- 1Department of Life Sciences, University of Modena and Reggio Emilia, Modena, Italy
- 2Biogest-Siteia, University of Modena and Reggio Emilia, Modena, Italy
- 3Department of Molecular Medicine, University of Padova, Padova, Italy
Twelve strains of Leuconostoc carnosum from meat products were investigated in terms of biochemical, physiological, and functional properties. The spectrum of sugars fermented by L. carnosum strains was limited to few mono- and disaccharides, consistently with the natural habitats of the species, including meat and fermented vegetables. The strains were able to grow from 4 to 37°C with an optimum of approximately 32.5°C. The ability to grow at temperatures compatible with refrigeration and in presence of up to 60 g/L NaCl explains the high loads of L. carnosum frequently described in many meat-based products. Six strains produced exopolysaccharides, causing a ropy phenotype of colonies, according to the potential involvement on L. carnosum in the appearance of slime in packed meat products. On the other side, the study provides evidence of a potential protective role of L. carnosum WC0321 and L. carnosum WC0323 against Listeria monocytogenes, consistently with the presence in these strains of the genes encoding leucocin B. Some meat-based products intended to be consumed without cooking may harbor up to 108 CFU/g of L. carnosum; therefore, we investigated the potential impact of this load on health. No strains survived the treatment with simulated gastric juice. Three selected strains were challenged for the capability to colonize a mouse model and their immunomodulatory properties were investigated. The strains did not colonize the intestine of mice during 10 days of daily dietary administration. Intriguingly, despite the loss of viability during the gastrointestinal transit, the strains exhibited different immunomodulatory effect on the maturation of dendritic cells in vivo, the extent of which correlated to the production of exopolysaccharides. The ability to stimulate the mucosal associated immune system in such probiotic-like manner, the general absence of antibiotic resistance genes, and the lack of the biosynthetic pathways for biogenic amines should reassure on the safety of this species, with potential for exploitation of selected starters.
Introduction
The genus Leuconostoc encompasses heterofermentative Lactobacillales sharing facultative anaerobiosis, intrinsic vancomycin resistance, catalase negativity, ovococcoid morphology, and dextran production (Björkroth et al., 2014). Carbohydrates are catabolized through pentose phosphate and phosphoketolase pathway, yielding lactic acid, CO2, and ethanol and/or acetic acid (Candeliere et al., 2021). The ratio between the amounts of ethanol and acetic acid depends on the ability of the microorganism to re-oxidize the NADH generated in the early stages of the process along with the energy requirements.
Leuconostoc carnosum frequently occurs in the microbiota of foods, mostly meat-based products (Shaw and Harding, 1989; Samelis et al., 2000; Goto et al., 2004; Raimondi et al., 2018; Raimondi et al., 2019), but bacteria belonging to this species have been isolated from processed vegetables as well (Hong et al., 2014; Jung et al., 2014). It is highly adapted to grow in meat environments where it can outcompete undesired microorganisms and reach high levels of viable bacteria (Raimondi et al., 2018, 2019). For instance, L. carnosum tend to dominate the microbiota of vacuum-packaged or modified atmosphere packaged (MAP) meat products, appearing in the first days after packaging and reaching remarkably high loads (in the order of 108 CFU/g) toward the end of the shelf life, when they dominate the microbiota (Björkroth et al., 1998; Vasilopoulos et al., 2010; Raimondi et al., 2019). Other meat products colonized by L. carnosum are sausages, vacuum-packaged smoked bacon, and sliced cooked poultry (Geeraerts et al., 2018; Li et al., 2019).
The high load of Leuconostoc carnosum in several foods emphasizes the need of a deeper investigation of this species, taking also into account the controversial role in spoilage and in biopreservation. Indeed, L. carnosum has been claimed as responsible of meat deterioration by souring, discoloration, gas production, and slime formation (Shaw and Harding, 1989; Björkroth et al., 1998; Samelis et al., 2006; Raimondi et al., 2019). Likewise other lactic acid bacteria (LAB) in food, members of the genus Leucosnostoc take part to a wide range of metabolic processes affecting the taste, flavor, and sensorial properties, such as the fermentation of carbohydrates into organic acids, the metabolism of amino acids, citrate, polysaccharides, polyols, and aldehydes, and the hydrolysis of glycosides, proteins, and lipids (Hemme and Foucaud-Scheunemann, 2004; Ammor and Mayo, 2007; Morandi et al., 2013). Moreover, the release of organic acids and hydrogen peroxide, which exert an intrinsic antimicrobial effect, and production of bacteriocins concur to food preservation and has been well documented in LAB and Leuconostoc (Ammor and Mayo, 2007; Morandi et al., 2013).
Based on genomic annotation, most of the strains of Leuconostoc carnosum have the genes for bacteriocin synthesis, secretion, and immunity, including the production of leucocin B, a class IIc bacteriocin effective against L. monocytogenes (Felix et al., 1994; Candeliere et al., 2021). Comparative genomics revealed that L. carnosum consist of a compact group of closely related bacteria sharing most of the metabolic features (Candeliere et al., 2021). Adaptation to a nitrogen-rich environment such as meat is consistent with a number of peptidase genes in the core genome and by the auxotrophy for nitrogen compounds including some amino acids, vitamins, and cofactors. Interestingly, L. carnosum genome does not harbor genes for biogenic amines production nor genetic determinants for antibiotic resistances. Although genomics of L. carnosum have been investigated, the metabolic activities of this species can be only partially deduced from the genome and additional biochemical efforts are required to evaluate the phenotypic potential of strains that possibly impact on sensorial features of foods and that can present potential industrial interest. Moreover, information on the potential effect of this species on health is still lacking, especially considering that remarkably high counts of live L. carnosum are ingested with the consumption of certain ready-to-use meat-based foods.
In this study, the phenotypic diversity of 12 strains of Leuconostoc carnosum was thoroughly investigated, including metabolic, technological, and health-related properties. All the tested strains have been genome sequenced (BioProject accession number PRJNA542256) and annotated and were isolated from MAP cooked ham or from fresh sausages (Candeliere et al., 2020, 2021). In vitro experiments aimed to assess the role of L. carnosum in food spoilage and/or biopreservation. The growth kinetics at different temperatures, the resistance to oxidative, and osmotic stress, the substrate preferences, the proteolytic, and lipolytic capabilities, the ability to yield biogenic amines, and exopolysaccharides, the susceptibility to antibiotics, and the production of anti-Listeria bacteriocins were investigated. For the first time, an animal trial was carried out to assess whether L. carnosum has potential to reach and colonize the intestine of mice and to exert some effect on the immune system.
Materials and Methods
Strains, Media, and Culture Conditions
All chemicals were purchased from Sigma-Aldrich (St. Louis, MO, United States) unless otherwise stated. The strains of Leuconostoc carnosum, all originating from MAP sausage or cooked ham (Raimondi et al., 2018, 2019), were obtained from our laboratory collection. The strains were routinely cultured for 48 h at 30°C in static tubes of deMan, Rogosa, and Sharpe broth (Lactobacilli MRS Broth; BD Difco, Sparks, MD, United States), hereinafter referred to as MRS.
Cultures were incubated at 4, 8, 15, 23, 30, 37, and 42°C to determine the effect of temperature on growth kinetic and yield. Turbidity at 600 nm (OD600) was used to evaluate growth. OD600 data from the exponential tract of the growth curve were used to calculate the specific growth rate (μmax). Optimum, minimal, and maximal growth temperature (Topt, Tmin, and Tmax) were deduced with the Sym’Previus software1 fitting in a secondary growth model the μmax data as a function of temperature (Rosso et al., 1993).
Tolerance to Osmotic and Oxidative Stress
Tolerance of Leuconostoc carnosum strains to osmotic and oxidative stress was tested in MRS broth supplemented with increasing concentrations of NaCl (0, 20, 40, 60, 80, and 100 g/L) and H2O2 (0, 0.0625, 0.125, 0.25, 0.5, and 0.75 g/L), respectively (Montanari et al., 2018; Fessard and Remize, 2019). The cultures were seeded (5% v/v) with 24-h MRS cultures and incubated at 30°C for 48 h, then the OD600 was measured.
Biochemical Characterization
The strains were tested for the fermentation of 49 carbohydrates using API 50 CH test strips (bioMerieux, Marcy-l’Etoile, France), according to the manufacturer’s instructions. Briefly, the bacterial biomass from the surface of MRS-agar plates was used to create a suspension with a turbidity equivalent to 2 McFarland in 10 ml of API 50 CHL Medium. The suspension was used to inoculate each tube of the strip. Anaerobiosis was obtained in the inoculated cupules by using sterile paraffin oil. The results were read after 48 h of incubation at 30°C.
A modified formulation of MRS, where glucose was replaced by 5 g/L arginine (hereinafter referred to as MRS-arg), was used to evaluate arginine utilization. MRS-arg cultures, firstly seeded (5% v/v) with 24-h MRS cultures, were incubated for 24 h and propagated six times in the same medium. Then, OD600 was measured and compared with that achieved by control MRS cultures.
Citrate fermentation was evaluated in citrate medium (Milliere et al., 1989), containing the following: 4.5 g/L citric acid, 10 g/L proteose peptone (no. 3; BD Difco), 5 g/L yeast extract, 2 g/L K2HPO4, 5 g/L sodium acetate ⋅ 3H2O, 1 g/L Tween 80, and 0.2 g/L MgSO4⋅7H2O. Cultures in citrate medium, firstly seeded (5% v/v) with 24-h MRS cultures, were incubated for 48 h and propagated six times in the same medium. Then, OD600 was measured and compared with that achieved by control cultures containing glucose.
Proteolytic and Lipolytic Activity
Proteolytic activity was assayed in MRS-agar plates supplemented with 30 g/L gelatin (BD Difco) (Smith and Goodner, 1958). Spots of 5 μl of grown cultures of Leuconostoc carnosum were deposited onto the surface of the plates. After 72 h of incubation at 30°C, the plates were placed at 4°C for 5 h, then the diameter of the hydrolysis halo around the spots was measured.
