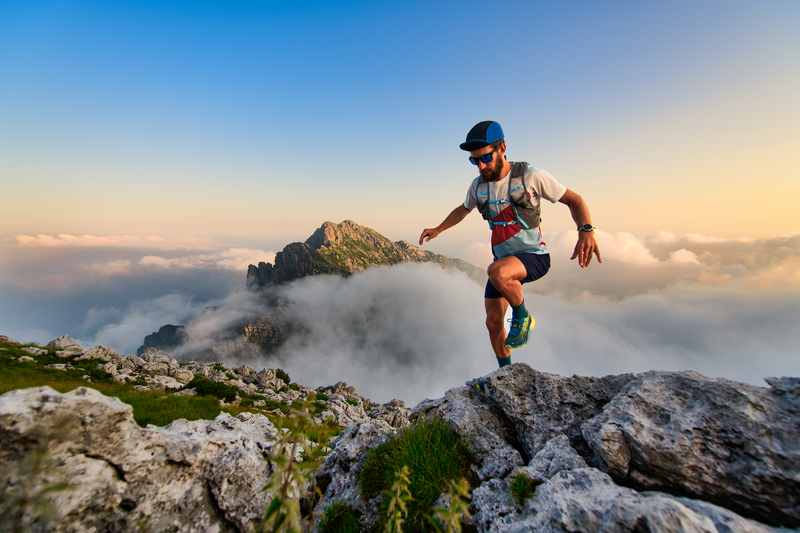
95% of researchers rate our articles as excellent or good
Learn more about the work of our research integrity team to safeguard the quality of each article we publish.
Find out more
ORIGINAL RESEARCH article
Front. Microbiol. , 20 September 2021
Sec. Infectious Agents and Disease
Volume 12 - 2021 | https://doi.org/10.3389/fmicb.2021.726958
This article is part of the Research Topic The Role of Glycans in Infectious Disease View all 15 articles
Sporothrix schenckii, Sporothrix brasiliensis, and Sporothrix globosa are the main causative agents of sporotrichosis, a human subcutaneous mycosis. Differences in virulence patterns are associated with each species but remain largely uncharacterized. The S. schenckii and S. brasiliensis cell wall composition and virulence are influenced by the culturing media, with little or no influence on S. globosa. By keeping constant the culturing media, we compared the cell wall composition of three S. schenckii and two S. brasiliensis strains, previously described as presenting different virulence levels on a murine model of infection. The cell wall composition of the five Sporothrix spp. strains correlated with the biochemical composition of the cell wall previously reported for the species. However, the rhamnose-to-β-glucan ratio exhibits differences among strains, with an increase in cell wall rhamnose-to-β-glucan ratio as their virulence increased. This relationship can be expressed mathematically, which could be an important tool for the determination of virulence in Sporothrix spp. Also, structural differences in rhamnomannan were found, with longer side chains present in strains with lower virulence reported for both species here studied, adding insight to the importance of this polysaccharide in the pathogenic process of these fungi.
Sporotrichosis, a cutaneous and subcutaneous mycosis of humans and other mammals, is caused by species described within the pathogenic clade of the Sporothrix genus, of which S. brasiliensis, S. schenckii, and S. globosa are the three species of major clinical importance (de Beer et al., 2016). All species of the Sporothrix genus are thermo-dimorphic fungi, presenting a saprophytic sporulating mycelial phase at 25–28°C and a yeast-like pathogenic phase at 36–37°C. In humans, the disease is characterized by cutaneous and subcutaneous lesions with regional lymphocutaneous dissemination, although some pulmonary and systemic infections have been reported (Callens et al., 2006). It is a neglected infectious disease with a worldwide distribution, and a higher incidence in tropical and subtropical countries (Barros et al., 2011; Chakrabarti et al., 2014). The cutaneous disease begins with a traumatic inoculation of the fungus by contaminated soil or plant debris or through bites and scratches from infected cats (Barros et al., 2011; Chakrabarti et al., 2014). Multiple infections might arise from a single source, which can lead to outbreaks (Chakrabarti et al., 2014).
Sporothrix schenckii is the most widespread species of the pathogenic clade present in the Americas, Europe, Africa, and Asia and is mainly associated with a sapronosis (Zhang et al., 2015), similarly to S. globosa, which is predominant in Asia. Furthermore, S. brasiliensis is an emerging species related to cat-transmitted sporotrichosis, mainly described in Brazil but now, also, present in other South American countries (Chakrabarti et al., 2014; Etchecopaz et al., 2020; Rossow et al., 2020).
Differences in the virulence profiles in experimental models of infection have been reported within the pathogenic clade. Sporothrix brasiliensis is reported as the most virulent species, followed by S. schenckii, and S. globosa, with the latter been reported as the species with the lowest virulence of the three (Arrillaga-Moncrieff et al., 2009; Almeida-Paes et al., 2015; Clavijo-Giraldo et al., 2016; Lozoya-Pérez et al., 2020). However, differences within S. schenckii clinical isolates have also been reported, ranging from highly virulent to non-virulent isolates (Fernandes et al., 2013; Almeida-Paes et al., 2015). Some factors, such as melanization, thermotolerance, protein secretion, and immunogenicity have been related to the differences in virulence patterns between the Sporothrix spp. and within clinical isolates (Fernandes et al., 2013; Almeida-Paes et al., 2015).
The fungal cell wall protects the fungus, acting as an initial barrier against hostile environments while preserving the cell’s integrity against internal turgor pressure. It is a dynamic structure, presenting continuous changes in composition and structural organization as the cell grows or undergoes morphological changes (Latgé, 2007). These changes are strongly regulated during the cell cycle, or in response to environmental conditions, stress, and mutations in the cell wall biosynthetic processes (Klis et al., 2006; Ruiz-Herrera et al., 2006).
