale in kb. Circles 2 and 3 show the GC content and GC skew, respectively. Circles 4–25 are the regions homologous to A20 and the other 20 strains, respectively. Similar parts are represented by lines of different shades, and the regions without similar hits leave blank. The strains used for comparison in this study are listed in Supplementary Table 2.
Antibiotic susceptibility testing showed that A20 exhibited intermediate resistance to nalidixic acid. P. wenzhouensis A20 had the highest MIC levels for fosfomycin (>512 μg/mL), cefazolin (256 μg/mL), and cefoxitin (128 μg/mL) and higher MIC levels for aztreonam (32 μg/mL) and cefotaxime (8 μg/mL) (Table 3). As the breakpoints for fosfomycin, cefazolin, cefoxitin, aztreonam, and cefotaxime were not available for P. aeruginosa in CLSI interpretation criteria, the breakpoints for Enterobacteriaceae in the CLSI guidelines were referred to, and the MIC values of P. wenzhouensis A20 were equivalent to those of resistant enterobacteria for these five antimicrobial agents. The isolate was susceptible to some third- and fourth-generation cephalosporins (e.g., ceftazidime, cefoperazone, cefoselis, and cefepime) and carbapenems (such as meropenem). When analyzing the resistance mechanism of the bacterium, especially for β-lactam antibiotics, we found that only one predicted ampC β-lactamase gene was annotated within the whole genome, which showed an identity of more than 50% with the functionally characterized resistance gene blaPDC–211 (57.4%, MF281075.1). The predicted gene was then cloned, and the resistance function was further determined.
Table 3. Minimum inhibitory concentrations of antimicrobials for P. wenzhouensis A20, the recombinants, and the control strain (μg/mL).
To determine the resistance characteristics of blaPRC–1 to β-lactam antibiotics, the coding sequence of blaPRC–1 together with its upstream promoter region was amplified and cloned into the pUCP24 vector and then transformed into E. coli DH5α. The results revealed that blaPRC–1 conferred resistance to some penicillins and first- and third-generation cephalosporins (Table 3). Compared with the control strains (DH5α and pUCP24/DH5α), the recombinant strain (pUCP24-blaPRC–1/DH5α) exhibited increased MIC levels for ampicillin, cefotaxime, and amoxicillin by 8-, 8-, and 32-fold, respectively. The MICs of ceftriaxone, cefazolin, penicillin G, and cefotaxime exhibited a lower increase of 4-fold. However, the recombinant strain did not show any MIC level changes for carbapenems or monobactams. The activity of PRC-1 was poorly inhibited by classical class A β-lactamase inhibitors such as clavulanic acid and tazobactam, while a significant decrease in resistance to ampicillin was observed in the presence of avibactam.
Multiple sequence alignment of the deduced amino acid sequence of PRC-1 with those of functionally characterized β-lactamases revealed that PRC-1 had identities of 57.7, 57.5, 57.3, 57.1, 57.0, 56.8, and 56.8% with PDC-211, PDC-241, PDC-7, PDC-68, PDC-3, PDC-1, and PDC-315, respectively. The deduced amino acid sequence carries the characteristic catalytic residues of the serine active site of β-lactamases, including motifs of S-X-X-K (serine-isoleucine-serine-lysine) with the initial amino acid sequence number at positions 64–67, Y-S-N (tryptophan-serine-asparagine) at positions 150–152, and K-T-G (lysine-threonine-glycine) at positions 315–317 (Figure 2).
Figure 2. Multiple alignment of the deduced amino acid sequences of PRC-1 and other chromosomal class C β-lactamases. Three conserved motifs are shaded in black. Identical amino acids are indicated by dots, and the absence of amino acids at those positions is shown with hyphens. The numbering of PRC-1 is based on a standard numbering scheme for class C β-lactamases (Mack et al., 2020). The sequences and their accession numbers are PDC-211 (ARX71249), PDC-241 (AUT06978), PDC-7 (ACQ82812), PDC-68 (AIG20005), PDC-3 (ACQ82808), PDC-1 (AAM08945), and PDC-315 (AYF58375).