Lipolytic activity was assayed in a MRS-agar medium containing triglyceride emulsions. It was composed of 900 ml of MRS, supplemented with 20 ml of 1 g/L rhodamine solution and an emulsion of 50 ml ddH2O, 30 ml of seed oil, and 150 μl of Tween 80. Rhodamine solution and the oil emulsion were sterilized separately (i.e., filtered at 0.22 μm and autoclaved, respectively) and mixed to the sterile MRS. Spots of 5 μl of grown cultures of Leuconostoc carnosum were deposited onto the surface of the plates. After 72 h of incubation at 30°C, the plates were placed under an UV lamp and the diameter of the hydrolysis halo around the spots was measured.
Antibiotic Susceptibility
The antibiotic susceptibility of Leuconostoc carnosum was assayed according to the EUCAST protocol (Matuschek et al., 2014). The 24-h MRS cultures were diluted in sterile saline to create suspensions with a turbidity equivalent to 0.5 McFarland and spread onto MRS-agar plates, on the surface of which the antibiotic paper disks were applied. The following antibiotics were assayed: amoxicillin/clavulanic acid, AMC (30 μg); ampicillin, AMP (10 μg); azithromycin, AZM (15 μg); bacitracin, BAC (10 IU); cefixime, CFM (5 μg); ciprofloxacin, CIP (5 μg); clarithromycin, CLR (15 μg); kanamycin, KAN (30 μg); neomycin, NEO (30 μg); tetracycline, TET (5 μg); vancomycin, VAN (5 μg). The inhibition halo was measured after 48 h of incubation at 30°C. Strains were assessed as sensitive (≥20 mm), intermediate (15–19 mm), and resistant (≤14 mm), as previously described (Szutowska and Gwiazdowska, 2021). Escherichia coli ATCC 2592 was used as reference strain in the disk diffusion test.
Anti-Listeria Activity and Cross-Immunity
Grown cultures of Leuconostoc carnosum strains and the corresponding supernatants were assayed for the ability to inhibit Listeria monocytogenes in a plate assay. The supernatants were recovered by centrifugation (5,000 × g for 10 min) and split in aliquots, treated with one of the following: (1) no treatment; (2) the pH was adjusted to 6.0 with 1 M NaOH; (3) addition of 0.1 mg/ml catalase, incubation at 25°C for 30 min, then at 80°C for 10 min; (4) addition of 0.2 mg/ml proteinase K, incubation at 37°C for 1 h, then at 65°C for 10 min. The supernatant aliquots were filter-sterilized at 0.22 μm. A lawn of L. monocytogenes was streaked on surface of Brain Heart Infusion (BHI) agar plates (BD Difco), then 20 μl of sample (i.e., L. carnosum culture or supernatant aliquots) was spotted on the plates. The diameter of the zone of inhibition was measured after 16 h of incubation at 37°C.
For cross-inhibition test, Leuconostoc carnosum strains were inoculated at approximately 107 CFU/ml in agarized MRS plates and challenged, by the agar well diffusion method, against 50 μl of filter-sterilized supernatant of each L. carnosum MRS culture grown at 30°C for 48 h. The diameter of the inhibition halos was measured after 48 h of incubation at 30°C.
EPS and Biofilm Production
Exopolysaccharide (EPS) production was deduced from the mucoid aspect of Leuconostoc carnosum colonies that originated onto MRS-agar plates supplemented with 40 g/L sucrose, after 14 days of incubation at 4°C or 48 h at 25, 30, and 37°C (Fessard et al., 2016).
Biofilm formation was quantified with crystal violet in a microtiter assay (Landeta et al., 2013). Leuconostoc carnosum was inoculated in MRS broth supplemented with 0.1 g/L Tween 80 and incubated for 24 h at 30°C. Grown cultures were 10-fold diluted in the same medium and poured in a 96-well microtiter plate, 150 μl each well. After 14 days of incubation at 4°C or 48 h at 30°C, the supernatants were removed and the biofilms adhering the wells were washed twice with PBS and stained with 125 μl of 1% Crystal Violet dye for 15 min. Stained biofilms were rinsed six times with PBS and extracted with ethanol/acetone solution (8:2 v/v) for 15 min. The absorbance of the extract was measured at 570 nm and normalized by the OD600 of the culture. Strains with values >1 were regarded as positive to biofilm formation (Amaretti et al., 2020).
Tolerance to Simulated Gastric and Intestinal Juices
Simulated gastric juice consisted of 3.2 g/L pepsin, 0.084 M HCl, and 0.03 M NaCl, with the pH adjusted to 1.4 (Vecchione et al., 2018). Simulated intestinal fluid consisted of 8.5 g/L NaCl, 3.0 g/L bile salts (Oxgall, BD Difco), and 1.0 g/L pancreatin, with the pH adjusted to 7.4 (Castro-Rodríguez et al., 2015). Both the gastric and the intestinal fluid were filter sterilized at 0.22 μM.
The biomass of the strains was recovered by centrifugation (13,000 × g at 4°C for 5 min) from 1 ml of a 24-h MRS culture and resuspended in sterile saline. The suspension was diluted to 106 CFU/ml in the gastric or the intestinal juice and incubated at 37°C for 1 or 2 h, respectively. Viable counts were determined with MRS-agar plates. To test the resistance to a sequential treatment with simulated gastric and intestinal fluids, the biomass was recovered by centrifugation (13,000 × g at 4°C for 5 min) after the gastric incubation, washed in PBS, then resuspended and incubated in simulated intestinal fluid.
Mice Colonization
All experimental protocols involving mice were approved by the Animal Care and Use Ethics Committee of the University of Padova under license from the Italian Ministry of Health, and they were in compliance with the national and European guidelines for handling and use of experimental animals.
The biomass of three strains (Leuconostoc carnosum WC 0318, L. carnosum WC 0319, and L. carnosum WC 0321) grown for 48 h in MRS broth was harvested by centrifugation, suspended in PBS containing 15% v/v glycerol to obtain single-dose aliquots of 5 × 108 CFU in 150 μl, then stored at −80°C. Aliquots were thawed one by one and used in the animal trial.
Six-week-old Balb/c male mice were obtained from Envigo Laboratories (Oderzo, Italy) and housed in groups of two per individually ventilated cage, at 22 ± 2°C under a 12-h light–dark cycle. Chow food and water were provided ad libitum. Mice were allowed to acclimate to the laboratory for 1 week before entering experimentation. Three groups of six mice were established, each group being assigned a Leuconostoc carnosum strain. Each mouse received a daily dose of L. carnosum biomass via gastric gavage for 10 days. A control group consisted of six mice daily receiving 150 μl of PBS containing 15% v/v glycerol. Fecal pellets were collected at the end of the treatment, then immediately stored at −80°C in pre-weighted tubes containing 50% glycerol. Viable L. carnosum in mice feces were enumerated with pour plate method within MRS-agar supplemented with 2 μg/ml vancomycin, 25 μg/ml trimethoprim, and 12.5 μg/ml cycloheximide. The plates were incubated at 30°C for 72 h.
In vivo Effect on Immune System
The mice were sacrificed after 10 days of daily supplementation with Leuconostoc carnosum WC 0318, L. carnosum WC 0319, L. carnosum WC 0321, or PBS. The whole ileum was collected, washed in cold PBS, and the Peyer’s patches were isolated under a dissecting microscope. Mucosa was scraped off from the underlying muscle layer of distal ∼5 cm of ileum and immediately frozen in liquid nitrogen and stored at −80°C.
Peyer’s patches were placed in DMEM containing dithiothreitol for 30 min to remove the mucus and then incubated for 90 min at 37°C in Hanks’ balanced salt solution without calcium but added with EDTA to remove epithelial cells. Peyer’s patches were extensively washed with Hanks’ balanced salt solution without calcium and then digested with collagenase D (2 mg/ml) and DNase (10 μg/ml) for 5 min at 37°C. Mononuclear cells were incubated for 30 min in ice-cold PBS containing 2% BSA or murine serum (blocking buffer) to block unspecific bindings. Cells were washed twice by centrifugation in PBS (1,600 rpm, 6 min) and incubated with the appropriate antibodies (Supplementary Table 1). Finally, cells were washed with blocking buffer and analyzed using a FACSCalibur (Becton Dickinson, San Jose, CA, United States).
Cells were first selected on a forward scatter and side scatter dot plot. CD11c double-positive cells were recorded in 10,000 events. For intrakine analysis, CD4 or CD8 positive lymphocytes were selected on FL-3/SSC dot plot and CD25/Foxp3 or IL17 positive cells were recorded in 50,000 events.
Frozen mucosal specimens were weighed and placed in ice-cold PBS buffer (1:10 w/vol ratio) supplemented with protease inhibitors (0.1 mM PMSF, 1 μM leupeptin, 150 nM aprotinin) and homogenized for 30 s. Cellular debris was removed by centrifugation (10,000 × g for 10 min at 4°C). Levels of interleukin (IL)-1β and IL-10 were determined in the cleared supernatants by ELISA, using commercially available kits (eBioscience, Prodotti Gianni, Milano, Italy). The assays were conducted following the manufacturer’s recommended protocols.
Statistical Analyses
Comparisons were performed with Student’s t-test or one-way ANOVA followed by Tukey post hoc test. Statistical analysis was done using SPSS Statistics 21 (IBM Corp., Armonk, NY, United States).
Jaccard’s similarity scores among the strains were calculated with the software Past ver. 4.05 (Hammer et al., 2001) considering their metabolic profile, antibiotic susceptibility, and other physiological properties (Supplementary Table 2) and were used to compute an UPGMA (unweighted pair group method with arithmetic mean) cladogram.
Results
Growth Kinetics and Temperature Optimum
The growth kinetics of 12 Leuconostoc carnosum strains was studied in MRS broth. All the strains grew in the temperature ranging from 4 to 37°C, with difficulty at 42°C. The optimum temperature was predicted in the range of 30.6–34.2°C (median = 32.5°C), while minimum and maximum ones lay in the range of 0.3–3.0°C (median = 1.4°C) and 42.6–44.8 (median = 44.0°C), respectively, (Figure 1). The maximum specific growth rate (μmax) of all the strains was the highest at 30°C (p < 0.05), ranging from 0.23 to 0.34 h–1 with a median of 0.30 h–1 (Figure 2A). For all the strains, the highest biomass yields were achieved at 23°C (p < 0.05), laying in the OD600 range 1.30–3.39, with a median value of 2.56 (Figure 2B). The medium generally presented a pH between 4 and 5 at the end of the growth phase, regardless of the growth temperature (data not shown).
