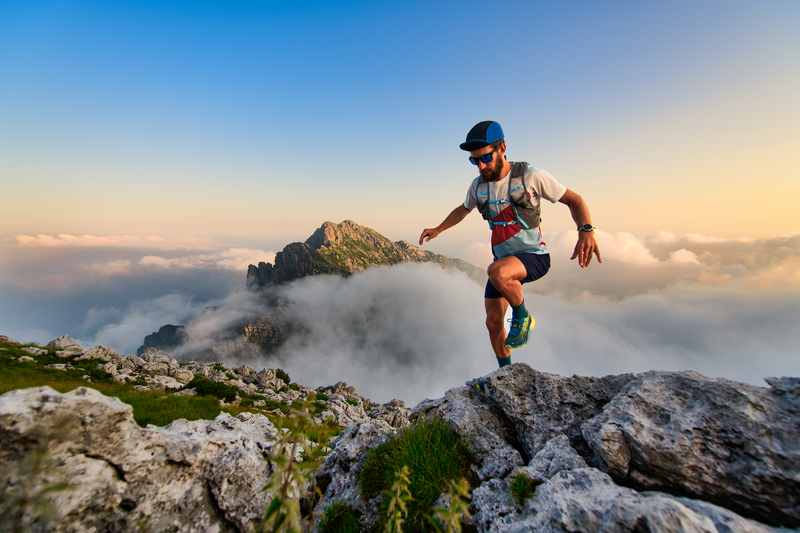
94% of researchers rate our articles as excellent or good
Learn more about the work of our research integrity team to safeguard the quality of each article we publish.
Find out more
ORIGINAL RESEARCH article
Front. Microbiol. , 01 October 2021
Sec. Aquatic Microbiology
Volume 12 - 2021 | https://doi.org/10.3389/fmicb.2021.721422
1,3-xylan is present in the cell walls of some red and green algae and is an important organic carbon in the ocean. However, information on its bacterial degradation is quite limited. Here, after enrichment with 1,3-xylan, the diversity of bacteria recovered from marine algae collected in Hainan, China, was analyzed with both the 16S rRNA gene amplicon sequencing and the culture-dependent method. Bacteria recovered were affiliated with more than 19 families mainly in phyla Proteobacteria and Bacteroidetes, suggesting a high bacterial diversity. Moreover, 12 strains with high 1,3-xylanase-secreting ability from genera Vibrio, Neiella, Alteromonas, and Gilvimarinus were isolated from the enrichment culture. The extracellular 1,3-xylanases secreted by Vibrio sp. EA2, Neiella sp. GA3, Alteromonas sp. CA13-2, and Gilvimarinus sp. HA3-2, which were taken as representatives due to their efficient utilization of 1,3-xylan for growth, were further characterized. The extracellular 1,3-xylanases secreted by these strains showed the highest activity at pH 6.0–7.0 and 30–40°C in 0–0.5M NaCl, exhibiting thermo-unstable and alkali-resistant characters. Their degradation products on 1,3-xylan were mainly 1,3-xylobiose and 1,3-xylotriose. This study reveals the diversity of marine bacteria involved in the degradation and utilization of 1,3-xylan, helpful in our understanding of the recycling of 1,3-xylan driven by bacteria in the ocean and the discovery of novel 1,3-xylanases.
Marine algae generate approximately 104.9 petagram organic carbon per year that mainly deposit as polysaccharides, the most promising candidates for biomass conversion (Field et al., 1998; Hehemann et al., 2014). There are many unusual polysaccharides in the ocean that are not found in land plants, including 1,3-xylan. In the cell walls of land plants, xylans are the most abundant polysaccharides secondary to cellulose. They are heteroxylans, and their β-1,4-linked D-xylopyranosyl backbone can be replaced by various side chains, such as α-L-arabinose, 4-O-methyl-glucuronic acid, and acetate (Déjean et al., 2013). Different from that, 1,3-xylan is a linear homopolysaccharide composed of β-1,3-linked D-xylose units (Iriki et al., 1960). 1,3-xylan, which is considered the main xylan structure in marine algae (Qeshmi et al., 2020), has been found in the cell walls of many green algae (Caulerpa, Dichotomosiphon, Halimeda, Penicillus, and Udotea spp.) and some red algae (Porphyra and Bangia spp.; Iriki et al., 1960; Veluraja and Atkins, 1987; Usov and Zelinsky, 2013).
1,3-xylanases (EC 3.2.1.32), capable of cleaving the β-1,3-xylosidic linkages in 1,3-xylan, are crucial in the degradation and recycling of 1,3-xylan in the ocean. They also represent a vast potential for algal biomass conversion, functional xylooligosaccharides production, and protoplast preparation (Araki et al., 1994; Maeda et al., 2012; Umemoto et al., 2012). However, to date, only a limited number of 1,3-xylanases have been studied. In the 1980s, six extracellular 1,3-xylanases produced by a fungus, Aspergillus terreus A-07, were purified (Chen et al., 1986). Later, several 1,3-xylanase-secreting marine bacteria were isolated, which are Pseudomonas sp. PT-5 (Yamaura et al., 1990), Alcaligenes sp. XY-234 (Araki et al., 1998), Vibrio sp. XY-214 (Araki et al., 1999), and Vibrio sp. AX-4 (Araki et al., 1987; Kiyohara et al., 2005). The genes encoding 1,3-xylanases from these bacteria were cloned and functionally characterized. In recent years, three other 1,3-xylanases were experimentally identified from Thermotoga neapolitana DSM 4359 (Okazaki et al., 2013), Pseudomonas vesicularis MA103 (Liang et al., 2015), and Flammeovirga pacifica WPAGA1 (Cai et al., 2018). So far, all the discovered 1,3-xylanases belong to glycoside hydrolase (GH) family 26 according to the assignment in the Carbohydrate Active Enzymes (CAZy) database (Lombard et al., 2014).1 Despite these studies, bacterial degradation on 1,3-xylan is still a largely unexplored field. The diversity of marine bacteria participating in the degradation and utilization of 1,3-xylan still lacks investigation, and more 1,3-xylanase-secreting bacteria and 1,3-xylanases await discovery and exploitation.
