- 1Clinical Microbiology Research Center, Ilam University of Medical Sciences, Ilam, Iran
- 2Department of Microbiology, School of Medicine, Tehran University of Medical Sciences, Tehran, Iran
- 3Infectious and Tropical Diseases Research Center, Health Research Institute, Ahvaz Jundishapur University of Medical Sciences, Ahvaz, Iran
- 4Department of Microbiology, School of Medicine, Ahvaz Jundishapur University of Medical Sciences, Ahvaz, Iran
- 5Laboratory Sciences Research Center, Golestan University of Medical Sciences, Gorgan, Iran
- 6Department of Microbiology, School of Medicine, Shahid Beheshti University of Medical Sciences, Tehran, Iran
- 7Department of Laboratory Sciences, School of Paramedical Sciences, Sabzevar University of Medical Sciences, Sabzevar, Iran
- 8Cellular and Molecular Research Center, Sabzevar University of Medical Sciences, Sabzevar, Iran
Multidrug-resistant (MDR) isolates of Mycobacterium tuberculosis (MTB) remain a primary global threat to the end of tuberculosis (TB) era. Delamanid (DLM) is a nitro-dihydro-imidazooxazole derivative utilized to treat MDR-TB. DLM has distinct mechanism of action, inhibiting methoxy- and keto-mycolic acid (MA) synthesis through the F420 coenzyme mycobacteria system and generating nitrous oxide. While DLM resistance among MTB strains is uncommon, there are increasing reports in Asia and Europe, and such resistance will prolong the treatment courses of patients infected with MDR-TB. In this review, we address the antimycobacterial properties of DLM, report the global prevalence of DLM resistance, discuss the synergism of DLM with other anti-TB drugs, and evaluate the documented clinical trials to provide new insights into the clinical use of this antibiotic.
Introduction
Notwithstanding the use of potent agents in varying combination treatment regimens, Mycobacterium tuberculosis (MTB) has an independent ability to resist antitubercular drugs (Li et al., 2019). A proper pharmacological regimen can help bacteriological and clinical treatments and inhibit the emergence and spread of resistance. However, limited treatment options make the management of multidrug-resistant tuberculosis (MDR-TB) and other chronic TB cases clinically challenging, as well as raise public health concerns. Likewise, the lack of sufficient active drugs during the intensive and continuation phases of treatment not only hinders the survival of the patients but also induces further resistance (Borisov et al., 2017; Heidary et al., 2019).
Drug progress in TB therapeutics has evolved considerably with the introduction of two new drugs, namely, bedaquiline (BDQ) and delamanid (DLM). The European Medicines Agency conditionally approved DLM as a first-in-class bicyclic nitroimidazole, relying on promising results from phase IIb trial and with regard to medical need for treating MDR-TB (Blair and Scott, 2015; Khoshnood et al., 2021).
Based on the 2017 WHO Global TB Report, using BDQ and DLM was initiated in 89 and 54 countries, respectively (Cox et al., 2018). The rapid acquisition of resistance by DLM highlights the demand for the proper use of such new drugs and medication, and emphasizes the significance of drug resistance surveillance. Combination therapy with DLM and other active anti-TB agents is suggested for the prevention of acquired resistance (Diel et al., 2015).
This review aims to discuss the synergism of DLM with other antimycobacterial agents, to summarize the available evidence on DLM resistance among drug-resistant MTB isolates, and to evaluate the clinical use of this drug, in order to provide new insights into this phenomenon.
Antimicrobial Properties
Structure of Drug
DLM, developed by Otsuka Pharmaceutical Co., Ltd. (Tokyo, Japan), belongs to a class of bicyclic nitroimidazole (Saliu et al., 2007; World Health Organization, 2020). It is one of the two new anti-TB drugs globally approved in the past 40 years (Ryan and Lo, 2014; von Groote-Bidlingmaier et al., 2019). Bicyclic nitroimidazooxazole, an analog of azomycin known as 2-nitro-imidazole, is isolated from Streptomyces eurocidicus and has potent in vitro and in vivo anti-TB activity (Igarashi, 2017). Substituents in the 2-position of 6-nitro-2,3-dihydroimidazo[2,1-b]oxazole accelerated anti-TB activity and declined mutagenicity. However, substitution in the 2-position of the side chain with a heteroatom eliminated mutagenicity (Figure 1). The 6-nitro-2,3-dihydroimidazo[2,1-b]oxazole is a racemic mixture so that right-handed, but not left-handed, enantiomers have activity against Mycobacterium tuberculosis complex (MTBC). The 6-nitro-2,3-dihydro-imidazooxazol (C25H25F3N4O6), commercially named Deltyba, was introduced as a safe compound with in vivo and in vitro optimal performance (Sasaki et al., 2006; Matsumoto et al., 2007; Mukherjee and Boshoff, 2011; Tsubouchi et al., 2016).
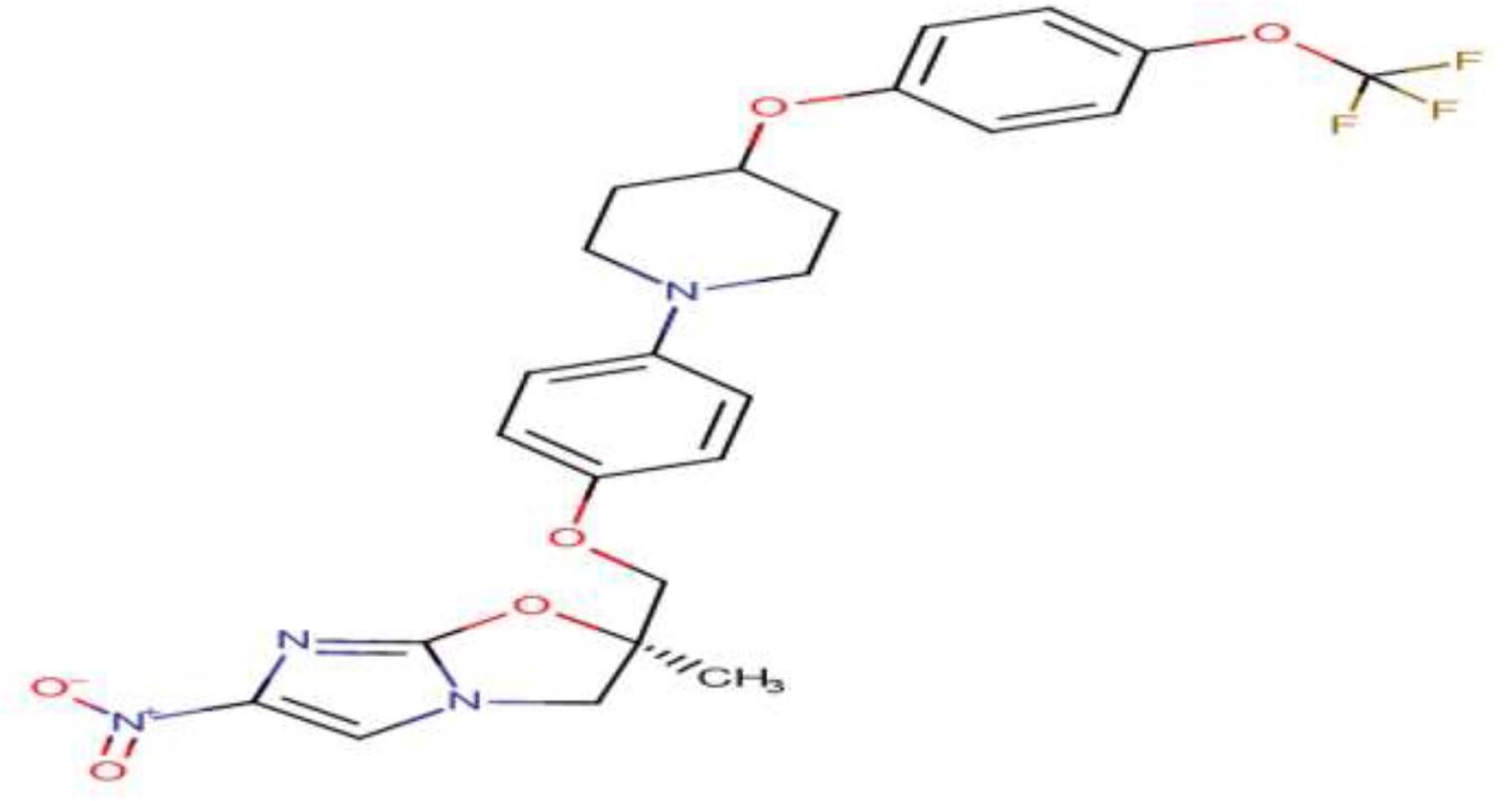
Figure 1. Chemical structure of delamanid (Szumowski and Lynch, 2015).
Mechanism of Action
Delamanid (DLM) is a prodrug that confers mycobactericidal activity by inhibiting the synthesis of methoxy and keto MA through the mycobacteria F420 system and generating nitrous oxide (Singh et al., 2008; Vilchèze, 2020). Both DLM and isoniazid (INH) act by preventing the synthesis of MA, which plays a pivotal role in the survival of mycobacterial genus. Compared with DLM, INH, as the inhibitor of α-MA synthesis, has a different strategy for impeding the cell wall synthesis (Lewis and Sloan, 2015). Enoyl-acyl carrier protein reductase, InhA, is a specific factor for the function of INH, while DLM needs mycobacterial F420 system for its activation (Parikh et al., 2000; Deane and Porkess, 2018). This system is the analog of flavin mononucleotide complex and composed of two enzymes, deazaflavin-dependent nitroreductase (Ddn, Rv3547) and F420-dependent glucose-6-phosphate dehydrogenase (G6PD; FGD1, Rv0407), as well as four coenzymes, FbiA (Rv3361), FbiB (Rv3261), FbiC (Rv1173), and Rv0132c (Bashiri et al., 2010; Hartkoorn et al., 2014). All of these genes and coenzymes are involved in the synthesis and recycling of cofactor F-420. As depicted in Figure 2, DLM has undergone the influence of the Ddn enzyme for converting into its active and inactive forms, an unknown reactive intermediate metabolite that is active against MTB and a desnitro (inactive) form, respectively. The main function of DLM in preventing MA biosynthesis is attributed to the reactive intermediate metabolite. The removal of this major compound from the Mycobacterium cell wall leads to the destruction of this bacterium. G6PD is also responsible for returning the F420 to the reduced form (Gurumurthy et al., 2012; Greening et al., 2016; Schuster Bruce et al., 2019).