Because no detailed resistance spectrum is available for blaPDC–211, blaPDC–241, blaPDC–7, and blaPDC–68, which shared relatively higher amino acid sequence identities with PRC-1, we compared the resistance profile of blaPDC–3 with that of blaPRC–1. PDC-3 (ACQ82808) is a chromosomal AmpC β-lactamase of P. aeruginosa with an amino acid identity of 57.0% (216/379) with PRC-1. Unlike blaPDC–3, blaPRC–1 exhibited a relatively narrow resistance spectrum and did not confer resistance to piperacillin, cefepime, ceftazidime, or even aztreonam. In addition, blaPRC–1 showed lower MIC values than blaPDC–3 against other β-lactam antibiotics (Barnes et al., 2018). Additionally, we found that the blaPRC–1 gene did not show any resistance to cefoxitin but showed a relatively lower MIC level for cefazolin, even though P. wenzhouensis A20 showed much higher MIC levels for them. This finding may indicate the existence of unknown resistance mechanisms within P. wenzhouensis A20.
The novel β-lactamase gene blaPRC–1 is 1140 bp in size and encodes a 379-amino acid putative protein. The mature protein has a predicted molecular weight of 41.48 kDa and a predicted pI of 6.44. The secretory precursor peptide, which consists of 22 amino acids, in PRC-1 is defined by alanine residues at positions 22 and 23. The molecular weight of the protein without the signal peptide is 39.07 kDa. The purified protein PRC-1 exhibited a single band on SDS-PAGE, and its molecular size was in agreement with the predicted one. The kinetic parameters of PRC-1 were determined by measuring the rates of catalysis for various β-lactam antibiotics at different substrate concentrations. The results demonstrated that PRC-1 was a typical cephalosporinase with a high kcat and strong hydrolytic activity (kcat/Km ratios were 220 × 10–2 μM–1⋅s–1) for the first-generation cephalosporin cefazolin. PRC-1 showed moderate hydrolysis activities against some third-generation cephalosporins (cefotaxime and ceftriaxone) and penicillins (ampicillin and benzylpenicillin) but very poor hydrolytic activity against ceftazidime (the third-generation cephalosporin) (Table 4). However, the result of the enzyme kinetic hydrolytic activity test was not completely consistent with the MIC level change of the recombinant strain (pUCP24-blaPRC–1/DH5α) in the antimicrobial susceptibility test (Table 3). For example, PRC-1 showed hydrolytic activity against ceftazidime, but the recombinant strain carrying blaPRC–1 did not exhibit a significant change in the MIC of ceftazidime compared with that for the control bacteria. This result may be attributed to its low activity in vitro. A similar case in a previous study reported that BAT-2 and BSU-2 exhibited slight hydrolytic activities against ampicillin, but the two genes did not show detectable resistance activities to ampicillin in the recombinant strains carrying them (Toth et al., 2016). We also found that the catalytic efficiency (kcat/Km) of ceftazidime was the lowest among all the tested substrates. Moreover, the affinity of poor substrates, such as aztreonam, cefepime, or imipenem, was higher than those of other substrates in the competitive inhibition test with nitrocefin; the Ki values for aztreonam, cefepime, and imipenem were (307 ± 21) × 10–3, (134 ± 20) × 10–3, and (72 ± 7) × 10–3 μM, respectively, and no hydrolysis was determined for these substrates at saturating concentrations (Table 4), which was in line with the susceptibility test results. Conversely, PDC-3, which shares 57.0% global amino acid identity with PRC-1, exhibited hydrolytic activities against cefepime and imipenem (Rodriguez-Martinez et al., 2009). Both β-lactamases showed similar catalytic efficiencies for benzylpenicillin, whereas PRC-1 displayed higher Km and kcat values, revealing that higher turnover numbers are compensated by higher Km values (Table 4). The IC50 (50% inhibitory concentration) of β-lactamase inhibitors showed that avibactam (IC50: 0.0003922 μM) has a strong inhibitory effect on PRC-1, while clavulanic acid (IC50: 23.43 μM) has a weaker inhibitory effect. This result is in line with the properties of β-lactam inhibitors for AmpC enzymes.
To analyze the possible origin of blaPRC–1, a total of 40 predicted proteins with an amino acid similarity ≥85% were retrieved from the NCBI-nr database, and all of them were from the genus Pseudomonas. The phylogenetic tree showed that PRC-1 was closest to a putative AmpC β-lactamase found in P. mendocina (WP_147810921.1), and they shared the highest amino acid similarity (91.29% identity and 100% coverage) (Figure 3). These findings indicate the importance of Pseudomonas as a reservoir for PRC-1-like relatives, and additional Pseudomonas genomes must be sequenced to find proteins with higher identities with PRC-1.