Algal surfaces harbor a rich community mainly composed of bacteria that can benefit from various organic substances produced by algae (Armstrong et al., 2001; Lachnit et al., 2011). They are good sources to isolate bacteria capable of degrading algal polysaccharides (Dong et al., 2012; Martin et al., 2015). In this study, we investigated the diversity of 1,3-xylan-utilizing bacteria associated with marine algae Caulerpa sp. and Chaetomorpha sp. and characterized the extracellular 1,3-xylanases secreted by several representative strains. Algal samples were collected from a Caulerpa lentillifera aquaculture base and the nearby beach in Hainan, China. After enrichment with 1,3-xylan, the bacterial diversity was analyzed using the 16S rRNA gene amplicon sequencing. The diversity of culturable bacteria was also analyzed. Moreover, 12 strains with high 1,3-xylanase-secreting ability belonging to 4 genera were isolated and identified. The extracellular 1,3-xylanases secreted by 4 representative strains were further characterized. The results shed new light on 1,3-xylan degradation and 1,3-xylanase-secreting bacteria.
Four algal samples, named C, E, G, and H, were collected from a Caulerpa lentillifera aquaculture base and the nearby beach in Wenchang, Hainan Province, China (111.045°E, 19.646°N) in September 2019 (Figure 1A). Samples C, E, and H were from the Caulerpa lentillifera aquaculture base, and sample G was from the beach. Samples C, E, G, and H are Caulerpa sertularioides, rotten Caulerpa lentillifera, rotten algae of which the taxonomy was unable to determine and Chaetomorpha sp., respectively (Figure 1B). The temperature and pH of the seawater in the sampling sites were 30°C and 8.0–8.2, respectively.
Figure 1. Geographic location of the sampling station (A) and sample images (B). At the sampling station, fresh and rotten seaweeds were collected from a Caulerpa lentillifera aquaculture base (samples C, E, and H) and the nearby beach (sample G) in Wenchang, Hainan, China. Samples C, E, G, and H are Caulerpa sertularioides, rotten Caulerpa lentillifera, unknown rotten algae and Chaetomorpha sp., respectively.
1,3-xylan was extracted from Caulerpa lentillifera according to the method of Lahaye et al. (2003) with minor modifications. Briefly, Caulerpa lentillifera was washed with deionized water, kiln-dried, and smashed into a powder. Then, the algal powder (10g) was boiled in 1.25% NaOH (500mL) for 30min. The same operation was repeated with 1.25% H2SO4, and then, the sample was bleached with 1.0% NaClO4 (500mL) at room temperature. Subsequently, 1,3-xylan was extracted with 10% NaOH (500mL) at 4°C for 4h and precipitated with absolute ethanol (2L) at 4°C overnight. The precipitate was collected and washed with 33% acetic acid and deionized water. Finally, the precipitate was freeze-dried to obtain water-insoluble 1,3-xylan. 1,3-xylooligosaccharides were prepared by enzymatic hydrolysis of 1,3-xylan with 1,3-xylanase XYL4 from Vibrio sp. AX-4 as previously described (Kiyohara et al., 2006). The gene encoding XYL4 was synthesized in the Beijing Genomics Institute (BGI; Beijing, China) and expressed in Escherichia coli BL21(DE3). The recombinant XYL4 was purified with Ni2+-nitrilotriacetic acid (NTA) resin (GE Healthcare, USA) followed by desalination on PD-10 desalting columns (GE Healthcare, USA). The purified XYL4 was stored in 10mM Tris–HCl buffer (pH 8.0) containing 100mM NaCl at −80°C for further use. Enzymatic hydrolysis of 1,3-xylan by the purified XYL4 to prepare 1,3-xylooligosaccharides was carried out as follows: A reaction mixture consisting of 0.4g 1,3-xylan and 200mg XYL4 in 40mL phosphate-buffered saline (PBS; 20mM, pH 7.0) was incubated at 30°C for 24h. The products were then separated by gel filtration on a Superdex 30 Increase 10/300 GL column (GE Healthcare, USA) by high-performance liquid chromatography (HPLC) equipped with a RID-20A refractive index detector (HPLC-RID). The injected volume was 100μL. The products were eluted with deionized water for 60min with a flow rate of 0.37mL/min. A mixture of xylose and 1,4-xylooligosaccharides (1,4X2-X6; Megazyme, Ireland) was used as the marker. The peaks corresponding to 1,3-xylobiose (1,3X2) and 1,3-xylotriose (1,3X3) were collected for further use (Supplementary Figure 1).
Two media, A and B, were used for enrichment cultivation. Medium A contained 0.2% 1,3-xylan, 0.05% NH4Cl, and 0.1% (v/v) vitamin solution in artificial seawater (ASW). Medium B contained the same components as medium A and an additional organic nitrogen source, 0.02% casein hydrolysate. ASW was composed of 30g NaCl, 6g Tris, 3g MgCl2.6H2O, 2g K2SO4, 0.2g K2HPO4, 10mg CaCl2, 6mg FeCl3.6H2O, 5mg Na2MoO2.7H2O, and 4mg CuCl2.2H2O in 1L deionized water (Li et al., 2021). The pH was adjusted to 8.0 with HCl to keep consistence with that of the sampling sites (8.0–8.2). The compositions of the vitamin solution were 20mg nicotinic acid, 20mg pyridoxine-HCl, 20mg riboflavin, 20mg calcium pantothenate, 10mg thiamine-HCl, 10mg p-aminobenzoic acid, 1mg biotin, and 1mg cyanocobalamin in 1L deionized water (Kanagawa et al., 1982). Each algal sample was cut into small pieces using sterile scissors, and then, approximately 1g of each algal sample was put in 20mL media A and B. After aerobic incubation at 30°C with continuous shaking of 180rpm for 6–7days, 0.2mL enrichment culture was inoculated into 20mL the same fresh medium and incubated under the same conditions. After repeated enrichment, the bacterial diversity of each sample was analyzed in Shanghai Biozeron Biotechnology Co., Ltd. (Shanghai, China) by using the 16S rRNA gene amplicon sequencing. Briefly, the V3 to V4 hypervariable region of the bacterial 16S rRNA gene was amplified from the total bacterial genomic DNA using the universal primers 341F (5'-CCTAYGGGRBGCASCAG-3') and 806R (5'-GGACTACNNGGGTATCTAAT-3'). High-throughput sequencing of the PCR amplification products was performed on an Illumina MiSeq platform. The paired-end reads were merged into longer contigs by FLASH and quality-filtered by Trimmomatic. After removing chimeric sequences, the remaining sequences were assigned into operational taxonomic units (OTUs) at similarities of 97% using UPARSE version 7.1 (Edgar, 2013). The taxonomy of each 16S rRNA gene sequence was analyzed by the ribosomal database project (RDP) classifier algorithm against the Silva 16S rRNA database using a confidence threshold of 70% (Wang et al., 2007). The richness (Chao-1) and diversity (Shannon) indexes were analyzed using Mothur (Chappidi et al., 2019). Principal component analysis (PCA) was performed in R package Vegan and used to evaluate the differences among bacterial communities. Differences were considered significant at p-values less than 0.001.