In general, fungal cell walls are bilayered structures, with the innermost layer comprising a core of covalently attached and branched β-(1,3) glucan, forming intrachain hydrogen bonds with chitin assembled into fibrous microfibrils, and all together forming a scaffold around the cell (Gow et al., 2017). The β-(1,3) glucan is a highly immunogenic molecule and is one of the main fungal pathogen-associated molecular patterns (PAMP) that bind to a very specific pathogen recognition receptor (PRR) present on the surface of the host’s immune cells, the C-type lectin dectin-1 (Hernández-Chávez et al., 2017). Chitin, is an important immunoreactive polysaccharide, that interacts with different PRRs in a size-dependent mechanism, where big (70–100μm) or very small (<2μm) chitin particles do not trigger immune reactions, while medium-sized chitin particles (40–70μm) induce a proinflammatory response, whereas small-sized chitin particles (2–10μm) trigger an anti-inflammatory response (Hernández-Chávez et al., 2017). In general, these two polysaccharides are often masked by the components of the cell wall outer layer, which differs from the inner scaffold layer (Erwig and Gow, 2016). The S. schenckii and S. brasiliensis cell wall is mainly composed of structural polysaccharides, β-glucans, and chitin and has a peptide-rhamnomannan (PRM) outermost layer (Lopes-Bezerra et al., 2018). More recently, it has been reported that the culture media have an influence on changes in the cell wall composition and structure, as well as on the virulence of S. schenckii and S. brasiliensis but not on S. globosa (Lozoya-Pérez et al., 2020).
Within the frame of all the previous bodies of evidence, in the present work, we examine and compare the S. schenckii and S. brasiliensis cell wall composition in different strains. The isolates studied here, showed distinct virulence profiles (Nascimento et al., 2008; Castro et al., 2013), and the analysis of possible differences in the composition and/or the relative content of cell wall components may add new important aspects that correlate with their difference in virulence profiles.
Fungal strains used in this study are listed in Table 1. The yeast morphology was obtained by growing cells on Brain Heart Infusion (BHI, Oxoid, Hampshire, United Kingdom) liquid medium, with continuous shaking at 100rpm for 4days at 37°C. Cells were inspected under a phase-contrast microscope (Nikon Optiphot, Japan) before being used to check for contamination or partial differentiation.
Yeast cells from cultures in exponential phase were collected by centrifugation at 8,000 x g for 1h at 10°C. Briefly, the fungal pellets were suspended in distilled water with an equal volume of glass beads (0.45–0.50mm diameter) and shaken five times in a Braun homogenizer (Braun, Melsungen, Germany) for 1min, followed by 1min cooling on ice between shakings. Cell disruption was followed by light microscopy. Cell homogenates were washed out of glass beads with distilled water and centrifuged at 480×g for 5min at 4°C. The pellet was freeze-dried, weighted, and fractionated by alkaline separation (Previato et al., 1979; San-Blas and San-Blas, 1994; Lopes-Bezerra et al., 2018). Briefly, the freeze-dried material was re-suspended in 1M NaOH for 16h, and the suspension was centrifuged to separate the alkali-insoluble material from the supernatant (fraction 1). The supernatant was neutralized with 1N HCl, centrifuged and the pellet (alkali-soluble and acid-insoluble, fraction 2) separated from the supernatant (alkali and acid-soluble, fraction 3), which was further analyzed as described previously (Lopes-Bezerra et al., 2018). Rhamnomannan was obtained by treating fraction 3 with Fehling’s reagent at 4°C as reported previously (Previato et al., 1979). The insoluble copper complexes generated, were centrifuged, washed three times with 3% KOH, twice with neat ethanol, and collected. The resulting residue was suspended in distilled water and cations removed with Dowex 50W-X4 (H+ form; Sigma-Aldrich, St. Louis, MO, United States) for 1h at room temperature; the supernatant was precipitated by the addition of four volumes of neat ethanol. The residue was collected by centrifugation at 8,000×g for 10min (fraction 4, rhamnomannan). The mother liquor of the copper complexes was neutralized with acetic acid and centrifuged. The supernatant was dialyzed for 72h against distilled water and deionized with a mixture of Dowex 1 (HCO3− form; Sigma-Aldrich, St. Louis, MO, United States) and Dowex 50W-X4 (H+ form), the filtrate was concentrated, and the polysaccharides present were precipitated by the addition of three volumes of neat ethanol (fraction 5). All fractions obtained were freeze-dried.
Sugar and total amino acid content of cell wall fractions were determined as follows: for hexose content, 10mg of each cell wall fraction was resuspended in 1ml of 1M HCl, sealed in a 2ml Wheaton 176,776 ampoule, and heated for 3h at 100 ̊C. Hydrolyzed samples were diluted 1/10 or 1/100. Sugar quantification was accomplished by the Anthrone method for hexose content quantification in concentrated H2SO4. To determine amino acid and amino sugar contents, 10mg of each sample was resuspended in 1ml 6M HCl, sealed in a 2ml Wheaton 176,776 ampoule, and heated for 16h at 100 ̊C. Amino acid and amino sugar content were determined employing alanine and glucosamine solutions as standards, as described previously (Rondle and Morgan, 1955; Yemm et al., 1955). For rhamnose quantification, 10mg of fraction 3 was resuspended in 1ml of 1M HCl, sealed in a 2ml Wheaton 176,776 ampoule, and heated for 3h at 100 ̊C. Hydrolyzed samples were diluted 1/10 or 1/100. Quantification of methyl pentoses was conducted (Dische and Shettles, 1948) using 85.7% H2SO4 and 3% cysteine in the reaction mixtures and rhamnose to construct a standard curve.