Spectrum of Activity
Activity Against Mycobacterium Tuberculosis
Delamanid (DLM) has in vitro potential activity against standard, clinical susceptible and resistant strains of MDR-TB and also against extensive drug-resistant tuberculosis (XDR-TB) (Stinson et al., 2016). This drug is a narrow-spectrum antibiotic able to eliminate only MTBC, and its activity against non-tuberculosis mycobacteria (NTM) is unknown. Unpublished data from an earlier research has portrayed that DLM has no activity against aerobic and anaerobic intestinal microflora (Liu et al., 2018). However, another survey reflected that DLM affects the visceral leishmaniasis caused by Leishmania donovani (Patterson et al., 2016). Due to the lack of cross-resistance and antagonistic activity with other drugs, including INH, rifampin (RIF), and ethambutol (ETB), DLM has been suggested to treat TB (Parikh et al., 2000; Lewis and Sloan, 2015). Based on the fractional inhibitory concentration index (FICI), the aforesaid drugs are classified as synergistic or partially synergistic for tested strains (Matsumoto et al., 2006).
Delamanid (DLM) shows dose-dependent bactericidal activity in murine and guinea pig models infected by MTB (Chen et al., 2017). Similar to RIF (3 μg/ml), DLM (0.1 μg/ml at 4 h) has a dose-dependent activity against intracellular mycobacteria in human macrophage. The required dose of DLM to reduce 95% of colony-forming unit (CFU) was 0.625 mg/kg. This dose is 5.6 to 256 times less than the dose needed for first-line drugs, such as RIF, INH, and ETB, used in the treatment of MTB (Matsumoto et al., 2006). Early bactericidal activity (EBA) of DLM (in four doses: 100, 200, 300, and 400 mg daily) was assessed by Diacon et al. in 48 patients with pulmonary TB. The reported mean of the EBA of DLM in all doses was relatively low (0.04 log10 CFU/m). The pharmacokinetic (PK) assay in that study revealed that exposure to DLM did not increase in proportion to the drug dose and plateaued at a dosage of 300 mg (Diacon et al., 2011). Stinson and associates reported a very low minimum inhibitory concentration (MIC; 0.001–0.05 μg/ml) of DLM against the clinical and reference strains of MTB (Stinson et al., 2016). However, MTB mutant strains with a mutation in each of the five genes of the F420-dependent pathway developed resistance against DLM (MIC > 8 μg/ml) (Fujiwara et al., 2018; Jing et al., 2019; Polsfuss et al., 2019). Delamanid is accumulated in the cell and has pharmacological potential activity against the replicating, dormant, and intra- and extracellular bacilli (Chen et al., 2017). Gler and colleagues reported the results of the phase II trial (trial 204) on 481 patients infected with pulmonary MDR-TB. The patients received the optimized background regimens (OBR) plus 100 mg (161 patients), 200 mg (160 patients), or placebo (160 patients) of DLM twice daily for 8 weeks. Sputum-culture conversion (SCC) was defined as a series of five or more sequential cultures negative for MTB. Accordingly, DLM was accompanied by an increase in SCC at 2 months among patients infected with MDR-TB. This finding emphasizes DLM as a treatment option for MDR-TB (Gler et al., 2012). In another clinical trial study (trial 208) reported by Skripconoka et al., treatment with DLM at two doses (100 and 200 mg twice daily) was continued in 421 patients for 6 months. In that study, 192/421 patients received DLM in combination with an OBR for ≥6 months, compared with 229/421 cases that received DLM for ≤2 months. In both trial studies (trials 204 and 208), sputum smear was negative in patients receiving DLM in comparison with patients receiving placebo or an OBR. However, the mortality rate decreased to 1% in 74.5% of patients who received DLM for 6 months, while this rate reduced by 8.3% in those who received the drug for 2 months. A favorable outcome was ultimately observed in patients with XDR-TB who consumed DLM (Skripconoka et al., 2013).
A former study inconsistently explored no association of DLM with an increase in SCC among patients infected with MDR-TB over 6 months. That research reported the results of phase three trial of DLM treatment in patients with pulmonary TB who received an OBR in Europe, Asia, Africa, and South America. Moreover, patients with MDR-TB were treated with either oral DLM at a dose of 100 mg twice daily for 2 months, followed by 200 mg once daily for 4 months (226 patients) or placebo with the same regimen (101 patients). Results of the same study showed that the difference in the median time for SCC between the two groups was insignificant during 6 months (von Groote-Bidlingmaier et al., 2019). Contradictory outcomes in the abovementioned surveys necessitate further investigation of DLM to determine its efficacy in SCC of MDR-TB (Szumowski and Lynch, 2015).
Tuberculosis (TB) is caused by various MTBC members such as MTB sensu stricto and M. africanum, as the main causes of TB in humans (Senghore et al., 2020). MAs, long-chain α-alkyl, and β-hydroxy fatty acids vary in size and structure and are indisputably necessary for the survival of MTBC arising in the cell envelope (Yimer et al., 2020). Although the lipidomics of MAs is very different among MTBC strains, the biosynthesis pathway of these fatty acids is equal (Portevin et al., 2014; Yimer et al., 2020). Mutations in the genes of the F420 signaling pathway of the MTBC, including dnn, fgd1, fbiA, fbiB, fbiC, and fbiD, can lead to DLM resistance (Reichmuth et al., 2020). Delamanid, as a broadly used anti-TB agent, exerted a greater in vitro antibacterial activity than other drugs, such as pretomanid (PTM), against MDR- and XDR-TB strains (Jing et al., 2019).
Delamanid (DLM) normally exists as a prodrug and is activated by a nitroreductase encoded by deazaflavin (F420)-dependent nitroreductase (ddn) gene in MTBC. This agent is cleaved into two reactive nitrogen species, including nitric oxide and nitrous acid with dual anti-TB effects via the disruption of the MA biosynthesis pathway, which is essential to the growth, survival, and disruption of the respiratory activity (Reichmuth et al., 2020).
The anti-TB activity of DLM has been examined in the cell culture system, wherein the intracellular activity against MTB is the main objective. The killing efficiency of DLM was similar to RIF, a reliable key antibiotic for intracellular MTB (Szumowski and Lynch, 2015). DLM is able to substantially inhibit the biosynthesis of cell wall during the disruption of the MA biosynthesis pathway, eventually giving rise to the elimination of MTB (Rustomjee and Zumla, 2015). Preclinical results have signified that DLM is effective against growing or dormant bacilli, both in vitro and in vivo. M. bovis is a member of MTBC cultured under aerobic and anaerobic conditions to evaluate its killing capability in growing and dormant phenotypes. The in vitro results uncovered that M. bovis, the same as MTB, is susceptible to 0.016 mg/L of DLM in a growing state, and the dormant phenotype is more resistant to DLM (0.04 mg/L) (Szumowski and Lynch, 2015). In a guinea pig model of TB, the effective administration dose of DLM daily was 100 mg/kg to kill MTB within hypoxic lesions of the lungs (Chen et al., 2017). There were also several resistance-conferring mutations in ddn gene found in MTB and M. bovis, and DLM activation was abrogated during ddn mutation (Reichmuth et al., 2020).
Activity Against Non-tuberculous Mycobacteria
Despite the fact that MTB is the main cause of pulmonary disease (PD), the incidence and mortality of non-tuberculous mycobacterial pulmonary disease (NTM-PD) are escalating worldwide (Kim et al., 2019). The most common pathogens for NTM-PD are Mycobacterium avium (M. avium) complex (MAC), Mycobacterium abscessus, and Mycobacterium kansasii (Koh, 2017). According to a report by Krieger et al., DLM showed a wide variation in MIC against MAC and Mycobacterium intracellulare (0.013 to 0.4 μg ml–1). This result is the first evidence showing that DLM is a potential agent for the treatment of diseases caused by M. avium and M. intracellulare (Krieger et al., 2016).
The MIC of DLM against NTM is very variable. Soni et al. implied that the MICs of DLM against NTM were greatly different (0.1 to > 100 μg ml–1), and DLM indicated a higher MIC against only M. kansasii (0.1 μg mL–1) compared with other tested NTMs (Koh, 2017). Kim et al., however, denoted that MTB had high MIC values against NTM, except for M. kansasii (Kim et al., 2019). Recently, Yu et al. have confirmed the highly variable antimicrobial activity of DLM against NTM and reported that DLM has a high MIC against slowly growing Mycobacterium species of NTM, i.e., M. avium and M. kansasii (Yu et al., 2019).
Delamanid Resistance
Mechanisms of Resistance
Drug-resistant TB is globally a challenge for the TB control program performance. As predicted by mathematical modeling, the incidence of MDR- and XDR-TB will raise dramatically in the forthcoming decades (Koch et al., 2018). In this situation, emerging resistance to new drugs can create more complicated conditions for the treatment of TB (Nguyen et al., 2020). Therefore, the comprehension of fundamental mechanisms of drug resistance is essential to develop strategies for the optimal consumption of new effective drugs.
Resistance to DLM can be induced by non-synonymous mutations in five genes (ddn, fbiA, fbiB, fbiC, and fgd1) whose products are main proteins and coenzymes for the biosynthesis and modification of F420 (Figure 2). The F420-dependent nitroreductase coded by ddn gene activates F420. The mentioned enzyme can convert bicyclic nitroimidazole drugs to intermediate metabolites and desnitro form of DLM. FbiA gene encodes a transferase that catalyzes the transfer of a phosphoenolpyruvate moiety to F420-0.
FbiB, however, encodes a γ-glutamyl ligase that is involved in the conversion of dehydro-F420-0 to form F420-0 and then catalyzes the GTP-dependent successive addition of multiple gamma-linked L-glutamates to F420-0. FbiC encodes a F420-0 synthase that catalyzes the formation of the F420 precursor F420-0 (Fujiwara et al., 2018; Rifat et al., 2020). Another recently cloned gene involved in the biosynthetic pathway for the cofactor F420 is fbiD (Rv2983), which synthesizes the phosphoenolpyruvate moiety for subsequent steps done by fbiA (Bashiri et al., 2019). Ultimately, G6PD coded by fgd1 gene is necessary for the redox re-cycle of F420 and catalyzes the oxidation of glucose-6-phosphate to 6-phosphogluconolactone using coenzyme F420 as an electron acceptor (Fujiwara et al., 2018; Hameed et al., 2018).