The enrichment cultures were diluted (10−2–10−6 dilution) and spread on the corresponding screening plates containing medium A or B supplemented with 1.5% agar powder. The plates were then incubated at 30°C until detectable colonies and hydrolytic zones formed. Morphologically different colonies were selected and purified with 2216E medium. The compositions of 2216E medium were 5g tryptone, 1g yeast extract, and 30g sea salts (Sigma, USA) in 1L deionized water. Strains with apparent hydrolytic zones were taken as 1,3-xylanase-secreting bacteria.
The 16S rRNA genes were amplified by PCR with the primers 27F (5'-AGAGTTTGATCCTGGCTCAG-3') and 1492R (5'-GGTTACCTTGTTACGACTT-3'; Dong et al., 2012). The PCR products were sequenced in BGI (Beijing, China). The sequence alignment was performed in the EZBioCloud database2 (Yoon et al., 2017). The phylogenetic tree was constructed based on the neighbor-Joining method and using the Kimura 2-parameter model (Kimura, 1980) with MEGA X (Kumar et al., 2018).
The strains Vibrio sp. EA2, Neiella sp. GA3, Alteromonas sp. CA13-2, and Gilvimarinus sp. HA3-2, which were all isolated from medium A, were aerobically cultured in 2216E medium at 30°C and 180rpm for 24h. The cells were collected, washed three times with sterile ASW, and resuspended in ASW to an optical density at 600nm of 0.8. Then, 1mL cell suspension was inoculated into 20mL medium A in triplicate and aerobically cultured at 30°C and 180rpm. At intervals of 6h, 1mL culture was removed into a 1.5-mL Eppendorf tube and let stand for 10min. As a result, water-insoluble 1,3-xylan precipitated and the bacterial cells still suspended in the culture. Then, the cell suspension was removed into a 96-well microplate and its optical density at 600nm was measured to generate growth curves. In the growth assays with Caulerpa lentillifera, the strains were cultured in the same conditions except that 1.0% dry Caulerpa lentillifera was used instead of 0.2% 1,3-xylan.
To determine the extracellular 1,3-xylanase activity, the culture (1mL) taken at intervals of 6h was centrifuged at 13,000rpm and 4°C for 5min, and the culture supernatant was collected. The extracellular 1,3-xylanase activity in the supernatant was determined by the dinitrosalicylic acid (DNS) method (Miller et al., 1960). A standard reaction system contained 10μL culture supernatant and 90μL 1,3-xylan (1.0%) in PBS (20mM, pH 7.0). The reaction system was incubated at 30°C for 1h (for the 1,3-xylanases from Vibrio sp. EA2 and Alteromonas sp. CA13-2) or 3.5h (for the 1,3-xylanases from Neiella sp. GA3 and Gilvimarinus sp. HA3-2). Then, the reaction was terminated by the addition of 200μL DNS, and the reaction mixture was boiled for 5min for coloring. Finally, the absorbance of the reaction mixture at 550nm was measured. One unit of enzyme activity is defined as the amount of enzyme required to release 1μmol xylose per min.
Vibrio sp. EA2, Neiella sp. GA3, Alteromonas sp. CA13-2, and Gilvimarinus sp. HA3-2 were aerobically cultured in medium A at 30°C and 180rpm for 24h, 24h, 72h, and 30h, respectively, to their late-log phase. The cultures were centrifuged, and the supernatants were collected and used for the characterization of the extracellular 1,3-xylanases. The effect of temperature on the activity of extracellular 1,3-xylanases was determined from 10°C to 60°C in PBS at their respective optimum PHs. The effect of pH on the activity of extracellular 1,3-xylanases was determined with the Britton-Robinson buffer from pH 4.0 to 10.0 at their respective optimum temperatures. The effect of NaCl concentration on the activity of extracellular 1,3-xylanases was determined in PBS containing 0 to 5.0M NaCl at their respective optimum temperatures and pHs. In the thermo-stability assay, after incubation of 1,3-xylanases at 10°C to 60°C for different time periods, the residual activity was determined at their respective optimum temperatures and pHs in PBS. In the pH stability assay, 40μL 1,3-xylanases were diluted in 360μL Britton-Robinson buffers (pH 4.0–10.0) and preincubated at 4°C for 24h. After incubation, 3.6mg 1,3-xylan was added to the 400μL mixture and the residual activity was determined at the optimum temperature. 1,3-xylanases diluted in the corresponding Britton-Robinson buffers without preincubation were used as controls. Each experiment was performed in triplicate independently.
Culture supernatant from each strain (20μL) was incubated with 1.0% 1,3-xylan (180μL) in PBS (pH 7.0) for 24h. Then, the products were analyzed by HPLC equipped with an evaporative light scattering detector (HPLC-ELSD). The column used was a Superdex 30 Increase 10/300 GL column, and the eluent was deionized water. The injected volume was 30μL. The products were eluted with deionized water for 60min with a flow rate of 0.37mL/min. A mixture of xylose, 1,3X2, and 1,3X3 was used as the marker. The reaction system without 1,3-xylanases was used as the control.
The 16S rRNA gene sequences of 12 1,3-xylanase-secreting bacteria in this study were deposited in GenBank with the following accession numbers: Vibrio sp. CB1, MZ340577; Vibrio sp. EA6, MZ340626; Vibrio sp. EA17, MZ342596; Vibrio sp. EA2, MZ342745; Vibrio sp. EA3, MZ342757; Neiella sp. GA1-2, MZ342758; Neiella sp. GA3, MZ342759; Alteromonas sp. CA11-2, MZ340547; Alteromonas sp. CA13-2, MZ340550; Gilvimarinus sp. HB14, MZ342767; Gilvimarinus sp. HA9-2S, MZ342768; and Gilvimarinus sp. HA3-2, MZ342787. The accession number for Marisediminitalea sp. HA8 is MZ821015, and the other 133 strains are from MZ816037 to MZ816169. The 16S rRNA gene amplicon sequencing data have been deposited in Sequence Read Archive (SRA) under the accession numbers from SRR15511288 to SRR15511295.
1,3-xylan-containing green algae, including Caulerpa lentillifera, are widely distributed in tropical seas. To investigate the bacteria involved in 1,3-xylan degradation, a Caulerpa lentillifera aquaculture base and the nearby beach in Wenchang, Hainan Province, China, were chosen for sample collection (Figure 1A). Four algal samples were collected, named C, E, G, and H (Figure 1B), which are Caulerpa sertularioides (C), rotten Caulerpa lentillifera (E), rotten algae of which the taxonomy was unable to determine (G), and Chaetomorpha sp. (H), respectively (Figure 1B). Seawater in the sampling sites exhibited a slightly alkaline pH (8.0–8.2), and the temperature was approximately 30°C.