Samples were prepared as KBr pellets. IR spectra were recorded from 3,500 to 500cm−1, using a Nicolet iS10 IR spectrometer (Thermo Fisher Scientific, Waltham, MA, United States), coupled to the OMNIC 8.0 software, following the indications of the Infrared Spectroscopy Service, Center of Chemistry, IVIC, Caracas, Venezuela.
To obtain the structural data, 13C and 1H NMR were employed, briefly, samples of the polysaccharide fraction to be analyzed and standards (ca. 20mg) were solubilized in D2O or 2% NaOD and the spectra obtained at 75MHz with a recollecting time of 16h and 70°C using a Bruker 300 Ultrashield spectrometer, according to the indication of the Nuclear Magnetic Resonance Service, Center of Chemistry, IVIC, Caracas, Venezuela.
For chitin exposure analysis, cells were stained with 1mg/ml wheat germ agglutinin-fluorescein isothiocyanate (Sigma-Aldrich, St. Louis, MO, United States), for 60min at room temperature. Flow cytometry was performed in a MoFlo XDP apparatus (Beckman Coulter), collecting 50,000 singlet events. Fluorescence of positive events was recovered from the compensated FL3 (green) channel using unlabeled yeast cells. Total population densities were gated and analyzed using FlowJo (version 10.0.7) software.
The heat-killed (HK) cells were prepared by incubating at 60°C for 2h. The cellular death was confirmed by incubating aliquots of the preparations in YPD plates at 37°C for 5days.
Quantifications of cell wall components were made by triplicate. Statistical analyses were done by the Tukey Honestly Significant Difference (HSD) post hoc test. Differences were considered statistically significant at p<0.05.
Structural and chemical analyses of polysaccharides from yeast walls of S. schenckii strains MYA 4820, MYA 4821, MYA 4822, and S. brasiliensis strains MYA 4823 and MYA 4824 were analyzed (Table 1). Cell walls from BHI-grown cells were purified and fractioned by the acid and alkali solubility and insolubility methods, as previously reported for Sporothrix cell wall analyses (Previato et al., 1979; Lopes-Bezerra et al., 2018). For polysaccharide structural characterization, IR spectroscopies, as well as proton and 13C nuclear magnetic resonance (1H-NMR and 13C-NMR respectively) were used, and the generated spectra compared with IR, 1H-NMR, and 13C-NMR spectra previously reported for S. schenckii (Travassos et al., 1973; Gorin et al., 1977; Gow et al., 1987; Lopes-Alves et al., 1992; Lopes-Bezerra et al., 2018). For the five strains analyzed, IR spectra of the alkali-insoluble cell wall fraction showed characteristic polysaccharide absorption signals (Figure 1), showing a strong and wideband around 3,400cm1 and additional bands around 2,921, 1,641, and 1,412cm−1 (Rodríguez-Brito et al., 2010). Absorption bands around 1,557 and 1,662cm−1 evidenced the presence of chitin, while β-glucan is evidenced by absorption bands at around 897 and 1,378cm−1 (Rodríguez-Brito et al., 2010). Also, the presence of absorption peaks belonging to β-(1,3)-(1,6)-glucan (1,160, 1,078, and 1,044cm−1; Synytsya and Novak, 2014), is present in all the IR spectra obtained from all the strains.
Figure 1. Sporothrix schenckii strains IR spectra of alkali-insoluble polysaccharides. Here, the signals corresponding to all strains are shown. Chitin and β-glucan signals are indicated with blue and purple arrows respectively, also are present signals of β-glucans (1,3 and 1,6 evidenced by peaks at 1156, 1076, and 1,041cm−1).
The rhamnomannan characterization was followed by 1H-NMR and 13C-NMR. For all the cases, 1H-NMR spectra showed the presence of the methyl group that belongs to rhamnose (1.18–1.17ppm) and the signals corresponding to the proton linked to carbon 5 (3.32–4.10ppm) next to the methylene group from carbon six in the mannose ring (Figure 2B). The H1 region of the 1H-NMR spectra for all the strains under study is shown in Figure 3, presenting proton signals 5.21–5.26, 5.08–5.13, and 4.84–4.88ppm, which are characteristic of Sporothrix rhamnomannan, as previously reported (Travassos et al., 1973, 1974). Signals 5.08–5.12 and 5.21–5.25ppm are related to the presence of Rha(α1-4)GlcA(α1,2)Man(α1,2)Man-ol, as previously reported (Lopes-Alves et al., 1992). Also, the presence of a proton signal 4.97ppm previously reported as present in S. brasiliensis strain MYA 4823 (Lopes-Bezerra et al., 2018) is notoriously absent from the rhamnomannan of all the other strains (Figure 3D). The pattern of the 13C-NMR spectrum (Figure 2A) allowed us to determine how the rhamnose and mannan are linked in the rhamnomannan polymer. The rhamnomannan backbone is composed of mannose linked by α-1,6-glycosidic bonds and single units of rhamnose as side chains, which has been reported as characteristic of rhamnomannans isolated at 37°C from the S. schenckii yeast phase, first described as rhamnomannan type I (Figure 2A; Table 2; Travassos et al., 1973, 1974; Gorin et al., 1977). It is worth mentioning that the 13C-NMR spectra for the cell wall of S. schenckii, strain MYA4822, and S. brasiliensis MYA4824, showed unique signals at 98.15, 101.4, and 102.4ppm, associated with the C-1 of α-L-Rhap nonreducing end unit of α-L-Rhap-(l,2)-α-Rhap and 2,4-di-0-substituted α-D-mannopyranose units, which suggest longer side chains in the cell wall rhamnomannan for these two strains when compared to the other strains under study (Figure 4).