Thus, any mutations in this pathway result in the reduction of Mycobacterium bacilli to metabolize prodrug and low- to high-level DLM resistance. The prompt development of resistance to DLM highlights the necessity for the optimal employment of new drugs, stringent stewardship for drug resistance surveillance, and thorough awareness of drug resistance mechanisms. Therefore, supplementary studies can help understand the genetic and phenotypic changes attributed to clinically relevant DLM resistance, in order to establish rapid drug susceptibility testing methods (Li et al., 2019).
Epidemiology of Resistance
A few studies have implied the DLM resistance in MTB isolates (Table 1). The spontaneous frequency of DLM resistance has been reported as 6.44 × 10–6 to 4.19 × 10–5, highlighting the importance of this drug in combination with other active anti-TB drugs during therapy (Szumowski and Lynch, 2015). A systematic review of mutations related to anti-TB drug resistance has stated 12 distinct mutations in the genes fbiA, fbiC, ddn, and fgd1 associated with DLM resistance. The most frequent gene mutation investigated in the literature was ddn (Rv3547) W88, which increases MIC > 200 times (Kadura et al., 2020). Fujiwara et al. disclosed that mutations in each of these five genes led to the low metabolizing potency of M. bovis BCG Tokyo and MTB H37Rv mutants in vitro.
They estimated that the in vitro spontaneous resistance frequencies for DLM ranged from 4.19 × 10–5 to 6.44 × 10–6 for MTB H37Rv and from 2.51 × 10–5 to 3.95 × 10–5 for M. bovis BCG Tokyo. This frequency of mutation is similar to INH and PTM mutation rates in laboratory conditions (Fujiwara et al., 2018). In another study, genotypic analysis revealed 11 new genetic polymorphisms in resistant strains compared with isolates recovered before starting DLM therapy. The two mutations in fbiA (D49Y and R175H) coincided with the development of phenotypic resistance to this new drug (Hoffmann et al., 2016).
Schena et al. evaluated DLM susceptibility testing of 194 MTB strains recovered from patients. By using resazurin microtiter assay (REMA), they identified four resistant isolates with MIC values of >32.0 mg/L. The genetic analysis of DLM resistance phenotypes illustrated four stop codon mutations in the ddn (Trp-88 → STOP) and fbiA (Lys-250→STOP) genes, leading to high MIC values in these strains (Schena et al., 2016). The finding of an in vitro drug susceptibility assessment of 90 clinical XDR MTB isolates in China indicated that four (4.4%) isolates increased MIC for DLM, and codon 318 mutation of fbiC gene was recognized as a novel mutation contributed to DLM resistance. The nucleotide sequence analysis of the other four genes related to DLM resistance showed no mutation in ddn, fgd1, fbiA, and fbiB genes. It seems that mutations in fbiC gene have a considerable role in DLM resistance, though further resistance mechanisms could not be neglected (Pang et al., 2017).
In 2018, Yang et al. investigated the MIC distributions of DLM in 420 clinical MTB strains in Korea. Considering the critical concentration of 0.2 mg/L, DLM resistance phenotypes were found in 41 strains (9.76%). Moreover, 31 out of 41 resistant strains harbored mutations in fbiA or ddn genes, and two non-silent mutations (Gly81Ser and Gly81Asp) were identified in ddn gene among the resistant strains. Following the observation of the Gly81Ser mutation in high frequency (75.6% in resistant strains and 81% in susceptible strains), the same authors interpreted that the importance of this mutation in DLM resistance is unclear (Yang et al., 2018).
In another evaluation of the in vitro activity of DLM accomplished by Jing et al. in China, among 220 clinical strains with no previous exposure to DLM, 3.18% of isolates were resistant. They attributed the detected unprecedented mutation in the fbiA gene (Glu249Lys) to high-level resistance to DLM and PTM in MTB, though no report of cross-resistance between DLM and PTM has been recorded to date (Jing et al., 2019).
In a multicenter study conducted from 2010 to 2019 in Korea, 633 patients with MDR-TB were analyzed. According to the results of MICs for DLM, three (11.5%) patients were considered resistant with interim critical concentrations of 0.016 mg/L. Among the three DLM patients resistant to TB, one had never been administrated anti-TB drugs in the past, and the remaining two patients had a history of only first-line anti-TB drug treatments (Lee et al., 2021). In a randomized phase III trial carried out in seven countries, 341 patients with MDR-TB received DLM-containing regimens for 6 months (DLM group), and 170 participants received placebo plus an OBR (placebo group). The rates of DLM baseline resistance and acquired DLM resistance in the DLM group were 0.39% (2/511 patients) and 1.17% (4/341 patients), respectively. However, no resistance to DLM was observed in the placebo group (von Groote-Bidlingmaier et al., 2019).
It is important to note that the addition of DLM to the therapeutic regimen of MDR- and XDR-TB patients should be based on individual evaluation, including drug susceptibility information, safety and tolerability of drug, and assessment of risk–benefit ratio. Additionally, the clinical administration of DLM to patients needs to be concomitant with the establishment of a systematic and consistent drug susceptibility testing, in order to recognize the primary emergence of drug resistance and to prevent the transition of resistant isolates (Stinson et al., 2016).
Synergism of Delamanid With Other Antituberculosis Drugs
Despite the use of a wide variety of potent antibiotics for TB, an effective treatment is a serious challenge in the TB-affected patients (Heidary and Nasiri, 2016). Thus, the synergistic combination effect of drugs is regarded as an innovative approach to control TB infections (Ramón-García et al., 2011). The new anti-TB drug, DLM, is used to treat MDR- and XDR-TB. Several studies assessed the synergistic combination effect of DLM and BDQ and reached the conclusion that these drugs have a synergistic effect on INH- and RIF-monoresistant and also XDR-MTB clinical isolates (Ferlazzo et al., 2018; Pontali et al., 2018; Chandramohan et al., 2019; Olayanju et al., 2020).
The REMA suggested that the MICs (μg/ml) of DLM were 0.063 (alone) and 0.015 (combination) for INH-monoresistant, 0.063 (alone) and 0.015 (combination) for RIF-monoresistant, and 0.125 (alone) and 0.015 (combination) for XDR isolates. However, the MICs of BDQ were 0.25 (alone) and 0.015 (combination) for INH-monoresistant, 0.125 (alone) and 0.015 (combination) for RIF-monoresistant, and 0.063 (alone) and 0.015 (combination) for XDR isolates. Fractional inhibitory concentration indices (FICI) was also calculated using REMA-based calorimetric checkerboard assay (FICI ≤ 0.5 defined as synergy) to evaluate the synergistic effect of DLM. The obtained FICI for INH-monoresistant, RIF-monoresistant, and XDR isolates were 0.31, 0.30, and 0.37, respectively (Chandramohan et al., 2019).
In a prospective study, the effects of a single-drug regimen (BDQ) were compared with those of combination-drug regimen (BDQ-DLM) in South African patients with drug-resistant TB. The results revealed that the combination group possessed high prolongation of the QTcF (> 60 ms from baseline or >450 ms during treatment), but the long-term safety was greater for the combination than for the single-drug regimen (Olayanju et al., 2020). In this respect, the WHO recommended clinicians for using these antibiotics under specific conditions and not in combination, owing to their high risk for cardiotoxicity. In exceptional circumstances, clinicians may be obligated to combine these drugs; for instance, when the number of effective drugs (at least four drugs for an efficient regimen or in cases with few treatment options) is insufficient, as well as in difficult-to-treat cases or in the emergence of excessive XDR-TB resistance.
Ferlazzo et al., in a retrospective cohort study, described the early safety and efficacy of BDQ-DLM combination regimen, i.e., 100 mg of DLM twice a day and BDQ at a dose of 400 mg once a day for 2 weeks, followed by 200 mg of BDQ three times a week. That study was performed on 28 patients with drug-resistant TB in South Africa, Armenia, and India. Ferlazzo and associates observed no additive or synergistic QTc prolongation effect on BDQ-DLM regimen. It should be noted that no published data has yet reported the combined use of these drugs in children (Matteelli et al., 2015; Migliori et al., 2017; Ferlazzo et al., 2018; Pontali et al., 2018). In another retrospective cohort study conducted in Mumbai, India, treatment outcomes on 49 (70%) patients who received BDQ-DLM combination regimen for 24 weeks were represented to be successful. The majority (69%) of patients showed culture conversion, and effective end-of-treatment results were reported in 49 (70%) patients (Das et al., 2020a).
An in vitro and in vivo study of DLM exhibited low MICs (in the range of 0.006–0.024 μg/ml) and low doses for an effective therapeutic activity on MDR-TB. In addition, DLM was more effective in intracellular MTB compared with RIF (at the concentrations of 3 and 0.1 μg/ml, respectively). In another investigation, a combination regimen, comprising of DLM (2.5 mg/kg), RIF (5 mg/kg), and pyrazinamide (PYR; 100 mg/kg), known as synergistic combination drugs, was employed for the treatment of mice (n = 6) (Matsumoto et al., 2006).
The results of that study indicated faster eradication (by at least 2 months) and shorter duration of clinical treatment of viable TB in the lungs of murine compared with the standard regimen, i.e., RIF (5 mg/kg), INH (10 mg/kg), ETB (100 mg/kg), and PYR (100 mg/kg) (Matsumoto et al., 2006). Given that new cephalosporins possess a broad spectrum of antibacterial activities, it is likely that these antibiotics have high efficiency for TB therapy. In this respect, in vitro synergy assays have suggested strong synergistic interaction between β-lactams (cephradine and faropenem) and DLM (FICI of ≤0.5) for MDR- and XDR-TB, which might possibly be effectual in reducing treatment length. Hence, an in vivo study could validate the synergistic effect of DLM on β-lactams (Ramón-García et al., 2016).
