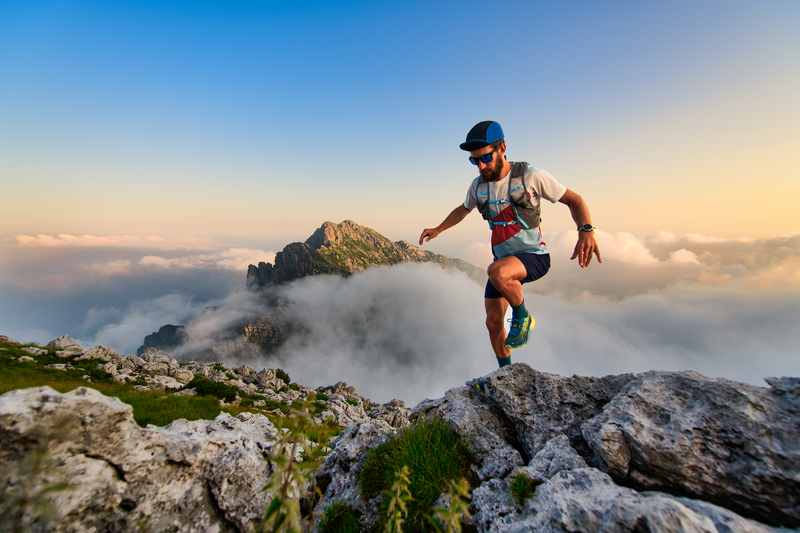
95% of researchers rate our articles as excellent or good
Learn more about the work of our research integrity team to safeguard the quality of each article we publish.
Find out more
REVIEW article
Front. Microbiol. , 25 August 2021
Sec. Infectious Agents and Disease
Volume 12 - 2021 | https://doi.org/10.3389/fmicb.2021.730543
This article is part of the Research Topic The Role of Glycans in Infectious Disease View all 15 articles
Arabinogalactans (AGs) are structural polysaccharides of the plant cell wall. A small proportion of the AGs are associated with hemicellulose and pectin. Furthermore, AGs are associated with proteins forming the so-called arabinogalactan proteins (AGPs), which can be found in the plant cell wall or attached through a glycosylphosphatidylinositol (GPI) anchor to the plasma membrane. AGPs are a family of highly glycosylated proteins grouped with cell wall proteins rich in hydroxyproline. These glycoproteins have important and diverse functions in plants, such as growth, cellular differentiation, signaling, and microbe-plant interactions, and several reports suggest that carbohydrate components are crucial for AGP functions. In beneficial plant-microbe interactions, AGPs attract symbiotic species of fungi or bacteria, promote the development of infectious structures and the colonization of root tips, and furthermore, these interactions can activate plant defense mechanisms. On the other hand, plants secrete and accumulate AGPs at infection sites, creating cross-links with pectin. As part of the plant cell wall degradation machinery, beneficial and pathogenic fungi and bacteria can produce the enzymes necessary for the complete depolymerization of AGs including endo-β-(1,3), β-(1,4) and β-(1,6)-galactanases, β-(1,3/1,6) galactanases, α-L-arabinofuranosidases, β-L-arabinopyranosidases, and β-D-glucuronidases. These hydrolytic enzymes are secreted during plant-pathogen interactions and could have implications for the function of AGPs. It has been proposed that AGPs could prevent infection by pathogenic microorganisms because their degradation products generated by hydrolytic enzymes of pathogens function as damage-associated molecular patterns (DAMPs) eliciting the plant defense response. In this review, we describe the structure and function of AGs and AGPs as components of the plant cell wall. Additionally, we describe the set of enzymes secreted by microorganisms to degrade AGs from AGPs and its possible implication for plant-microbe interactions.
Cell wall is an essential component of plant cells; they confer flexibility and mechanical support to the cell and perform important functions such as the maintenance of cell, preservation of osmotic pressure, movement of water and nutrients, management of intercellular communication between adjacent cells and prominent involvement in plant-microbe interactions, constituting the main barrier against potential pathogens (Burton et al., 2010; Keegstra, 2010).
Chemically, a plant cell wall (PCW) is composed of cellulosic polysaccharides (cellulose microfibrils from 40.6–51.2% of dry weight), non-cellulosic polysaccharides, lignin, and proteins. Non-cellulosic polysaccharides form a gel-like matrix with remarkable heterogeneity and structural complexity (Burton et al., 2010). The principal non-cellulosic polysaccharides are hemicelluloses (28.5–37.2% of dry weight), which comprise a complex of heteropolysaccharides (the second most abundant type of polysaccharide in nature) assembled into laterally branched and generally amorphous structures on a xylose backbone (xylan) or mannose and glucose backbones (mannan and glucomannan) with galactose, arabinose, and acetic/glucuronic acid ramifications. Depending on their structure, hemicelluloses are classified as xyloglucan, glucuronoxylan, glucuronoarabinoxylan, glucomannan, galactomannan, and β-(1,3; 1,4)-glucan (Pauly and Keegstra, 2008, 2016; Scheller and Ulvskov, 2010). On the other hand, pectin (30–35% of dry weight) constitutes a complex family of polysaccharides that are rich in galacturonic acid, including homogalacturonan, rhamnogalacturonan I and II (the substituted galacturonans), and xylogalacturonan (Mohnen, 2008; Chen, 2014). Finally, lignin (27–32% in woody plants and 15–30% in herbaceous plants) is a complex polymer composed of aromatic residues (coumaroyl alcohol, coniferyl alcohol, and synapyl alcohol) (Chen, 2014).
Plant cell wall proteins (CWPs) constitute ∼5–10% of dry weight of the PCW mass. Analysis of the Arabidopsis thaliana proteome has provided important information regarding the diversity of these proteins. CWPs are classified into nine functional classes according to their predicted domains, and their possible partners have been proposed. The nine CWPs classes are proteins that act on carbohydrates; oxide reductases, proteases, proteins with interaction domains, proteins potentially involved in signaling, structural proteins, proteins related to lipid metabolism, miscellaneous proteins, and proteins with unknown function (Jamet et al., 2008; Albenne et al., 2013). An alternative classification of non-enzymatic proteins associated with the PCW into two groups has been proposed: hydroxyproline-rich glycoproteins (HRGPs), also known as the HRGP superfamily; glycine-rich proteins (GRPs) or the GRP superfamily. HRGPs are classified according to their hydroxyproline/proline proportion into different subfamilies such as extensins, proline-rich proteins (PRPs), arabinogalactan proteins (AGPs), solanaceous lectins, and subcellular PELPK proteins (Pro-Glu-Leu/Ile/Val-Pro-Lys). Alternately, GRPs have been classified into five classes based on their Gly-rich repeats (Class I to V) (Rashid, 2016).
Due to its composition, the PCW represents a recalcitrant and complex structure that must be overcome during plant-microbe interactions. Microorganisms, mainly bacteria and fungi, are capable of producing and secreting a plethora of cell wall-degrading enzymes (CWDEs), which carry out a coordinated and synergistic deconstruction of the main structural polysaccharides of the PCW, producing soluble sugars that constitute an abundant source of organic carbon to guarantee their nutrition and survival (Gibson et al., 2011; Kubicek et al., 2014). The set of CWDEs includes cellulases, hemicellulases, pectinases, ligninases and accessory enzymes such as monooxygenases, which significantly increase the action of other polysaccharidases. These CWDEs have been described in several species of fungi and bacteria because they have great biotechnological potential (Malgas et al., 2017; Matias de Oliveira et al., 2018). Although it has not been precisely established whether all polysaccharidases constitute virulence factors, at least some such as endo-β-(1,4)-xylanases (Brito et al., 2006), pectin methyl esterases (Sella et al., 2016), arabinofuranosidases (Wu et al., 2016), and polygalacturonases, among others (Nakajima and Akutsu, 2013; Villa-Rivera et al., 2017a), are known to be essential for the establishment of the infection. Several studies have shown that plant pathogenic bacteria and fungi secrete a set of enzymes that degrade arabinogalactans from AGPs, however, little attention has been paid to their role in infection processes. Although there is evidence that AGPs play important functions in plant-microbe interactions, most studies on this topic have focused on the responses of plants to beneficial or pathogenic microorganisms. In this review, we describe the structure and function of AGs and AGPs as components of the PCW. Additionally, we describe the set of enzymes secreted by microorganisms to degrade AGs from AGPs and what is known regarding its role in plant-microbe interactions.
AGs are structural components of the PCW; they are mainly composed of galactose and arabinose and are ubiquitously distributed in the plant kingdom (Seifert and Roberts, 2007; Tan et al., 2012). Depending on their structure, AGs are grouped into three main types.
Arabinogalactan type I (AG type I), also designated arabino-4-galactan, is composed of a linear galactopyranose backbone linked by β-1,4 anchors and substituted with α<(1,5) arabinofuranosyl residues (Figure 1A; Clarke et al., 1979). Nevertheless, type I arabinogalactans from potato, soybean, onion and citrus also contain galactopyranose residues linked by β-(1,3) bonds as part of their main backbone (Hinz et al., 2005). AG type I have been shown to be a component of pectic complexes in seeds, bulbs, leaves, and coniferous wood (Clarke et al., 1979).
Figure 1. Structure of AGs and AGPs. (A) Structure of AG Type I. (B) Structure of AG Type II. (C) The third type of structure reported for AGs, (D) Protein core of AtAGP14 (Accession number: AAG24282). (E) Three-dimensional structure model of AtAGP16 (Accession number: AAG24284).
Arabinogalactan type II (AG type II) also known as arabino-3-6-galactan, consists of a main chain of D-galactopyranose linked by β-(1,3) bonds and branches of C(O)6 with β-(1,6)-galactosyl chains linked by β-(1,6) bonds. Non-reducing ends of the branches may present L-arabinopyranose, L-arabinofuranose, L-rhamnose, D-mannose, D-xylose, D-glucose, L-fucose, D-glucosamine, and D-glucuronic acid (Figure 1B; Clarke et al., 1979; Gaspar et al., 2001; Showalter, 2001; Seifert and Roberts, 2007). AG type II is found in mosses, coniferous woods, gums, saps, and exudates of angiosperms, organs such as seeds, leaves, roots, and fruits, as well as the media of various tissues in culture, particularly in polysaccharides with arabinogalactan side chains and pectic complexes such as rhamnogalacturonans (Clarke et al., 1979; Leivas et al., 2016).
Nuclear magnetic resonance (NMR) spectroscopy analyzes performed in different models have demonstrated substantial variability in the structure of AG type II; however, three common characteristics have been observed: first, a main backbone composed of two blocks of three galactopyranose residues linked by β-(1,3) bonds, with the junction between the three galactosyl blocks being a β-(1,6) bond; second, bifurcated branches of arabinose, rhamnose, glucuronic acid, and galactose anchored in the main chain at residues one and two of galactopyranoses; third, a common branch consisting of six residues of α-L-(1,5)-arabinofuranose and two α-L-(1,3)-arabinofuranoses grouped into one unit, with α-L-(1,4)-rhamnose, β-D-(1-6)-glucuronic acid forming a second unit. Both units are anchored to the main chain of β-(1,3)-galactopyranoses (Tan et al., 2010, 2012).