1,3-xylan, which was extracted from Caulerpa lentillifera, was used as the carbon source in media A and B to recover 1,3-xylan-utilizing bacteria from the 4 algal samples. Medium A contained NH4Cl as the nitrogen source. Medium B also contained an additional organic nitrogen source, casein hydrolysate, in case some 1,3-xylan-utilizing bacteria are not able to utilize inorganic nitrogen. The community compositions of the recovered bacteria were analyzed with the 16S rRNA gene amplicon sequencing. Based on the observed OTUs and Chao1 index, sample H showed the highest species richness and sample C showed the lowest species richness (Table 1). Between media A and B, the OTUs and Chao1 indexes of each sample were similar, indicating that the species richness of these samples was little affected by the nitrogen source (Table 1). According to the Shannon diversity indexes, in media A and B, sample G was the most diverse with the highest Shannon index and sample E was the least diverse with the lowest Shannon index (Table 1). Except sample H that exhibited a lower Shannon index in medium B than in medium A, the Shannon indexes were similar between media A and B for samples C, E, and G (Table 1), indicating that the nitrogen source generally had little influence on the bacterial diversity of the samples.
Table 1. Richness and diversity indexes of the bacteria recovered from algal samples with 1,3-xylan based on 16S rRNA gene amplicon sequencing.
Totally, 9 phyla, 15 classes, 55 orders, 94 families, and 194 genera were identified in these samples. At the phylum level, Proteobacteria (71.4%) and Bacteroidetes (28.1%) were two abundant groups, and the others accounted for less than 1% (Supplementary Figure 2). At the family level, there were 19 families with a relative abundance of higher than 1% in at least one sample, 18 of which were recovered from both media A and B (Figures 2A,B). Overall, Rhodobacteraceae (30.5%), Flavobacteriaceae (18.9%), Rhizobiaceae (9.5%), and Vibrionaceae (8.3%) were four abundant families (Figure 2A). Based on a Venn diagram analysis, 15 of the 19 families were common in all algal samples (Figure 2C), but the dominant families varied among these samples (Figure 2A; Supplementary Table 1). Bradymonadaceae, which was only recovered from medium B (Figure 2B), was unique in sample G, and Rickettsiales was unique in sample H (Figure 2C). The results indicated a diversity of the core bacteria involving in the 1,3-xylan utilization. The PCA result showed that the community structures of samples G and H were similar, which were significantly different from those of samples C and E (Figure 2D). In addition, the communities from media A and B exhibited only a slight difference for each sample, indicating that the nitrogen source had little impact on the community structure (Figure 2D), although the dominant families from media A and B varied for samples C, G, and H (Figure 2A; Supplementary Table 1). Together, these results reveal the high diversity of 1,3-xylan-utilizing bacteria associated with marine algae.
Figure 2. The bacterial communities recovered from algal samples with 1,3-xylan based on 16S rRNA gene amplicon sequencing. (A) Relative abundance of recovered bacteria at the family level. (B) A venn diagram analysis of recovered families from algal samples. (C) A venn diagram analysis of recovered families from media A and B. (D) Principal component analysis (PCA) of the bacterial communities.
After enrichment, bacteria were further isolated from the enrichment culture on plates with 1,3-xylan as the carbon source. After incubation at 30°C for 4–6days, a lot of colonies appeared on the plates. Morphologically different colonies (12–24 colonies each sample) were purified and identified. Totally, 146 strains were isolated, which were mainly distributed in Proteobacteria (87.7%) and Bacteroidetes (11.6%; Table 2). These strains were affiliated with 18 families in Proteobacteria, 5 families in Bacteroidetes, and 1 family in Actinobacteria (Table 2). Among these families, Vibrionaceae (16.4%) was predominant, followed by Rhodobacteraceae (13.0%), Phyllobacteriaceae (11.6%), and Cellvibrionaceae (9.6%; Table 2). A total of 33 genera were isolated, among which, 1, 6, 9, and 3 genera were exclusive to samples C, E, G, and H, respectively. Vibrio species were isolated from all algal samples (Table 2; Supplementary Figure 3). In addition, among the isolates, strains HA8 and HB14 share low 16S rRNA gene sequence similarities with Marisediminitalea aggregate WH169 (similarity: 95.13%) and Gilvimarinus chinensis DSM 19667 (similarity: 96.65%), respectively. They might be potential novel species and deserved to be investigated in the future. Together, these results further indicate a high diversity of marine bacteria capable of utilizing 1,3-xylan.
Table 2. Phylogenetic classification and relative abundance of culturable bacteria isolated from the enrichment cultures of the algal samplesa.
Among the culturable bacteria, 12 strains formed apparent hydrolytic zones on the plates containing 1,3-xylan (Table 3; Figure 3A), suggesting that these strains have a high 1,3-xylanase-secreting ability. A neighbor-joining tree based on the 16S rRNA gene sequences illustrated their phylogenetic relationship with different genera (Figure 3B). The 12 strains were closely related to their closest neighbors in Vibrio (5 of 12 strains), Neiella (2 of 12 strains), Altermonas (2 of 12 strains), and Gilvimarinus (3 of 12 strains), all belonging to phylum Proteobacteria (Table 3; Figure 3B). Also, all the reference strains are from marine sources. In these genera, only Vibrio strains have been reported to secrete 1,3-xylanase, including Vibrio sp. XY214 (Araki et al., 1999) and Vibrio sp. AX-4 (Araki et al., 1987). Strains in the other 3 genera are first found to have the 1,3-xylanase-secreting ability.
Figure 3. Hydrolytic zones of the isolated 1,3-xylanase-secreting bacteria cultured on plates containing 0.2% 1,3-xylan (A) and the neighbor-joining phylogenetic tree based on the 16S rRNA gene sequences of these strains and their closest neighbors (B). These strains were cultured at 30°C for 5days for the observation of their hydrolytic zones. The neighbor-joining tree was built using the Kimura 2-parameter model (Kimura, 1980). A bootstrap test of 1,000 replicates was conducted, and values above 50% are shown. Representative strains for detailed study are marked with solid circles.
Vibrio sp. EA2, Neiella sp. GA3, Alteromonas sp. CA13-2, and Gilvimarinus sp. HA3-2 were chosen from the four genera as representatives for further study. With 1,3-xylan as the carbon source, we determined the growth curves of the 4 strains. Meanwhile, the extracellular 1,3-xylanase activity in the culture during bacterial growth was monitored. The results showed that these 4 strains effectively used 1,3-xylan as the carbon source for their growth (Figure 4). The extracellular 1,3-xylanase activity reached the highest level at the late-log phase for each strain (Figure 4). In addition, when cultured with Caulerpa lentillifera, these 4 strains also effectively decomposed intact algae for their growth (Supplementary Figure 4).