Figure 2. Structural analysis of the rhamnomannan present in the Sporothrix strains employing 13C-NMR and 1H-NMR (A,B, respectively). On the image, the spectrum corresponding to the rhamnomannan fraction of S. schenckii strain MYA 4820 is presented as a representative spectrum of both, S. schenckii and S. brasiliensis strains under study. (A) The signals corresponding to the carbon atoms in the mannose and rhamnose residues are shown as arrows, red for mannose and black for rhamnose. The corresponding signals are shown in Table 1. (B) Show the presence of the methyl group belonging to rhamnose (1.18 and 1.17p.p.m.) and the signals correspond to the proton bound to carbon 5 next to the methylene group for carbon 6 in the mannose ring (3.32, 3.35, and 3.39p.p.m.).
Figure 3. 1H-NMR of the rhamnomannan fraction isolated from S. schenckii and S. brasiliensis yeast cells. The H1 region of the rhamnomannans of S. schenckii (A–C) and S. brasiliensis (D,E) is enlarged. The red arrow shows a unique signal for S. brasiliensis strain 4823 as was described recently (Lopes-Bezerra et al., 2018).
Figure 4. 13C-NMR of the rhamnomannan fraction isolated from the strains presenting less virulence in yeast phase. Sporothrix schenckii 4822 (A) and S. brasiliensis 4824 (B) show the signals associated to type I rhamnomannan (red and black arrows), but also a signal corresponding to C-1 of α-L-Rhap nonreducing end unit of α-L-Rhap-(l, 2)-α—Rhap and 2,4-di-0-substituted α-D-mannopyranose units (Cian arrows; Travassos, 1985), which suggest longer side chains in the cell wall for these two strains.
For polysaccharide quantification, the fractions obtained by alkali and acid fractionation were further analyzed by colorimetric techniques as described in the methods section. Table 3 shows the relative cell wall polysaccharides content for S. schenckii and S. brasiliensis strains. The polysaccharide analysis for the cell walls of all strains in the yeast phase, showed a higher chitin content (around 27%) for the two S. brasiliensis strains, when compared to the S. schenckii strains (Table 3; Figure 5), as previously reported (Lopes-Bezerra et al., 2018). A difference was evident for the cell wall β-glucan relative content (around 28% more β-glucan) of the lower virulent S. schenckii MYA 4822 and S. brasiliensis MYA 4824 strains when compared to the higher virulent strains (Table 3; Figure 5). Rhamnomannan relative contents were higher for both S. brasiliensis strains analyzed, when compared to the S. schenckii strains (up to 38% more rhamnomannan). Also, a higher rhamnomannan relative content could be observed in the more virulent S. schenckii MYA 4820 strain, when compared with the non-virulent S. schenckii MYA 4822 strain (over 30% higher; Table 3; Figure 5). The relationship between the level of virulence reported and the β-glucan/rhamnomannan cell wall ratio can be represented mathematically by an ascendant curve, with an R2=1 for the polynomial function y=0.0388x4–0.3608x3+1.1913x2–1.1792x+1.6 (Figure 6), which shows an inverse relationship between the reported virulence and a higher ratio of cell wall β-glucans/rhamnomannan content.
Table 3. Cell wall polysaccharide content comparison of the Y phase of the S. schenckii and S. brasiliensis strains under study.
Figure 5. Comparison of polysaccharides represented as percentage in the cell wall of S. brasiliensis (A) and S. schenckii (B) strains, yeast phase. Percentage of polysaccharides are represented in colored bars: β glucan (red), rhamnomannan (blue) and chitin (black). *Tukey Honestly Significant Difference (HSD) post hoc test was used for intra and inter species comparative analyses. Value of p<0.05. Quantification of cell wall components was made by triplicate.
Figure 6. Relation between β glucan vs. rhamnomannan ratio with strain virulence. Black columns represent the ratio of β glucan to rhamnomannan present in the cell wall. Strains are arranged from higher to the lower virulence reported. The red curve represents the polynomial curve of relationship, mathematically represented by an ascendant curve with an R2=1 with the polynomial function y=0.0388x4–0.3608x3+1.1913x2–1.1792x+1.6. Bg, β-glucan; Rh-Mannan, rhamnomannan.
Rhamnose residues from PRM are known to be the main antigenic epitopes found on the S. schenckii cell surface (Fernandes et al., 1999). Here, the rhamnose content in S. brasiliensis strains was 40% higher compared to S. schenckii strains (Table 3). When comparing only the S. schenckii strains, the cell wall rhamnose content shows differences from high-to-low virulence for strains MYA 4820, MYA 4821, and MYA 4822 (Table 3; Figure 5). This observation fits the exponential curve: y=14.722e−0.049x, with an R2=1, that can be mathematically expressed as the linear equation: ρ=−0.049 (β)+2.7, where β represents the cell wall β-glucan content expressed as percentage and ρ represent the Ln(Rha), where Rha is the rhamnose cell wall content represented as a percentage (Figure 7). No significant differences were observed for the cell wall β-glucans among strains, except for S. schenckii MYA 4821, which had 20% more β-glucans than the rest of the analyzed strains.