A third structure of AGs has been described, which is mainly associated with pectic polysaccharides in several plant species, consisting of a main backbone of D-galactopyranose residues linked by β-(1,6) bonds with side chains at position O-3 composed of L-arabinoses, arabinans, or individual D-galactopyranose units (Figure 1C; Raju and Davidson, 1994; Dong and Fang, 2001; Capek et al., 2009; de Oliveira et al., 2013).
AG type II are commonly anchored to a protein core, and these glycoproteins are denominated arabinogalactan proteins (AGPs). The protein core of AGPs (10% of AGPs) is a short backbone composed of 10 to 20 amino acids. The peptide undergoes posttranslational modifications: conversions of proline residues to hydroxyproline (forming the hydroxyproline domain) and the O-glycosylation of hydroxyproline and possibly serine and threonine residues with AG type II (90% of AGPs). In some cases, the protein core of AGPs contains within its structure a small sequence consisting of basic amino acids, and has a hydrophobic transmembrane domain located at the C-terminal end (Figures 1D,E; Gaspar et al., 2001; Showalter, 2001; Schultz et al., 2002; Showalter and Basu, 2016b). AGPs are found in the PCW, the apoplastic space, and some secretions such as exudates, and some adhere to the plasma membrane through a glycosylphosphatidylinositol (GPI) anchor in the hydrophobic domain. GPI binds to AGPs through a phosphoethanolamine linked to an oligosaccharide composed of D-mannose-(1-2)-α-D-mannose-(1,6)-α-D-mannose (1-4)-α-D-N-acetylglucosamine bound to a lipid residue of inositylphosphoceramide (Oxley and Bacic, 1999; Seifert and Roberts, 2007; Ellis et al., 2010). A consensus cleavage site has been identified in the classic AGP-deduced amino acid sequences, which constitutes a GPI-anchored recognition signal and is located before the transmembrane domain (Schultz et al., 2002).
AGPs are members of the HRGP superfamily, and based on their polypeptide core and the presence/absence of particular motifs/domains, they are classified into classic and non-classic AGPs (Showalter, 2001; Showalter et al., 2010). Classical AGPs are characterized by an N-terminal signal peptide, a central domain of variable length enriched in proline, alanine, serine, and threonine (PAST) (Figure 1D), and the C-terminal GPI anchor. Other classic AGPs are AG peptides, the structure of which contains 10–13 amino acids (Schultz et al., 2000). Conversely, non-classic AGPs have a low content of hydroxyproline and are enriched in cysteine and asparagine. Analyses of different plant tissues have revealed a high heterogeneity in the structure and composition of AGPs (both core proteins and carbohydrate moieties) (Gaspar et al., 2001; Showalter, 2001). Non-classical or chimeric AGPs have different conserved domains by which they are classified into subfamilies: lysine-rich AGPs with PAST domains separated by Lys-rich regions (Yang and Showalter, 2007), fasciclin-like arabinogalactan proteins (FLAs), with a fasciclin domain possibly involved in cell adhesion (Johnson et al., 2003), phytocyanin-like AGPs (PAGs) (Mashiguchi et al., 2009; Ma et al., 2017), and xylogen-like AGPs (XYLPs) with non-specific lipid transfer protein (nsLTP) domains (Kobayashi et al., 2011). AGPs-extensin hybrids (HAEs) have also been identified (Showalter et al., 2010).
Typical assays for the detection and/or functional analysis of AGPs use the dye β-glucosyl Yariv reagent (β-Glc Yariv), which binds to the β-(1,3)-galactose backbone (Yariv et al., 1967; Kitazawa et al., 2013) and/or specific antibodies against AGP-glycans (LM2, LM6, MAC207, JIM8, JIM13, and JM14). For example, these analyses have been conducted in suspension culture cells (Maurer et al., 2010) and different plant tissues, such as roots and seeds (van Hengel and Roberts, 2003), stems and wood (Zhang et al., 2003), leaves (Kremer et al., 2004), gametophytes and sporophytes (Lee et al., 2005), and flowers and embryos (Hu et al., 2006), among others. In addition, studies focused on the protein components of AGPs have used biochemical and molecular tools such as protein purification (Hu et al., 2006), isolation of genes and heterologous expression (Zhang et al., 2003), genetic expression (Pereira et al., 2006), genetic disruption of the core proteins (van Hengel and Roberts, 2003; Acosta-Garcia and Vielle-Calzada, 2004; Gaspar et al., 2004; Lee et al., 2005), overexpression (Park et al., 2003; Motose et al., 2004; Zhang et al., 2011), suppression (Li et al., 2010), and GFP labeling of genetic promotor sequences (Coimbra et al., 2008), among others. Furthermore, bioinformatics analyses of genomes and transcriptomes from several plant species have allowed the identification of thousands of candidate AGPs genes (Ma and Zhao, 2010; Showalter et al., 2010; Han et al., 2017; Johnson et al., 2017; Ma et al., 2017; Pfeifer et al., 2020). Therefore, AGPs are conserved in the plant kingdom and are expressed in different tissues and stages of plant development.
Regarding how AGPs work, it has also been proposed that soluble AGPs could be involved in cell-cell signaling (Schultz et al., 1998; Motose et al., 2004; Pereira et al., 2014), and GPI-AGPs in lipid rafts/nanodomains in eudicots could be involved in cell-cell communication, signal transduction, immune response, and transport (Borner et al., 2005; Grennan, 2007; Johnson et al., 2017). In support of these hypotheses, some studies have shown that classic AGPs bind reversibly to Ca2+ in a pH-dependent manner, suggesting that AGP- Ca2+ oscillators might integrate most signaling pathways downstream of the initial Ca2+ signal, which would explain the participation of AGPs in many biological process (Lamport and Varnai, 2013; Lamport et al., 2014). On the other hand, there is evidence in Arabidopsis that the mechanism responsible for clathrin-mediated endocytosis of extracellular lanthanum cargoes, requires extracellular AGPs anchored to the plasma membrane (Wang et al., 2019), and classic lysine-rich AtAGP18 could function as a coreceptor that binds to signaling molecules and interacts with transmembrane proteins, possibly receptor-like-kinases (RLKs) (Zhang et al., 2011). Specifically, it has been proposed that FLA AGPs are involved in cell-to-cell adhesion and cell signaling (Shi et al., 2003; Showalter and Basu, 2016a).
Currently, despite many studies on AGPs, it remains unclear whether their function resides in the protein backbones, in the glycan epitopes or both. However, given that the mass of AGPs constitutes more than 90% of sugars and that the oligosaccharides play a role in signal transduction in plants, it seems logical to consider these sugars as representatives of the interactive molecular surface defining their function in multiple plant processes. In this sense, heterologous expression studies, in vitro enzyme assay and analyses of knockout mutants of genes encoding Hyp-galactosyltransferases (GALTs and HPGTs) that specifically add galactose to AGPs in A. thaliana, have shown that glycosylation is essential for plant growth and development (Showalter and Basu, 2016b). On the other hand, enzymatic degradation of the AG type II of AGPs is a strategy that it utilized by microorganisms during their interactions with plants.
Fungi and bacteria are capable of synthesizing and secreting the enzymes necessary for complete hydrolysis of AG type II, and these enzymes are listed in Table 1 and schematized in Figure 2.
Table 1. Microorganisms that produce AG type II-degrading enzymes and the families of glycosyl hydrolases (GH) to which they belong.
Figure 2. Enzymes that degrade AG Type II. (A) S. avermitilis ABP, PDB code: 3A21. (B) Thermobacillus xylanolyticus ABF GH51, PDB code: 2VRQ. (C) Endo1,6GAL modeling of Colletotrichum lindemuthianum (Villa-Rivera et al., 2017b). (D) Aspergillus luchuensis ABF GH 54, PDB code: 1WD3. (E) Phanerochaete chrysosporium Exo1,3 GAL, PDB code: 7BYS.
Endo and exo-β-(1,3)-D-galactanases degrade the main β-(1,3)-D-galactose backbone of AG type II. Exo-β-(1,3)-D-galactanases (exo1,3 GAL) (EC 3.2.1.145) catalyze the sequential hydrolysis of β-(1,3) linkages at non-reducing ends, releasing galactose and, occasionally, β-(1,6)-galacto-oligosaccharides (Tsumuraya et al., 1990; Pellerin and Brillouet, 1994). Native and recombinant enzymes have been analyzed (heterologous expression in Escherichia coli and Pichia pastoris), and genes encoding the exo1,3GAL have also been characterized (Table 1). Analysis of the crystallized enzyme and deduced amino acid sequences of these enzymes have classified them into subfamily 24 of family 43 of glycosyl hydrolases (GH43) (Jiang et al., 2012; Matsuyama et al., 2020). The topology of exo1,3GAL consists of a catalytic domain structured in a five-blade propeller fold, with each blade including four-stranded antiparallel β-sheets to form a closed propeller ring with the putative catalytic site located in a central hole (Figure 2E; Jiang et al., 2012).
In addition, a C-terminal carbohydrate binding module (CBM) has been described as part of the three-dimensional structure of exo1,3GAL, classified as CBM13 in Clostridium thermocellum (symmetric β-trefoil fold topology) (Jiang et al., 2012) and CBM35 in Phanerochaete chrysosporium (β-jellyroll fold with a single calcium ion-binding site) (Figure 2E; Matsuyama et al., 2020). Interestingly, several studies have shown that typical side chains of AG type II do not interfere with the exo1,3Gal activity, and the enzyme structure contains a space capable of accommodating the β-(1,6)-galactose residues, which allows the protein to surpass the branches of the AG structure (Matsuyama et al., 2020). Nevertheless, the activity of exo1,3GAL has been shown to increase significantly in response to the action of β-(1,6)-D-galactanase, β-L-arabinopyranosidase and α-L-arabinofuranosidase (Okawa et al., 2013).
β-(1,3)-D-galactose chains are also depolymerized by endo-β-(1,3)-D-galactanases (Endo1,3GAL) (EC 3.2.1.145). The action of these enzymes releases β-(1,3)-D-galacto-oligosaccharides (galacto-hexoses at early steps) and sometimes galactose in an endo-manner (Kotake et al., 2011). Characterizations of the endo1,3GAL gene and protein have been conducted using only fungal species as a study model (Table 1). The synergistic activity of exo and endo1,3GAL has been suggested; apparently, endo1,3GAL creates internal breakpoints in the main chains of AG type II, increasing the number of attack sites for exo1,3GAL (Yoshimi et al., 2017).