Figure 4. Growth and the extracellular 1,3-xylanase activities of Vibrio sp. EA2 (A), Neiella sp. GA3 (B), Alteromonas sp. CA13-2 (C), and Gilvimarinus sp. HA3-2 (D) cultured with 0.2% 1,3-xylan as the carbon source. The 1,3-xylanase activities were determined at 30°C in PBS (pH 7.0), and the highest activity was taken as 100%. The highest activities were 0.25±0.01U/mL (for Vibrio sp. EA2), 0.06±0.001U/mL (for Neiella sp. GA3), 0.21±0.01U/mL (for Alteromonas sp. CA13-2), and 0.10±0.01U/mL (for Gilvimarinus sp. HA3-2). Cultures without 1,3-xylan were treated as controls. The data shown in the graph are from triplicate experiments (mean±S.D.).
To characterize the extracellular 1,3-xylanases, culture supernatants of Vibrio sp. EA2, Neiella sp. GA3, Alteromonas sp. CA13-2, and Gilvimarinus sp. HA3-2 at the late-log phase were collected. The extracellular 1,3-xylanases produced by Vibrio sp. EA2 exhibited the highest activity at 40°C and pH 7.0 in 0M NaCl. After 1-h incubation at 50°C, they retained 45% activity, indicating a thermo-unstable character at 50°C. However, they showed an excellent tolerance over a wide pH range, retaining more than 80% activity at pH 5.0–10.0 (Table 4; Supplementary Figure 5). Their products released from 1,3-xylan were 1,3X2, 1,3X3 and a small amount of 1,3X4 (Figure 5A). The extracellular 1,3-xylanases produced by Neiella sp. GA3 exhibited the highest activity at 40°C and pH 7.0 in 0.5M NaCl. They retained 57% activity after 1-h incubation at 50°C and~90% activity over a wide pH range of 6.0–10.0 (Table 4; Supplementary Figure 6). Their products released from 1,3-xylan were 1,3X2, 1,3X3, 1,3X4, and a trace amount of xylose (Figure 5B). The extracellular 1,3-xylanases produced by Alteromonas sp. CA13-2 exhibited the highest activity at 30°C and pH 7.0 in 0.1M NaCl. After 1-h incubation at 40°C and 50°C, 22% and 1% activities were retained, respectively. After 24-h incubation at pH 4.0–9.0, more than 80% activity was retained. Even at pH 10.0, they retained 66% activity, indicating their alkali resistance (Table 4; Supplementary Figure 7). They acted on 1,3-xylan and released 1,3X2, 1,3X3, and a small amount of xylose and 1,3X4 (Figure 5C). The extracellular 1,3-xylanases produced by Gilvimarinus sp. HA3-2 exhibited the highest activity at 30°C and pH 6.0 in 0.1M NaCl. They lost almost all activity after 1-h incubation at 50°C. At pH 10.0, they retained 58% activity (Table 4; Supplementary Figure 8). Their products released from 1,3-xylan were 1,3X2 and 1,3X3 and a small amount of 1,3X4. The produced xylose was negligible (Figure 5D). Together, the extracellular 1,3-xylanases produced by these 4 strains exhibit the highest activity at mesophilic conditions and neutral pH in 0–0.5M NaCl and hydrolyze 1,3-xylan into xylooligosaccharides mainly 1,3X2 and 1,3X3. In addition, these 1,3-xylanases are thermo-unstable at 50°C, but exhibit good alkali resistance.
Table 4. Biochemical characterization of the extracellular 1,3-xylanases secreted by Vibrio sp. EA2, Neiella sp. GA3, Alteromonas sp. CA13-2, and Gilvimarinus sp. HA3-2a.
Figure 5. The products released from 1,3-xylan by the hydrolysis of the extracellular 1,3-xylanases secreted by Vibrio sp. EA2 (A), Neiella sp. GA3 (B), Alteromonas sp. CA13-2 (C), and Gilvimarinus sp. HA3-2 (D). Extracellular 1,3-xylanases from each strain were incubated with 1,3-xylan at 30°C for 24h. Then, the products were analyzed by HPLC-ELSD. The intensity of the 1,3-xylotriose in each chromatogram was normalized as 100%. The reaction system without 1,3-xylanases was used as the control. The data are representative of the results of triplicate experiments.
Bacteria that decompose algal polysaccharides are thought to be pivotal in marine carbon cycling (Williams et al., 2013; Hehemann et al., 2014). However, for 1,3-xylan, the main structural constituent in the cell walls of some red and green algae (Iriki et al., 1960; Veluraja and Atkins, 1987), only 4 1,3-xylanase-secreting bacteria have been isolated from marine sources including Vibrio sp. AX-4 (Araki et al., 1987), Pseudomonas sp. PT-5 (Yamaura et al., 1990), Alcaligenes sp. XY-234 (Araki et al., 1998), and Vibrio sp. XY-214 (Araki et al., 1999). Pseudomonas sp. PT-5 was isolated from edible seaweed, nori, and the other 3 bacterial strains were isolated from the sea mud samples. Despite these studies, there has been no report on the diversity of bacteria involved in the degradation and utilization of 1,3-xylan. In this study, with the 16S rRNA gene amplicon sequencing and the culture-dependent method, the diversity of 1,3-xylan-utilizing bacteria from algae collected from Hainan, China, was investigated. After enrichment with 1,3-xylan, bacteria from phyla Proteobacteria and Bacteroidetes take a leading role in the bacterial community, consistent with the finding that Proteobacteria and Bacteroidetes are master decomposers for algal polysaccharides (Teeling et al., 2012; Barbeyron et al., 2016). In these two phyla, at least 19 families were determined, unveiling that a wide range of marine bacteria has the 1,3-xylan-utilizing ability.
In this study, we investigate the bacteria involved in 1,3-xylan degradation and utilization associated with Caulerpa sertularioides, Caulerpa lentillifera, and Chaetomorpha sp. Among these algae, only the bacterial diversity associated with Caulerpa lentillifera has been analyzed with the 16S rRNA gene amplicon sequencing (Liang et al., 2019), which showed that the bacteria are predominantly from phyla Proteobacteria (52.1%), Planctomycetes (21.1%), Bacteroidetes (13.5%), and Cyanobacteria (7.8%). Our results in this study showed that 1,3-xylan-utilizing bacteria associated with Caulerpa lentillifera mainly belong to phyla Proteobacteria (71.4%) and Bacteroidetes (28.1%); however, the recovered Planctomycetes bacteria (0.02%) are negligible and no Cyanobacteria bacteria are recovered from Caulerpa lentillifera.