Figure 7. A mathematical model for the Rhamnose/β-glucan composition as expression of virulence. With an increase in reported virulence, the rhamnose proportion rise and β-glucan decreases. This observation fit to an exponential curve with an R2=1, that could be expressed as a linear equation: ρ=−0.049(β)+2.7, where β represents the β-glucan composition and ρ=Ln(Rha), where Rha is the rhamnose cell wall percentage. This model might be useful to predict the virulence level employing the β-glucan and rhamnose percentage ratio. This mathematical expression infers the highest rhamnose percentage to 15% (β=0) and for the lowest rhamnose percentage (1%) β=55.1%.
Chitin exposure on the cell wall for the five strains under study was determined in BHI-grown yeast cells. The highest chitin exposure on the cell wall was found for S. schenckii strain MYA 4822 (Figure 8), followed by S. schenckii strain MYA 4820. The lowest cell wall chitin exposure was observed for S. schenckii MYA 4821, and the two S. brasiliensis strains MYA 4823 and MYA 4824, all of them reported as presenting higher virulence patterns (Nascimento et al., 2008; Castro et al., 2013).
Figure 8. Comparison of the chitin exposure on the yeast cells of S. schenckii and S. brasiliensis grown in Brain Heart Infusion (BHI) broth. The smooth bars represent the exposure of chitin under normal and live cell (Alive). The bars with frames represent the percentage of exposed chitin after the cells were heat inactivated (HK).
The cell wall is the first point of contact with the host upon infection and colonization; understanding its composition allow unveiling specific mechanisms triggered by PAMPs and their corresponding PRRs (Gow et al., 2017). Recently, it was reported that carbon or nitrogen limitation during growth of yeast cells of S. brasiliensis and S. schenckii resulted in a reduced virulence, and the mechanism is related to affect the cell wall composition, where an increase in cell wall β-glucan, and a reduction of rhamnose and mannose was observed (Lozoya-Pérez et al., 2020). Also, the virulence-reduced strains showed a higher exposure of β-glucan, leading to an increase in the uptake of the fungus by hemocytes of Galleria mellonella (Lozoya-Pérez et al., 2020).
In the present work, we compared the cell relative composition of the polysaccharides of the pathogenic yeast morphotype of five Sporothrix strains, of which three were S. schenckii and two S. brasiliensis strains, with differences in virulence levels reported in a murine model (Table 1; Nascimento et al., 2008; Castro et al., 2013). To normalize the comparison, all the strains were grown under identical conditions in BHI broth, a widely used culture medium for Sporothrix spp. (Kong et al., 2006; Brito et al., 2007; Teixeira et al., 2009; Della Terra et al., 2017; De Almeida et al., 2018).
As previously reported, the main polysaccharides present in the cell wall of both S. schenckii and S. brasiliensis strains were: β-glucan, as major cell wall polysaccharide, followed by rhamnomannan and chitin (Table 3; Figure 5; Lopes-Bezerra et al., 2018; Lozoya-Pérez et al., 2020). A higher cell wall chitin content was observed in the cell wall of S. brasiliensis strains compared to S. schenckii strains, which also have been previously reported (Lopes-Bezerra et al., 2018). However, when comparing the cell wall polysaccharide composition of the five Sporothrix spp. strains, a pattern appeared to emerge, with higher β-glucans and lower rhamnomannan levels in cell wall contents present in the previously reported non-virulent or low virulent strains (S. schenckii MYA 4822 and MYA 4821 and S. brasiliensis MYA 4824). In contrast, lower β-glucan and higher rhamnomannan levels in cell wall content were shown in those strains for which higher virulence have been reported, regardless of the species (S. brasiliensis MYA 4823 and S. schenckii MYA 4820; Table 3; Figure 5). Therefore, the β-glucan/rhamnomannan cell wall ratio can be mathematically represented by a polynomial function showing an inverse relationship to the virulence increase (Figure 6). Then, we focused on S. schenckii, for which we had strains with three different levels of virulence reported (Table 1) and noticed that cell wall rhamnose content increased, while the cell wall β-glucan content decreased when compared from the less to the highest reported virulence phenotype (Table 3; Figure 5). This observation can be mathematically expressed as a linear equation (Figure 7), which extrapolates the highest virulence for S. schenckii strains when the rhamnose percentage in the cell wall reaches 15% and the β-glucans cell wall content is 0%, and the lowest virulence when the rhamnose percentage is 1% and the β-glucan content is 55.1% (intersection points on the y and x axis of the linear curve, respectively, Figure 7).
Recently, a bilayered cell wall model based on experimental data was proposed for S. schenckii and S. brasiliensis yeast cells (Lopes-Bezerra et al., 2018), which positioned the structural and more immunogenic chitin and β-glucans at the inner-most layer, and the PRM as an outermost layer covering the former.
The structural cell wall glycoconjugates, β-1-3 and β-1-6-glucans, as well as chitin, are found in pathogenic fungal species as involved in the innate immune response as PAMPs, so the exposure of β-glucans and chitin on the fungal surface favors their binding to their corresponding PRRs presented on the host cells surface, allowing the uptake of the microorganism and/or triggering the secretion of specific cytokines (Hernández-Chávez et al., 2017). A Sporothrix spp. strain with a higher β-glucans/rhamnomannan ratio might favor the exposition of the immunogenic β-glucans to the host immune system, triggering its response before the infection can be established, therefore presenting a lower level of virulence. Indeed, Lozoya-Pérez et al. (2020) recently reported that a higher β-glucan exposure is in close relation with a lower virulence phenotype in Sporothrix spp. To determine whether chitin also might be playing a role in the differences in virulence levels, chitin exposition was measured in the Y pathogenic phase for the five Sporothrix strains. Only the non-virulent S. schenckii MYA 4822 presented a high chitin exposition on its cell surface under the growth conditions used in the present study (Figure 8), which together with the high β-glucans/rhamnomannan ratio, builds up evidence for the involvement in the non-virulence phenotype reported, and by triggering the host immune system more efficiently.