The side chains of β-(1,6)-D-galactoses in AG type II are hydrolyzed by endo-β-(1,6)-galactanase (endo1,6GAL) (EC 3.2.1.164) and β-(1,6)-galactanase (1,6GAL). They catalyze the hydrolysis of the β-(1,6) anchors releasing galactobiose, galactooligosaccharides or galactose (Brillouet et al., 1991; Okemoto et al., 2003). The main difference between endo1,6GAL and 1,6GAL is that the former acts on chains with a degree of polymerization greater than or equal to three residues and releases galactooligosaccharides of 2 to 5 residues (Okemoto et al., 2003), while 1,6GAL catalyzes the hydrolysis of galactobiose to release monomers of galactose (Sakamoto et al., 2007; Sakamoto and Ishimaru, 2013). Endo1,6GAL and 1,6GAL are active only on dearabinosylated substrates (Brillouet et al., 1991; Luonteri et al., 2003); therefore, prior action of arabinofuranosidases is required and efficient removal of the side chains of AGP type II depends on the combined action of galactanases and arabinofuranosidases (Takata et al., 2010).
There are no crystallized structures of endo1,6GAL and 1,6GAL; however, a prediction of the three-dimensional structure of Colletotrichum lindemuthianum endo1,6GAL has been reported. The endo1,6GAL model adopts a (β/α)8 TIM barrel fold topology (eight-stranded parallel β-strand, forming a central barrel surrounded by eight α-helices) with a putative active site located at the C-terminus, which is consistent with the characteristic structure of GH30 family enzymes (Figure 2C; Villa-Rivera et al., 2017b). These enzymes have been characterized from fungi and bacteria and have also been expressed in heterologous models (Table 1).
Other enzymes involved in the depolymerization of AG type II are β-galactosidases (βGAL) (EC 3.2.1.23). Although these enzymes have mainly been characterized in plants (Gunter et al., 2009), Hypocrea jecorina β-GAL has been purified. The enzyme breaks β-D-galactose bonds at non-reducing ends; however, it is inhibited by its degradation product (β-D-galactose) (Gamauf et al., 2007).
The α-L-arabinofuranosidases (ABFs) (EC 3.2.1.55) are exo-type enzymes that remove the α-L-arabinosyl side chains linked through α-L-(1,2), α-L-(1.3), α-L-(1,5) O-glycosidic bonds at the non-reducing ends of arabinoxylans, arabinoxylo-oligosaccharides, arabinan, arabinogalactans and arabino-oligosaccharides of the PCW (Lagaert et al., 2014). According to the Carbohydrate-Active enZYmes database (CAZY), and based on their amino acid sequences, ABFs are classified into families 2, 3, 5, 10, 39, 43, 51, 54, and 62 of glycoside hydrolases1(Lombard et al., 2014). ABFs have been purified and characterized from bacteria, fungi and plants (Numan and Bhosle, 2006). Despite many reports on ABFs, only a few have measured the activity of native and recombinant ABFs using AGs as substrates (Table 1). Thus, traces of ABF activity in the presence of AGs have been reported in Aspergillus sojae and Aspergillus nidulans (Kimura et al., 1995; Wilkens et al., 2016), and additionally, AGs have been successfully evaluated as inducers of ABF activity in cultures of Aspergillus niger, Aureobasidium pullulans and Penicillium purpurogenum (vd Veen et al., 1991; Saha and Bothast, 1998; De Ioannes, 2000).
Based on the specificity of the substrate, ABFs are classified into three types: arabinofuranosidase A is capable of hydrolyzing α-(1,5)-L-arabinofuranosyl bonds of arabino-xylooligosaccharides but does not act on polysaccharides; arabinofuranosidase B acts on linear and branched arabino-oligosaccharides and polymers; and the third type of arabinofuranosidase shows a high specificity for complex natural substrates and is known generically as an arabinofuranohydrolase (Numan and Bhosle, 2006; Lagaert et al., 2014; Poria et al., 2020). The regional selectivity of ABFs has also been evaluated. Accordingly, ABFs belonging to GH51 and GH54 are capable of removing arabinosyl residues from the internal and terminal non-reducing ends of xylopyranosyl residues with mono- and disubstitutions. However, the ABFs of the GH54 family show weak activity on internal di-substitutions compared with GH51, which are more versatile in terms of substrate specificity (Koutaniemi and Tenkanen, 2016; Dos Santos et al., 2018). On the other hand, ABFs of the GH62 family show selectivity toward α-(1,2) and α-(1,3) anchors in arabinoxylans mono-substituted with arabinofuranosyl residues (Sarch et al., 2019). Interestingly, bifunctional enzymes with α-L-arabinofuranosidase/xylobiohydrolase (Ravanal et al., 2010) or α-L-arabinofuranosidase/β-xylosidase activities have also been described (Huy et al., 2013).
Several crystallized and characterized ABFs, which present a diversity of structures generally consisting of two domains: the catalytic domain and the arabinose binding module (ABD). The structure of the Streptomyces avermitilis ABF belonging to the GH43 family, presents a core catalytic domain composed of a five blanched β-propeller with an ABD similar to CBM42 located at the C-terminus adopting a β-trefoil fold (three similar subdomains assembled against one another around a pseudo3-fold axis) topology (Fujimoto et al., 2010). With respect to ABFs of the GH51 family, the enzymes from Geobacillus stearethermophilus (Hovel et al., 2003), Clostridium thermocellum (Taylor et al., 2006), and Thermobacillus xylanilyticus (Paes et al., 2008) have been crystallized. The catalytic domain of the GH51 family ABFs has a (β/α)8 TIM-barrel fold topology and a C-terminal ABD domain with a jellyroll topology (Figure 2B; Hovel et al., 2003; Paes et al., 2008). The structure of Aspergillus luchuensis ABFs (formerly known as A. kawachii), belonging to the GH54 family, is composed of a catalytic domain (β-sandwich fold topology) similar to clan B of GH54 and an arabinose binding module (β-trefoil fold topology) similar to CBM13 (Figure 2D; Miyanaga et al., 2004).
Regarding other accessory enzymes for the deconstruction of AG type II, β-L-arabinopyranosidases (ABPs) (EC3.2.1.88) have also been reported. ABP hydrolyzes β-arabinopyranose from the non-reducing end of AG side chains (Ichinose et al., 2009). Two bifunctional proteins (Fo/AP1 and Fo/AP2) with β-L-arabinopiranosidase/α-D-galactopyranosidase activity have been purified from Fusarium oxysporum, both of which are active toward larch wood arabinogalactan (LWAG), releasing only arabinopyranose (Sakamoto et al., 2010). ABPs have been purified and characterized from bacteria and fungi, and they have also been expressed in heterologous systems (Table 1). The three-dimensional structure of ABP consists in a catalytic domain (antiparallel β-domain) characteristic of the GH27 family and a CMB13 (antiparallel β-domain adopting a jellyroll structure) at the C-terminal end (Ichinose et al., 2009; Lansky et al., 2014; Figure 2A).
In bacteria, ABF and ABP enzymes are non-cellulosomal hydrolases; however, a synergy between cellulosomal and non-cellulosomal hydrolases has been detected during hydrolysis of the PCW by Clostridium cellulovorans (Kosugi et al., 2002). Finally, β-glucuronidase (EC3.2.1.31) hydrolyzes the non-reducing ends of 4-O-methyl glucuronic acid of the β-1,6 galactosyl side chains of AG type II (Table 1; Haque et al., 2005).
It is important to mention that the combined action of exo1,3GAL, 1,6GAL, ABF, and ABP is more efficient than the sum of the independent activities in the depolymerization of AG type II (Okawa et al., 2013). Efficient hydrolysis of this polymer depends of the removal of β-(1,6)-galactose branches (Sakamoto and Ishimaru, 2013).
AGPs play an interesting role in the response pathways of plants to abiotic stress (caused by low and high temperatures, drought, high salinity, excessive light, and floods) and biotic stress (caused by bacteria, fungi, nematodes, and viruses) (Mareri et al., 2018). Particularly, they are important for plant-microbe interactions, whether beneficial or pathogenic.
During beneficial plant-microbe interactions, plant roots produce a complex mucilage that constitutes an important carbon source for rhizosphere microorganisms. Root mucilage from pea, cowpea, wheat, maize, and rice has been reported to contain high levels of galactose and arabinose, the main components of AGs (Knee et al., 2001). Moreover, root tips and border-like cells (BLC) of A. thaliana can secrete pectic polysaccharides and AGPs to the rhizosphere (Vicre et al., 2005). Additionally, AGPs have been located in several root structures: epidermal, cortical, and endodermal cells, pericycle, apical meristem, and root infection structures (Nguema-Ona et al., 2013). In this sense, AGPs can act as attractants for symbiotic species of fungi and bacteria, promoting the development of infection structures and, therefore, root tip colonization. It has been observed that the induced alterations in the structure of AGPs trigger an inhibition in the attachment of Rhizobium to BLC and the root tip of A. thaliana (Vicre et al., 2005; Cannesan et al., 2012; Xie et al., 2012).
Furthermore, AGPs are found at the physical interface between root cells and the infecting structures of microorganisms, allowing for the root-symbiont nutrient exchange necessary for microbe survival. For this purpose, soil microbes such as Trichoderma viride and S. avermitilis, among other, produce polysaccharidases (Table 1) that allow them to access and obtain monosaccharides or disaccharides derived from the hydrolysis of AGs, useful as a carbon source (Knee et al., 2001). On the other hand, it has been suggested that AGPs can prevent infection by pathogenic microorganisms or inhibit their development because the degradation products (oligosaccharides or glycopeptides) generated by hydrolytic enzymes of pathogens can act as damage-associated molecular patterns (DAMPs) and promote the plant defense response. Moreover, the colonization of the rhizosphere by beneficial microbes supported by AGPs, would also activate plant defense mechanisms such as induced systemic resistance (ISR), protecting the plant against pathogen attack, while symbiotic microorganisms could act as antagonists of pathogens and avoid infections. Finally, AGPs have been proposed to be modulators of the plant immune system, favoring the colonization of beneficial microbes (Nguema-Ona et al., 2013).
Regarding the pathogen microbe-plant interaction, an accumulation of HRGPs has been detected as a result of the contact between the pathogen and PCW. For instance, during the infection of tomato roots with F. oxysporum, late accumulation of HRGPs has been observed in susceptible plants (Benhamou, 1990). Along the same lines, in response to F. oxysporum a consistent increase in the abundance of AGPs was observed, particularly in the roots of resistant cultivars of wax gourd (Benicasa hispida Cong.) but not in susceptible ones (Xie et al., 2011). Furthermore, immunohistochemical analysis of Sesbania exaltata tissues infected by Colletotrichum gloeosporioides has revealed a rapid accumulation of AGPs at the border between the vascular tissue and the necrotic lesion (Bowling et al., 2010). This evidence suggests that HRGP enhancement is a prerequisite for an efficient and localized plant defense response (Benhamou, 1990; Nguema-Ona et al., 2013). At the transcriptomic level, seven extensin genes and 23 genes encoding AGPs were differentially expressed in banana cultivars before and after infection with F. oxysporum. These data revealed that extensins and AGPs were dynamic components of the plant cell wall (Wu et al., 2017). In addition, 38 NbFLAs from Nicotiana benthamiana were significantly downregulated by infection with turnip mosaic virus (TuMV) or by infection with Pseudomonas syringae pv tomato strain DC3000 (Pst DC3000), suggesting a relationship between FLAs and immunity (Wu et al., 2017).