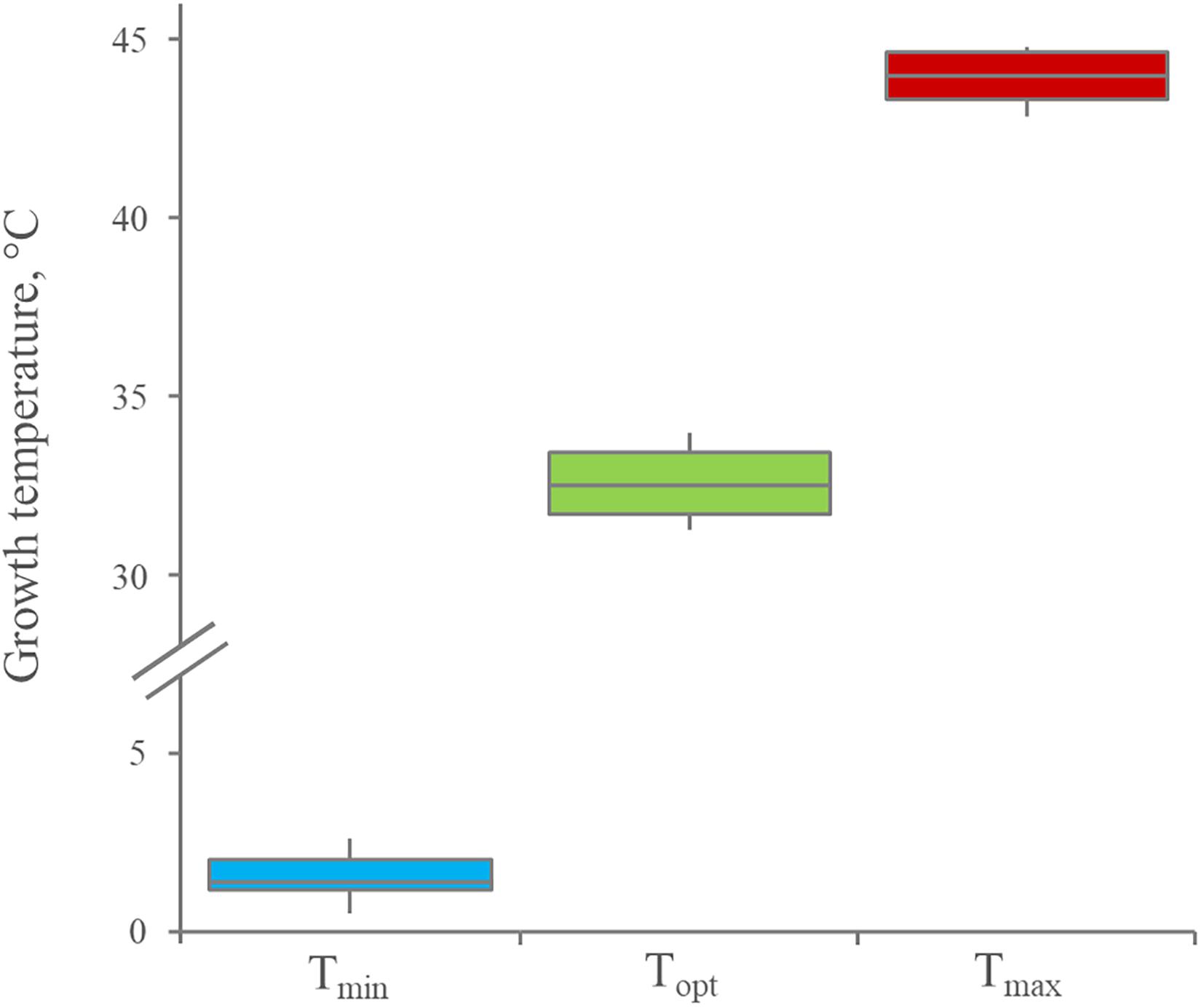
Figure 1. Distribution of minimum, optimum, and maximum growth temperature for 12 Leuconostoc carnosum strains, cultured in MRS broth for 48 h. Boxes indicate the 25th, 50th, and 75th percentiles; whiskers indicate the 10th and 90th percentiles.
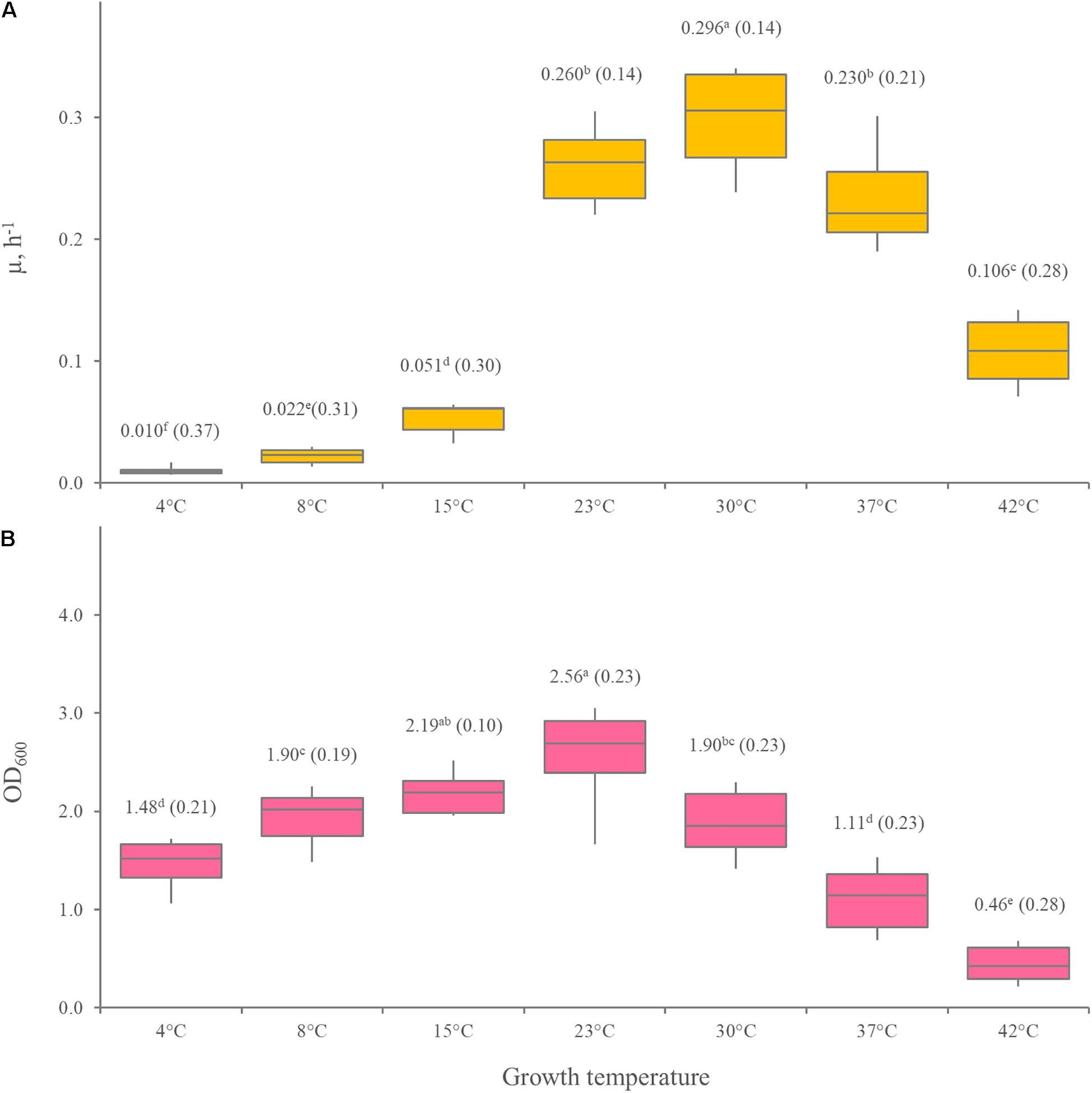
Figure 2. Effect of temperature on μmax (A) and the biomass yield (B) of 12 Leuconostoc carnosum strains, cultured in MRS broth for 48 h. (B) Boxes indicate the 25th, 50th, and 75th percentiles; whiskers indicate the 10th and 90th percentiles. Mean values are reported in the labels, with the coefficient of variation in brackets. Within each panel, means with different letters significantly differ (p < 0.05, ANOVA with Tukey’s post hoc).
Resistance to Oxidative and Osmotic Stress
To evaluate the adaptation to oxidative and osmotic stress, Leuconostoc carnosum strains were cultured in MRS broth with increasing concentrations of H2O2 and NaCl. H2O2 restricted the biomass yield by at least 1 magnitude (p < 0.05) at the concentration of 0.0625 g/L, while higher concentrations completely inhibited growth (Supplementary Figure 1). Differences among the strains were registered. In presence of 0.0625 g/L H2O2, L. carnosum WC319 did not grow, most strains achieved an OD600 < 0.2, while L. carnosum WC0329 performed better, yielding an OD600 of 0.36.