A conserved general structure of the cell wall polysaccharides for all Sporothrix strains in their yeast phase was evidenced by the IR spectra analyzed. However, some differences were observed when the rhamnomannans from the cell walls of the five Sporothrix strains were characterized by 1H-NMR and 13C-NMR. Although a general pattern for both spectra was apparent for all the five strains studied (Figure 2), a closer inspection of the 13C-NMR spectra allowed us to identify unique signals for the cell wall rhamnomannan of the non-virulent and low virulent S. schenckii MYA 4822 and S. brasiliensis MYA 4824, respectively at 98.15, 101.4, and 102.4, associated with the C-1 of α-L-Rhap non-reducing end unit of α-L-Rhap-(l,2)-α-Rhap and 2,4-di-0-substituted α-D-mannopyranose units, suggesting longer side chains in the cell wall rhamnomannan for these two strains. Methylation analyses of the rhamnomannan present in the reportedly least virulent strains and comparison with the higher virulent strains would provide further insight into such differences. Also, the comparison of the 1H-NMR spectra for the rhamnomannan of all the strains studied, confirmed a previous report, showing the presence of a 4.97ppm signal only for the S. brasiliensis MYA 4823, which has been reported as a high virulent strain (Castro et al., 2013). The analysis of virulent and non-virulent strains of the Sporothrix genus, suggests that the rhamnomannans of the cell wall determines the exposure of chitin and β-glucans, which ultimately triggers a strong immune response that explains the resulting virulence phenotype. To overcome the limitations of the present work and to either strengthen or discard the mathematical model of virulence here proposed, a broader study including more strains, testing their virulence in a single mathematical model of virulence for sporotrichosis, and exploring the alterations in cell wall composition from strains cultured in different media and their possible impacts on virulence would be necessary, and will definitely either reinforce or discard this model to assess virulence, specifically for the Sporthrix genus.
The original contributions presented in the study are included in the article/supplementary material, further inquiries can be directed to the corresponding author.
HV-D, LL-B, and GN-V conceived and designed the experiments. HV-D, LB, ÁA-A, BF, and NL-P performed the experiments. HV-D, GN-V, LL-B, and HM-M analyzed the data. HV-D and GN-V wrote the paper. All authors contributed to the article and approved the submitted version.
HV-D was supported by Instituto Venezolano de Investigaciones Cientificas, Venezuela (Project 112). GN-V was supported by CONACYT-Mexico (Ref. CF-2019-170701). HM-M was supported by CONACYT-Mexico (Ref. FC 2015-02-834). Flow cytometry analysis was supported by CONACYT (grants 3013–205744 and 2019–300286).
The authors declare that the research was conducted in the absence of any commercial or financial relationships that could be construed as a potential conflict of interest.
All claims expressed in this article are solely those of the authors and do not necessarily represent those of their affiliated organizations, or those of the publisher, the editors and the reviewers. Any product that may be evaluated in this article, or claim that may be made by its manufacturer, is not guaranteed or endorsed by the publisher.
Almeida-Paes, R., De Oliveira, L. C., Oliveira, M. M. E., Gutierrez-Galhardo, M. C., Nosanchuk, J. D., and Zancopé-Oliveira, R. M. (2015). Phenotypic characteristics associated with virulence of clinical isolates from the sporothrix complex. Biomed. Res. Int. 2015:212308. doi: 10.1155/2015/212308
Arrillaga-Moncrieff, I., Capilla, J., Mayayo, E., Marimon, R., Mariné, M., Gené, J., et al. (2009). Different virulence levels of the species of Sporothrix in a murine model. Clin. Microbiol. Infect. 15, 651–655. doi: 10.1111/j.1469-0691.2009.02824.x
Barros, M. B., de Almeida Paes, R., and Oliveira Schubach, A. (2011). Sporothrix schenckii and Sporotrichosis. Clin. Microbiol. Rev. 24, 633–654. doi: 10.1128/CMR.00007-11
Brito, M. M. S., Conceição-Silva, F., Morgado, F. N., Raibolt, P. S., Schubach, A., Schubach, T. P., et al. (2007). Comparison of virulence of different Sporothrix schenckii clinical isolates using experimental murine model. Med. Mycol. 45, 721–729. doi: 10.1080/13693780701625131
Callens, S. F. J., Kitetele, F., Lukun, P., Lelo, P., Van Rie, A., Behets, F., et al. (2006). Pulmonary Sporothrix schenckii infection in a HIV positive child. J. Trop. Pediatr. 52, 144–146. doi: 10.1093/tropej/fmi101
Castro, R. A., Kubitschek-Barreira, P. H., Teixeira, P. A. C., Sanches, G. F., Teixeira, M. M., Quintella, L. P., et al. (2013). Differences in cell morphometry, cell wall topography and Gp70 expression correlate with the virulence of Sporothrix brasiliensis clinical isolates. PLoS One 8:e75656. doi: 10.1371/journal.pone.0075656