The accumulation of HRGPs at the infection sites can be explained because the PCW is not just a physical and passive barrier against pathogens; recently, this complex structure has emerged as a dynamic defense structure involved in sensing and monitoring stressing conditions that result in compensatory responses essential for the maintenance of cell integrity and stability. Abiotic and biotic factors, such as the disruption of the PCW during infection by pathogens, trigger molecular mechanisms of the signaling pathways that sense and maintain cell wall integrity, including sensors that detect changes in the cell surface and signals originating from the wall that transduce downstream signals (Bacete et al., 2018; Rui and Dinneny, 2020). Additionally, CWPs have an important role in defense against pathogens; this proteins carry out (a) a reinforcement of the plant cell wall through insolubilization and oxidative cross-linking of extensins and sensors resident in the plasma membrane (PRPs) through H2O2 and peroxidases, (b) AGP secretion and accumulation at sites of infection by pathogens (Figure 3A), (c) binding of GRPs to pathogenic RNA to degrade its genetic material and, (d) transcription of genes that encode pathogen-related proteins (PRs) using AGPs as a soluble molecular signal (Figure 3B; Rashid, 2016; Bacete et al., 2018).
Figure 3. Functions of AGPs during plant-microbe interactions. (A) Accumulation of AGPs at sites of pathogen infection. (B) AGPs are involved in transduction pathways and promote plant defense responses.
Thus, the role of AGPs in the defense responses of plants against pathogens comprises the secretion and clumping of AGPs at the infection sites and the creation of cross-links with cell wall polysaccharides such as pectin. Covalent bonds have been described between the AGP At3g4530 from A. thaliana, arabinoxylan and rhamnogalacturonan I/homogalacturonan, which form an APAP1 structure (Tan et al., 2013); therefore, the action of enzymes that degrade AGs is necessary to allow the pathogen to surpass the PCW and penetrate the protoplast.
On the other hand, AGPs and other proteins attached to the plasma membrane by GPI anchoring, are involved in connecting the intracellular and extracellular space, and are good candidates for transducing signals from the extracellular space to the cytoplasm. In this sense, the receptor like kinase (RLK) family, AGPs, the mitogen-activated protein kinase (MAPK) pathway, and the target of rapamycin (TOR) pathway could be potential components of perceptual mechanisms of cell wall integrity (Pogorelko et al., 2013). It has been reported that some AGPs contain a domain of six cysteine residues designated the proline-AGP-cysteine domain (PAC) (like that identified in Cys-rich LAT52); this domain can interact with RLK receptors (Tang et al., 2002). In this way, it has been suggested that PAC domain might mediate the binding between some AGPs and RLKs (Figure 3B; Seifert and Roberts, 2007). Moreover, the fasciclin-like AGP SOS 5, has been reported as a GPI anchored protein that acts in a pathway involving two cell wall RLKs (FEI1 and FEI2) (Showalter and Basu, 2016a).
Evidence for the role of AGPs in cell signaling during plant-microbe interactions shows that AGP17 from A. thaliana is necessary for the activation of systemic acquired resistance (SAR). This glycoprotein seems to be involved in the transduction pathway of intracellular changes in salicylic acid levels and genetic expression of the gene encoding PR1 (Gaspar et al., 2004). Furthermore, binding of β-GlcYariv to plant surface AGPs, triggers wound-like defense responses that include PCW reinforcement and callose synthesis (Guan and Nothnagel, 2004). In addition, treatment with β-Glc Yariv suppresses the expression of genes involved in gibberellin signaling, an effect similar to that caused by elicitors such as chitin (Mashiguchi et al., 2008).
As mentioned above, AG type II are essential components for the function of AGPs. In vitro assays using exo1,3GAL have shown that complete hydrolysis of arabinogalactan terminated its reactivity with β-Glc Yariv reagent, confirming that the AGP side chains are responsible for its activity (Kiyohara et al., 1997). On the other hand, the expression of a fungal exo1,3GAL in A. thaliana leads to a decrease in AGPs reactive to β-Glc Yariv and a severe tissue disorganization in hypocotyl and cotyledons. Furthermore, oligosaccharides released from AG type II were detected in the soluble fraction of transgenic plants (Yoshimi et al., 2020). Thus, hydrolysis of the carbohydrate component of AGPs by hydrolytic enzymes secreted by fungi and bacteria during penetration of the PCW would have consequences for the mechanisms of detection and monitoring of the integrity of the cell wall, favoring infection by pathogens. Damage to the PCW caused by the combination of cellulase and pectinase activities is responsible for the accumulation of jasmonic and salicylic acids in plants, and RLK (FEI2), and mechanosensitive Ca+2 channels localized in the plasma membrane (MCA1) are responsible for the activation of responses to damage (osmosensitive responses) (Engelsdorf et al., 2018).
Therefore, the secretion of AG type II-degrading enzymes by phytopathogens appears to be crucial for the establishment of host infection. Deletion of the MoAbfB gene encoding an α-N-arabinofuranosidase B from Magnaporthe oryzae resulted in a reduction in disease severity in rice (Wu et al., 2016). Likewise, comparison of the genetic expression of an endo1,6GAL between pathogenic and non-pathogenic strains of C. lindemuthianum, showed that compared with most of the evaluated conditions, the levels of genetic expression were higher in pathogenic than non-pathogenic strains, supporting a role for this enzyme in the PCW degradation during the establishment of the infection (Villa-Rivera et al., 2017b). Finally, the inactivation of Malus domestica AGPs with β-Glc Yariv reagent, which exhibit an effect similar to that of degradation by enzymes, causes a more rapid progress of Penicillium spinulosum infection, thus reinforcing the notion that the inactivation of AG type II has an impact on the PCW integrity and the activation of plant defense responses (Leszczuk et al., 2019).
Several lines of evidence support the proposal that AGPs play different and crucial roles in plant-microorganism interactions. In general, most studies on the role of AGPs in plant-microorganism interactions have focused on the response of plants. Evidence shown that in plant root tips AGPs are attractants of symbiotic species of fungi or bacteria and promoters of the development of infectious structures and colonization, as well, these interactions can activate plant defense mechanisms such as ISR. Furthermore, plants secrete and accumulate AGPs at infection sites, creating cross-links with pectin and probably other PCW polymers. In this sense, it is proposed that AGPs could prevent infection by pathogenic microorganisms because oligosaccharides or glycopeptides generated by hydrolytic enzymes of pathogens act as DAMPs and elicits the plant defense response. But then the question arises why the degradation of AGPs by successful pathogens is crucial for the establishment of the host infection. Moreover, the binding of β-Glc Yariv to AGPs on the plant surface elicits wound-like defense responses, yet, in contrast it can also promote a more rapid progress of fungal infections, similar to the action of the polysaccharidases. On the other hand, although it is well established that phytopathogenic and beneficial fungi and bacteria secrete a set of glycosyl hydrolases that degrade AG type II of AGPs, most of the reports available on these enzymes have focused on their biotechnological applications. Clearly, it is necessary to develop studies on the expression and secretion of AG type II-degrading enzymes, and on their degradation products and their role during the establishment of host infection.
MV-R and MZ-P wrote the first version of the manuscript and figures. MZ-P, HC-C, and EL-R corrected and improved the manuscript. All authors read and approved the submitted version of the manuscript.
Consejo Nacional de Ciencia y Tecnología (CONACYT, research project FONSEC-CB2017-2018-A1-S-27298 to MZ-P), and by Coordinación de la Investigación Científica de la Universidad Michoacana de San Nicolás de Hidalgo, (CIC-UMSNH, research project 2020–2021 to HC-C).
The authors declare that the research was conducted in the absence of any commercial or financial relationships that could be construed as a potential conflict of interest.
All claims expressed in this article are solely those of the authors and do not necessarily represent those of their affiliated organizations, or those of the publisher, the editors and the reviewers. Any product that may be evaluated in this article, or claim that may be made by its manufacturer, is not guaranteed or endorsed by the publisher.
Acosta-Garcia, G., and Vielle-Calzada, J. P. (2004). A classical arabinogalactan protein is essential for the initiation of female gametogenesis in Arabidopsis. Plant Cell 16, 2614–2628. doi: 10.1105/tpc.104.024588
Albenne, C., Canut, H., and Jamet, E. (2013). Plant cell wall proteomics: the leadership of Arabidopsis thaliana. Front. Plant Sci. 4:111. doi: 10.3389/fpls.2013.00111
Bacete, L., Melida, H., Miedes, E., and Molina, A. (2018). Plant cell wall-mediated immunity: cell wall changes trigger disease resistance responses. Plant J. 93, 614–636. doi: 10.1111/tpj.13807
Benhamou, N. (1990). Immunocytochemical localization of hydroxyproline-rich glycoproteins in tomato root cells infected by Fusarium oxysporum f. sp. radicis-lycopersici: study of a compatible interaction. Phytopathology 80, 163–173. doi: 10.1094/Phyto-80-163
Borner, G. H. H., Sherrier, D. J., Weimar, T., Michaelson, L. V., Hawkins, N. D., Macaskill, A., et al. (2005). Analysis of detergent-resistant membranes in Arabidopsis. Evidence for plasma membrane lipid rafts. Plant Physiol. 137, 104–116. doi: 10.1104/pp.104.053041
Bowling, A. J., Vaughn, K. C., Hoagland, R. E., Stetina, K., and Boyette, C. D. (2010). Immunohistochemical investigation of the necrotrophic phase of the fungus Colletotrichum gloeosporioides in the biocontrol of hemp sesbania (Sesbania exaltata. Papilionaceae). Am. J. Bot. 97, 1915–1925. doi: 10.3732/ajb.1000099
Brillouet, J.-M., Williams, P., and Moutounet, M. (1991). Purification and some properties of a novel endo-β-(1 → 6)-d-galactanase from Aspergillus niger. Agric. Biol. Chem. 55, 1565–1571. doi: 10.1080/00021369.1991.10870802
Brito, N., Espino, J. J., and Gonzalez, C. (2006). The endo-beta-1,4-xylanase xyn11A is required for virulence in Botrytis cinerea. Mol. Plant Microbe. Interact. 19, 25–32. doi: 10.1094/MPMI-19-0025
Burton, R. A., Gidley, M. J., and Fincher, G. B. (2010). Heterogeneity in the chemistry, structure and function of plant cell walls. Nat. Chem. Biol. 6, 724–732. doi: 10.1038/nchembio.439
Cannesan, M. A., Durand, C., Burel, C., Gangneux, C., Lerouge, P., Ishii, T., et al. (2012). Effect of arabinogalactan proteins from the root caps of pea and Brassica napus on Aphanomyces euteiches zoospore chemotaxis and germination. Plant Physiol. 159, 1658–1670. doi: 10.1104/pp.112.198507
Capek, P., Matulova, M., Navarini, L., and Liverani, F. S. (2009). A comparative study of arabinogalactan-protein isolates from instant coffee powder of Coffea arabica beans. J. Food Nutr. Res. 48, 80–86. doi: 10.1016/j.carbpol.2009.11.016
Chen, H. (2014). Chemical composition and structure of natural lignocellulose in Biotechnology of Lignocellulose. Dordrecht: Springer, 25–71.