In presence of NaCl, Leuconostoc carnosum grew with decreasing yields up to 60 g/L (Figure 3). Marked differences were observed among the strains (Supplementary Figure 2). In presence of 60 g/L NaCl, L. carnosum WC0327 and L. carnosum WC0318 presented the lowest and the highest growth yield, with OD600 values of 0.03 and 0.38, respectively. Only L. carnosum WC0328 grew to some extent also with 80 g/L NaCl, even though with an OD600 < 0.1
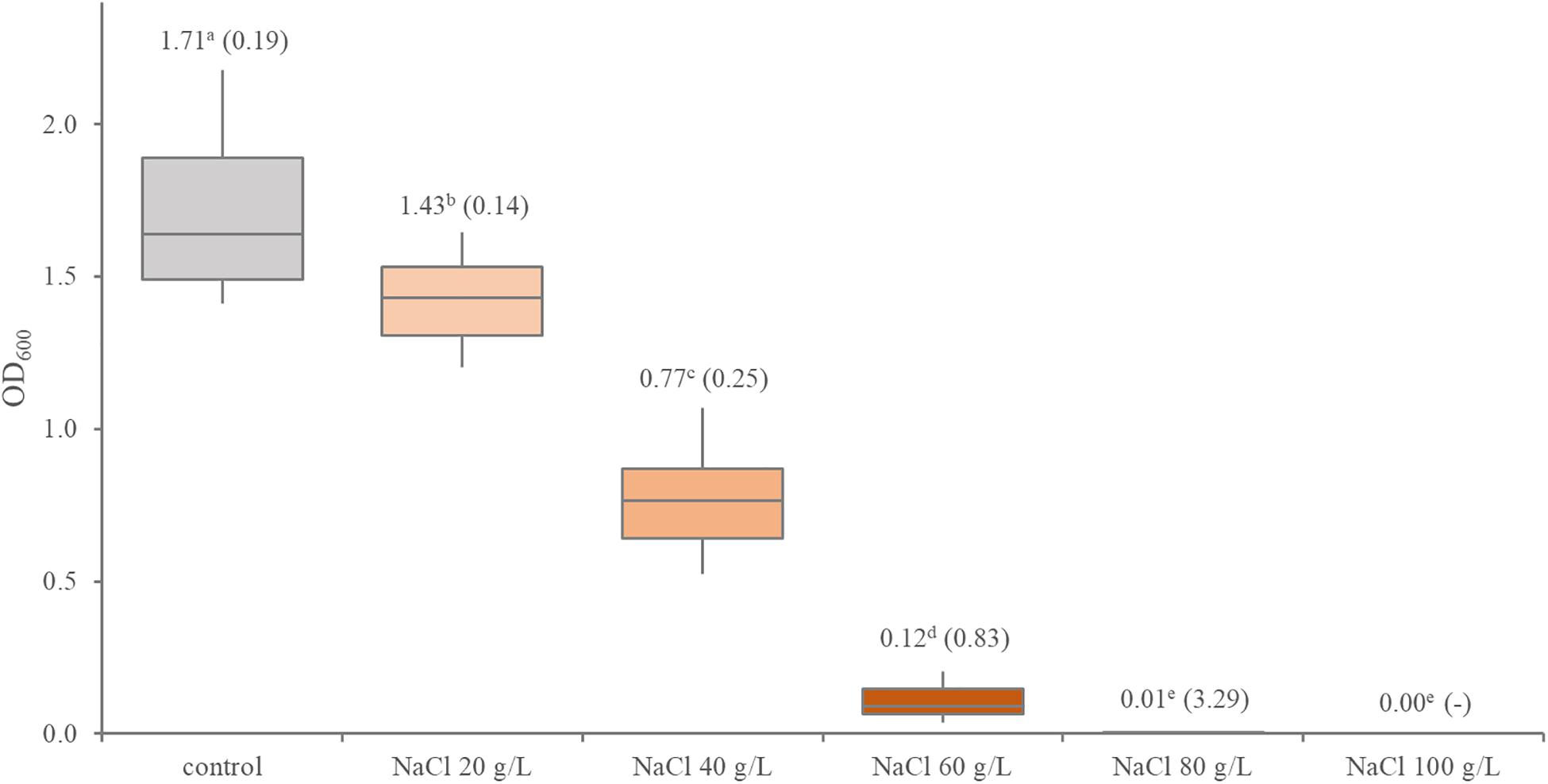
Figure 3. Distribution of the growth performance of 12 Leuconostoc carnosum strains in presence of increasing concentrations of NaCl. Boxes indicate the 25th, 50th, and 75th percentiles; whiskers indicate the 10th and 90th percentiles. The labels report the mean and, in brackets, the coefficient of variation; different letter superscripts indicate means that significantly differ (p < 0.05, ANOVA with Tukey’s post hoc).
Biochemical Characterization
Substrate utilization by the 12 Leuconostoc carnosum strains was assessed with API 50 CH strips and specific growth experiments in modified MRS media (Table 1 and Supplementary Figure 3). All the strains were positive for the utilization of glucose, ribose, sucrose, and esculin. Fructose, mannose, methyl-α-glucopyranoside, N-acetyl-glucosamine, trehalose, and turanose were used by at least 10 strains, while cellobiose, maltose, gentibiose, and gluconate were used by 5–7 strains. On the other hand, all the strains were unable to use 37 substrates, among which glycerol, erythritol, D- and L-arabinose, D- and L-xylose, galactose, L-sorbose, L-rhamnose, inositol, mannitol, sorbitol, lactose, melibiose, inulin, melezitose, raffinose, amidon, glycogen, xylitol, tagatose, fucose, citrate, and arginine. L. carnosum WC0329 exhibited the smallest range of growth substrates (6, i.e., glucose, fructose, ribose, sucrose, gluconate, and esculin) and L. carnosum WC0323 the largest one (14, i.e., glucose, fructose, ribose, mannose, methyl-α-glucopyranoside, N-acetyl-glucosamine, cellobiose, maltose, esculin, sucrose, trehalose, gentibiose, turanose, and gluconate).
Proteolitic and lipolytic activity were also assessed, showing that all the strains were negative to gelatin and triglyceride hydrolysis.
Antibiotic Resistance
The 12 Leuconostoc carnosum strains were screened for susceptibility to 11 antibiotics (Figure 4). All the strains were resistant to cefixime and vancomycin and most of them were resistant or gave an intermediate response to ciprofloxacin and kanamycin. On the other hand, the strains were all susceptible to amoxicillin/clavulanic acid, azithromycin, clarithromycin, and tetracycline and were susceptible or gave an intermediate response to neomycin. Most strains were susceptible to ampicillin and bacitracin, except for a few resistant or intermediate strains. L. carnosum WC0329 was resistant to ampicillin, while L. carnosum WC0319 and L. carnosum WC0322 were resistant to bacitracin.
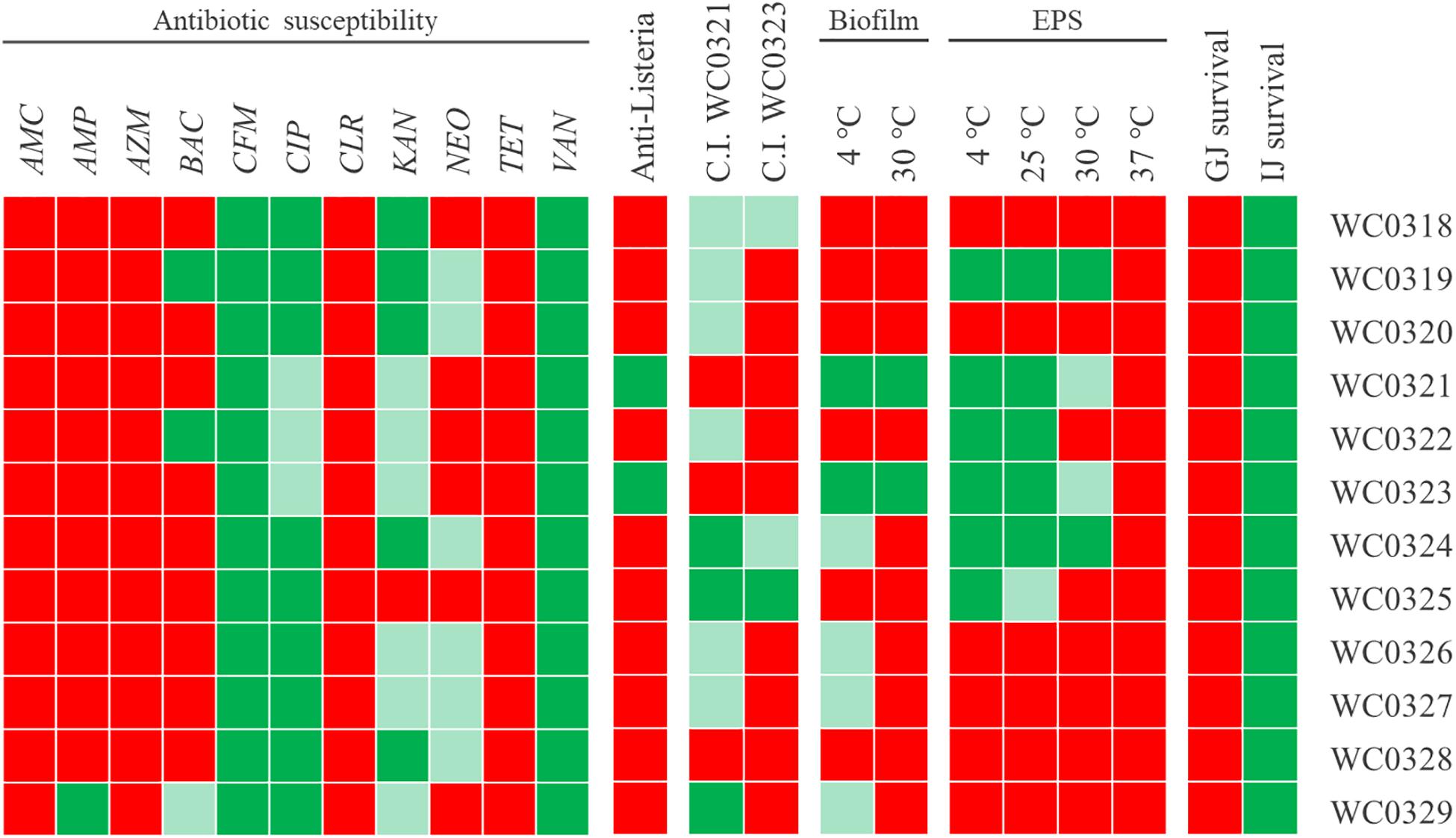
Figure 4. Phenotypic traits of 12 Leuconostoc carnosum strains. Resistance (green), susceptibility (red), and intermediate behavior (light green) to amoxicillin/clavulanic acid (AMC), ampicillin (AMP), azithromycin (AZM), bacitracin (BAC), cefixime (CFM), ciprofloxacin (CIP), clarithromycin (CLR), kanamycin (KAN), neomycin (NEO), tetracycline (TET), and vancomycin (VAN); anti-Listeria activity and Cross-Immunity test (red, absence of growth inhibition; light green, inhibition zone with diameter <10 mm; green, diameter ≥10 mm); biofilm production (red, OD 570/OD600 < 1; light green, ≥1; green, ≥5); EPS production (red, normal colonies; light green, slightly mucoid colonies; green, mucoid aspect); survival to gastric and intestinal juices (red, absence of viable cells; green, presence of residual growth).