Since the substitution of moxifloxacin for the first-line drug regimen may be more effective in MDR-TB and DLM has a substantial impact on MDR- and XDR-TB, a combination of these drugs could serve as an effective synergistic combinatorial therapy. In this context, Chandramohan et al. conducted a study focusing on the in vitro synergistic effect of DLM on moxifloxacin against drug-resistant clinical MTB isolates (INH- and RIF-monoresistant, MDR, Pre-XDR, and XDR-MTB. The MICs of the drug were evaluated by the REMA, and REMA-based calorimetric checkerboard assay was carried out to assess the FICIs (FICI ≤ 0.5 defined as synergy). The synergistic effect was exhibited against INH- and RIF-monoresistant and XDR isolates with FICI of 0.5, 0.5, and 0.24, respectively (Chandramohan et al., 2019).
The MICs (μg/ml) of DLM and BDQ were, respectively, 0.063 and 0.25 for INH-monoresistant, 0.063 and 0.125 for RIF-monoresistant, and 0.125 and 0.063 for XDR isolates, when used alone, while the MIC of the two drugs in combination was 0.015 for all the isolates. However, WHO reported that the combination of moxifloxacin and DLM might increase the risk of QT prolongation (World Health Organization, 2016). Additionally, owing to the high rate of moxifloxacin resistance, using a combination of these drugs for treating XDR-TB was inappropriate in clinical settings. Thus, an in vivo study is highly needed to validate the toxicity, efficacy, and safety of these drugs (Chandramohan et al., 2019).
There is an abundant room for future progress in determining the particular characteristics of pharmacokinetics and drug safety of new regimen, including DLM, for vulnerable populations such as pregnant women, children, and patients with HIV (Lienhardt et al., 2020). In 2016, the WHO issued an interim guideline for administrating DLM in 6- to 17-year-old children. However, evidence on the safety and efficacy of DLM treatment in children is limited. One of the largest cohorts of children and adolescents treated with new anti-TB regimens was carried out in India. For 24 patients (aged 0–19 years) with fluoroquinolone (FQ)-resistant TB, a treatment regimen, including DLM and BDQ in combination and separately, was utilized between September 2014 and June 2018. The results of final treatment outcomes and culture conversion showed that the treatment was successful in 96% of patients, and only 2/12 cases had side effects during treatment with the new TB drugs (Das et al., 2020b).
In a study conducted by Pieterman et al. (2021), the synergistic effect of combination regimen (DLM (2.5 mg/kg), BDQ (25 mg/kg), and linezolid (100 mg/kg) was compared with the standard regimen (ETB, PYR, RIF, and INH) in TB-infected mice. Their results confirmed that the combination regimen is more effective than the standard regimen. One reason for this superiority was culture negativity in the lungs, which was observed at 8 weeks in combination regimen vs. 20 weeks in the standard regimen. Another reason was that in the combination regimen, relapse was found only in one mouse, while in the standard regimen, it was continued at 24 weeks. Consequently, in view of very limited clinical studies, further investigations are necessary to determine the synergistic combination effect of DLM on other drugs, and more in vivo surveys are required to corroborate the synergistic effect of DLM and to determine their toxicity, efficacy, and safety.
Pharmacokinetics and Pharmacodynamics
After catalyzing to the human-unique metabolite M, which is formed by the cleavage of the 6-nitro-2,3-dihydroimidazo[2,1-b]oxazole moiety, DLM was metabolized by three individual pathways. Hydroxylation of the oxazole moiety of M1, the most crucial starting point, to form M2, and also consecutive oxidation to the ketone form (M3), are two main pathways in humans that are mostly performed by CYP3A4 (Figure 3) (Sasahara et al., 2015).
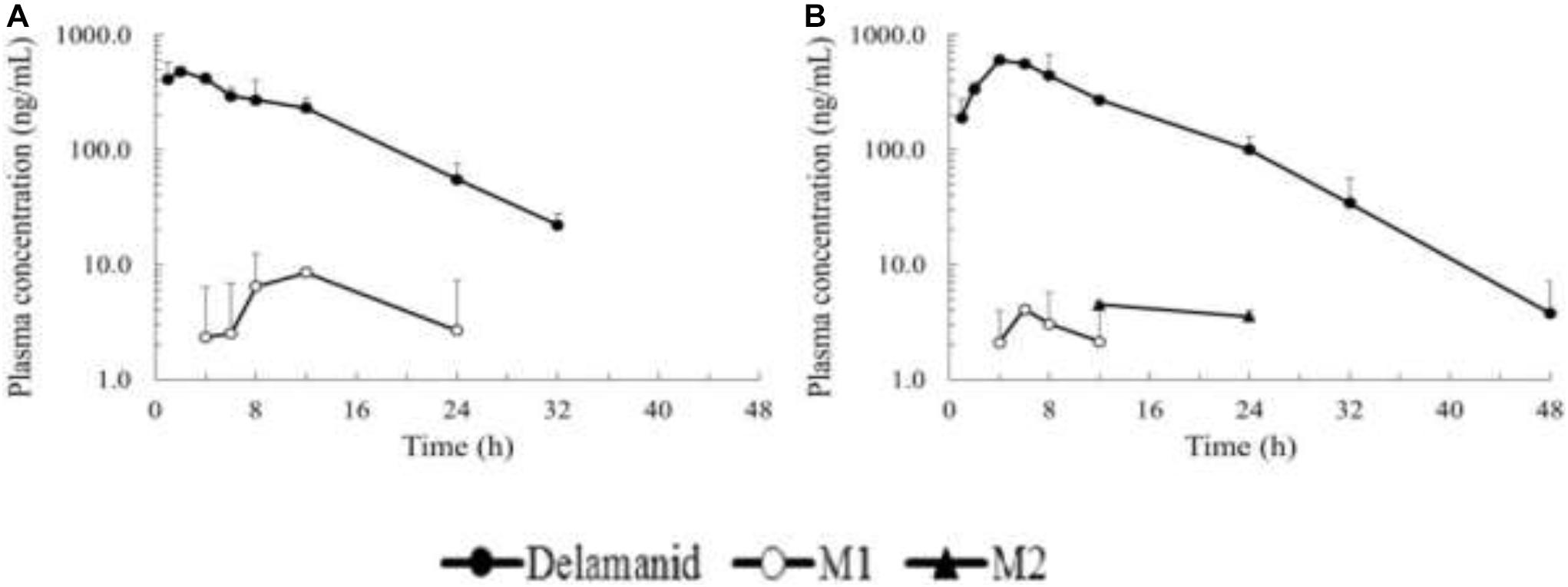
Figure 3. Plasma concentration time profiles of delamanid single oral dose and M1/M2 metabolites in mice (A) and rats (B).
For anti-TB compounds, there are various mechanisms of interindividual PK variability and drug–drug interactions. One of these mechanisms is the induction of metabolism or its inhibition through hepatic cytochrome p450 enzymes. Another mechanism includes drug transportation by P-glycoprotein, a significant efflux transporter that affects intracellular pharmacokinetics by transporting foreign substances out of the cells. In preclinical surveys, DLM is recognized as highly protein bound (> 97%), and its metabolism is mainly mediated by plasma albumin. However, the hepatic CYP enzymes, particularly CYP3A4, CYP1A1, CYP2D6, and CYP2E1, likely show a lesser extent. In animal (e.g., dog, rat, and mouse) models, DLM has been explored to have an oral bioavailability of 35%–60% (Miyamoto et al., 2005; Lewis and Sloan, 2015).
Using fresh human hepatocytes and human liver microsomes, in vitro investigations studied the effect of DLM on human cytochrome p450 enzymes. DLM did not show any effect on CYP1A1/2, CYP2A6, CYP2B6, CYP2C8/9, CYP2C19, CYP2D6, CYP2E1, and CYP3A4 at concentrations <100 μmol/L (Matsumoto et al., 2006). In healthy volunteers, an interaction was observed between DLM and the strong CYP3A4 enzyme inducer, RIF, which diminished exposure to DLM by 47%. This reductive behavior could be the main reason that the coadministration of DLM with the mentioned CYP enzyme inducers was contraindicated by the European Medicines Agency. In vitro evidence revealed that clinically relevant interactions of DLM with drugs, particularly those whose disposition is dependent on ATP-binding cassette (ABC) and solute carrier transporters, breast cancer-resistant proteins (BCRP/ABCG2), organic anion-transporting polypeptides, or organic cation transporter 1, are impossible (Hu et al., 2016).
The complete oral bioavailability of DLM has not yet been measured; however, it seems to be between 25 and 47%. During dose-escalation studies, administration of higher oral doses was associated with a less than proportional increase in plasma exposure. Exposure to DLM, unlike a number of first-line anti-TB drugs (especially RIF), was enhanced by food, i.e., high-fat meal in particular. Exposure in a fed state is almost three times higher than that of fasted state. In combination regimens, varied absorption profiles between drugs may make coadministration complicated. DLM, a high percentage (> 99%) protein bound, has an exceptional apparent volume of distribution (Oye et al., 2013; Chang and Sotgiu, 2017).
The elimination of DLM is performed directly from plasma, but not urine, with a half-life of between 30 and 38 h. Its metabolization is also thought to be carried out, to a great extent, by plasma albumin. Although the full metabolic profile of DLM is unknown, this drug is seemingly converted to its primary metabolite, DM-6705, following the reaction of amino groups in serum albumin to this agent. Thereafter, the DM-6705 is broken down by hydrolysis and CYP3A4 and converted to some other metabolites, which concentration raises to steady state during 6–10 weeks. These metabolites often show weak anti-TB activity, whereas other metabolites may indicate DLM-related toxicity. For instance, the DM-6705 metabolite has a key function in the prolongation of QTc. However, the metabolic pathway of DLM appeals for further investigation to fully be elucidated (Oye et al., 2013; Lewis and Sloan, 2015).
Adverse Events
Apart from many beneficial impacts of DLM on MDR- and XDR-TB, the side effects of its use have been reported in some patients. Research of genotoxicity and carcinogenic potential indicates no serious impact on human and animals (Lewis and Sloan, 2015; Hanaki et al., 2017). Although the concern about hepatotoxicity of DLM is not as much as other anti-TB drugs such as RIF, INH, and PYR, it has been suggested that DLM may rarely have the potential effects on the liver.