Clarke, A. E., Anderson, R. L., and Stone, B. A. (1979). Form and function of arabinogalactans and arabinogalactan-proteins. Phytochemistry 18, 521–540. doi: 10.1016/s0031-9422(00)84255-7
Coimbra, S., Jones, B., and Pereira, L. G. (2008). Arabinogalactan proteins (AGPs) related to pollen tube guidance into the embryo sac in Arabidopsis. Plant Signal Behav. 3, 455–456. doi: 10.4161/psb.3.7.5601
De Ioannes, P. (2000). An α-L-arabinofuranosidase from Penicillium purpurogenum: production, purification and properties. J. Biotechnol. 76, 253–258. doi: 10.1016/s0168-1656(99)00190-x
de Oliveira, A. J., Cordeiro, L. M., Goncalves, R. A., Ceole, L. F., Ueda-Nakamura, T., and Iacomini, M. (2013). Structure and antiviral activity of arabinogalactan with (1–>6)-beta-D-galactan core from Stevia rebaudiana leaves. Carbohydr. Polym. 94, 179–184. doi: 10.1016/j.carbpol.2012.12.068
Degrassi, G., Vindigni, A., and Venturi, V. (2003). A thermostable α-arabinofuranosidase from xylanolytic Bacillus pumilus: purification and characterisation. J. Biotechnol. 101, 69–79. doi: 10.1016/s0168-1656(02)00304-8
Dong, Q., and Fang, J. N. (2001). Structural elucidation of a new arabinogalactan from the leaves of Nerium indicum. Carbohydr. Res. 332, 109–114. doi: 10.1016/s0008-6215(01)00073-8
Dos Santos, C. R., de Giuseppe, P. O., de Souza, F. H. M., Zanphorlin, L. M., Domingues, M. N., Pirolla, R. A. S., et al. (2018). The mechanism by which a distinguishing arabinofuranosidase can cope with internal di-substitutions in arabinoxylans. Biotechnol. Biofuels 11:223. doi: 10.1186/s13068-018-1212-y
Ellis, M., Egelund, J., Schultz, C. J., and Bacic, A. (2010). Arabinogalactan-proteins: key regulators at the cell surface? Plant Physiol. 153, 403–419. doi: 10.1104/pp.110.156000
Engelsdorf, T., Gigli-Bisceglia, N., Veerabagu, M., McKenna, J. F., Vaahtera, L., Augstein, F., et al. (2018). The plant cell wall integrity maintenance and immune signaling systems cooperate to control stress responses in Arabidopsis thaliana. Sci. Signal 11:aao3070. doi: 10.1126/scisignal.aao3070
Fujimoto, Z., Ichinose, H., Maehara, T., Honda, M., Kitaoka, M., and Kaneko, S. (2010). Crystal structure of an exo-1,5-α-L-arabinofuranosidase from Streptomyces avermitilis provides insights into the mechanism of substrate discrimination between exo- and endo-type enzymes in glycoside hydrolase family 43. J. Biol. Chem. 285, 34134–34143. doi: 10.1074/jbc.M110.164251
Fujita, K., Sakaguchi, T., Sakamoto, A., Shimokawa, M., and Kitahara, K. (2014). Bifidobacterium longum subsp. longum exo-beta-1,3-galactanase, an enzyme for the degradation of type II arabinogalactan. Appl. Environ. Microbiol. 80, 4577–4584. doi: 10.1128/AEM.00802-14
Gamauf, C., Marchetti, M., Kallio, J., Puranen, T., Vehmaanpera, J., Allmaier, G., et al. (2007). Characterization of the bga1-encoded glycoside hydrolase family 35 beta-galactosidase of Hypocrea jecorina with galacto-beta-D-galactanase activity. FEBS J. 274, 1691–1700. doi: 10.1111/j.1742-4658.2007.05714.x
Gaspar, Y., Johnson, K. L., McKenna, J. A., Bacic, A., and Schultz, C. J. (2001). “The complex structures of arabinogalactan-proteins and the journey towards understanding function.” in Plant Cell and Walls. eds N. C. Carpita, M. Campbell and M. Tierney. (Dordrecht: Springer), 161–176.
Gaspar, Y. M., Nam, J., Schultz, C. J., Lee, L. Y., Gilson, P. R., Gelvin, S. B., et al. (2004). Characterization of the Arabidopsis lysine-rich arabinogalactan-protein AtAGP17 mutant (rat1) that results in a decreased efficiency of Agrobacterium transformation. Plant Physiol. 135, 2162–2171. doi: 10.1104/pp.104.045542
Gibson, D. M., King, B. C., Hayes, M. L., and Bergstrom, G. C. (2011). Plant pathogens as a source of diverse enzymes for lignocellulose digestion. Curr. Opin. Microbiol. 14, 264–270. doi: 10.1016/j.mib.2011.04.002
Grennan, A. K. (2007). Lipid rafts in plants. Plant Physiol. 143, 1083–1085. doi: 10.1104/pp.104.900218
Guan, Y., and Nothnagel, E. A. (2004). Binding of arabinogalactan proteins by Yariv phenylglycoside triggers wound-like responses in Arabidopsis cell cultures. Plant Physiol. 135, 1346–1366. doi: 10.1104/pp.104.039370
Gunter, E. A., Popeyko, O. V., and Ovodov, Y. S. (2009). Action of beta-galactosidase in medium on the Lemna minor (L.) callus polysaccharides. Carbohydr. Res. 344, 2602–2605. doi: 10.1016/j.carres.2009.08.031
Han, T., Dong, H., Cui, J., Li, M., Lin, S., Cao, J., et al. (2017). Genomic, molecular evolution, and expression analysis of genes encoding putative classical AGPs, lysine-rich AGPs, and AG peptides in Brassica rapa. Front. Plant Sci. 8:397. doi: 10.3389/fpls.2017.00397
Haque, M., Kotake, T., and Tsumuraya, Y. (2005). Mode of action of beta-glucuronidase from Aspergillus niger on the sugar chains of arabinogalactan-protein. Biosci. Biotechnol. Biochem. 69, 2170–2177. doi: 10.1271/bbb.69.2170
Hashimoto, T., and Nakata, Y. (2003). Synergistic degradation of arabinoxylan with alpha-L-arabinofuranosidase, xylanase and beta-xylosidase from soy sauce koji mold. Aspergillus oryzae, in high salt condition. J. Biosci. Bioeng. 95, 164–169. doi: 10.1016/s1389-1723(03)80123-8
Hinz, S. W., Verhoef, R., Schols, H. A., Vincken, J. P., and Voragen, A. G. (2005). Type I arabinogalactan contains beta-D-Galp-(1–>3)-beta-D-Galp structural elements. Carbohydr. Res. 340, 2135–2143. doi: 10.1016/j.carres.2005.07.003
Hovel, K., Shallom, D., Niefind, K., Belakhov, V., Shoham, G., Baasov, T., et al. (2003). Crystal structure and snapshots along the reaction pathway of a family 51 alpha-L-arabinofuranosidase. EMBO J. 22, 4922–4932. doi: 10.1093/emboj/cdg494
Hu, Y., Qin, Y., and Zhao, J. (2006). Localization of an arabinogalactan protein epitope and the effects of Yariv phenylglycoside during zygotic embryo development of Arabidopsis thaliana. Protoplasma 229, 21–31. doi: 10.1007/s00709-006-0185-z
Huy, N. D., Thayumanavan, P., Kwon, T. H., and Park, S. M. (2013). Characterization of a recombinant bifunctional xylosidase/arabinofuranosidase from Phanerochaete chrysosporium. J. Biosci. Bioeng. 116, 152–159. doi: 10.1016/j.jbiosc.2013.02.004
Ichinose, H., Fujimoto, Z., Honda, M., Harazono, K., Nishimoto, Y., Uzura, A., et al. (2009). A beta-L-Arabinopyranosidase from Streptomyces avermitilis is a novel member of glycoside hydrolase family 27. J. Biol. Chem. 284, 25097–25106. doi: 10.1074/jbc.M109.022723
Ichinose, H., Kotake, T., Tsumuraya, Y., and Kaneko, S. (2006a). Characterization of an exo-beta-1,3-D-galactanase from Streptomyces avermitilis NBRC14893 acting on arabinogalactan-proteins. Biosci. Biotechnol. Biochem. 70, 2745–2750. doi: 10.1271/bbb.60365
Ichinose, H., Kotake, T., Tsumuraya, Y., and Kaneko, S. (2008). Characterization of an endo-beta-1,6-Galactanase from Streptomyces avermitilis NBRC14893. Appl. Environ. Microbiol. 74, 2379–2383. doi: 10.1128/AEM.01733-07
Ichinose, H., Kuno, A., Kotake, T., Yoshida, M., Sakka, K., Hirabayashi, J., et al. (2006b). Characterization of an exo-beta-1,3-galactanase from Clostridium thermocellum. Appl. Environ. Microbiol. 72, 3515–3523. doi: 10.1128/AEM.72.5.3515-3523.2006
Ichinose, H., Yoshida, M., Kotake, T., Kuno, A., Igarashi, K., Tsumuraya, Y., et al. (2005). An exo-beta-1,3-galactanase having a novel beta-1,3-galactan-binding module from Phanerochaete chrysosporium. J. Biol. Chem. 280, 25820–25829. doi: 10.1074/jbc.M501024200
Inacio, J. M., Correia, I. L., and de Sa-Nogueira, I. (2008). Two distinct arabinofuranosidases contribute to arabino-oligosaccharide degradation in Bacillus subtilis. Microbiology 154, 2719–2729. doi: 10.1099/mic.0.2008/018978-0
Ishida, T., Fujimoto, Z., Ichinose, H., Igarashi, K., Kaneko, S., and Samejima, M. (2009). Crystallization of selenomethionyl exo-beta-1,3-galactanase from the basidiomycete Phanerochaete chrysosporium. Acta Crystallogr. Sect. F Struct. Biol. Cryst. Commun. 65, 1274–1276. doi: 10.1107/S1744309109043395
Jamet, E., Albenne, C., Boudart, G., Irshad, M., Canut, H., and Pont-Lezica, R. (2008). Recent advances in plant cell wall proteomics. Proteomics 8, 893–908. doi: 10.1002/pmic.200700938
Jiang, D., Fan, J., Wang, X., Zhao, Y., Huang, B., Liu, J., et al. (2012). Crystal structure of 1,3Gal43A, an exo-beta-1,3-galactanase from Clostridium thermocellum. J. Struct. Biol. 180, 447–457. doi: 10.1016/j.jsb.2012.08.005