Chakrabarti, A., Bonifaz, A., Gutierrez-Galhardo, M. C., Mochizuki, T., and Li, S. (2014). Global epidemiology of sporotrichosis. Med. Mycol. 53, 3–14. doi: 10.1093/mmy/myu062
Clavijo-Giraldo, D. M., Matínez-Alvarez, J. A., Lopes-Bezerra, L. M., Ponce-Noyola, P., Franco, B., Almeida, R. S., et al. (2016). Analysis of Sporothrix schenckii sensu stricto and Sporothrix brasiliensis virulence in Galleria mellonella. J. Microbiol. Methods 122, 73–77. doi: 10.1016/j.mimet.2016.01.014
De Almeida, J. R. F., Jannuzzi, G. P., Kaihami, G. H., Breda, L. C. D., Ferreira, K. S., and De Almeida, S. R. (2018). An immunoproteomic approach revealing peptides from Sporothrix brasiliensis that induce a cellular immune response in subcutaneous sporotrichosis. Sci. Rep. 8:4192. doi: 10.1038/s41598-018-22709-8
de Beer, Z. W., Duong, T. A., and Wingfield, M. J. (2016). The divorce of Sporothrix and Ophiostoma: solution to a problematic relationship. Stud. Mycol. 83, 165–191. doi: 10.1016/j.simyco.2016.07.001
Della Terra, P. P., Rodrigues, A. M., Fernandes, G. F., Nishikaku, A. S., Burger, E., and de Camargo, Z. P. (2017). Exploring virulence and immunogenicity in the emerging pathogen Sporothrix brasiliensis. PLoS Negl. Trop. Dis. 11:e0005903. doi: 10.1371/journal.pntd.0005903
Dische, Z., and Shettles, L. B. (1948). A specific color reaction of methylpentoses and a spectrophotometric micromethod for their determination. J. Biol. Chem. 175, 595–603. doi: 10.1016/S0021-9258(18)57178-7
Erwig, L. P., and Gow, N. A. R. (2016). Interactions of fungal pathogens with phagocytes. Nat. Rev. Microbiol. 14, 163–176. doi: 10.1038/nrmicro.2015.21
Etchecopaz, A. N., Lanza, N., Toscanini, M. A., Devoto, T. B., Pola, S. J., Daneri, G. L., et al. (2020). Sporotrichosis caused by Sporothrix brasiliensis in Argentina: case report, molecular identification and in vitro susceptibility pattern to antifungal drugs. J. Mycol. Med. 30:100908. doi: 10.1016/j.mycmed.2019.100908
Fernandes, G. F., dos Santos, P. O., Rodrigues, A. M., Sasaki, A. A., Burger, E., and de Camargo, Z. P. (2013). Characterization of virulence profile, protein secretion and immunogenicity of different Sporothrix schenckii sensu stricto isolates compared with S. globosa and S. brasiliensis species. Virulence 4, 241–249. doi: 10.4161/viru.23112
Fernandes, K. S. S., Mathews, H. L., and Bezerra, L. M. L. (1999). Differences in virulence of Sporothrix schenckii conidia related to culture conditions and cell-wall components. J. Med. Microbiol. 48, 195–203. doi: 10.1099/00222615-48-2-195
Gorin, P. A. J., Haskins, R. H., Travassos, L. R., and Mendonca-Previato, L. (1977). Further studies on the rhamnomannans and acidic rhamnomannans of Sporothrix schenckii and Ceratocystis stenoceras. Carbohydr. Res. 55, 21–33. doi: 10.1016/S0008-6215(00)84440-7
Gow, N. A. R., Gooday, G. W., Russell, J. D., and Wilson, M. J. (1987). Infrared and X-ray diffraction data on chitins of variable structure. Carbohydr. Res. 165, 1–160.
Gow, N. A. R., Latge, J., and Munro, C. A. (2017). The fungal cell wall: structure, biosynthesis, and function. Microbiol. Spectr. 5, 1–25. doi: 10.1128/microbiolspec.FUNK-0035-2016
Hernández-Chávez, M. J., Pérez-García, L. A., Niño-Vega, G. A., and Mora-Montes, H. M. (2017). Fungal strategies to evade the host immune recognition. J. Fungi 3, 1–28. doi: 10.3390/jof3040051
Klis, F. M., Boorsma, A., and De Groot, P. W. J. (2006). Cell wall construction in Saccharomyces cerevisiae. Yeast 23, 185–202. doi: 10.1002/yea.1349
Kong, X., Xiao, T., Lin, J., Wang, Y., and Chen, H. D. (2006). Relationships among genotypes, virulence and clinical forms of Sporothrix schenckii infection. Clin. Microbiol. Infect. 12, 1077–1081. doi: 10.1111/j.1469-0691.2006.01519.x
Latgé, J. P. (2007). The cell wall: a carbohydrate armour for the fungal cell. Mol. Microbiol. 66, 279–290. doi: 10.1111/j.1365-2958.2007.05872.x
Lopes-Alves, L. M., Mendonça-Previato, L., Fournet, B., Degand, P., and Previato, J. O. (1992). O-Glycosidically linked oligosaccharides from peptidorhamnomannans of Sporothrix schenckii. Glycoconj. J. 9, 75–81. doi: 10.1007/BF00731702
Lopes-Bezerra, L. M., Walker, L. A., Niño-Vega, G., Mora-Montes, H. M., Neves, G. W. P., Villalobos-Duno, H., et al. (2018). Cell walls of the dimorphic fungal pathogens Sporothrix schenckii and Sporothrix brasiliensis exhibit bilaminate structures and sloughing of extensive and intact layers. PLoS Negl. Trop. Dis. 12, 1–25. doi: 10.1371/journal.pntd.0006169
Lozoya-Pérez, N. E., Clavijo-Giraldo, D. M., Martínez-Duncker, I., García-Carnero, L. C., López-Ramírez, L. A., Niño-Vega, G. A., et al. (2020). Influences of the culturing media in the virulence and cell wall of Sporothrix schenckii, Sporothrix brasiliensis, and Sporothrix globosa. J. Fungi 6:323. doi: 10.3390/jof6040323