Figure 3. Phylogenetic analysis of PRC-1 with other putative class C β-lactamases (≥85% amino acid similarity). PRC-1 from this study is represented with a red dot.
To analyze the genetic environments of blaPRC–1 and its relatives, nine sequences of approximately 20 kb in length with blaPRC–1-like genes at the center were retrieved from the NCBI nucleotide database, and these blaPRC–1-like genes shared over 85% amino acid similarities with PRC-1. No mobile genetic element was predicted in its surrounding area. Comparative genomic analysis of the 10 sequences (including the one from this work, P. wenzhouensis A20) revealed that the upstream regions (from folD to acoR) of the blaPRC–1 and blaPRC–1-like (ampC) genes of all 10 sequences have a conserved structure in terms of gene context and gene order. Three sequences (P. mendocina S5, P. mendocina 5, and Pseudomonas sp. B11D7D) have the most similar structure to the sequence from this study, except two additional genes (dmlR and bdcA) downstream of the blaPRC–1-like (ampC) genes that were found in these three sequences. Three sequences (P. mendocina NEB698, P. mendocina NK-01, and P. mendocina strain NCTC 10897) had an extra two or more genes upstream of the blaPRC–1-like (ampC) genes. The remaining three sequences had the same (P. sediminis B10D7D) or nearly the same (P. alcaliphila JAB1 and P. sihuiensis 32246) gene context as that of the one from this work in the regions downstream of blaPRC–1; however, the upstream regions of these three sequences were totally different from P. wenzhouensis A20. All 10 sequences were from the genus Pseudomonas, and the three sequences with the most similarity with P. wenzhouensis A20 were from P. mendocina (P. mendocina S5 and P. mendocina CPS5) and an unclassified Pseudomonas (P. sp. B11D7D) (Figure 4). These results suggested that the gene context of blaPRC–1 and its relatives is conserved in species of the genus Pseudomonas.
Figure 4. Comparative genomics analysis of the genetic context of blaPRC–1 with the sequences carrying their homologous genes. The direction of genes is shown via an arrow. The blaPRC–1 gene and putative ampC (blaPRC–1-like) genes are colored in red, and the other genes are colored based on gene function classification. The predicted hypothetical genes are in gray. The sequences and their accession numbers are as follows: P. alcaliphila JAB1 chromosome (CP016162.1), P. sihuiensis 32246 chromosome (LT629797.1), P. sediminis B10D7D chromosome (CP060009.1), P. mendocina S5.2 chromosome (CP013124.1), P. mendocina CPS5 chromosome (CP060288.1), Pseudomonas sp. B11D7D chromosome (CP060008.1), P. mendocina NEB698 chromosome (CP027657.1), P. mendocina NK-01 chromosome (CP002620.1), and P. mendocina NCTC 10897 chromosome (LR134290.1).
In this work, we characterized a novel AmpC β-lactamase-encoding gene, blaPRC–1, in the chromosome of P. wenzhouensis A20, an isolate of a newly classified species of the Pseudomonas genus. blaPRC–1 shared the highest amino acid similarity (57.7%) with the functionally characterized AmpC enzyme PDC-211 from P. aeruginosa and conferred resistance to β-lactam antibiotics, including some cephalosporins, such as cefazolin, ceftriaxone, and cefotaxime. Similar to other AmpC β-lactamases, the novel β-lactamase is strongly inhibited by avibactam, but inhibitors of class A enzymes such as clavulanic acid have a weaker inhibitory effect against it. blaPRC–1-like genes with amino acid similarities of more than 85% were identified in many bacteria of different species, and further research would be carried out to determine the functions of these potential resistance genes. Deciphering more antibacterial resistance mechanisms will be crucial to assist clinics in using effective antibiotics to treat infections caused by unusual pathogens.
The datasets presented in this study can be found in online repositories. The names of the repository/repositories and accession number(s) can be found in the article/Supplementary Material.
HZ, MZ, and QB: conception and design of the study. JLL, XL, WS, MG, and PR: acquisition of data. PZ, XD, KZ, JLL, CF, XL, and KL: data analysis and interpretation. PZ, XD, KZ, JWL, QB, and HZ: drafting of manuscript. PZ, XD, WS, MG, QL, and XZ: performed the experiments. All authors contributed to the article and approved the submitted version.
This study was supported by the Science & Technology Project of Wenzhou City, China (2020Y0358 and N20210001), Zhejiang Provincial Natural Science Foundation of China (LY19C060002 and LQ17H190001), the National Natural Science Foundation of China (81973382), and the Special Project for Significant New Drug Research and Development in the Major National Science and Technology Projects of China (2020ZX09201002).