Johnson, K. L., Cassin, A. M., Lonsdale, A., Wong, G. K.-S., Soltis, D. E., Miles, N. W., et al. (2017). Insights into the evolution of hydroxyproline-rich glycoproteins from 1000 plant transcriptomes. Plant Physiol. 174, 904–921. doi: 10.1104/pp.17.00295
Johnson, K. L., Jones, B. J., Bacic, A., and Schultz, C. J. (2003). The fasciclin-like arabinogalactan proteins of Arabidopsis. A multigene family of putative cell adhesion molecules. Plant Physiol. 133, 1911–1925. doi: 10.1104/pp.103.031237
Kimura, I., Sasahara, H., and Tajima, S. (1995). Purification and characterization of two xylanases and an arabinofuranosidase from Aspergillus sojae. J. Ferment. Bioeng. 80, 334–339. doi: 10.1016/0922-338x(95)94200-b
Kitazawa, K., Tryfona, T., Yoshimi, Y., Hayashi, Y., Kawauchi, S., Antonov, L., et al. (2013). beta-galactosyl Yariv reagent binds to the beta-1,3-galactan of arabinogalactan proteins. Plant Physiol. 161, 1117–1126. doi: 10.1104/pp.112.211722
Kiyohara, H., Zhang, Y., and Yamada, H. (1997). Effect of exo-β-D-(1→3)-galactanase digestion on complement activating activity of neutral arabinogalactan unit in a pectic arabinogalactan from roots of Angelica acutiloba Kitagawa. Carbohydr. Polym. 32, 249–253. doi: 10.1016/s0144-8617(97)00004-0
Knee, E. M., Gong, F. C., Gao, M., Teplitski, M., Jones, A. R., Foxworthy, A., et al. (2001). Root mucilage from pea and its utilization by rhizosphere bacteria as a sole carbon source. Mol. Plant Microbe. Interact. 14, 775–784. doi: 10.1094/MPMI.2001.14.6.775
Kobayashi, Y., Motose, H., Iwamoto, K., and Fukuda, H. (2011). Expression and genome-wide analysis of the xylogen-type gene family. Plant Cell Physiol. 52, 1095–1106. doi: 10.1093/pcp/pcr060
Konishi, T., Kotake, T., Soraya, D., Matsuoka, K., Koyama, T., Kaneko, S., et al. (2008). Properties of family 79 beta-glucuronidases that hydrolyze beta-glucuronosyl and 4-O-methyl-beta-glucuronosyl residues of arabinogalactan-protein. Carbohydr. Res. 343, 1191–1201. doi: 10.1016/j.carres.2008.03.004
Kosugi, A., Murashima, K., and Doi, R. H. (2002). Characterization of two noncellulosomal subunits. ArfA and BgaA, from Clostridium cellulovorans that cooperate with the cellulosome in plant cell wall degradation. J. Bacteriol. 184, 6859–6865. doi: 10.1128/jb.184.24.6859-6865.2002
Kotake, T., Hirata, N., Degi, Y., Ishiguro, M., Kitazawa, K., Takata, R., et al. (2011). Endo-beta-1,3-galactanase from winter mushroom Flammulina velutipes. J. Biol. Chem. 286, 27848–27854. doi: 10.1074/jbc.M111.251736
Kotake, T., Kaneko, S., Kubomoto, A., Haque, M. A., Kobayashi, H., and Tsumuraya, Y. (2004). Molecular cloning and expression in Escherichia coli of a Trichoderma viride endo-beta-(1–>6)-galactanase gene. Biochem. J. 377, 749–755. doi: 10.1042/BJ20031145
Kotake, T., Kitazawa, K., Takata, R., Okabe, K., Ichinose, H., Kaneko, S., et al. (2009). Molecular cloning and expression in Pichia pastoris of a Irpex lacteus exo-beta-(1–>3)-galactanase gene. Biosci. Biotechnol. Biochem. 73, 2303–2309. doi: 10.1271/bbb.90433
Koutaniemi, S., and Tenkanen, M. (2016). Action of three GH51 and one GH54 alpha-arabinofuranosidases on internally and terminally located arabinofuranosyl branches. J. Biotechnol. 229, 22–30. doi: 10.1016/j.jbiotec.2016.04.050
Kremer, C., Pettolino, F., Bacic, A., and Drinnan, A. (2004). Distribution of cell wall components in Sphagnum hyaline cells and in liverwort and hornwort elaters. Planta 219, 1023–1035. doi: 10.1007/s00425-004-1308-4
Kubicek, C. P., Starr, T. L., and Glass, N. L. (2014). Plant cell wall-degrading enzymes and their secretion in plant-pathogenic fungi. Annu. Rev. Phytopathol. 52, 427–451. doi: 10.1146/annurev-phyto-102313-045831
Kuroyama, H., Tsutsui, N., Hashimoto, Y., and Tsumuraya, Y. (2001). Purification and characterization of a β-glucuronidase from Aspergillus niger. Carbohydr. Res. 333, 27–39. doi: 10.1016/s0008-6215(01)00114-8
Lagaert, S., Pollet, A., Courtin, C. M., and Volckaert, G. (2014). beta-xylosidases and alpha-L-arabinofuranosidases: accessory enzymes for arabinoxylan degradation. Biotechnol. Adv. 32, 316–332. doi: 10.1016/j.biotechadv.2013.11.005
Lamport, D. T., and Varnai, P. (2013). Periplasmic arabinogalactan glycoproteins act as a calcium capacitor that regulates plant growth and development. New Phytol. 197, 58–64. doi: 10.1111/nph.12005
Lamport, D. T. A., Varnai, P., and Seal, C. E. (2014). Back to the future with the AGP-Ca2+ flux capacitor. Ann. Bot. 114, 1069–1085. doi: 10.1093/aob/mcu161
Lansky, S., Salama, R., Solomon, H. V., Feinberg, H., Belrhali, H., Shoham, Y., et al. (2014). Structure-specificity relationships in Abp, a GH27 beta-L-arabinopyranosidase from Geobacillus stearothermophilus T6. Acta Crystallogr. Sect. D Biol. Crystallogr. 70, 2994–3012. doi: 10.1107/S139900471401863X
Lee, K. J. D., Sakata, Y., Mau, S.-L., Pettolino, F., Bacic, A., Quatrano, R. S., et al. (2005). Arabinogalactan proteins are required for apical cell extension in the moss Physcomitrella patens. Plant Cell 17, 3051–3065. doi: 10.1105/tpc.105.034413
Leivas, C. L., Iacomini, M., and Cordeiro, L. M. (2016). Pectic type II arabinogalactans from starfruit (Averrhoa carambola L.). Food Chem. 199, 252–257. doi: 10.1016/j.foodchem.2015.12.020
Leszczuk, A., Pieczywek, P. M., Gryta, A., Frac, M., and Zdunek, A. (2019). Immunocytochemical studies on the distribution of arabinogalactan proteins (AGPs) as a response to fungal infection in Malus x domestica fruit. Sci. Rep. 9:17428. doi: 10.1038/s41598-019-54022-3
Li, Y., Liu, D., Tu, L., Zhang, X., Wang, L., Zhu, L., et al. (2010). Suppression of GhAGP4 gene expression repressed the initiation and elongation of cotton fiber. Plant Cell Rep. 29, 193–202. doi: 10.1007/s00299-009-0812-1
Ling, N. X., Lee, J., Ellis, M., Liao, M. L., Mau, S. L., Guest, D., et al. (2012). An exo-beta-(1–>3)-D-galactanase from Streptomyces sp. provides insights into type II arabinogalactan structure. Carbohydr. Res. 352, 70–81. doi: 10.1016/j.carres.2012.02.033
Lombard, V., Golaconda Ramulu, H., Drula, E., Coutinho, P. M., and Henrissat, B. (2014). The carbohydrate-active enzymes database (CAZy) in 2013. Nucleic Acids Res. 42, D490–D495. doi: 10.1093/nar/gkt1178
Luonteri, E. (1998). Substrate specificities of Aspergillus terreus α-arabinofuranosidases. Carbohydr. Polym. 37, 131–141. doi: 10.1016/s0144-8617(98)00052-6
Luonteri, E., Laine, C., Uusitalo, S., Teleman, A., Siika-aho, M., and Tenkanen, M. (2003). Purification and characterization of Aspergillus β-D-galactanases acting on β-1,4- and β-1,3/6-linked arabinogalactans. Carbohydr. Polym. 53, 155–168. doi: 10.1016/s0144-8617(02)00303-x
Ma, H., and Zhao, J. (2010). Genome-wide identification, classification, and expression analysis of the arabinogalactan protein gene family in rice (Oryza sativa L.). J. Exp. Bot. 61, 2647–2668. doi: 10.1093/jxb/erq104
Ma, Y., Yan, C., Li, H., Wu, W., Liu, Y., Wang, Y., et al. (2017). Bioinformatics prediction and evolution analysis of arabinogalactan proteins in the plant kingdom. Front. Plant Sci. 8:66. doi: 10.3389/fpls.2017.00066
Malgas, S., Thoresen, M., van Dyk, J. S., and Pletschke, B. I. (2017). Time dependence of enzyme synergism during the degradation of model and natural lignocellulosic substrates. Enzyme Microb. Technol. 103, 1–11. doi: 10.1016/j.enzmictec.2017.04.007
Mareri, L., Romi, M., and Cai, G. (2018). Arabinogalactan proteins: actors or spectators during abiotic and biotic stress in plants? Plant Biosyst. 153, 173–185. doi: 10.1080/11263504.2018.1473525
Mashiguchi, K., Asami, T., and Suzuki, Y. (2009). Genome-wide identification, structure and expression studies, and mutant collection of 22 early nodulin-like protein genes in Arabidopsis. Biosci. Biotechnol. Biochem. 73, 2452–2459. doi: 10.1271/bbb.90407
Mashiguchi, K., Urakami, E., Hasegawa, M., Sanmiya, K., Matsumoto, I., Yamaguchi, I., et al. (2008). Defense-related signaling by interaction of arabinogalactan proteins and beta-glucosyl Yariv reagent inhibits gibberellin signaling in barley aleurone cells. Plant Cell Physiol. 49, 178–190. doi: 10.1093/pcp/pcm175
Matias de Oliveira, D., Rodrigues Mota, T., Marchiosi, R., Ferrarese-Filho, O., Dantas, et al. (2018). Plant cell wall composition and enzymatic deconstruction. AIMS Bioeng. 5, 63–77. doi: 10.3934/bioeng.2018.1.63
Matsuo, N., Kaneko, S., Kuno, A., Kobayashi, H., and Kusakabe, I. (2000). Purification, characterization and gene cloning of two alpha-L-arabinofuranosidases from Streptomyces chartreusis GS901. Biochem. J. 346, 9–15.