Nascimento, R. C., Espíndola, N. M., Castro, R. A., Teixeira, P. A. C., Penha, C. V. L., Lopes-Bezerra, L. M., et al. (2008). Passive immunization with monoclonal antibody against a 70-kDa putative adhesin of Sporothrix schenckii induces protection in murine sporotrichosis. Eur. J. Immunol. 38, 3080–3089. doi: 10.1002/eji.200838513
Previato, J., Gorin, P., Haskins, R., and Travassos, L. (1979). Soluble and insoluble glucans from different cell types of the human pathogen Sporothrix schenckii. Exp. Mycol. 3, 92–105. doi: 10.1016/S0147-5975(79)80021-3
Rodríguez-Brito, S., Niño-Vega, G., and San-Blas, G. (2010). Caspofungin affects growth of Paracoccidioides brasiliensis in both morphological phases. Antimicrob. Agents Chemother. 54, 5391–5394. doi: 10.1128/AAC.00617-10
Rondle, C. J. M., and Morgan, W. T. J. (1955). The determination of glucosamine and galactosamine. Biochem. J. 61, 586–589. doi: 10.1042/bj0610586
Rossow, J. A., Queiroz-Telles, F., Caceres, D. H., Beer, K. D., Jackson, B. R., Pereira, J. G., et al. (2020). A one health approach to combatting Sporothrix brasiliensis: narrative review of an emerging zoonotic fungal pathogen in South America. J. Fungi 6, 1–27. doi: 10.3390/jof6040247
Ruiz-Herrera, J., Victoria Elorza, M., Valentín, E., and Sentandreu, R. (2006). Molecular organization of the cell wall of Candida albicans and its relation to pathogenicity. FEMS Yeast Res. 6, 14–29. doi: 10.1111/j.1567-1364.2005.00017.x
San-Blas, G., and San-Blas, F. (1994). “Preparation and analysis of purified cell walls of the mycelial and yeast phase of Paracoccidioides brasiliensis,” in Molecular Biology of Pathogenic Fungi: A Laboratory Manual. eds. B. Maresca and G. Kobayashi (New York: Telos Press), 489–498.
Synytsya, A., and Novak, M. (2014). Structural analysis of glucans. Ann. Transl. Med. 2, 1–14. doi: 10.3978/j.issn.2305-5839.2014.02.07
Teixeira, P. A. C., de Castro, R. A., Nascimento, R. C., Tronchin, G., Torres, A. P., Lazéra, M., et al. (2009). Cell surface expression of adhesins for fibronectin correlates with virulence in Sporothrix schenckii. Microbiology 155, 3730–3738. doi: 10.1099/mic.0.029439-0
Travassos, L. R. (1985). “Sporothrix schenckii,” in Fungal Dimorphism. eds. P. J. Szaniszlo and J. L. Harris (New York, London: Plenum Press), 121–163.
Travassos, L. R., Gorin, P. A. J., and Lloyd, K. O. (1973). Comparison of the rhamnomannans from the human pathogen Sporothrix schenckii with those from the Ceratocystis species. Infect. Immun. 8, 685–693. doi: 10.1128/iai.8.5.685-693.1973
Travassos, L. R., Gorin, P. A. J., and Lloyd, K. O. (1974). Discrimination between Sporothrix schenckii and Ceratocystis stenoceras rhamnomannans by proton and carbon-13 magnetic resonance spectroscopy. Infect. Immun. 9, 674–680. doi: 10.1128/iai.9.4.674-680.1974
Yemm, E. W., Cocking, E. C., and Ricketts, R. E. (1955). The determination of amino-acids with ninhydrin. Analyst 80, 209–214. doi: 10.1039/an9558000209
Keywords: Sporothrix spp., fungal cell wall, beta-gucan, fungal virulence, Rhamnose, Rhamnomannan
Citation: Villalobos-Duno HL, Barreto LA, Alvarez-Aular A, Mora-Montes HM, Lozoya-Pérez NE, Franco B, Lopes-Bezerra LM and Niño-Vega GA (2021) Comparison of Cell Wall Polysaccharide Composition and Structure Between Strains of Sporothrix schenckii and Sporothrix brasiliensis . Front. Microbiol. 12:726958. doi: 10.3389/fmicb.2021.726958
Received: 17 June 2021; Accepted: 24 August 2021;
Published: 20 September 2021.
Edited by:
Leonardo Nimrichter, Federal University of Rio de Janeiro, BrazilReviewed by:
Max Carlos Ramírez-Soto, Universidad Peruana Cayetano Heredia, PeruCopyright © 2021 Villalobos-Duno, Barreto, Alvarez-Aular, Mora-Montes, Lozoya-Pérez, Franco, Lopes-Bezerra and Niño-Vega. This is an open-access article distributed under the terms of the Creative Commons Attribution License (CC BY). The use, distribution or reproduction in other forums is permitted, provided the original author(s) and the copyright owner(s) are credited and that the original publication in this journal is cited, in accordance with accepted academic practice. No use, distribution or reproduction is permitted which does not comply with these terms.
*Correspondence: Gustavo A. Niño-Vega, Z3VzdGF2by5uaW5vQHVndG8ubXg=
Disclaimer: All claims expressed in this article are solely those of the authors and do not necessarily represent those of their affiliated organizations, or those of the publisher, the editors and the reviewers. Any product that may be evaluated in this article or claim that may be made by its manufacturer is not guaranteed or endorsed by the publisher.
Research integrity at Frontiers
Learn more about the work of our research integrity team to safeguard the quality of each article we publish.