The authors declare that the research was conducted in the absence of any commercial or financial relationships that could be construed as a potential conflict of interest.
All claims expressed in this article are solely those of the authors and do not necessarily represent those of their affiliated organizations, or those of the publisher, the editors and the reviewers. Any product that may be evaluated in this article, or claim that may be made by its manufacturer, is not guaranteed or endorsed by the publisher.
The authors would like to acknowledge all study participants and individuals who contributed to this study.
The Supplementary Material for this article can be found online at: https://www.frontiersin.org/articles/10.3389/fmicb.2021.732932/full#supplementary-material
Almagro Armenteros, J. J., Tsirigos, K. D., Sonderby, C. K., Petersen, T. N., Winther, O., Brunak, S., et al. (2019). SignalP 5.0 improves signal peptide predictions using deep neural networks. Nat. Biotechnol. 37, 420–423. doi: 10.1038/s41587-019-0036-z
Barnes, M. D., Taracila, M. A., Rutter, J. D., Bethel, C. R., Galdadas, I., Hujer, A. M., et al. (2018). Deciphering the evolution of cephalosporin resistance to ceftolozane-tazobactam in Pseudomonas aeruginosa. mBio 9:e02085-18. doi: 10.1128/mBio.02085-18
Behzadi, P., Barath, Z., and Gajdacs, M. (2021). It’s not easy being green: a narrative review on the microbiology, virulence and therapeutic prospects of multidrug-resistant Pseudomonas aeruginosa. Antibiotics (Basel) 10:42. doi: 10.3390/antibiotics10010042
Bonomo, R. A. (2017). β-lactamases: a focus on current challenges. Cold Spring Harb. Perspect. Med. 7:a025239. doi: 10.1101/cshperspect.a025239
Bush, K., Jacoby, G. A., and Medeiros, A. A. (1995). A functional classification scheme for β-lactamases and its correlation with molecular structure. Antimicrob. Agents Chemother. 39, 1211–1233. doi: 10.1128/AAC.39.6.1211
Chen, Q., Zhou, W., Qian, C., Shen, K., Zhu, X., Zhou, D., et al. (2019). OXA-830, a novel chromosomally encoded extended-spectrum class D β-lactamase in Aeromonas simiae. Front. Microbiol. 10:2732. doi: 10.3389/fmicb.2019.02732
Choi, T. J., and Geletu, T. T. (2018). High level expression and purification of recombinant flounder growth hormone in E. coli. J. Genet. Eng. Biotechnol. 16, 347–355. doi: 10.1016/j.jgeb.2018.03.006
CLSI (2020). Performance Standards for Antimicrobial Susceptibility Testing, CLSI Supplement M100, 30th Edn. Wayne, PA: Clinical and Laboratory Standards Institute.
Cock, P. J., Antao, T., Chang, J. T., Chapman, B. A., Cox, C. J., Dalke, A., et al. (2009). Biopython: freely available Python tools for computational molecular biology and bioinformatics. Bioinformatics 25, 1422–1423. doi: 10.1093/bioinformatics/btp163
De Smet, J., Hendrix, H., Blasdel, B. G., Danis-Wlodarczyk, K., and Lavigne, R. (2017). Pseudomonas predators: understanding and exploiting phage-host interactions. Nat. Rev. Microbiol. 15, 517–530. doi: 10.1038/nrmicro.2017.61
Docquier, J. D., and Mangani, S. (2018). An update on β-lactamase inhibitor discovery and development. Drug Resist. Updat. 36, 13–29. doi: 10.1016/j.drup.2017.11.002
Faheem, M., Rehman, M. T., Danishuddin, M., and Khan, A. U. (2013). Biochemical characterization of CTX-M-15 from Enterobacter cloacae and designing a novel non-β-lactam-β-lactamase inhibitor. PLoS One 8:e56926. doi: 10.1371/journal.pone.0056926
Fisher, J. F., and Mobashery, S. (2014). The sentinel role of peptidoglycan recycling in the β-lactam resistance of the Gram-negative Enterobacteriaceae and Pseudomonas aeruginosa. Bioorg. Chem. 56, 41–48. doi: 10.1016/j.bioorg.2014.05.011