Recent surveys have demonstrated the potential of DLM and/or its metabolites on cardiac repolarization, which resulted in increased QTcF prolongation (Lewis and Sloan, 2015). Because of QTc-prolonging effects on several second-line MDR-TB drugs, including FQs, co-administration of clofazimine and BDQ with DLM increases the concerns about cardiac arrhythmias (Matsumoto et al., 2006). It is speculated that the increased QTc prolongation is associated with the main DLM metabolite DM-6705 (Deltyba Epar Product Information, 2014). It has also been recommended that in patients with hypoalbuminemia (level\2.8 g/dL), DLM therapy should not be administrated due to the associated increased risk of QTc prolongation (Ryan and Lo, 2014). Other frequent drug adverse events, including gastrointestinal problems (vomiting, decreased appetite, gastritis, and nausea), tremor, asthenia, and hypokalemia, were reported in patients who received DLM (Chang and Sotgiu, 2017). In a study conducted in Addis Ababa from 2017 to 2019, 51 drug-resistant TB patients were registered. Thirty (58.8%) patients developed side effects, the most common of which were gastrointestinal and hematological disorders and QTc prolongation. Also, 20 patients permanently discontinued the offending drug (Tesema et al., 2021).
In a multinational phase II trial, 204 patients received an OBR combined with DLM or placebo for 2 months. A comparison between the DLM and placebo groups showed that the prevalence of QTc interval prolongation in patients who received DLM was significantly higher than that in the placebo group. However, no clinical symptoms, such as arrhythmias or syncope, were observed in patients with the episodes of QT interval prolongation (Gler et al., 2012). More investigation was performed for patients who completed trial 204, and DLM was administrated twice daily together with an OBR for an additional 24-month period. In that observational study, serious adverse events happened in 11.7% of the DLM-treated group, and the most prevalent adverse events reported were anemia, hemoptysis, QTc interval prolongation, and psychotic disorder (Skripconoka et al., 2013).
The Korea Centers for Disease Control and Prevention Agency reviewed 318 patients who were administrated with BDQ and/or DLM for 6 months, to evaluate treatment outcomes. They reported two patients with QTcF prolongation (QTcF interval 506 and 511 ms) and five cases with gastrointestinal problems during treatment with DLM. The physician decided to permanently discontinue DLM due to adverse events for all seven patients (Kang et al., 2020). A pediatric clinical trial determined the safety and pharmacokinetics of DLM for patients with MDR-TB. Among 19 enrolled patients, a 17-year-old patient with XDR-TB had experienced renal impairment, severe vomiting, and severe electrolyte disorders, and subsequently QTcF prolongation. After temporary discontinuation of treatment and the management of electrolyte imbalance and vomiting, the therapy was re-established (Tadolini et al., 2016). In another retrospective cohort study in South Korea, the safety and tolerability of DLM in 32 enrolled patients were assessed. Three patients exhibited increased QTcF prolongation (QTcF interval of >500 ms) during treatment with DLM. Therapy with this drug re-initiated at 7–10 days after the transient discontinuation of treatment (Mok et al., 2018).
Lee et al. published an analysis of medical records of 129 patients who treated for MDR-TB from January 2005 through December 2017 in South Korea. Regardless of the most frequent side effects related to Linezolid, an increased QTcF prolongation was observed in one patient with FQ-sensitive MDR-TB during treatment with DLM (Lee et al., 2019). Hughes et al. followed up 58 patients with RIF-resistant TB to determine the adverse effects of DLM treatment program in South Africa. The most common side effects related to DLM consumption were vomiting, QTcB > 450 ms, and myalgia. Moreover, the gradually worsening QT interval prolongation and cardiac symptoms, including chest pain, dizziness, and palpitations, were observed in one patient (Hughes et al., 2019).
Mok et al. retrospectively reviewed 49 patients who received DLM and had final treatment outcomes after 24 weeks. They described four patients, of whom three cases died due to sudden cardiac problems, pneumonia, and acute myocardial infarction at 1 to 3 months following the completion of DLM therapy. Besides, the remaining one patient experienced an increased QTcF interval at 4 weeks after the administration of DLM (Mok et al., 2019). It was recommended that before the beginning and throughout the period of treatment with DLM, frequent monitoring of electrocardiograms is essential. In patients with QTcF > 500 ms, DLM treatment either should not be initiated or should be interrupted. Moreover, the serum electrolytes are required to be assessed at baseline, and in patients with electrolyte disturbances, especially hypokalemia and hypocalcemia, DLM should not be administrated (Deltyba Epar Product Information, 2014).
Clinical Trials
Although European Medicines Agency’s Committee for Medicinal Products for Human Use approved the DLM 8 years ago for use in a combination therapy for pulmonary MDR-TB cases, there are a few clinical studies investigating the exact role of DLM in the treatment of MDR- and XDR-TB (Lohrasbi et al., 2018). Gler et al., in a randomized controlled trial (RCT), presented the outcomes for 481 HIV-negative patients with pulmonary MDR-TB who received oral DLM at higher (200 mg twice daily) or lower (100 mg twice daily) doses, or placebo for 8 weeks plus a background drug regimen developed based on WHO guidelines.
The sputum of each patient was cultured weekly in Lowenstein–Jensen medium and in a mycobacterial growth indicator tube (MGIT) system, and ≥5 successive weekly cultures negative for MTB were defined as culture conversion. The results of both Lowenstein–Jensen and MGIT cultures demonstrated that the patients who received 100 or 200 mg of DLM twice daily had a significantly higher rate of culture conversion compared with those who received placebo. The analysis of adverse events in that clinical trial showed that the patients who received 100 mg of DLM had fewer adverse events than those who received 200 mg; most of the events were of similar incidence to patients who received placebo. While the groups receiving DLM had more frequent QT prolongation relative to the placebo group, no episodes of a prolonged QT were associated with clinical events. Overall, the outcomes of that investigation recognized DLM as a promising treatment options for MDR-TB (Gler et al., 2012).
In another double-blind phase three RCT, which was performed by Von Groote-Bidlingmaier and colleagues, the safety and efficacy of DLM for pulmonary MDR-TB therapy were investigated (von Groote-Bidlingmaier et al., 2019). A total of 511 patients received DLM at a dose of 100 mg twice daily for 8 weeks, followed by 200 mg once daily for 16 weeks or placebo plus an OBR, developed based on WHO guidelines. The sputum of patients were cultured weekly in the MGIT system, and the results showed no difference in mean time to culture conversion between DLM and placebo groups. Safety assessments indicated that 98% of patients had at least one clinical manifestation, of which approximately 27% were serious. None of the fatal adverse events were considered to be related to DLM. The outcomes of that study demonstrated no clinically significant episodes of a prolonged QT and provided evidence of the safety profile of DLM to support its use with other QT-prolonging antibiotics (such as BDQ) for MDR-TB therapy (von Groote-Bidlingmaier et al., 2019).
Recently, Dooley et al. conducted a phase two RCT on the effects of DLM and/or BDQ on QT interval in patients with drug-resistant TB. They enrolled 84 cases with MDR-TB or RIF-resistant TB taking multidrug background therapy. Clofazimine was not allowed, and levofloxacin replaced moxifloxacin. The patients received DLM at a dose of 100 mg twice daily for 6 months and BDQ at a dose of 400 mg daily for 2 weeks, followed by 200 mg three times weekly for 5.5 months. The results of electrocardiograms showed that the median QTc change from baseline was 8.6 ms (DLM), 12.3 ms (BDQ), and 20.7 ms (DLM + BDQ). Therefore, a QTc interval of DLM + BDQ was not more than additive. In addition, no participants had a grade 3 or 4 QTc manifestation, and no deaths occurred during the study. These outcomes provide supportive evidence of the safety of simultaneous DLM and BDQ use in patients with MDR-TB or RIF-resistant TB (Dooley et al., 2021).
Mallikaarjun et al. carried out a phase 1 RCT to investigate the interactions between DLM and other anti-TB antibiotics, including ETB and Rifater (RIF + INH + pyrazinamide) in healthy individuals. In that study, 55 individuals received multiple oral doses of DLM + placebo or DLM + ETB-Rifater or placebo + ETB-Rifater once daily. Plasma samples were then analyzed for DLM and its metabolites by liquid chromatography-tandem mass spectrometry assay. The results showed that the coadministration of DLM with other anti-TB antibiotics has no clinically significant interactions (Mallikaarjun et al., 2016). In another phase 2 RCT, Zhang et al. performed a study to indicate the clinical benefit of DLM during MDR-TB therapy (Zhang et al., 2013).
In their survey, 38 HIV-negative patients with pulmonary MDR-TB assigned and received 100 or 200 mg of DLM twice daily or placebo for 8 weeks in combination with an OBR, including six anti-TB agents for MDR-TB therapy. During 10 weeks, culture conversion occurred among 32 patients. The safety analysis indicated clinical manifestations, including QT prolongation, hypercortisolemia, and psychiatric adverse events, among 13 cases. Taken together, their evaluation demonstrated that DLM was effective and well tolerated for MDR-TB treatment (Zhang et al., 2013).
In another study conducted by Koirala et al., the effectiveness of BDQ (and/or DLM) containing regimens in TB patients was evaluated. The antibiotic resistance pattern of the patients was severe (> 30% with XDR-TB). The median number of resistant drugs was 6 (Blair and Scott, 2015; Diel et al., 2015; Diel et al., 2015; Cox et al., 2018; World Health Organization, 2020; Khoshnood et al., 2021) and 5 (Blair and Scott, 2015; Cox et al., 2018; Heidary et al., 2019; Khoshnood et al., 2021) in patients with a final outcome and overall cohort, respectively. The proportion of patients achieving culture conversion and sputum smear ranged from 88.8 to 89.3% (patients with a final outcome) to 92.8% and 93.4% (whole cohort), respectively. Among the patients treated with BDQ, but not DLM, 284 (74.2%) achieved treatment success. However, 63 (16.5%) patients were lost to follow-up, 11 (2.9%) failed, and 25 (6.5%) died (Koirala et al., 2021).
Conclusion
The increasing global prevalence of MDR- and XDR-TB highlights an urgent need for more effective drugs. Unfortunately, a few studies have hitherto addressed the effect of DLM on drug-resistant MTB. Available evidence suggests that due to the low resistance of DLM among MDR and XDR strains of MTB, this antibiotic can be considered as a promising anti-TB drug. Moreover, the synergism of DLM in combination with BDQ can ameliorate the effectiveness of this agent against pan-drug-resistant MTB isolates.