Matsuyama, K., Kishine, N., Fujimoto, Z., Sunagawa, N., Kotake, T., Tsumuraya, Y., et al. (2020). Unique active-site and subsite features in the arabinogalactan-degrading GH43 exo-beta-1,3-galactanase from Phanerochaete chrysosporium. J. Biol. Chem. 295, 18539–18552. doi: 10.1074/jbc.RA120.016149
Maurer, J. B. B., Bacic, A., Pereira-Netto, A. B., Donatti, L., Zawadzki-Baggio, S. F., and Pettolino, F. A. (2010). Arabinogalactan-proteins from cell suspension cultures of Araucaria angustifolia. Phytochemistry 71, 1400–1409. doi: 10.1016/j.phytochem.2010.04.021
McKee, L. S., and Brumer, H. (2015). Growth of Chitinophaga pinensis on plant cell wall glycans and characterisation of a glycoside hydrolase family 27 beta-L-arabinopyranosidase implicated in arabinogalactan utilisation. PLoS One 10:e0139932. doi: 10.1371/journal.pone.0139932
Miyanaga, A., Koseki, T., Matsuzawa, H., Wakagi, T., Shoun, H., and Fushinobu, S. (2004). Crystal structure of a family 54 alpha-L-arabinofuranosidase reveals a novel carbohydrate-binding module that can bind arabinose. J. Biol. Chem. 279, 44907–44914. doi: 10.1074/jbc.M405390200
Mohnen, D. (2008). Pectin structure and biosynthesis. Curr. Opin. Plant Biol. 11, 266–277. doi: 10.1016/j.pbi.2008.03.006
Motose, H., Sugiyama, M., and Fukuda, H. (2004). A proteoglycan mediates inductive interaction during plant vascular development. Nature 429, 873–878. doi: 10.1038/nature02613
Nakajima, M., and Akutsu, K. (2013). Virulence factors of Botrytis cinerea. J. Gen. Plant Pathol. 80, 15–23. doi: 10.1007/s10327-013-0492-0
Nguema-Ona, E., Vicre-Gibouin, M., Cannesan, M. A., and Driouich, A. (2013). Arabinogalactan proteins in root-microbe interactions. Trends Plant Sci. 18, 440–449. doi: 10.1016/j.tplants.2013.03.006
Numan, M. T., and Bhosle, N. B. (2006). Alpha-L-arabinofuranosidases: the potential applications in biotechnology. J. Ind. Microbiol. Biotechnol. 33, 247–260. doi: 10.1007/s10295-005-0072-1
Okawa, M., Fukamachi, K., Tanaka, H., and Sakamoto, T. (2013). Identification of an exo-β-1,3-D-galactanase from Fusarium oxysporum and the synergistic effect with related enzymes on degradation of type II arabinogalactan. Appl. Microbiol. Biotechnol. 97, 9685–9694. doi: 10.1007/s00253-013-4759-3
Okemoto, K., Uekita, T., Tsumuraya, Y., Hashimoto, Y., and Kasama, T. (2003). Purification and characterization of an endo-β-(1→6)-galactanase from Trichoderma viride. Carbohydr. Res. 338, 219–230. doi: 10.1016/s0008-6215(02)00405-6
Oxley, D., and Bacic, A. (1999). Structure of the glycosylphosphatidylinositol anchor of an arabinogalactan protein from Pyrus communis suspension-cultured cells. Proc. Natl. Acad. Sci. USA 96, 14246–14251. doi: 10.1073/pnas.96.25.14246
Paes, G., Skov, L. K., O’Donohue, M. J., Remond, C., Kastrup, J. S., Gajhede, M., et al. (2008). The structure of the complex between a branched pentasaccharide and Thermobacillus xylanilyticus GH-51 arabinofuranosidase reveals xylan-binding determinants and induced fit. Biochemistry 47, 7441–7451. doi: 10.1021/bi800424e
Park, M. H., Suzuki, Y., Chono, M., Knox, J. P., and Yamaguchi, I. (2003). CsAGP1, a gibberellin-responsive gene from cucumber hypocotyls, encodes a classical arabinogalactan protein and is involved in stem elongation. Plant Physiol. 131, 1450–1459. doi: 10.1104/pp.015628
Pauly, M., and Keegstra, K. (2008). Cell-wall carbohydrates and their modification as a resource for biofuels. Plant J. 54, 559–568. doi: 10.1111/j.1365-313X.2008.03463.x
Pauly, M., and Keegstra, K. (2016). Biosynthesis of the plant cell wall matrix polysaccharide xyloglucan. Annu. Rev. Plant. Biol. 67, 235–259. doi: 10.1146/annurev-arplant-043015-112222
Pellerin, P., and Brillouet, J.-M. (1994). Purification and properties of an exo-(1→3)-β-d-galactanase from Aspergillus niger. Carbohydr. Res. 264, 281–291. doi: 10.1016/S0008-6215(05)80012-6
Pereira, A. M., Masiero, S., Nobre, M. S., Costa, M. L., Solis, M. T., Testillano, P. S., et al. (2014). Differential expression patterns of arabinogalactan proteins in Arabidopsis thaliana reproductive tissues. J. Exp. Bot. 65, 5459–5471. doi: 10.1093/jxb/eru300
Pereira, L. G., Coimbra, S., Oliveira, H., Monteiro, L., and Sottomayor, M. (2006). Expression of arabinogalactan protein genes in pollen tubes of Arabidopsis thaliana. Planta 223, 374–380. doi: 10.1007/s00425-005-0137-4
Pfeifer, L., Shafee, T., Johnson, K. L., Bacic, A., and Classen, B. (2020). Arabinogalactan-proteins of Zostera marina L. contain unique glycan structures and provide insight into adaption processes to saline environments. Sci. Rep. 10:8232. doi: 10.1038/s41598-020-65135-5
Pogorelko, G., Lionetti, V., Bellincampi, D., and Zabotina, O. (2013). Cell wall integrity: targeted post-synthetic modifications to reveal its role in plant growth and defense against pathogens. Plant Signal. Behav. 8:25435. doi: 10.4161/psb.25435
Poria, V., Saini, J. K., Singh, S., Nain, L., and Kuhad, R. C. (2020). Arabinofuranosidases: Characteristics, microbial production, and potential in waste valorization and industrial applications. Bioresour. Technol. 304:123019. doi: 10.1016/j.biortech.2020.123019
Raju, T. S., and Davidson, E. A. (1994). Structural features of water-soluble novel polysaccharide components from the leaves of Tridax procumbens Linn. Carbohydr. Res. 258, 243–254. doi: 10.1016/0008-6215(94)84090-3
Rashid, A. (2016). Defense responses of plant cell wall non-catalytic proteins against pathogens. Physiol. Mol. Plant Pathol. 94, 38–46. doi: 10.1016/j.pmpp.2016.03.009
Ravanal, M. C., Callegari, E., and Eyzaguirre, J. (2010). Novel bifunctional alpha-L-arabinofuranosidase/xylobiohydrolase (ABF3) from Penicillium purpurogenum. Appl. Environ. Microbiol. 76, 5247–5253. doi: 10.1128/AEM.00214-10
Rui, Y., and Dinneny, J. R. (2020). A wall with integrity: surveillance and maintenance of the plant cell wall under stress. New Phytol. 225, 1428–1439. doi: 10.1111/nph.16166
Saha, B. C., and Bothast, R. J. (1998). Effect of carbon source on production of alpha-L-arabinofuranosidase by Aureobasidium pullulans. Curr. Microbiol. 37, 337–340. doi: 10.1007/s002849900388
Sakamoto, T., Inui, M., Yasui, K., Hosokawa, S., and Ihara, H. (2013). Substrate specificity and gene expression of two Penicillium chrysogenum alpha-L-arabinofuranosidases (AFQ1 and AFS1) belonging to glycoside hydrolase families 51 and 54. Appl. Microbiol. Biotechnol. 97, 1121–1130. doi: 10.1007/s00253-012-3978-3
Sakamoto, T., and Ishimaru, M. (2013). Peculiarities and applications of galactanolytic enzymes that act on type I and II arabinogalactans. Appl. Microbiol. Biotechnol. 97, 5201–5213. doi: 10.1007/s00253-013-4946-2
Sakamoto, T., and Kawasaki, H. (2003). Purification and properties of two type-B α-l-arabinofuranosidases produced by Penicillium chrysogenum. Biochim. Biophys. Acta 1621, 204–210. doi: 10.1016/s0304-4165(03)00058-8
Sakamoto, T., Tanaka, H., Nishimura, Y., Ishimaru, M., and Kasai, N. (2011). Characterization of an exo-beta-1,3-D: -galactanase from Sphingomonas sp. 24T and its application to structural analysis of larch wood arabinogalactan. Appl. Microbiol. Biotechnol. 90, 1701–1710. doi: 10.1007/s00253-011-3219-1
Sakamoto, T., Taniguchi, Y., Suzuki, S., Ihara, H., and Kawasaki, H. (2007). Characterization of Fusarium oxysporum beta-1,6-galactanase, an enzyme that hydrolyzes larch wood arabinogalactan. Appl. Environ. Microbiol. 73, 3109–3112. doi: 10.1128/AEM.02101-06
Sakamoto, T., Tsujitani, Y., Fukamachi, K., Taniguchi, Y., and Ihara, H. (2010). Identification of two GH27 bifunctional proteins with beta-L-arabinopyranosidase/alpha-D-galactopyranosidase activities from Fusarium oxysporum. Appl. Microbiol. Biotechnol. 86, 1115–1124. doi: 10.1007/s00253-009-2344-6
Salama, R., Alalouf, O., Tabachnikov, O., Zolotnitsky, G., Shoham, G., and Shoham, Y. (2012). The abp gene in Geobacillus stearothermophilus T-6 encodes a GH27 beta-L-arabinopyranosidase. FEBS Lett. 586, 2436–2442. doi: 10.1016/j.febslet.2012.05.062
Sarch, C., Suzuki, H., Master, E. R., and Wang, W. (2019). Kinetics and regioselectivity of three GH62 alpha-L-arabinofuranosidases from plant pathogenic fungi. Biochim. Biophys. Acta Gen. Subj. 1863, 1070–1078. doi: 10.1016/j.bbagen.2019.03.020
Scheller, H. V., and Ulvskov, P. (2010). Hemicelluloses. Annu. Rev. Plant. Biol. 61, 263–289. doi: 10.1146/annurev-arplant-042809-112315
Schultz, C., Gilson, P., Oxley, D., Youl, J., and Bacic, A. (1998). GPI-anchors on arabinogalactan-proteins: implications for signalling in plants. Trends Plant Sci. 3, 426–431. doi: 10.1016/S1360-1385(98)01328-4
Schultz, C. J., Johnson, K. L., Currie, G., and Bacic, A. (2000). The classical arabinogalactan protein gene family of Arabidopsis. Plant Cell 12, 1751–1768. doi: 10.1105/tpc.12.9.1751
Schultz, C. J., Rumsewicz, M. P., Johnson, K. L., Jones, B. J., Gaspar, Y. M., and Bacic, A. (2002). Using genomic resources to guide research directions. The arabinogalactan protein gene family as a test case. Plant Physiol. 129, 1448–1463. doi: 10.1104/pp.003459
Seifert, G. J., and Roberts, K. (2007). The biology of arabinogalactan proteins. Annu. Rev. Plant Biol. 58, 137–161. doi: 10.1146/annurev.arplant.58.032806.103801