Bacteria from genera Vibrio, Pseudomonas, and Alcaligenes have been reported to secrete 1,3-xylanases (Yamaura et al., 1990; Araki et al., 1998, 1999; Kiyohara et al., 2005). In this study, 12 bacterial strains with high 1,3-xylanase-secreting ability were isolated, which formed apparent hydrolytic zones on plates containing 1,3-xylan. Among them, bacteria belonging to genera Neiella, Altermonas, and Gilvimarinus are first found to secrete 1,3-xylanases. Interestingly, the 12 strains isolated in this study and their closest neighbors are all from coastal environments, where the bacterial response to phytoplankton blooms is dynamic (Teeling et al., 2012). The neighbors Gilvimarinus chinensis DSM 19667 and Alteromonas portus HB161718 have been shown to have agar- and alginate-digesting capacity, respectively (Du et al., 2009; Huang et al., 2020). Probably, the 12 strains isolated in this study also exhibit the capacity to degrade other algal polysaccharides and participate in coastal polysaccharides degradation as specific or versatile degraders, which, however, needs further investigation. Notably, only 12 of 146 (8.2%) culturable bacteria were isolated with high 1,3-xylanase-secreting ability. There are two possible reasons for this. First, there may be a considerable number of bacteria whose 1,3-xylanase-secreting capacity is too weak to form an apparent hydrolytic zone around its colony. Second, a proportion of culturable bacteria may be unable to degrade 1,3-xylan and rely on 1,3-xylooligosaccharides generated by other bacteria for growth.
1,3-xylanases play a key role in 1,3-xylan degradation and recycling in the ocean, which also exhibit vast potentials in bioenergy, pharmaceutical, and biotechnology industries (Araki et al., 1994; Maeda et al., 2012; Umemoto et al., 2012). Thus, the discovery of 1,3-xylanases from 1,3-xylanase-secreting bacteria will facilitate the utilization of algal resources. Previously reported 1,3-xylanase from Vibrio sp. AX-4 has the highest activity at pH 6.0–7.5 and 30°C (Araki et al., 1987; Kiyohara et al., 2005). The 1,3-xylanase from Pseudomonas sp. PT-5 shows its maximum activity at pH 7.5 and is stable at pH 5.5–8.0 (Yamaura et al., 1990). 1,3-xylanases from Alcaligenes sp. XY-234 (Araki et al., 1998) and Vibrio sp. XY-214 (Araki et al., 1999) have been well studied. They exhibit the highest activity at pH 7.0–7.5 and 37–40°C and are stable under 40°C and 30°C, respectively. Moreover, the 1,3-xylanases produced by Alcaligenes sp. XY-234 are stable at pH 6.0–10.0, showing good alkali resistance. In this study, extracellular 1,3-xylanases secreted by 4 representative strains were biochemically characterized. The 1,3-xylanases from the strains show the highest activity at pH 6.0–7.0 and 30–40°C and remain high activities at pH 8.0, suggesting their adaptation to the marine environment where these strains were isolated. These 1,3-xylanases are thermo-unstable but alkali resistance. At the corresponding optimum conditions, the activities of the 1,3-xylanases from the 4 strains are in the range of 0.07–0.53U/mL, higher than that produced by Vibrio sp. AX-4 (0.03U/ml; Araki et al., 1987). Moreover, the products of these enzymes to hydrolyze 1,3-xylan are mainly 1,3X2 and 1,3X3, similar to those of xylanases produced by Vibrio sp. AX-4 (Araki et al., 1987), Alcaligenes sp. XY-234 (Araki et al., 1998), and Vibrio sp. XY-214 (Araki et al., 1999). Thus, these 1,3-xylanases may have potential in the preparation of 1,3-xylooligosaccharides. These results lay a foundation for the discovery of 1,3-xylanases.
In summary, this study reveals the diversity of marine bacteria involved in the degradation and utilization of 1,3-xylan. Twelve strains with high 1,3-xylanase-secreting capacity were isolated, and the extracellular 1,3-xylanases secreted by 4 representative strains were biochemically characterized. These findings shed light on the ecological functions of 1,3-xylanase-secreting bacteria and their extracellular 1,3-xylanases. The research also helps in further elucidating the degradation mechanism of 1,3-xylan and discovering novel 1,3-xylanases. Detailed studies on the 1,3-xylanase-secreting bacteria and 1,3-xylanases are on the way.
The datasets presented in this study can be found in online repositories. The names of the repository/repositories and accession number(s) can be found at: https://www.ncbi.nlm.nih.gov/genbank/ (MZ340577, MZ340626, MZ342596, MZ342745, MZ342757, MZ342758, MZ342759, MZ340547, MZ340550, MZ342767, MZ342768, MZ342787, MZ821015, and from MZ816037 to MZ816169) and at: https://www.ncbi.nlm.nih.gov/sra/ (from SRR15511288 to SRR15511295).
FZ, X-LC, and H-NS designed and directed the research. H-NS and C-MY performed the experiments. Z-GF helped in sample collection. H-HF and PW helped in data analysis. FZ and H-NS wrote the manuscript. X-LC and Y-ZZ revised the manuscript. All authors contributed to the article and approved the submitted version.
The work was supported by the Major Scientific and Technological Innovation Project (MSTIP) of Shandong Province (2019JZZY010817 awarded to Y-ZZ), the National Science Foundation of China (grants U2006205 and U1706207, awarded to X-LC and Y-ZZ, respectively), and Taishan Scholars Program of Shandong Province (tspd20181203 awarded to Y-ZZ).
The authors declare that the research was conducted in the absence of any commercial or financial relationships that could be construed as a potential conflict of interest.
All claims expressed in this article are solely those of the authors and do not necessarily represent those of their affiliated organizations, or those of the publisher, the editors and the reviewers. Any product that may be evaluated in this article, or claim that may be made by its manufacturer, is not guaranteed or endorsed by the publisher.
We thank Cai-Yun Sun and Xiang-Mei Ren from State Key Laboratory of Microbial Technology of Shandong University for help and guidance in HPLC. We also thank Guang-Ya Zhang from Department of Biotechnology and Bioengineering of Huaqiao University for technical help in 1,3-xylan extraction.