Antimicrobial Activity
Only Leuconostoc carnosum WC0321 and L. carnosum WC0323 inhibited the growth of L. monocytogenes to some extent (Figure 4). For both the strains, inhibition of Listeria occurred with the culture, the supernatant, the neutralized supernatant, and the supernatant treated with catalase, while the supernatant treated with proteinase K did not exert any inhibition (data not shown).
Growth inhibition by Leuconostoc carnosum WC0321 or, to a lesser extent, by L. carnosum WC0323 was observed also in the other L. carnosum strains, with the exception of L. carnosum WC0328 that was immune (Figure 4). L. carnosum WC0324 and L. carnosum WC0325 were the most sensitive, particularly to the supernatant of L. carnosum WC0321.
EPS and Biofilm Production
EPS production was assayed at 4, 25, 30, and 37°C (Figure 4). The ability to produce EPS was observed in six strains (i.e., Leuconostoc WC0319, L. carnosum WC0321, L. carnosum WC0322, L. carnosum WC0323, L. carnosum WC0324, and L. carnosum WC0325) that presented mucoid colonies when cultured at 4 and 25°C. EPS production tended to reduce with the increase of temperature. A decrease in mucosity was observed in the colonies of L. carnosum WC0325 at 25°C and in those of L. carnosum WC0321 and L. carnosum WC0323 at 30°C. No strains presented mucoid colonies at 37°C.
Leuconostoc carnosum WC0321 and L. carnosum WC0323 produced considerable biofilm both at 4 and 30°C. A slight biofilm formation was observed only at 4 and not at 30°C for L. carnosum WC0324, L. carnosum WC0326, L. carnosum WC0327, and L. carnosum WC0329 (Supplementary Figure 4).
Resistance to Simulated Gastric and Intestinal Juices
The treatment with simulated gastric juice was lethal for all the strains (Figure 4). On the other hand, all the strains survived to some extent to the treatment with simulated intestinal juice, presenting a residual viability in the range of 16–100% (Figure 4 and Supplementary Table 3). Coherently, no strains survived the sequential incubation, first in the gastric and then in the intestinal juice.
Hierarchical Clustering of Phenotypic Diversity
The hierarchical clustering of the 12 Leuconostoc carnosum strains recognized three clades according to Jaccard’s similarity of the phenotypic traits (Figure 5). One clade encompassed L. carnosum WC0319, L. carnosum WC0324, L. carnosum WC0325, and L. carnosum WC0329, which were all unable to use the disaccharides cellobiose, maltose, and gentiobiose and were negative to bacteriocin production and biofilm formation, at least at 30°C. The second clade encompassed L. carnosum WC0321, L. carnosum WC0322, and L. carnosum WC0323, which were the most sensitive to kanamycin and neomycin, and produced EPS, with L. carnosum WC0321 and L. carnosum WC0323 that also exerted anti-Listeria activity and were the main producers of biofilm. The third clade, encompassing L. carnosum WC0318, L. carnosum WC0320, L. carnosum WC0326, L. carnosum WC0327, and L. carnosum WC0328, differed from the first one for the wider spectrum of substrates, particularly the disaccharides.
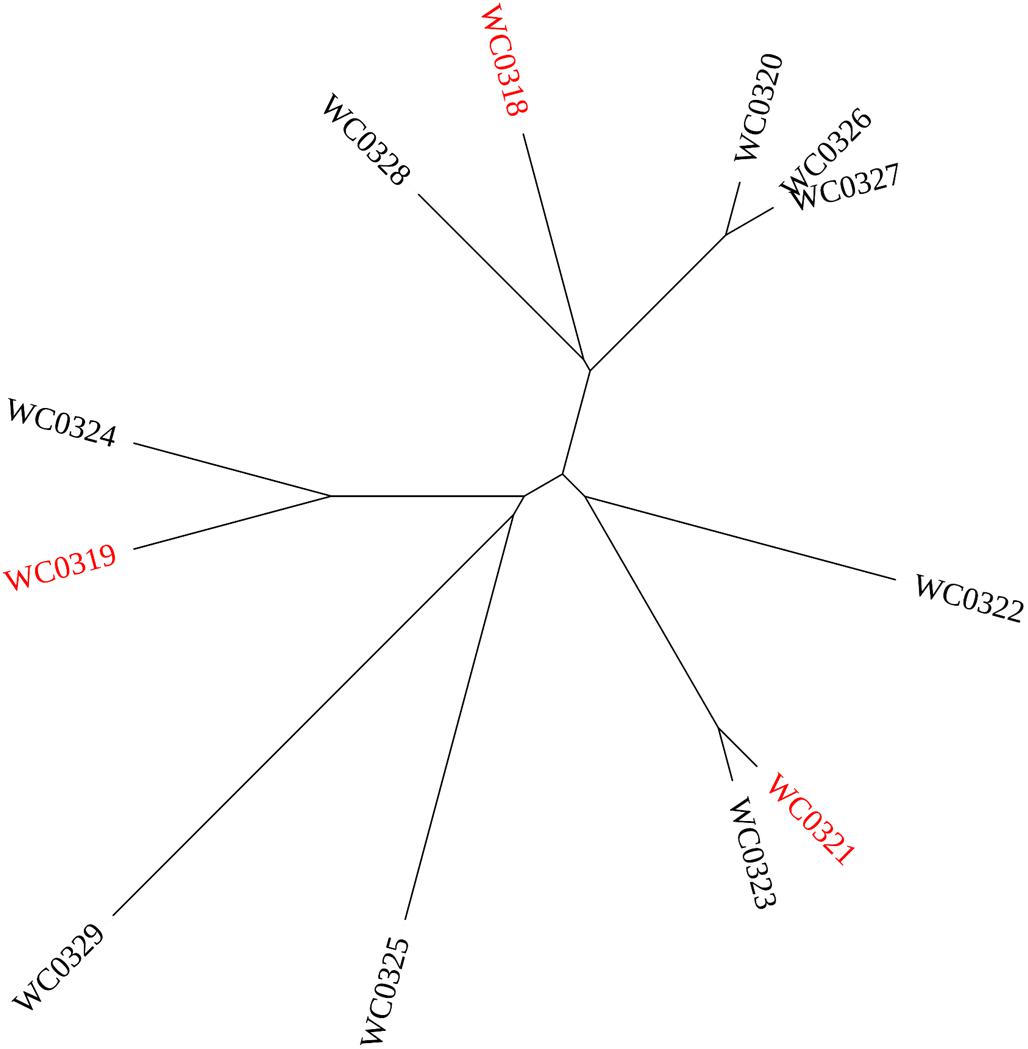
Figure 5. UPGMA cladogram of the 12 strains Leuconostoc carnosum according to their phenotypic traits. The strains selected for the mice colonization study are labeled in red.
Colonization of Mice Gut
The strains Leuconostoc carnosum WC0318, L. carnosum WC0319, and L. carnosum WC0321, each belonging to a different clade, were selected to investigate their potential in colonizing the intestine of mice. They all failed to colonize the intestine of Balb/c mice after 10 days of daily administration at the dose of 5 × 108 cells. For the three mice groups, each receiving a single strain, the viable counts in the fecal samples onto MRS plates did not yield any colony (data not shown).
Effect on the Intestinal Mucosa-Associated Immune System
To evaluate the impact of Leuconostoc carnosum on the immune system, the phenotype of dendritic cells (DC) and lymphocytes in Peyer’s patches was analyzed. Following 10 days of supplementation, L. carnosum WC0321 enhanced all the measured markers of DC maturation (namely CD-80, MHC-II, TLR2, and TLR4), while L. carnosum WC0318 and L. carnosum WC0319 had a limited effect (Figure 6). L. carnosum effects were restricted to DC, since no effects on polarization of CD3+ lymphocytes toward regulatory (CD4+ CD25+ FoxP3+) or inflammatory (CD3+ CD8+ IL17+) phenotype were observed.
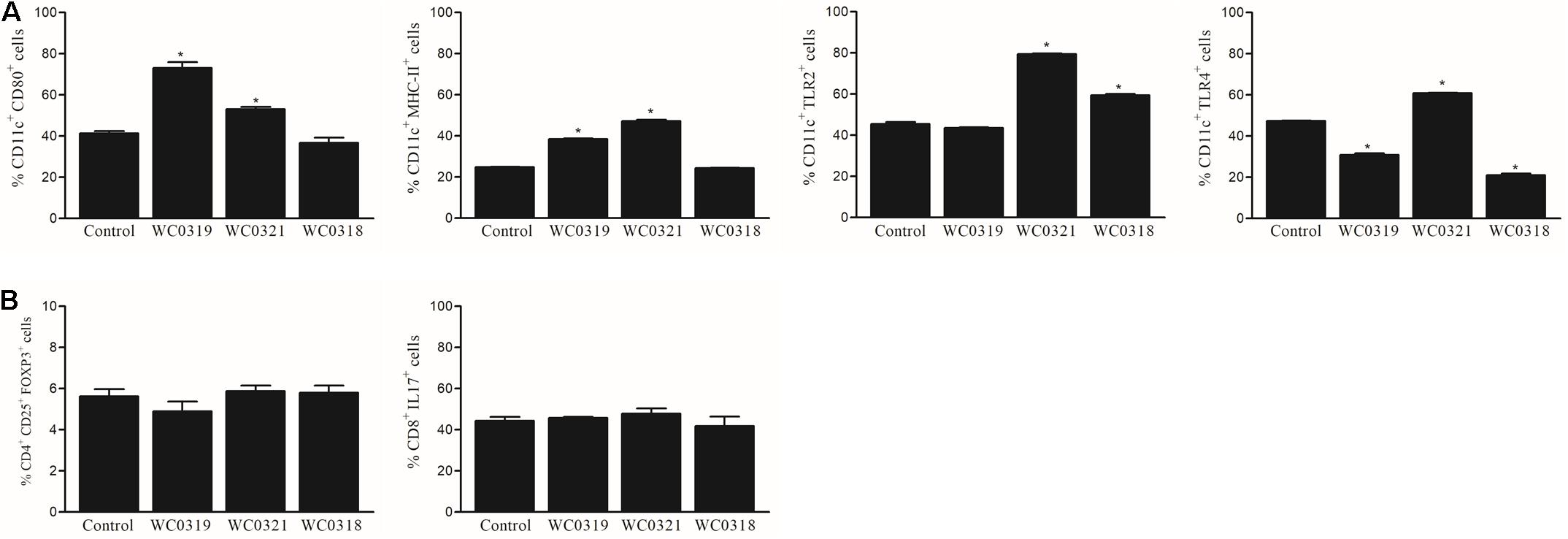
Figure 6. Effect of daily oral supplementation with 5 × 108 CFU of Leuconostoc carnosum WC0318, L. carnosum WC0319, and L. carnosum WC0321 on intestinal mucosa immune cells, determined by FACS: (A) DC (CD11c+); (B) CD3 + lymphocytes. * Indicates significant difference versus the control (p < 0.01, t-test).