Author Contributions
All authors listed have made a substantial, direct and intellectual contribution to the work, and approved it for publication.
Conflict of Interest
The authors declare that the research was conducted in the absence of any commercial or financial relationships that could be construed as a potential conflict of interest.
Publisher’s Note
All claims expressed in this article are solely those of the authors and do not necessarily represent those of their affiliated organizations, or those of the publisher, the editors and the reviewers. Any product that may be evaluated in this article, or claim that may be made by its manufacturer, is not guaranteed or endorsed by the publisher.
References
Bashiri, G., Antoney, J., Jirgis, E. N., Shah, M. V., Ney, B., Copp, J., et al. (2019). A revised biosynthetic pathway for the cofactor F 420 in prokaryotes. Nat. Commun. 10:1558.
Bashiri, G., Rehan, A. M., Greenwood, D. R., Dickson, J. M., and Baker, E. N. (2010). Metabolic engineering of cofactor F 420 production in mycobacterium smegmatis. PLoS One 5:e15803. doi: 10.1371/journal.pone.0015803
Battaglia, S., Spitaleri, A., Cabibbe, A. M., Meehan, C., Utpatel, C., Ismail, N., et al. (2020). Characterization of genomic variants associated with resistance to bedaquiline and delamanid in naïve Mycobacterium tuberculosis clinical strains. J. Clin. Microbiol. 58, e01304–e1320.
Blair, H. A., and Scott, L. J. (2015). Delamanid: a review of its use in patients with multidrug-resistant tuberculosis. Drugs 75, 91–100. doi: 10.1007/s40265-014-0331-4
Bloemberg, G. V., Gagneux, S., and Böttger, E. C. (2015). Acquired resistance to bedaquiline and delamanid in therapy for tuberculosis. New Engl. J. Med. 373:1986. doi: 10.1056/nejmc1505196
Borisov, S. E., Dheda, K., Enwerem, M., Leyet, R. R., D’Ambrosio, L., Centis, R., et al. (2017). Effectiveness and safety of bedaquiline-containing regimens in the treatment of MDR-and XDR-TB: a multicentre study. Eur. Respir. J. 49:1700387.
Chandramohan, Y., Padmanaban, V., Bethunaickan, R., Tripathy, S., Swaminathan, S., and Ranganathan, U. D. (2019). In vitro interaction profiles of the new antitubercular drugs bedaquiline and delamanid with moxifloxacin against clinical mycobacterium tuberculosis isolates. J. Glob. Antimicrob. Resist. 19, 348–353. doi: 10.1016/j.jgar.2019.06.013
Chang, K. C., and Sotgiu, G. (2017). Treatment options for MDR-and XDR-TB. Anti Infect. Lung ERS Monogr. 75:232. doi: 10.1183/2312508x.10005616
Chen, X., Hashizume, H., Tomishige, T., Nakamura, I., Matsuba, M., Fujiwara, M., et al. (2017). Delamanid kills dormant mycobacteria in vitro and in a guinea pig model of tuberculosis. Antimicrob. Agents Chemother. 61:e02402.
Cox, V., Brigden, G., Crespo, R., Lessem, E., Lynch, S., Rich, M., et al. (2018). Global programmatic use of bedaquiline and delamanid for the treatment of multidrug-resistant tuberculosis. Int. J. Tuberc. Lung Dis. 22, 407–412. doi: 10.5588/ijtld.17.0706
Das, M., Dalal, A., Laxmeshwar, C., Ravi, S., Mamnoon, F., Meneguim, A. C., et al. (2020a). One step forward: successful end-of-treatment outcomes of patients with drug-resistant tuberculosis who received concomitant bedaquiline and delamanid in Mumbai, India. Clin. Infect. Dis. 20:ciaa1577.
Das, M., Mamnoon, F., Mansoor, H., Meneguim, A., Singh, P., Shah, I., et al. (2020b). New TB drugs for the treatment of children and adolescents with rifampicin-resistant TB in Mumbai, India. Int. J. Tuberc. Lung Dis. 24, 1265–1271. doi: 10.5588/ijtld.20.0165
Deane, B. R., and Porkess, S. (2018). Clinical trial transparency update: an assessment of the disclosure of results of company-sponsored trials associated with new medicines approved in Europe in 2014. Curr. Med. Res. Opin. 34, 1239–1243. doi: 10.1080/03007995.2017.1415057
Deltyba Epar Product Information. (2014). Annex I Summary of Product Characteristics. Committee for Medicinal Products for Human Use. Amsterdam: European Medicines Agency.
Diacon, A., Dawson, R., Hanekom, M., Narunsky, K., Venter, A., Hittel, N., et al. (2011). Early bactericidal activity of delamanid (OPC-67683) in smear-positive pulmonary tuberculosis patients. Int. J. Tuberc. Lung Dis. 15, 949–954. doi: 10.5588/ijtld.10.0616
Diel, R., Hittel, N., and Schaberg, T. (2015). Cost effectiveness of treating multi-drug resistant tuberculosis by adding DeltybaTM to background regimens in Germany. Respir. Med. 109, 632–641. doi: 10.1016/j.rmed.2015.01.017
Dooley, K. E., Rosenkranz, S. L., Conradie, F., Moran, L., Hafner, R., von Groote-Bidlingmaier, F., et al. (2021). QT effects of bedaquiline, delamanid, or both in patients with rifampicin-resistant tuberculosis: a phase 2, open-label, randomised, controlled trial. Lancet Infect. Dis. 21, 975–983. doi: 10.1016/s1473-3099(20)30770-2
Ferlazzo, G., Mohr, E., Laxmeshwar, C., Hewison, C., Hughes, J., Jonckheere, S., et al. (2018). Early safety and efficacy of the combination of bedaquiline and delamanid for the treatment of patients with drug-resistant tuberculosis in Armenia, India, and South Africa: a retrospective cohort study. Lancet Infect. Dis. 18, 536–544. doi: 10.1016/s1473-3099(18)30100-2
Fujiwara, M., Kawasaki, M., Hariguchi, N., Liu, Y., and Matsumoto, M. (2018). Mechanisms of resistance to delamanid, a drug for Mycobacterium tuberculosis. Tuberculosis 108, 186–194. doi: 10.1016/j.tube.2017.12.006
Gler, M. T., Skripconoka, V., Sanchez-Garavito, E., Xiao, H., Cabrera-Rivero, J. L., Vargas-Vasquez, D. E., et al. (2012). Delamanid for multidrug-resistant pulmonary tuberculosis. N. Engl. J. Med. 366, 2151–2160.
Greening, C., Ahmed, F. H., Mohamed, A. E., Lee, B. M., Pandey, G., Warden, A. C., et al. (2016). Physiology, biochemistry, and applications of F420-and Fo-dependent redox reactions. Microbiol. Mol. Biol. Rev. 80, 451–493. doi: 10.1128/mmbr.00070-15
Gurumurthy, M., Mukherjee, T., Dowd, C. S., Singh, R., Niyomrattanakit, P., Tay, J. A., et al. (2012). Substrate specificity of the deazaflavin−dependent nitroreductase from Mycobacterium tuberculosis responsible for the bioreductive activation of bicyclic nitroimidazoles. FEBS J. 279, 113–125. doi: 10.1111/j.1742-4658.2011.08404.x
Hameed, H., Islam, M. M., Chhotaray, C., Wang, C., Liu, Y., Tan, Y., et al. (2018). Molecular targets related drug resistance mechanisms in MDR-, XDR-, and TDR-Mycobacterium tuberculosis strains. Front. Cell. Infect. Microbiol. 8:114. doi: 10.3389/fcimb.2018.00114
Hanaki, E., Hayashi, M., and Matsumoto, M. (2017). Delamanid is not metabolized by Salmonella or human nitroreductases: a possible mechanism for the lack of mutagenicity. Regul. Toxicol. Pharmacol. 84, 1–8. doi: 10.1016/j.yrtph.2016.12.002
Hartkoorn, R. C., Ryabova, O. B., Chiarelli, L. R., Riccardi, G., Makarov, V., and Cole, S. T. (2014). Mechanism of action of 5-nitrothiophenes against mycobacterium tuberculosis. Antimicrob. Agents Chemother. 58, 2944–2947. doi: 10.1128/aac.02693-13
Heidary, M., Bostanabad, S. Z., Amini, S. M., Jafari, A., Nobar, M. G., Ghodousi, A., et al. (2019). The anti-mycobacterial activity of Ag, ZnO, and Ag-ZnO nanoparticles against MDR-and XDR-Mycobacterium tuberculosis. Infect. Drug Resist. 12:3425. doi: 10.2147/idr.s221408
Heidary, M., and Nasiri, M. J. (2016). Why has MDR-TB prevalence increased in Iran? J. Clin. Tuberc. Other Mycobact. Dis. 5:9. doi: 10.1016/j.jctube.2016.11.004
Hoffmann, H., Kohl, T. A., Hofmann-Thiel, S., Merker, M., Beckert, P., Jaton, K., et al. (2016). Delamanid and bedaquiline resistance in Mycobacterium tuberculosis ancestral Beijing genotype causing extensively drug-resistant tuberculosis in a Tibetan refugee. Am. J. Respir. Crit. Care Med. 193, 337–340. doi: 10.1164/rccm.201502-0372le
Hu, M., Zheng, C., and Gao, F. (2016). Use of bedaquiline and delamanid in diabetes patients: clinical and pharmacological considerations. Drug Design Dev. Ther. 10:3983. doi: 10.2147/dddt.s121630
Hughes, J., Reuter, A., Chabalala, B., Isaakidis, P., Cox, H., and Mohr, E. (2019). Adverse events among people on delamanid for rifampicin-resistant tuberculosis in a high HIV prevalence setting. Int. J. Tuberc. Lung Dis. 23, 1017–1023. doi: 10.5588/ijtld.18.0651
Igarashi, M. (2017). Development of new antituberculosis drugs from natural products. Biosci. Biotechnol. Biochem. 81, 32–37. doi: 10.1080/09168451.2016.1248369
Jing, W., Zhang, T., Zong, Z., Xue, Y., Shang, Y., Wang, F., et al. (2019). Comparison of in vitro activity of the nitroimidazoles delamanid and pretomanid against multidrug-resistant and extensively drug-resistant tuberculosis. Eur. J. Clin. Microbiol. Infect. Dis. 38, 1293–1296. doi: 10.1007/s10096-019-03551-w
Kadura, S., King, N., Nakhoul, M., Zhu, H., Theron, G., Köser, C. U., et al. (2020). Systematic review of mutations associated with resistance to the new and repurposed Mycobacterium tuberculosis drugs bedaquiline, clofazimine, linezolid, delamanid and pretomanid. J. Antimicrob. Chemother. 75, 2031–2043. doi: 10.1093/jac/dkaa136
Kang, H., Jo, K.-W., Jeon, D., Yim, J.-J., and Shim, T. S. (2020). Interim treatment outcomes in multidrug-resistant tuberculosis using bedaquiline and/or delamanid in South Korea. Respir. Med. 167:105956. doi: 10.1016/j.rmed.2020.105956
Kardan-Yamchi, J., Kazemian, H., Battaglia, S., Abtahi, H., Foroushani, A. R., Hamzelou, G., et al. (2020). Whole genome sequencing results associated with minimum inhibitory concentrations of 14 anti-tuberculosis drugs among rifampicin-resistant isolates of mycobacterium tuberculosis from Iran. J. Clin. Med. 9:465. doi: 10.3390/jcm9020465
Khoshnood, S., Goudarzi, M., Taki, E., Darbandi, A., Kouhsari, E., Heidary, M., et al. (2021). Bedaquiline: current status and future perspectives. J. Glob. Antimicrob. Resist. 25, 48–59.