Sella, L., Castiglioni, C., Paccanaro, M. C., Janni, M., Schafer, W., D’Ovidio, R., et al. (2016). Involvement of fungal pectin methylesterase activity in the interaction between Fusarium graminearum and wheat. Mol. Plant. Microbe Interact. 29, 258–267. doi: 10.1094/MPMI-07-15-0174-R
Shi, H., Kim, Y., Guo, Y., Stevenson, B., and Zhu, J. K. (2003). The Arabidopsis SOS5 locus encodes a putative cell surface adhesion protein and is required for normal cell expansion. Plant Cell 15, 19–32. doi: 10.1105/tpc.007872
Showalter, A. M. (2001). Arabinogalactan-proteins: structure, expression and function. Cell Mol. Life Sci. 58, 1399–1417. doi: 10.1007/PL00000784
Showalter, A. M., and Basu, D. (2016a). Extensin and arabinogalactan-protein biosynthesis: glycosyltransferases, research challenges, and biosensors. Front. Plant Sci. 7:814. doi: 10.3389/fpls.2016.00814
Showalter, A. M., and Basu, D. (2016b). Glycosylation of arabinogalactan-proteins essential for development in Arabidopsis. Commun. Integr. Biol. 9:e1177687. doi: 10.1080/19420889.2016.1177687
Showalter, A. M., Keppler, B., Lichtenberg, J., Gu, D., and Welch, L. R. (2010). A bioinformatics approach to the identification, classification, and analysis of hydroxyproline-rich glycoproteins. Plant Physiol. 153, 485–513. doi: 10.1104/pp.110.156554
Takata, R., Tokita, K., Mori, S., Shimoda, R., Harada, N., Ichinose, H., et al. (2010). Degradation of carbohydrate moieties of arabinogalactan-proteins by glycoside hydrolases from Neurospora crassa. Carbohydr. Res. 345, 2516–2522. doi: 10.1016/j.carres.2010.09.006
Tan, L., Eberhard, S., Pattathil, S., Warder, C., Glushka, J., Yuan, C., et al. (2013). An Arabidopsis cell wall proteoglycan consists of pectin and arabinoxylan covalently linked to an arabinogalactan protein. Plant Cell 25, 270–287. doi: 10.1105/tpc.112.107334
Tan, L., Showalter, A. M., Egelund, J., Hernandez-Sanchez, A., Doblin, M. S., and Bacic, A. (2012). Arabinogalactan-proteins and the research challenges for these enigmatic plant cell surface proteoglycans. Front. Plant Sci. 3:140. doi: 10.3389/fpls.2012.00140
Tan, L., Varnai, P., Lamport, D. T., Yuan, C., Xu, J., Qiu, F., et al. (2010). Plant O-hydroxyproline arabinogalactans are composed of repeating trigalactosyl subunits with short bifurcated side chains. J. Biol. Chem. 285, 24575–24583. doi: 10.1074/jbc.M109.100149
Tang, W., Ezcurra, I., Muschietti, J., and McCormick, S. (2002). A cysteine-rich extracellular protein. LAT52, interacts with the extracellular domain of the pollen receptor kinase LePRK2. Plant Cell 14, 2277–2287. doi: 10.1105/tpc.003103
Taylor, E. J., Smith, N. L., Turkenburg, J. P., D’Souza, S., Gilbert, H. J., and Davies, G. J. (2006). Structural insight into the ligand specificity of a thermostable family 51 arabinofuranosidase. Araf51, from Clostridium thermocellum. Biochem. J. 395, 31–37. doi: 10.1042/BJ20051780
Tsumuraya, Y., Mochizuki, N., Hashimoto, Y., and Kovac, P. (1990). Purification of an exo-beta-(1—-3)-D-galactanase of Irpex lacteus (Polyporus tulipiferae) and its action on arabinogalactan-proteins. J. Biol. Chem. 265, 7207–7215.
van Hengel, A. J., and Roberts, K. (2003). AtAGP30, an arabinogalactan-protein in the cell walls of the primary root, plays a role in root regeneration and seed germination. Plant J. 36, 256–270. doi: 10.1046/j.1365-313x.2003.01874.x
vd Veen, P., Flipphi, M. J., Voragen, A. G., and Visser, J. (1991). Induction, purification and characterisation of arabinases produced by Aspergillus niger. Arch. Microbiol. 157, 23–28. doi: 10.1007/BF00245330
Vicre, M., Santaella, C., Blanchet, S., Gateau, A., and Driouich, A. (2005). Root border-like cells of Arabidopsis. Microscopical characterization and role in the interaction with rhizobacteria. Plant Physiol. 138, 998–1008. doi: 10.1104/pp.104.051813
Villa-Rivera, M. G., Conejo-Saucedo, U., Lara-Marquez, A., Cano-Camacho, H., Lopez-Romero, E., and Zavala-Paramo, M. G. (2017a). The role of virulence factors in the pathogenicity of Colletotrichum sp. Curr. Protein. Pept. Sci. 18, 1005–1018. doi: 10.2174/1389203717666160813160727
Villa-Rivera, M. G., Zavala-Páramo, M. G., Conejo-Saucedo, U., López-Romero, E., Lara-Márquez, A., and Cano-Camacho, H. (2017b). Differences in the expression profile of endo-β-(1,6)-D-galactanase in pathogenic and non-pathogenic races of Colletotrichum lindemuthianum grown in the presence of arabinogalactan, xylan or Phaseolus vulgaris cell walls. Physiol. Mol. Plant Pathol. 99, 75–86. doi: 10.1016/j.pmpp.2016.10.002
Wang, L., Cheng, M., Yang, Q., Li, J., Wang, X., Zhou, Q., et al. (2019). Arabinogalactan protein-rare earth element complexes activate plant endocytosis. Proc. Natl. Acad. Sci. USA 116, 14349–14357. doi: 10.1073/pnas.1902532116
Wilkens, C., Andersen, S., Petersen, B. O., Li, A., Busse-Wicher, M., Birch, J., et al. (2016). An efficient arabinoxylan-debranching alpha-L-arabinofuranosidase of family GH62 from Aspergillus nidulans contains a secondary carbohydrate binding site. Appl. Microbiol. Biotechnol. 100, 6265–6277. doi: 10.1007/s00253-016-7417-8
Wood, T. M., and McCrae, S. I. (1996). Arabinoxylan-degrading enzyme system of the fungus Aspergillus awamori: purification and properties of an alpha-L-arabinofuranosidase. Appl. Microbiol. Biotechnol. 45, 538–545. doi: 10.1007/BF00578468
Wu, J., Wang, Y., Park, S. Y., Kim, S. G., Yoo, J. S., Park, S., et al. (2016). Secreted alpha-N-arabinofuranosidase B protein is required for the full virulence of Magnaporthe oryzae and triggers host defences. PLoS One 11:e0165149. doi: 10.1371/journal.pone.0165149
Wu, Y., Fan, W., Li, X., Chen, H., Takac, T., Samajova, O., et al. (2017). Expression and distribution of extensins and AGPs in susceptible and resistant banana cultivars in response to wounding and Fusarium oxysporum. Sci. Rep. 7:42400. doi: 10.1038/srep42400
Xie, D., Ma, L., Samaj, J., and Xu, C. (2011). Immunohistochemical analysis of cell wall hydroxyproline-rich glycoproteins in the roots of resistant and susceptible wax gourd cultivars in response to Fusarium oxysporum f. sp. Benincasae infection and fusaric acid treatment. Plant Cell Rep. 30, 1555–1569. doi: 10.1007/s00299-011-1069-z
Xie, F., Williams, A., Edwards, A., and Downie, J. A. (2012). A plant arabinogalactan-like glycoprotein promotes a novel type of polar surface attachment by Rhizobium leguminosarum. Mol. Plant Microbe. Interact. 25, 250–258. doi: 10.1094/MPMI-08-11-0211
Yang, J., and Showalter, A. M. (2007). Expression and localization of AtAGP18, a lysine-rich arabinogalactan-protein in Arabidopsis. Planta 226, 169–179. doi: 10.1007/s00425-007-0478-2
Yariv, J., Lis, H., and Katchalski, E. (1967). Precipitation of arabic acid and some seed polysaccharides by glycosylphenylazo dyes. Biochem. J. 105, 1C–2C. doi: 10.1042/bj1050001c
Yoshimi, Y., Hara, K., Yoshimura, M., Tanaka, N., Higaki, T., Tsumuraya, Y., et al. (2020). Expression of a fungal exo-beta-1,3-galactanase in Arabidopsis reveals a role of type II arabinogalactans in the regulation of cell shape. J. Exp. Bot. 71, 5414–5424. doi: 10.1093/jxb/eraa236
Yoshimi, Y., Yaguchi, K., Kaneko, S., Tsumuraya, Y., and Kotake, T. (2017). Properties of two fungal endo-beta-1,3-galactanases and their synergistic action with an exo-beta-1,3-galactanase in degrading arabinogalactan-proteins. Carbohydr. Res. 45, 26–35. doi: 10.1016/j.carres.2017.10.013
Zhang, Y., Brown, G., Whetten, R., Loopstra, C. A., Neale, D., Kieliszewski, M. J., et al. (2003). An arabinogalactan protein associated with secondary cell wall formation in differentiating xylem of loblolly pine. Plant Mol. Biol. 52, 91–102. doi: 10.1023/A:1023978210001
Keywords: arabinogalactan proteins, plant cell wall, hydrolytic enzymes, plant-microbe interaction, infection
Citation: Villa-Rivera MG, Cano-Camacho H, López-Romero E and Zavala-Páramo MG (2021) The Role of Arabinogalactan Type II Degradation in Plant-Microbe Interactions. Front. Microbiol. 12:730543. doi: 10.3389/fmicb.2021.730543
Received: 25 June 2021; Accepted: 04 August 2021;
Published: 25 August 2021.
Edited by:
Ivan Martinez Duncker, Universidad Autónoma del Estado de Morelos, MexicoReviewed by:
Agata Leszczuk, Institute of Agrophysics (PAN), PolandCopyright © 2021 Villa-Rivera, Cano-Camacho, López-Romero and Zavala-Páramo. This is an open-access article distributed under the terms of the Creative Commons Attribution License (CC BY). The use, distribution or reproduction in other forums is permitted, provided the original author(s) and the copyright owner(s) are credited and that the original publication in this journal is cited, in accordance with accepted academic practice. No use, distribution or reproduction is permitted which does not comply with these terms.
*Correspondence: María Guadalupe Zavala-Páramo, Z3phdnBhckBob3RtYWlsLmNvbQ==; bWFyaWEuemF2YWxhLnBhcmFtb0B1bWljaC5teA==
Disclaimer: All claims expressed in this article are solely those of the authors and do not necessarily represent those of their affiliated organizations, or those of the publisher, the editors and the reviewers. Any product that may be evaluated in this article or claim that may be made by its manufacturer is not guaranteed or endorsed by the publisher.
Research integrity at Frontiers
Learn more about the work of our research integrity team to safeguard the quality of each article we publish.