The Supplementary Material for this article can be found online at: https://www.frontiersin.org/articles/10.3389/fmicb.2021.721422/full#supplementary-material
Araki, T., Aoki, T., and Kitamikado, M. (1987). Isolation and identification of a β-1,3-xylanase-producing bacterium. Nippon Suisan Gakkaishi 53, 2077–2081. doi: 10.2331/suisan.53.2077
Araki, T., Hayakawa, M., Tamaru, Y., Yoshimatsu, K., and Morishita, T. (1994). Isolation and regeneration of haploid protoplasts from Bangia Atropurpurea (Rhodophyta) with marine bacterial enzymes. J. Phycol. 30, 1040–1046. doi: 10.1111/j.0022-3646.1994.01040.x
Araki, T., Inoue, N., and Morishita, T. (1998). Purification and characterization of beta-1,3-xylanase from a marine bacterium, Alcaligenes sp. XY-234. J. Gen. Appl. Microbiol. 44, 269–274. doi: 10.2323/jgam.44.269
Araki, T., Tani, S., Maeda, K., Hashikawa, S., Nakagawa, H., and Morishita, T. (1999). Purification and characterization of beta-1,3-xylanase from a marine bacterium, vibrio sp. XY-214. Biosci. Biotechnol. Biochem. 63, 2017–2019.
Armstrong, E., Yan, L., Boyd, K. G., Wright, P. C., and Burgess, J. G. (2001). The symbiotic role of marine microbes on living surfaces. Hydrobiologia 461, 37–40. doi: 10.1023/A:1012756913566
Barbeyron, T., Thomas, F., Barbe, V., Teeling, H., Schenowitz, C., Dossat, C., et al. (2016). Habitat and taxon as driving forces of carbohydrate catabolism in marine heterotrophic bacteria: example of the model algae-associated bacterium Zobellia galactanivorans DsijT. Environ. Microbiol. 18, 4610–4627. doi: 10.1111/1462-2920.13584
Cai, Z. W., Ge, H. H., Yi, Z. W., Zeng, R. Y., and Zhang, G. Y. (2018). Characterization of a novel psychrophilic and halophilic β-1,3-xylanase from deep-sea bacterium, Flammeovirga pacifica strain WPAGA1. Int. J. Biol. Macromol. 118, 2176–2184. doi: 10.1016/j.ijbiomac.2018.07.090
Chappidi, S., Villa, E. C., and Cantarel, B. L. (2019). Using Mothur to determine bacterial community composition and structure in 16S ribosomal RNA datasets. Curr. Protoc. Bioinformatics 67:e83. doi: 10.1002/cpbi.83
Chen, W. P., Matsuo, M., and Yasui, T. (1986). Purification and some properties of β-1,3-xylanase from Aspergillus terreus A-07. Agric. Biol. Chem. 50, 1183–1194.
Déjean, G., Blanvillain-Baufumé, S., Boulanger, A., Darrasse, A., Dugé de Bernonville, T., Girard, A. L., et al. (2013). The xylan utilization system of the plant pathogen Xanthomonas campestris pv campestris controls epiphytic life and reveals common features with oligotrophic bacteria and animal gut symbionts. New Phytol. 198, 899–915. doi: 10.1111/nph.12187
Dong, S., Yang, J., Zhang, X. Y., Shi, M., Song, X. Y., Chen, X. L., et al. (2012). Cultivable alginate lyase-excreting bacteria associated with the Arctic brown alga Laminaria. Mar. Drugs 10, 2481–2491. doi: 10.3390/md10112481
Du, Z. J., Zhang, D. Z., Liu, S. N., Chen, J. X., Tian, X. L., Zhang, Z. N., et al. (2009). Gilvimarinus chinensis gen. nov., sp. nov., an agar-digesting marine bacterium within the class Gammaproteobacteria isolated from coastal seawater in Qingdao, China. Int. J. Syst. Evol. Microbiol. 59, 2987–2990. doi: 10.1099/ijs.0.001313-0
Edgar, R. C. (2013). UPARSE: highly accurate OTU sequences from microbial amplicon reads. Nat. Methods 10, 996–998. doi: 10.1038/nmeth.2604
Field, C. B., Behrenfeld, M. J., Randerson, J. T., and Falkowski, P. (1998). Primary production of the biosphere: integrating terrestrial and oceanic components. Science 281, 237–240. doi: 10.1126/science.281.5374.237
Hehemann, J. H., Boraston, A. B., and Czjzek, M. (2014). A sweet new wave: structures and mechanisms of enzymes that digest polysaccharides from marine algae. Curr. Opin. Struct. Biol. 28, 77–86. doi: 10.1016/j.sbi.2014.07.009
Huang, H. Q., Mo, K. L., Li, S., Sun, D. M., Zhu, J., Zou, X. X., et al. (2020). Alteromonas portus sp. nov., an alginate lyase-excreting marine bacterium. Int. J. Syst. Evol. Microbiol. 70, 1516–1521. doi: 10.1099/ijsem.0.003884
Iriki, Y., Suzuki, T., Nisizawa, K., and Miwa, T. (1960). Xylan of siphonaceous green algae. Nature 187, 82–83. doi: 10.1038/187082a0
Kanagawa, T., Dazai, M., and Fukuoka, S. (1982). Degradation of O,O-dimethyl phosphorodithioate by Thiobacillus thioparus TK-1 and Pseudomonas AK-2. Agric. Biol. Chem. 46, 2571–2578.
Kimura, M. (1980). A simple method for estimating evolutionary rates of base substitutions through comparative studies of nucleotide sequences. J. Mol. Evol. 16, 111–120.