Kim, D. H., Jhun, B. W., Moon, S. M., Kim, S.-Y., Jeon, K., Kwon, O. J., et al. (2019). In vitro activity of bedaquiline and delamanid against nontuberculous mycobacteria, including macrolide-resistant clinical isolates. Antimicrob. Agents Chemother. 63:e00665.
Koch, A., Cox, H., and Mizrahi, V. (2018). Drug-resistant tuberculosis: challenges and opportunities for diagnosis and treatment. Curr. Opin. Pharmacol. 42, 7–15. doi: 10.1016/j.coph.2018.05.013
Koh, W.-J. (2017). Nontuberculous mycobacteria—overview. Microbiol. Spectr. 5, 653–661. doi: 10.1128/9781555819866.ch39
Koirala, S., Borisov, S., Danila, E., Mariandyshev, A., Shrestha, B., Lukhele, N., et al. (2021). Outcome of treatment of MDR-TB or drug-resistant patients treated with bedaquiline and delamanid: results from a large global cohort. Pulmonology 27, 403–412.
Krieger, D., Schönfeld, N., Vesenbeckh, S., Bettermann, G., Bauer, T. T., Rüssmann, H., et al. (2016). Is delamanid a potential agent in the treatment of diseases caused by Mycobacterium avium-intracellulare? Eur. Respir. J. 48, 1803–1804. doi: 10.1183/13993003.01420-2016
Lee, E. H., Yong, S. H., Leem, A. Y., Lee, S. H., Kim, S. Y., Chung, K. S., et al. (2019). Improved fluoroquinolone-resistant and extensively drug-resistant tuberculosis treatment outcomes. Open Forum Infect. Dis. 6:ofz118.
Lee, T., Lee, S. J., Jeon, D., Lee, H. Y., Kim, H.-J., Kang, B. H., et al. (2021). Additional drug resistance in patients with multidrug-resistant tuberculosis in korea: a multicenter study from 2010 to 2019. J. Korean Med. Sci. 36:e174.
Lewis, J. M., and Sloan, D. J. (2015). The role of delamanid in the treatment of drug-resistant tuberculosis. Ther. Clin. Risk Manag. 11:779. doi: 10.2147/tcrm.s71076
Li, Y., Sun, F., and Zhang, W. (2019). Bedaquiline and delamanid in the treatment of multidrug−resistant tuberculosis: promising but challenging. Drug Dev. Res. 80, 98–105.
Lienhardt, C., Nunn, A., Chaisson, R., Vernon, A. A., Zignol, M., Nahid, P., et al. (2020). Advances in clinical trial design: weaving tomorrow’s TB treatments. PLoS Med. 17:e1003059. doi: 10.1371/journal.pmed.1003059
Liu, Y., Matsumoto, M., Ishida, H., Ohguro, K., Yoshitake, M., Gupta, R., et al. (2018). Delamanid: from discovery to its use for pulmonary multidrug-resistant tuberculosis (MDR-TB). Tuberculosis 111, 20–30. doi: 10.1016/j.tube.2018.04.008
Lohrasbi, V., Talebi, M., Bialvaei, A. Z., Fattorini, L., Drancourt, M., Heidary, M., et al. (2018). Trends in the discovery of new drugs for mycobacterium tuberculosis therapy with a glance at resistance. Tuberculosis 109, 17–27.
Mallikaarjun, S., Wells, C., Petersen, C., Paccaly, A., Shoaf, S. E., Patil, S., et al. (2016). Delamanid coadministered with antiretroviral drugs or antituberculosis drugs shows no clinically relevant drug-drug interactions in healthy subjects. Antimicrob. Agents Chemother. 60, 5976–5985. doi: 10.1128/aac.00509-16
Matsumoto, M., Hashizume, H., Tomishige, T., Kawasaki, M., Tsubouchi, H., Sasaki, H., et al. (2006). OPC-67683, a nitro-dihydro-imidazooxazole derivative with promising action against tuberculosis in vitro and in mice. PLoS Med. 3:e466. doi: 10.1371/journal.pmed.0030466
Matsumoto, M., Hashizume, H., Tsubouchi, H., Sasaki, H., Itotani, M., Kuroda, H., et al. (2007). Screening for novel antituberculosis agents that are effective against multidrug resistant tuberculosis. Curr. Top. Med. Chem. 7, 499–507. doi: 10.2174/156802607780059727
Matteelli, A., D’Ambrosio, L., Centis, R., Tadolini, M., and Migliori, G. B. (2015). Compassionate and optimum use of new tuberculosis drugs. Lancet Infect. Dis. 15, 1131–1132. doi: 10.1016/s1473-3099(15)00294-7
Migliori, G. B., Pontali, E., Sotgiu, G., Centis, R., D’Ambrosio, L., Tiberi, S., et al. (2017). Combined use of delamanid and bedaquiline to treat multidrug-resistant and extensively drug-resistant tuberculosis: a systematic review. Int. J. Mol. Sci. 18:341.
Miyamoto, G., Shimokawa, Y., Itose, M., Koga, T., Hirao, Y., and Kashiyama, E. (2005). Unique PK profile of OPC-67683, A New Potent Anti-Tuberculous Drug. Intersci Conf Antimicrob Agents Chemother. Washington, DC: American Society for Microbiology.
Mok, J., Kang, H., Hwang, S. H., Park, J. S., Kang, B., Lee, T., et al. (2018). Interim outcomes of delamanid for the treatment of MDR-and XDR-TB in South Korea. J. Antimicrob. Chemother. 73, 503–508. doi: 10.1093/jac/dkx373
Mok, J., Kang, H., Koh, W.-J., Jhun, B. W., Yim, J.-J., Kwak, N., et al. (2019). Final treatment outcomes of delamanid-containing regimens in patients with MDR-/XDR-TB in South Korea. Eur. Respir. J. 54:1900811. doi: 10.1183/13993003.00811-2019
Mukherjee, T., and Boshoff, H. (2011). Nitroimidazoles for the treatment of TB: past, present and future. Future Med. Chem. 3, 1427–1454. doi: 10.4155/fmc.11.90
Nguyen, T. V. A., Anthony, R. M., Cao, T. T. H., Bañuls, A.-L., Nguyen, V. A. T., Vu, D. H., et al. (2020). Delamanid resistance: update and clinical management. Clin. Infect. Dis. 71, 3252–3259. doi: 10.1093/cid/ciaa755
Olayanju, O., Esmail, A., Limberis, J., and Dheda, K. (2020). A regimen containing bedaquiline and delamanid compared to bedaquiline in patients with drug-resistant tuberculosis. Eur. Respir. J. 55:1901181. doi: 10.1183/13993003.01181-2019
Oye, K., Baird, L., Chia, A., Hocking, S., Hutt, P., Lee, D., et al. (2013). Legal foundations of adaptive licensing: legal foundations of adaptive licensing 3 April 2012 european medicines agency, canary Wharf, London, UK. Clin. Pharmacol. Ther. 94, 309–311. doi: 10.1038/clpt.2013.95
Pang, Y., Zong, Z., Huo, F., Jing, W., Ma, Y., Dong, L., et al. (2017). In vitro drug susceptibility of bedaquiline, delamanid, linezolid, clofazimine, moxifloxacin, and gatifloxacin against extensively drug-resistant tuberculosis in Beijing, China. Antimicrob. Agents Chemother. 61, e00900–e0917.
Parikh, S. L., Xiao, G., and Tonge, P. J. (2000). Inhibition of InhA, the enoyl reductase from mycobacterium tuberculosis, by triclosan and isoniazid. Biochemistry 39, 7645–7650.
Patterson, S., Wyllie, S., Norval, S., Stojanovski, L., Simeons, F. R., Auer, J. L., et al. (2016). The anti-tubercular drug delamanid as a potential oral treatment for visceral leishmaniasis. Elife 5:e09744.
Pieterman, E. D., Keutzer, L., van der Meijden, A., van den Berg, S., Wang, H., Zimmerman, M. D., et al. (2021). Superior efficacy of a bedaquiline, delamanid and linezolid combination regimen in a mouse-TB model. J. infect. Dis. 27:jiab043.
Polsfuss, S., Hofmann-Thiel, S., Merker, M., Krieger, D., Niemann, S., Rüssmann, H., et al. (2019). Emergence of low-level delamanid and bedaquiline resistance during extremely drug-resistant tuberculosis treatment. Clin. Infect. Dis. 69, 1229–1231.