To further assess the impact of Leuconostoc carnosum on mucosal immune system balance, the expression level of IL-1β and IL-10 was measured after 10 days of supplementation. No significant effects on mucosal cytokine levels were observed (p > 0.05), as reported in Figure 7.
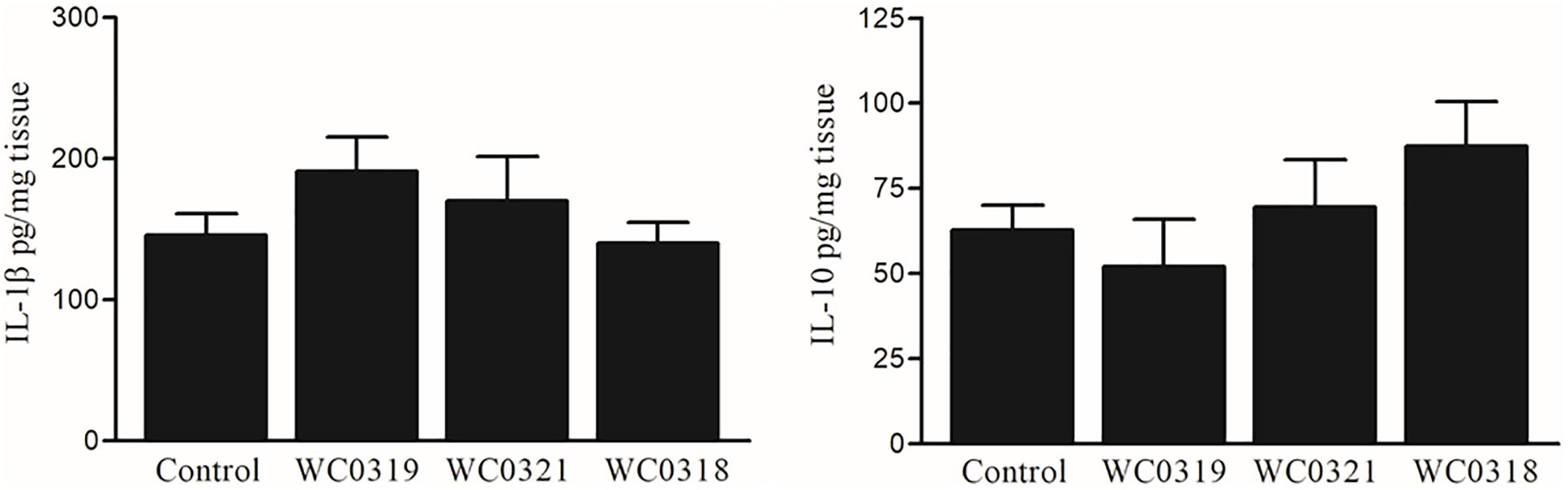
Figure 7. Effect of daily oral supplementation with 5 × 108 CFU of Leuconostoc carnosum WC0318, L. carnosum WC0319, and L. carnosum WC0321 on intestinal mucosa IL-1β and IL-10, quantified by ELISA. Cytokine levels were expressed as pg/ml of tissue lysate. Means did not significantly differ from the control (p > 0.05, t-test).
Discussion
Twelve strains of Leuconostoc carnosum, the genome of which has been recently subjected to comparative analysis (Candeliere et al., 2021), were investigated in terms of biochemical, physiological, and functional properties. The spectrum of sugars fermented by the strains was limited to few mono- and disaccharides but consistent with the main isolation sources of the species, i.e., meat products and fermented vegetables (Jung et al., 2014; Ishii et al., 2017; Raimondi et al., 2018). All the strains fermented glucose, sucrose, and ribose, coherently with the equipment of genes encoding the transporters for these sugars (i.e., specific phosphotransferase systems for glucose and sucrose, and ABC transporters for ribose) (Candeliere et al., 2021). In meat matrices, glucose derives from the hydrolysis of glycogen, while sucrose is present in plants or in fermented meat products where it may be supplemented to accelerate the fermentation rate. Ribose is abundant in certain vegetables and in meat, where it derives from the ribonucleotides of the muscle (Meinert et al., 2009). Most of the strains (10 out of 12) fermented mannose and/or fructose and were all equipped with PTS transport systems for these sugars which are abundant in fruits and vegetables. All the strains exhibited the ability to ferment trehalose that, mainly in conjugated forms, actively participates in signaling in plants and esculin, a plant glucoside. Even though cellobiose fermentation was restricted to a few strains, in agreement with previous indications (Shaw and Harding, 1989), all the strains harbor the genes for a cellobiose PTS transport system. The utilization of substrates such as trehalose, cellobiose, and esculin suggests a remote and efficient association of L. carnosum with plants (Jung et al., 2014; Ishii et al., 2017). Unlike Latilactobacillus sakei (former Lactobacillus sakei, Zheng et al., 2020) and other LAB that thrive in meat products gaining energy from arginine through the arginine deiminase pathway, L. carnosum did not catabolize arginine. Differently from other Leuconostoc species, L. carnosum was unable to catabolize citrate, coherently with the lack of the genes encoding citrate transporter, citrate lyase, and oxaloacetate decarboxylase, and thus relied only on carbohydrate fermentation for energy supply (Özcan et al., 2019; Candeliere et al., 2021).
As meat products are subjected to treatments or conditions that hamper growth of microorganisms, it is expected that efficient colonizers exhibit some adaptation to cope with hostile factors such as low temperature, pH variations, and osmotic and oxidative stress (D’Angelo et al., 2017). The study of the temperature limits indicated that Leuconostoc carnosum is mesophilic, but versatile enough to grow at temperatures that are compatible with refrigeration, thus explaining the bloom in many meat-based products especially throughout long shelf-life periods (Samelis et al., 2000). Furthermore, L. carnosum can take advantage of any temperature abuse occurring in refrigerators or over the cold chain since a rise from 4 to 8°C caused a twofold increase of the specific growth rate. The ability of L. carnosum to efficiently grow in presence of up to 60 g/L NaCl, although with reduced yields in presence of increasing the amount of salt, is consistent with his adaptability to high-salt food matrices. On the other side, all the strains resulted very sensitive to hydrogen peroxide, according to the absence of catalase.
The increasing demand of healthy, fresh, and natural foods devoid of added chemical preservatives and stabilizers has fostered the search of bioprotective starters, particularly among LAB. Various species belonging to the genus Leuconostoc, including Leuconostoc carnosum, produce bacteriocin of different classes that target Listeria (e.g., leucocins A and C) and other LAB such as Leuconostoc and Weissella (e.g., leucocin B) (Felix et al., 1994; Parente et al., 1996; Pérez-Sánchez et al., 2011; Wan et al., 2015). This study provides evidence of the protective role of L. carnosum WC0321 and L. carnosum WC0323 against L. monocytogenes, consistently with the presence in these strains of the genes encoding leucocin B and the ABC-like transporter LanT, the former harbored in plasmids and the latter in the genome (Candeliere et al., 2021). Interestingly, these strains exerted antimicrobial activity also against the other L. carnosum strains, and it should be investigated if such effect was mediated by the same bacteriocin. Coherently, L. carnosum WC0329, which harbors only the plasmid genes for leucocin B but lacks LanT, did not exert any anti-Listeria effect. The ability to inhibit other strains and L. monocytogenes is encouraging for a possible utilization of selected L. carnosum strains as bioprotective starters, to prevent growth of both pathogenic microorganisms and LAB that could participate to spoilage. In particular, bacterial starters that do not produce EPS, biogenic amines, and lipolytic or proteolytic activities could be used to extend the shelf-life of the products, provided that they have no adverse effects on visual, olfactory, and gustative properties (Ammor and Mayo, 2007; Singh et al., 2012; Zarour et al., 2012).
Ready-to-eat meat products may harbor up to 108 CFU/g of Leuconostoc carnosum; therefore, the potential impact of this species on health deserves attention. The fact that L. carnosum strains did not survive simulated gastric juice reassures on their abatement in the stomach even though they withstood to simulated intestinal fluid. Ingestion of L. carnosum is expected not to impact on health, or at least not to determine any intestine colonization, even transient, taking into account the unsuccessful recovery of L. carnosum from the feces of mice boosted L. carnosum. However, it is still possible that L. carnosum is part of the wide and understudied biodiversity of LAB within human feces (Rossi et al., 2016), and its presence within the gut microbiome deserves further investigation.
The dietary supplementation of Leuconostoc carnosum strains to mice did not affect the maturation of intestinal lymphocytes and mucosal levels of IL-1β and IL-10 but affected the maturation of surface markers of intestinal DC. The response of DC was higher with L. carnosum WC0321, compared with WC0318 and WC0319, confirming that some strains might exert favorable effects on the mucosal associated immune system. The significant difference among the strains is not surprising since strain-specific effects on mucosal immune system is well recognized, particularly in the field of probiotics (Luyer et al., 2005; Kekkonen et al., 2008; McFarland et al., 2018; Sagheddu et al., 2020). Since L. carnosum strains do not survive the gastric barrier, their immunomodulatory activity must be ascribed to some bacterial cell components. Indeed, it was demonstrated that capsular structures, cell wall extracts, DNA, and S-layer proteins of LAB can induce the activation of DCs through Toll-like receptor signaling, which play a critical role in the generation of protective immune responses, and that non-viable probiotics could also have significant beneficial effects on human health (Gueimonde et al., 2004; Taverniti and Guglielmetti, 2011; Lahtinen, 2012; Engevik et al., 2021). Intriguingly, L. carnosum WC0321 efficiently produced both EPS and biofilm and, unlike L. carnosum WC0318 and L. carnosum WC0319, harbors genes encoding some tyrosine kinases and phosphatases that regulate the formation of exopolysaccharides, EPS biosynthetic genes of the ywq group, and EPS glycosyltransferases. EPS mediate plenty of beneficial properties in probiotic strains and it should be further investigated whether it played some role in inducing the response of DC to L. carnosum WC0321 (Angelin and Kavitha, 2020).