Haenni, M., Chatre, P., and Madec, J. Y. (2014). Emergence of Escherichia coli producing extended-spectrum AmpC β-lactamases (ESAC) in animals. Front. Microbiol. 5:53. doi: 10.3389/fmicb.2014.00053
Jain, C., Rodriguez, R. L., Phillippy, A. M., Konstantinidis, K. T., and Aluru, S. (2018). High throughput ANI analysis of 90K prokaryotic genomes reveals clear species boundaries. Nat. Commun. 9:5114. doi: 10.1038/s41467-018-07641-9
Juan, C., Torrens, G., Gonzalez-Nicolau, M., and Oliver, A. (2017). Diversity and regulation of intrinsic β-lactamases from non-fermenting and other Gram-negative opportunistic pathogens. FEMS Microbiol. Rev. 41, 781–815. doi: 10.1093/femsre/fux043
Katoh, K., and Standley, D. M. (2013). MAFFT multiple sequence alignment software version 7: improvements in performance and usability. Mol. Biol. Evol. 30, 772–780. doi: 10.1093/molbev/mst010
Koren, S., Walenz, B. P., Berlin, K., Miller, J. R., Bergman, N. H., and Phillippy, A. M. (2017). Canu: scalable and accurate long-read assembly via adaptive k-mer weighting and repeat separation. Genome Res. 27, 722–736. doi: 10.1101/gr.215087.116
Kumar, S., Stecher, G., Li, M., Knyaz, C., and Tamura, K. (2018). MEGA X: molecular evolutionary genetics analysis across computing platforms. Mol. Biol. Evol. 35, 1547–1549. doi: 10.1093/molbev/msy096
Lachance, M. A., Lee, D. K., and Hsiang, T. (2020). Delineating yeast species with genome average nucleotide identity: a calibration of ANI with haplontic, heterothallic Metschnikowia species. Antonie Van Leeuwenhoek 113, 2097–2106. doi: 10.1007/s10482-020-01480-9
Lalucat, J., Mulet, M., Gomila, M., and Garcia-Valdes, E. (2020). Genomics in bacterial taxonomy: impact on the genus Pseudomonas. Genes (Basel) 11:139. doi: 10.3390/genes11020139
Letunic, I., and Bork, P. (2007). Interactive Tree Of Life (iTOL): an online tool for phylogenetic tree display and annotation. Bioinformatics 23, 127–128. doi: 10.1093/bioinformatics/btl529
Li, H., and Durbin, R. (2009). Fast and accurate short read alignment with Burrows-Wheeler transform. Bioinformatics 25, 1754–1760. doi: 10.1093/bioinformatics/btp324
Li, X. Z., Mehrotra, M., Ghimire, S., and Adewoye, L. (2007). β-Lactam resistance and β-lactamases in bacteria of animal origin. Vet. Microbiol. 121, 197–214. doi: 10.1016/j.vetmic.2007.01.015
Livermore, D. M. (1995). β-lactamases in laboratory and clinical resistance. Clin. Microbiol. Rev. 8, 557–584. doi: 10.1128/CMR.8.4.557
Mack, A. R., Barnes, M. D., Taracila, M. A., Hujer, A. M., Hujer, K. M., Cabot, G., et al. (2020). A standard numbering scheme for class C β-lactamases. Antimicrob. Agents Chemother. 64:e02247-19. doi: 10.1128/AAC.01841-19
McArthur, A. G., Waglechner, N., Nizam, F., Yan, A., Azad, M. A., Baylay, A. J., et al. (2013). The comprehensive antibiotic resistance database. Antimicrob. Agents Chemother. 57, 3348–3357. doi: 10.1128/AAC.00419-13
McKenna, A., Hanna, M., Banks, E., Sivachenko, A., Cibulskis, K., Kernytsky, A., et al. (2010). The genome analysis toolkit: a MapReduce framework for analyzing next-generation DNA sequencing data. Genome Res. 20, 1297–1303. doi: 10.1101/gr.107524.110
Moura, A., Soares, M., Pereira, C., Leitao, N., Henriques, I., and Correia, A. (2009). INTEGRALL: a database and search engine for integrons, integrases and gene cassettes. Bioinformatics 25, 1096–1098. doi: 10.1093/bioinformatics/btp105
Mulet, M., Lalucat, J., and Garcia-Valdes, E. (2010). DNA sequence-based analysis of the Pseudomonas species. Environ. Microbiol. 12, 1513–1530.