Pontali, E., Sotgiu, G., Tiberi, S., Tadolini, M., Visca, D., D’Ambrosio, L., et al. (2018). Combined treatment of drug-resistant tuberculosis with bedaquiline and delamanid: a systematic review. Eur. Respir. J. 52:1800934. doi: 10.1183/13993003.00934-2018
Portevin, D., Sukumar, S., Coscolla, M., Shui, G., Li, B., Guan, X. L., et al. (2014). Lipidomics and genomics of Mycobacterium tuberculosis reveal lineage-specific trends in mycolic acid biosynthesis. Microbiologyopen 3, 823–835. doi: 10.1002/mbo3.193
Ramón-García, S., Del Río, R. G., Villarejo, A. S., Sweet, G. D., Cunningham, F., Barros, D., et al. (2016). Repurposing clinically approved cephalosporins for tuberculosis therapy. Sci. Rep. 6:34293.
Ramón-García, S., Ng, C., Anderson, H., Chao, J. D., Zheng, X., Pfeifer, T., et al. (2011). Synergistic drug combinations for tuberculosis therapy identified by a novel high-throughput screen. Antimicrob. Agents Chemother. 55, 3861–3869. doi: 10.1128/aac.00474-11
Reichmuth, M. L., Hömke, R., Zürcher, K., Sander, P., Avihingsanon, A., Collantes, J., et al. (2020). Natural polymorphisms in mycobacterium tuberculosis conferring resistance to delamanid in drug-naïve patients. Antimicrob. Agents Chemother. 64:e00513.
Rifat, D., Li, S.-Y., Ioerger, T., Shah, K., Lanoix, J.-P., Lee, J., et al. (2020). Mutations in fbiD (Rv2983) as a novel determinant of resistance to pretomanid and delamanid in Mycobacterium tuberculosis. Antimicrob. Agents Chemother. 65:e01948.
Rustomjee, R., and Zumla, A. (2015). Delamanid expanded access novel treatment of drug resistant tuberculosis. Infect. Drug Resist. 8:359. doi: 10.2147/idr.s62119
Saliu, O. Y., Crismale, C., Schwander, S. K., and Wallis, R. S. (2007). Bactericidal activity of OPC-67683 against drug-tolerant Mycobacterium tuberculosis. J. Antimicrob. Chemother. 60, 994–998.
Sasahara, K., Shimokawa, Y., Hirao, Y., Koyama, N., Kitano, K., Shibata, M., et al. (2015). Pharmacokinetics and metabolism of delamanid, a novel anti-tuberculosis drug, in animals and humans: importance of albumin metabolism in vivo. Drug Metab. Dispos. 43, 1267–1276. doi: 10.1124/dmd.115.064527
Sasaki, H., Haraguchi, Y., Itotani, M., Kuroda, H., Hashizume, H., Tomishige, T., et al. (2006). Synthesis and antituberculosis activity of a novel series of optically active 6-nitro-2, 3-dihydroimidazo [2, 1-b] oxazoles. J. Med. Chem. 49, 7854–7860. doi: 10.1021/jm060957y
Schena, E., Nedialkova, L., Borroni, E., Battaglia, S., Cabibbe, A. M., Niemann, S., et al. (2016). Delamanid susceptibility testing of mycobacterium tuberculosis using the resazurin microtitre assay and the BACTECTM MGITTM 960 system. J. Antimicrob. Chemother. 71, 1532–1539.
Schuster Bruce, C., Brhlikova, P., Heath, J., and McGettigan, P. (2019). The use of validated and nonvalidated surrogate endpoints in two european medicines agency expedited approval pathways: a cross-sectional study of products authorised 2011–2018. PLoS Med. 16:e1002873. doi: 10.1371/journal.pmed.1002873
Senghore, M., Diarra, B., Gehre, F., Otu, J., Worwui, A., Muhammad, A. K., et al. (2020). Evolution of mycobacterium tuberculosis complex lineages and their role in an emerging threat of multidrug resistant tuberculosis in Bamako, Mali. Sci. Rep. 10:327.
Singh, R., Manjunatha, U., Boshoff, H. I., Ha, Y. H., Niyomrattanakit, P., Ledwidge, R., et al. (2008). PA-824 kills nonreplicating mycobacterium tuberculosis by intracellular NO release. Science 322, 1392–1395. doi: 10.1126/science.1164571
Skripconoka, V., Danilovits, M., Pehme, L., Tomson, T., Skenders, G., Kummik, T., et al. (2013). Delamanid improves outcomes and reduces mortality in multidrug-resistant tuberculosis. Eur. Respir. J. 41, 1393–1400. doi: 10.1183/09031936.00125812
Stinson, K., Kurepina, N., Venter, A., Fujiwara, M., Kawasaki, M., Timm, J., et al. (2016). MIC of delamanid (OPC-67683) against mycobacterium tuberculosis clinical isolates and a proposed critical concentration. Antimicrob. Agents Chemother. 60, 3316–3322. doi: 10.1128/aac.03014-15
Szumowski, J. D., and Lynch, J. B. (2015). Profile of delamanid for the treatment of multidrug-resistant tuberculosis. Drug Design Dev. Ther. 9:677. doi: 10.2147/dddt.s60923
Tadolini, M., Garcia-Prats, A. J., D’Ambrosio, L., Hewison, C., Centis, R., Schaaf, H. S., et al. (2016). Compassionate use of new drugs in children and adolescents with multidrug-resistant and extensively drug-resistant tuberculosis: early experiences and challenges. Eur. Respir. J. 48, 938–943. doi: 10.1183/13993003.00705-2016
Tesema, E., Wares, F., Bedru, A., Negeri, C., Molla, Y., Gemechu, D., et al. (2021). Experiences of introducing new drugs for drug-resistant TB at the ALERT Hospital, Addis Ababa, Ethiopia, 2017–2019. Public Health Action 11, 50–52.
Tsubouchi, H., Sasaki, H., Ishikawa, H., and Matsumoto, M. (2016). Discovery of delamanid for the treatment of multidrug-resistant pulmonary tuberculosis. Successful Drug Discov. 1, 137–161. doi: 10.1002/9783527800315.ch7
Vilchèze, C. (2020). Mycobacterial cell wall: a source of successful targets for old and new drugs. Appl. Sci. 10:2278. doi: 10.3390/app10072278
von Groote-Bidlingmaier, F., Patientia, R., Sanchez, E., Balanag, V. Jr., Ticona, E., Segura, P., et al. (2019). Efficacy and safety of delamanid in combination with an optimised background regimen for treatment of multidrug-resistant tuberculosis: a multicentre, randomised, double-blind, placebo-controlled, parallel group phase 3 trial. Lancet Respir. Med. 7, 249–259.
Wang, G., Jiang, G., Jing, W., Zong, Z., Yu, X., Chen, S., et al. (2021). Prevalence and molecular characterizations of seven additional drug resistance among multidrug-resistant tuberculosis in China: a subsequent study of a national survey. J. Infect. 82, 371–377.
World Health Organization. (2016). WHO Treatment Guidelines for Drug-Resistant Tuberculosis. Geneva: World Health Organization.
World Health Organization. (2020). Global Tuberculosis Report 2020: Executive Summary. Geneva: World Health Organization.
Yang, J. S., Kim, K. J., Choi, H., and Lee, S. H. (2018). Delamanid, bedaquiline, and linezolid minimum inhibitory concentration distributions and resistance-related gene mutations in multidrug-resistant and extensively drug-resistant tuberculosis in Korea. Ann. Lab. med. 38:563.
Yimer, S. A., Kalayou, S., Homberset, H., Birhanu, A. G., Riaz, T., Zegeye, E. D., et al. (2020). Lineage-specific proteomic signatures in the mycobacterium tuberculosis complex reveal differential abundance of proteins involved in virulence, DNA repair, CRISPR-Cas, bioenergetics and lipid metabolism. Front. Microbiol. 11:550760. doi: 10.3389/fmicb.2020.550760
Yoshiyama, T., Mitarai, S., Takaki, A., Aono, A., Okumura, M., Ohta, K., et al. (2020). Multi-drug resistant tuberculosis with simultaneously acquired-drug resistance to bedaquiline and delamanid. Clin. Infect. Dis. 30:ciaa1064.
Yu, X., Gao, X., Li, C., Luo, J., Wen, S., Zhang, T., et al. (2019). In vitro activities of bedaquiline and delamanid against nontuberculous mycobacteria isolated in Beijing, China. Antimicrob. Agents Chemother. 63:e00031.
Zhang, Q., Liu, Y., Tang, S., Sha, W., and Xiao, H. (2013). Clinical benefit of delamanid (OPC-67683) in the treatment of multidrug-resistant tuberculosis patients in China. Cell Biochem. Biophys. 67, 957–963.
Zheng, H., He, W., Jiao, W., Xia, H., Sun, L., Wang, S., et al. (2021). Molecular characterization of multidrug-resistant tuberculosis against levofloxacin, moxifloxacin, bedaquiline, linezolid, clofazimine, and delamanid in southwest of China. BMC Infect. Dis. 21:330. doi: 10.21203/rs.3.rs-159138/v1
Keywords: Mycobacterium tuberculosis, tuberculosis, delamanid, review, TB
Citation: Khoshnood S, Taki E, Sadeghifard N, Kaviar VH, Haddadi MH, Farshadzadeh Z, Kouhsari E, Goudarzi M and Heidary M (2021) Mechanism of Action, Resistance, Synergism, and Clinical Implications of Delamanid Against Multidrug-Resistant Mycobacterium tuberculosis. Front. Microbiol. 12:717045. doi: 10.3389/fmicb.2021.717045
Received: 02 June 2021; Accepted: 02 September 2021;
Published: 07 October 2021.
Edited by:
Wladimir Sougakoff, INSERM U1135 Centre d’Immunologie et de Maladies Infectieuses, FranceReviewed by:
Kaushiki Mazumdar, Kelly Government Solutions, United StatesJulian Ferreras, CONICET Institute of Subtropical Biology (IBS), Argentina
Copyright © 2021 Khoshnood, Taki, Sadeghifard, Kaviar, Haddadi, Farshadzadeh, Kouhsari, Goudarzi and Heidary. This is an open-access article distributed under the terms of the Creative Commons Attribution License (CC BY). The use, distribution or reproduction in other forums is permitted, provided the original author(s) and the copyright owner(s) are credited and that the original publication in this journal is cited, in accordance with accepted academic practice. No use, distribution or reproduction is permitted which does not comply with these terms.
*Correspondence: Mohsen Heidary, bW9oc2VuaGVpZGFyeTQwQGdtYWlsLmNvbQ==