On the other side, EPS-producing bacteria participate in deterioration of meat-based foods, such as MAP cooked ham, where they cause the appearance of a ropy slime that is considered unacceptable by consumers for certain products. Six out of 12 strains were positive to the ropy phenotype, with a stronger tendency to form viscous colonies at the lower growth temperatures, and they may be involved in the appearance of slime in food, causing the sensorial quality to decline throughout the shelf life.
Some resistance to antibiotics was highlighted, although it was not predicted by comparative genomics. Vancomycin resistance is a common trait of bacteria belonging to the Leuconostoc–Weissella group, ascribable to the structure of the active site of D-alanine–D-alanine ligase (Flórez et al., 2016). In a similar way, the shared resistance to the β-lactam cefixime and to the fluoroquinolone ciprofloxacin may be due to some intrinsic mechanism. On the other hand, the resistance to ampicillin or bacitracin exhibited by few strains can be due to some mechanism that escaped the detection by genome scanning.
Many traits herein investigated should reassure on the safety of Leuconostoc carnosum and leave ample scope for exploitation of selected starters in conserved meats, where the species, coping with low temperatures and presence of the salt, is very competitive. Potential candidates for such a technological application are the bacteriocin producers L. carnosum WC0321 and L. carnosum WC0323. These strains deserve to be challenged in real food matrix, aiming to validate their biopreservative action and to check if they have adverse effects food sensorial properties, for instance in relation to slime formation. A nutraceutical application of L. carnosum, apart from the potential as food biopreservative, could not be excluded but does not seem straightforward. In this perspective, the potential immunomodulatory effect of EPS has to be further investigated, to assess if some relevant health-promoting effect could be ascribed to this bacterial component.
Data Availability Statement
Publicly available datasets were analyzed in this study. This data can be found here: PRJNA542256.
Ethics Statement
The animal study was reviewed and approved by Animal Care and Use Ethics Committee of the University of Padova.
Author Contributions
SR and MR conceived the study. SR, FC, and GS carried out the microbiology experiments. PB and IC conceived and performed the animal trial and the immunology experiments. AA and SR carried out statistical analysis and curated data presentation. AA, MR, and SR wrote the article with contributions from all other authors. All authors contributed to the article and approved the submitted version.
Conflict of Interest
The authors declare that the research was conducted in the absence of any commercial or financial relationships that could be construed as a potential conflict of interest.
Publisher’s Note
All claims expressed in this article are solely those of the authors and do not necessarily represent those of their affiliated organizations, or those of the publisher, the editors and the reviewers. Any product that may be evaluated in this article, or claim that may be made by its manufacturer, is not guaranteed or endorsed by the publisher.
Supplementary Material
The Supplementary Material for this article can be found online at: https://www.frontiersin.org/articles/10.3389/fmicb.2021.730827/full#supplementary-material
Footnotes
References
Amaretti, A., Righini, L., Candeliere, F., Musmeci, E., Bonvicini, F., Gentilomi, G. A., et al. (2020). Antibiotic resistance, virulence factors, phenotyping, and genotyping of non-Escherichia coli Enterobacterales from the gut microbiota of healthy subjects. Int. J. Mol. Sci. 21:1847. doi: 10.3390/ijms21051847
Ammor, M. S., and Mayo, B. (2007). Selection criteria for lactic acid bacteria to be used as functional starter cultures in dry sausage production: an update. Meat Sci. 76, 138–146. doi: 10.1016/j.meatsci.2006.10.022
Angelin, J., and Kavitha, M. (2020). Exopolysaccharides from probiotic bacteria and their health potential. Int. J. Biol. Macromol. 162, 853–865. doi: 10.1016/j.ijbiomac.2020.06.190
Björkroth, J., Dicks, L. M. T., Endo, A., and Holzapfel, W. H. (2014). “The genus Leuconostoc,” in Lactic Acid Bacteria: Biodiversity and Taxonomy, eds W. H. Holzapfel and B. J. B. Wood (New York, NY: Wiley), 391–404.
Björkroth, K. J., Vandamme, P., and Korkeala, H. J. (1998). Identification and characterization of Leuconostoc carnosum, associated with production and spoilage of vacuum-packaged, sliced, cooked ham. Appl. Environ. Microbiol. 64, 3313–3319. doi: 10.1128/AEM.64.9.3313-3319.1998
Candeliere, F., Raimondi, S., Spampinato, G., Tay, M. Y. F., Amaretti, A., Schlundt, J., et al. (2020). Draft genome sequences of 12 Leuconostoc carnosum strains isolated from cooked ham packaged in a modified atmosphere and from fresh sausages. Microbiol. Resour. Announc. 9, e1247–e1219. doi: 10.1128/MRA.01247-19
Candeliere, F., Raimondi, S., Spampinato, G., Tay, M. Y. F., Amaretti, A., Schlundt, J., et al. (2021). Comparative genomics of Leuconostoc carnosum. Front. Microbiol. 11:605127. doi: 10.3389/fmicb.2020.605127
Castro-Rodríguez, D., Hernández-Sánchez, H., and Yáñez Fernández, J. (2015). Probiotic properties of Leuconostoc mesenteroides isolated from aguamiel of Agave salmiana. Probiotics Antimicrob. Proteins 7, 107–117. doi: 10.1007/s12602-015-9187-5
D’Angelo, L., Cicotello, J., Zago, M., Guglielmotti, D., Quiberoni, A., and Suarez, V. (2017). Leuconostoc strains isolated from dairy products: response against food stress conditions. Food Microbiol. 66, 28–39. doi: 10.1016/j.fm.2017.04.001
Engevik, M. A., Ruan, W., Esparza, M., Fultz, R., Shi, Z., Engevik, K. A., et al. (2021). Immunomodulation of dendritic cells by Lactobacillus reuteri surface components and metabolites. Physiol. Rep. 9:e14719. doi: 10.14814/phy2.14719
Felix, J. V., Papathanasopoulos, M. A., Smith, A. A., von Holy, A., and Hastings, J. W. (1994). Characterization of leucocin B-Ta11a: a bacteriocin from Leuconostoc carnosum Ta11a isolated from meat. Curr. Microbiol. 29, 207–212. doi: 10.1007/BF01570155
Fessard, A., Bourdon, E., Payet, B., and Remize, F. (2016). Identification, stress tolerance, and antioxidant activity of lactic acid bacteria isolated from tropically grown fruits and leaves. Can. J. Microbiol. 62, 550–561. doi: 10.1139/cjm-2015-0624
Fessard, A., and Remize, F. (2019). Genetic and technological characterization of lactic acid bacteria isolated from tropically grown fruits and vegetables. Int. J. Food Microbiol. 301, 61–72. doi: 10.1016/j.ijfoodmicro.2019.05.003
Flórez, A. B., Campedelli, I., Delgado, S., Alegría, Á, Salvetti, E., Felis, G. E., et al. (2016). Antibiotic susceptibility profiles of dairy Leuconostoc, analysis of the genetic basis of atypical resistances and transfer of genes in vitro and in a food matrix. PLoS One 11:e0145203. doi: 10.1371/journal.pone.0145203
Geeraerts, W., Pothakos, V., De Vuyst, L., and Leroy, F. (2018). Variability within the dominant microbiota of sliced cooked poultry products at expiration date in the Belgian retail. Food Microbiol. 73, 209–215. doi: 10.1016/j.fm.2018.01.019
Goto, S., Takahashi, H., Kawasaki, S., Kimura, B., Fujii, T., Nakatsuji, M., et al. (2004). Detection of Leuconostoc strains at a meat processing plant using polymerase chain reaction. Shokuhin Eiseigaku Zasshi. 45, 25–28. doi: 10.3358/shokueishi.45.25
Gueimonde, M., Delgado, S., Mayo, B., Ruas-Madiedo, P., Margolles, A., and De Los Reyes-Gavilan, C. G. (2004). Viability and diversity of probiotic Lactobacillus and Bifidobacterium populations included in commercial fermented milks. Food Res. Int. 37, 839–850. doi: 10.1016/j.foodres.2004.04.006
Hammer, Ø, Harper, D. A. T., and Ryan, P. D. (2001). PAST: paleontological statistics software package for education and data analysis. Palaeontol. Electron. 4, 1–9.