Palleroni, N. J. (2010). The Pseudomonas story. Environ. Microbiol. 12, 1377–1383. doi: 10.1111/j.1462-2920.2009.02041.x
Parte, A. C. (2014). LPSN–list of prokaryotic names with standing in nomenclature. Nucleic Acids Res 42, D613–D616. doi: 10.1093/nar/gkt1111
Peix, A., Ramirez-Bahena, M. H., and Velazquez, E. (2009). Historical evolution and current status of the taxonomy of genus Pseudomonas. Infect. Genet. Evol. 9, 1132–1147. doi: 10.1016/j.meegid.2009.08.001
Petkau, A., Stuart-Edwards, M., Stothard, P., and Van Domselaar, G. (2010). Interactive microbial genome visualization with GView. Bioinformatics 26, 3125–3126. doi: 10.1093/bioinformatics/btq588
Rodriguez-Martinez, J. M., Poirel, L., and Nordmann, P. (2009). Extended-spectrum cephalosporinases in Pseudomonas aeruginosa. Antimicrob. Agents Chemother. 53, 1766–1771. doi: 10.1128/AAC.01410-08
Seemann, T. (2014). Prokka: rapid prokaryotic genome annotation. Bioinformatics 30, 2068–2069. doi: 10.1093/bioinformatics/btu153
Siguier, P., Perochon, J., Lestrade, L., Mahillon, J., and Chandler, M. (2006). ISfinder: the reference centre for bacterial insertion sequences. Nucleic Acids Res. 34, D32–D36. doi: 10.1093/nar/gkj014
Silby, M. W., Winstanley, C., Godfrey, S. A., Levy, S. B., and Jackson, R. W. (2011). Pseudomonas genomes: diverse and adaptable. FEMS Microbiol. Rev. 35, 652–680. doi: 10.1111/j.1574-6976.2011.00269.x
Toth, M., Antunes, N. T., Stewart, N. K., Frase, H., Bhattacharya, M., Smith, C. A., et al. (2016). Class D β-lactamases do exist in Gram-positive bacteria. Nat. Chem. Biol. 12, 9–14. doi: 10.1038/nchembio.1950
Wick, R. R., Judd, L. M., Gorrie, C. L., and Holt, K. E. (2017). Unicycler: resolving bacterial genome assemblies from short and long sequencing reads. PLoS Comput. Biol. 13:e1005595. doi: 10.1371/journal.pcbi.1005595
Keywords: PRC-1, β-lactamase, Pseudomonas, AmpC, kinetic analysis, resistance
Citation: Zhang P, Dong X, Zhou K, Zhu T, Liang J, Shi W, Gao M, Feng C, Li Q, Zhang X, Ren P, Lu J, Lin X, Li K, Zhu M, Bao Q and Zhang H (2021) Characterization of a Novel Chromosome-Encoded AmpC β-Lactamase Gene, blaPRC–1, in an Isolate of a Newly Classified Pseudomonas Species, Pseudomonas wenzhouensis A20, From Animal Farm Sewage. Front. Microbiol. 12:732932. doi: 10.3389/fmicb.2021.732932
Received: 29 June 2021; Accepted: 12 November 2021;
Published: 17 December 2021.
Edited by:
Gerson Nakazato, State University of Londrina, BrazilReviewed by:
Marisa Fabiana Nicolás, National Laboratory for Scientific Computing (LNCC), BrazilCopyright © 2021 Zhang, Dong, Zhou, Zhu, Liang, Shi, Gao, Feng, Li, Zhang, Ren, Lu, Lin, Li, Zhu, Bao and Zhang. This is an open-access article distributed under the terms of the Creative Commons Attribution License (CC BY). The use, distribution or reproduction in other forums is permitted, provided the original author(s) and the copyright owner(s) are credited and that the original publication in this journal is cited, in accordance with accepted academic practice. No use, distribution or reproduction is permitted which does not comply with these terms.
*Correspondence: Mei Zhu, emh1bWVpX2RAMTYzLmNvbQ==; Qiyu Bao, YmFvcXlAZ2Vub21pY3MuY24=; Hailin Zhang, emhsd3o5N0Bob3RtYWlsLmNvbQ==
†These authors have contributed equally to this work
Disclaimer: All claims expressed in this article are solely those of the authors and do not necessarily represent those of their affiliated organizations, or those of the publisher, the editors and the reviewers. Any product that may be evaluated in this article or claim that may be made by its manufacturer is not guaranteed or endorsed by the publisher.
Research integrity at Frontiers
Learn more about the work of our research integrity team to safeguard the quality of each article we publish.