Kiyohara, M., Hama, Y., Yamaguchi, K., and Ito, M. (2006). Structure of beta-1,3-xylooligosaccharides generated from Caulerpa racemosa var. laete-virens beta-1,3-xylan by the action of beta-1,3-xylanase. J. Biochem. 140, 369–373. doi: 10.1093/jb/mvj173
Kiyohara, M., Sakaguchi, K., Yamaguchi, K., Araki, T., Nakamura, T., and Ito, M. (2005). Molecular cloning and characterization of a novel β-1,3-xylanase possessing two putative carbohydrate-binding modules from a marine bacterium Vibrio sp. strain AX-4. Biochem. J. 388, 949–957. doi: 10.1042/BJ20050190
Kumar, S., Stecher, G., Li, M., Knyaz, C., and Tamura, K. (2018). MEGA X: molecular evolutionary genetics analysis across computing platforms. Mol. Biol. Evol. 35, 1547–1549. doi: 10.1093/molbev/msy096
Lachnit, T., Meske, D., Wahl, M., Harder, T., and Schmitz, R. (2011). Epibacterial community patterns on marine macroalgae are host-specific but temporally variable. Environ. Microbiol. 13, 655–665. doi: 10.1111/j.1462-2920.2010.02371.x
Lahaye, M., Rondeau-Mouro, C., Deniaud, E., and Buléon, A. (2003). Solid-state 13C NMR spectroscopy studies of xylans in the cell wall of Palmaria palmata (L. Kuntze, Rhodophyta). Carbohydr. Res. 338, 1559–1569. doi: 10.1016/S0008-6215(03)00241-6
Li, C. Y., Wang, X. J., Chen, X. L., Sheng, Q., Zhang, S., Wang, P., et al. (2021). A novel ATP dependent dimethylsulfoniopropionate lyase in bacteria that releases dimethyl sulfide and acryloyl-CoA. eLife 10:e64045. doi: 10.7554/eLife.64045
Liang, W. S., Fang, T. Y., Lin, H. T., Liu, T. C., Lu, W. J., Tzou, W. S., et al. (2015). Expression, and characterization of Pseudomonas vesicularis MA103 beta-1,3-xylanase in Escherichia coli ClearColi BL21(DE3). Fish. Sci. 81, 1135–1143. doi: 10.1007/s12562-015-0933-0
Liang, Z., Liu, F., Wang, W., Zhang, P., Sun, X., Wang, F., et al. (2019). High-throughput sequencing revealed differences of microbial community structure and diversity between healthy and diseased Caulerpa lentillifera. BMC Microbiol. 19:225. doi: 10.1186/s12866-019-1605-5
Lombard, V., Golaconda Ramulu, H., Drula, E., Coutinho, P. M., and Henrissat, B. (2014). The carbohydrate-active enzymes database (CAZy) in 2013. Nucleic Acids Res. 42, D490–D495. doi: 10.1093/nar/gkt1178
Maeda, R., Ida, T., Ihara, H., and Sakamoto, T. (2012). Induction of apoptosis in MCF-7 cells by β-1,3-xylooligosaccharides prepared from Caulerpa lentillifera. Biosci. Biotechnol. Biochem. 76, 1032–1034. doi: 10.1271/bbb.120016
Martin, M., Barbeyron, T., Martin, R., Portetelle, D., Michel, G., and Vandenbol, M. (2015). The cultivable surface microbiota of the brown alga Ascophyllum nodosum is enriched in macroalgal-polysaccharide-degrading bacteria. Front. Microbiol. 6:1487. doi: 10.3389/fmicb.2015.01487
Miller, G. L., Blum, R., Glennon, W. E., and Burton, A. L. (1960). Measurement of carboxymethylcellulase activity. Anal. Biochem. 1, 127–132. doi: 10.1016/0003-2697(60)90004-X
Okazaki, F., Nakashima, N., Ogino, C., Tamaru, Y., and Kondo, A. (2013). Biochemical characterization of a thermostable β-1,3-xylanase from the hyperthermophilic eubacterium, Thermotoga neapolitana strain DSM 4359. Appl. Microbiol. Biotechnol. 97, 6749–6757. doi: 10.1007/s00253-012-4555-5
Qeshmi, F. I., Homaei, A., Fernandes, P., Hemmati, R., Dijkstra, B. W., and Khajeh, K. (2020). Xylanases from marine microorganisms: a brief overview on scope, sources, features and potential applications. Biochim. Biophys. Acta, Proteins Proteomics 1868:140312. doi: 10.1016/j.bbapap.2019.140312
Teeling, H., Fuchs, B. M., Becher, D., Klockow, C., Gardebrecht, A., Bennke, C. M., et al. (2012). Substrate-controlled succession of marine bacterioplankton populations induced by a phytoplankton bloom. Science 336, 608–611. doi: 10.1126/science.1218344
Umemoto, Y., Shibata, T., and Araki, T. (2012). D-xylose isomerase from a marine bacterium, Vibrio sp. strain XY-214, and D-xylulose production from β-1,3-xylan. Mar. Biotechnol. 14, 10–20. doi: 10.1007/s10126-011-9380-9
Usov, A. I., and Zelinsky, N. D. (2013). “Chemical structures of algal polysaccharides,” in Functional Ingredients From Algae for Foods and Nutraceuticals. ed. H. Dominguez (Cambridge: Elsevier), 23–86.
Veluraja, K., and Atkins, D. T. E. (1987). Helical structure of β(1-3) xylan and the water mediated hydrogen bonding schemes. Carbohydr. Polym. 7, 133–141. doi: 10.1016/0144-8617(87)90055-5
Wang, Q., Garrity, G. M., Tiedje, J. M., and Cole, J. R. (2007). Naive Bayesian classifier for rapid assignment of rRNA sequences into the new bacterial taxonomy. Appl. Environ. Microbiol. 73, 5261–5267. doi: 10.1128/AEM.00062-07
Williams, T. J., Wilkins, D., Long, E., Evans, F., DeMaere, M. Z., Raftery, M. J., et al. (2013). The role of planktonic Flavobacteria in processing algal organic matter in coastal East Antarctica revealed using metagenomics and metaproteomics. Environ. Microbiol. 15, 1302–1317. doi: 10.1111/1462-2920.12017
Yamaura, I., Matsumoto, T., Funatsu, M., and Mukai, E. (1990). Purification and some properties of endo-1,3-beta-D-xylanase from Pseudomonas sp. PT-5. Agric. Biol. Chem. 54, 921–926.
Keywords: marine bacteria, diversity, marine algae, 1,3-xylan, extracellular 1,3-xylanases
Citation: Sun H-N, Yu C-M, Fu H-H, Wang P, Fang Z-G, Zhang Y-Z, Chen X-L and Zhao F (2021) Diversity of Marine 1,3-Xylan-Utilizing Bacteria and Characters of Their Extracellular 1,3-Xylanases. Front. Microbiol. 12:721422. doi: 10.3389/fmicb.2021.721422
Received: 07 June 2021; Accepted: 26 August 2021;
Published: 01 October 2021.
Edited by:
Federico Baltar, University of Vienna, AustriaCopyright © 2021 Sun, Yu, Fu, Wang, Fang, Zhang, Chen and Zhao. This is an open-access article distributed under the terms of the Creative Commons Attribution License (CC BY). The use, distribution or reproduction in other forums is permitted, provided the original author(s) and the copyright owner(s) are credited and that the original publication in this journal is cited, in accordance with accepted academic practice. No use, distribution or reproduction is permitted which does not comply with these terms.
*Correspondence: Fang Zhao, emZhbmc5OTJAMTYzLmNvbQ==
Disclaimer: All claims expressed in this article are solely those of the authors and do not necessarily represent those of their affiliated organizations, or those of the publisher, the editors and the reviewers. Any product that may be evaluated in this article or claim that may be made by its manufacturer is not guaranteed or endorsed by the publisher.
Research integrity at Frontiers
Learn more about the work of our research integrity team to safeguard the quality of each article we publish.