Hemme, D., and Foucaud-Scheunemann, C. (2004). Leuconostoc, characteristics, use in dairy technology and prospects in functional foods. Int. Dairy J. 14, 467–494. doi: 10.1016/j.idairyj.2003.10.005
Hong, Y., Yang, H. S., Li, J., Han, S. K., Chang, H. C., and Kim, H. Y. (2014). Identification of lactic acid bacteria in salted Chinese cabbage by SDS-PAGE and PCR-DGGE. J. Sci. Food Agric. 94, 296–300. doi: 10.1002/jsfa.6257
Ishii, M., Matsumoto, Y., Nishida, S., and Sekimizu, K. (2017). Decreased sugar concentration in vegetable and fruit juices by growth of functional lactic acid bacteria. Drug Discov. Ther. 11, 30–34. doi: 10.5582/ddt.2016.01079
Jung, J. Y., Lee, S. H., and Jeon, C. O. (2014). Kimchi microflora: history, current status, and perspectives for industrial kimchi production. Appl. Microbiol. Biotechnol. 98, 2385–2393. doi: 10.1007/s00253-014-5513-1
Kekkonen, R. A., Lummela, N., Karjalainen, H., Latvala, S., Tynkkynen, S., Jarvenpaa, S., et al. (2008). Probiotic intervention has strain-specific anti-inflammatory effects in healthy adults. World J. Gastroenterol. 14, 2029–2036. doi: 10.3748/wjg.14.2029
Lahtinen, S. J. (2012). Probiotic viability – does it matter? Microb. Ecol. Health Dis. 23:18567. doi: 10.3402/mehd.v23i0.18567
Landeta, G., Curiel, J. A., Carrascosa, A. V., Muñoz, R., and de las Rivas, B. (2013). Characterization of coagulase-negative staphylococci isolated from Spanish dry cured meat products. Meat Sci. 93, 387–396. doi: 10.1016/j.meatsci.2012.09.019
Li, X., Li, C., Ye, H., Wang, Z., Wu, X., Han, Y., et al. (2019). Changes in the microbial communities in vacuum-packaged smoked bacon during storage. Food Microbiol. 77, 26–37. doi: 10.1016/j.fm.2018.08.007
Luyer, M. D., Buurman, W. A., Hadfoune, M., Speelmans, G., Knol, J., Jacobs, J. A., et al. (2005). Strain-specific effects of probiotics on gut barrier integrity following hemorrhagic shock. Infect. Immun. 7, 3686–3692. doi: 10.1128/IAI.73.6.3686-3692.2005
Matuschek, E., Brown, D. F., and Kahlmeter, G. (2014). Development of the EUCAST disk diffusion antimicrobial susceptibility testing method and its implementation in routine microbiology laboratories. Clin. Microbiol. Infect. 20, O255–O266. doi: 10.1111/1469-0691.12373
McFarland, L. V., Evans, C. T., and Goldstein, E. (2018). Strain-specificity and disease-specificity of probiotic efficacy: a systematic review and meta-analysis. Front. Med. 5:124. doi: 10.3389/fmed.2018.00124
Meinert, L., Schäfer, A., Bjergegaard, C., Aaslyng, M. D., and Bredie, W. L. (2009). Comparison of glucose, glucose 6-phosphate, ribose, and mannose as flavour precursors in pork; the effect of monosaccharide addition on flavour generation. Meat Sci. 81, 419–425. doi: 10.1016/j.meatsci.2008.08.018
Milliere, J. B., Mathot, A. G., Schmitt, P., and Divies, C. (1989). Phenotypic characterization of Leuconostoc species. J. appl. Bacteriol. 67, 529–542. doi: 10.1111/j.1365-2672.1989.tb02525.x
Montanari, C., Barbieri, F., Magnani, M., Grazia, L., Gardini, F., and Tabanelli, G. (2018). Phenotypic diversity of Lactobacillus sakei strains. Front. Microbiol. 9:2003. doi: 10.3389/fmicb.2018.02003
Morandi, S., Cremonesi, P., Silvetti, T., and Brasca, M. (2013). Technological characterization, antibiotic susceptibility and antimicrobial activity of wild-type Leuconostoc strains isolated from north Italian traditional cheeses. J. Dairy Res. 80, 457–466. doi: 10.1017/S0022029913000447
Özcan, E., Selvi, S. S., Nikerel, E., Teusink, B., Toksoy Öner, E., and Çakır, T. (2019). A genome-scale metabolic network of the aroma bacterium Leuconostoc mesenteroides subsp. cremoris. Appl. Microbiol. Biotechnol. 103, 3153–3165. doi: 10.1007/s00253-019-09630-4
Parente, E., Moles, M., and Ricciardi, A. (1996). Leucocin F10, a bacteriocin from Leuconostoc carnosum. Int. J. Food Microbiol. 33, 231–243. doi: 10.1016/0168-1605(96)01159-2
Pérez-Sánchez, T., Balcázar, J. L., García, Y., Halaihel, N., Vendrell, D., De Blas, I., et al. (2011). Identification and characterization of lactic acid bacteria isolated from rainbow trout, Oncorhynchus mykiss (Walbaum), with inhibitory activity against Lactococcus garvieae. J. Fish Dis. 34, 499–507. doi: 10.1111/j.1365-2761.2011.01260.x
Raimondi, S., Luciani, R., Sirangelo, T. M., Amaretti, A., Leonardi, A., Ulrici, A., et al. (2019). Microbiota of sliced cooked ham packaged in modified atmosphere throughout the shelf life: microbiota of sliced cooked ham in MAP. Int. J. Food Microbiol. 289, 200–208. doi: 10.1016/j.ijfoodmicro.2018.09.017
Raimondi, S., Nappi, M. R., Sirangelo, T. M., Leonardi, A., Amaretti, A., Ulrici, A., et al. (2018). Bacterial community of industrial raw sausage packaged in modified atmosphere throughout the shelf life. Int. J. Food Microbiol. 280, 78–86. doi: 10.1016/j.ijfoodmicro.2018.04.041
Rossi, M., Martínez-Martínez, D., Amaretti, A., Ulrici, A., Raimondi, S., and Moya, A. (2016). Mining metagenomic whole genome sequences revealed subdominant but constant Lactobacillus population in the human gut microbiota. Environ. Microbiol. Rep. 8, 399–406. doi: 10.1111/1758-2229.12405
Rosso, L., Lobry, J. R., and Flandrois, J. P. (1993). An unexpected correlation between cardinal temperatures of microbial growth highlighted by a new model. J. Theor. Biol. 162, 447–463. doi: 10.1006/jtbi.1993.1099
Sagheddu, V., Uggeri, F., Belogi, L., Remollino, L., Brun, P., Bernabè, G., et al. (2020). The biotherapeutic potential of Lactobacillus reuteri characterized using a target-specific selection process. Front. Microbiol. 11:532. doi: 10.3389/fmicb.2020.00532
Samelis, J., Björkroth, J., Kakouri, A., and Rementzis, J. (2006). Leuconostoc carnosum associated with spoilage of refrigerated whole cooked hams in Greece. J. Food Prot. 69, 2268–2273. doi: 10.4315/0362-028x-69.9.2268
Samelis, J., Kakouri, A., and Rementzis, J. (2000). Selective effect of the product type and the packaging conditions on the species of lactic acid bacteria dominating the spoilage microbial association of cooked meats at 4±C. Food Microbiol. 17, 329–340. doi: 10.1006/fmic.1999.0316
Shaw, B. G., and Harding, C. D. (1989). Leuconostoc gelidum sp. nov. and Leuconostoc carnosum sp. nov. from chill-stored meats. Int. J. Syst. Evol. Microbiol. 39, 217–223. doi: 10.1099/00207713-39-3-217
Singh, V. P., Pathak, V., and Verma, A. K. (2012). Fermented meat products: organoleptic qualities and biogenic amines-a review. Am. J. Food Technol. 7, 278–288. doi: 10.3923/ajft.2012.278.288
Smith, H. L. Jr., and Goodner, K. (1958). Detection of bacterial gelatinases by gelatin-agar plate methods. J. Bacteriol. 76, 662–665.
Szutowska, J., and Gwiazdowska, D. (2021). Probiotic potential of lactic acid bacteria obtained from fermented curly kale juice. Arch. Microbiol. 203, 975–988. doi: 10.1007/s00203-020-02095-4
Taverniti, V., and Guglielmetti, S. (2011). The immunomodulatory properties of probiotic microorganisms beyond their viability (ghost probiotics: proposal of paraprobiotic concept). Genes Nutr. 6, 261–274. doi: 10.1007/s12263-011-0218-x
Vasilopoulos, C., De Mey, E., Dewulf, L., Paelinck, H., De Smedt, A., Vandendriessche, F., et al. (2010). Interactions between bacterial isolates from modified-atmosphere-packaged artisan-type cooked ham in view of the development of a bioprotective culture. Food Microbiol. 27, 1086–1094. doi: 10.1016/j.fm.2010.07.013
Vecchione, A., Celandroni, F., Mazzantini, D., Senesi, S., Lupetti, A., and Ghelardi, E. (2018). Compositional quality and potential gastrointestinal behavior of probiotic products commercialized in Italy. Front. Med. (Lausanne) 5:59. doi: 10.3389/fmed.2018.00059
Wan, X., Saris, P. E. J., and Takala, T. M. (2015). Genetic characterization and expression of leucocin B, a class IId bacteriocin from Leuconostoc carnosum 4010. Res. Microbiol. 166, 494–503. doi: 10.1016/j.resmic.2015.04.003
Zarour, K., Benmechernene, Z., Hadadji, M., Moussa-Boudjemaa, B., Henni, D. J., and Kihal, M. (2012). Bioprospecting of Leuconostoc mesenteroides strains isolated from Algerian raw camel and goat milk for technological properties useful as adjunct starters. Afr. J. Microbiol. Res. 6, 3192–3201. doi: 10.5897/AJMR11.1542
Zheng, J., Wittouck, S., Salvetti, E., Franz, C. M. A. P., Harris, H. M. B., Mattarelli, P., et al. (2020). A taxonomic note on the genus Lactobacillus: description of 23 novel genera, emended description of the genus Lactobacillus Beijerinck 1901, and union of Lactobacillaceae and Leuconostocaceae. Int. J. Syst. Evol. Microbiol. 70, 2782–2858. doi: 10.1099/ijsem.0.004107
Keywords: Leuconostoc carnosum, growth kinetic, substrate preference, antibiotic resistance, biofilm, exopolysaccharide, immunomodulation
Citation: Raimondi S, Spampinato G, Candeliere F, Amaretti A, Brun P, Castagliuolo I and Rossi M (2021) Phenotypic Traits and Immunomodulatory Properties of Leuconostoc carnosum Isolated From Meat Products. Front. Microbiol. 12:730827. doi: 10.3389/fmicb.2021.730827
Received: 25 June 2021; Accepted: 27 July 2021;
Published: 25 August 2021.
Edited by:
Giorgio Giraffa, Council for Agricultural and Economics Research (CREA), ItalyReviewed by:
Flavio Tidona, Council for Agricultural and Economics Research (CREA), ItalyValentina Taverniti, University of Milan, Italy
Miriam Zago, Research Centre for Animal Production and Aquaculture, Italy
Copyright © 2021 Raimondi, Spampinato, Candeliere, Amaretti, Brun, Castagliuolo and Rossi. This is an open-access article distributed under the terms of the Creative Commons Attribution License (CC BY). The use, distribution or reproduction in other forums is permitted, provided the original author(s) and the copyright owner(s) are credited and that the original publication in this journal is cited, in accordance with accepted academic practice. No use, distribution or reproduction is permitted which does not comply with these terms.
*Correspondence: Maddalena Rossi, maddalena.rossi@unimore.it