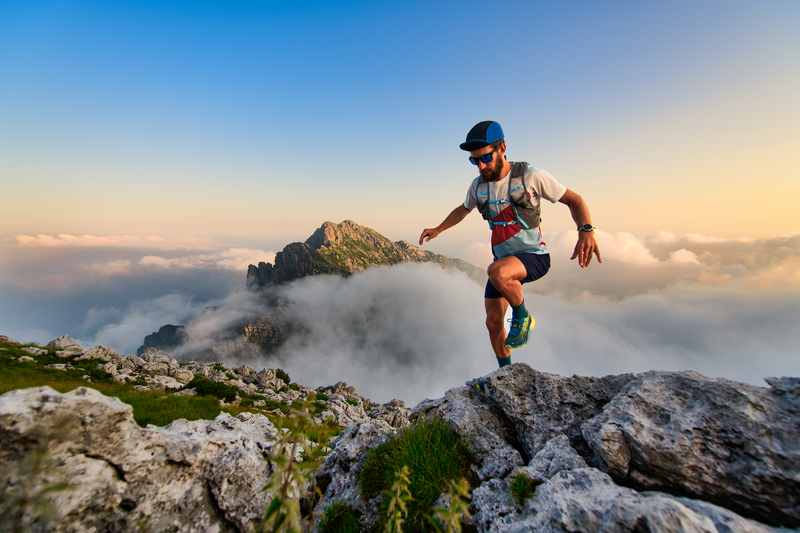
95% of researchers rate our articles as excellent or good
Learn more about the work of our research integrity team to safeguard the quality of each article we publish.
Find out more
ORIGINAL RESEARCH article
Front. Microbiol. , 20 July 2021
Sec. Antimicrobials, Resistance and Chemotherapy
Volume 12 - 2021 | https://doi.org/10.3389/fmicb.2021.712707
This article is part of the Research Topic Zoonotic Microorganisms and Spread of Acquired Polymyxin Resistance Determinants View all 15 articles
The emergence of the plasmid-mediated colistin resistance gene mcr-1 is threatening the last-line role of colistin in human medicine. With mcr-1-positive Escherichia coli (E. coli) isolated from food animal being frequently reported in China, the prevalence of mcr-1 in food animal has attracted public attention. In the present study, a total of 105 colistin-resistant E. coli strains were isolated from 200 fecal samples collected from six swine farms in northeastern China. mcr-PCR revealed that the prevalence of mcr-1 in colistin-resistant E. coli was 53.33% (56/105). mcr-1-positive E. coli showed extensive antimicrobial resistance profiles with the presence of additional resistance genes, increased expression of multidrug efflux pump-associated genes, and increased biofilm formation ability. MLST differentiated all the mcr-1-positive E. coli into 25 sequence types (STs) and five unknown ST, and the most common ST was ST10 (n = 11). By phylogenetic group classification, the distribution of all mcr-1-positive E. coli belonging to groups A, B1, B2, and D was 46.43, 35.71, 5.36, and 5.36%, respectively. Conjugation experiment demonstrated that most of the mcr-1 were transferable at frequencies of 2.68 × 10–6–3.73 × 10–3 among 30 representative mcr-1-positive E. coli. The plasmid replicon types IncI2 (n = 9), IncX4 (n = 5), IncHI2 (n = 3), IncN (n = 3), and IncP (n = 1) were detected in the transconjugants. The results of growth assay, competition experiment, and plasmid stability testing showed that acquisition of mcr-1-harboring plasmids could reduce the fitness of bacterial hosts, but mcr-1 remained stable in the recipient strain. Due to the potential possibility of these mcr-1-positive E. coli being transmitted to humans through the food chain or through horizontal transmission, therefore, it is necessary to continuously monitor the prevalence and dissemination of mcr-1 in food animal, particularly in swine.
The discovery and use of antibiotics in human medicine was regarded as one of the vast medical advancements over the past decades, and antibiotics also play an important role in food-animal agriculture (Worthington and Melander, 2013). The increasing amount of animal protein for human consumption accelerates the development of modern animal production. However, the widespread use of antibiotics in livestock has posed a significant public health threat, which can potentially increase selection pressure on antibiotic-resistant bacteria (ARB) and further promote the dissemination of ARB in livestock (You and Silbergeld, 2014). Moreover, the animal-origin ARB can be transmitted to humans through the environment and food chain as well as through direct contact (Graham et al., 2009).
Escherichia coli is one of the major pathogens in the swine industry, which is associated with gastrointestinal diseases and systemic infections, including diarrhea, edema disease, septicemia, polyserositis, mastitis, and urinary tract infections (Fairbrother et al., 2005). These diseases can lead to morbidity, mortality, and delayed growth, which are responsible for considerable economic losses and restrict the development of the swine industry. To maintain health and productivity, antibiotics are widely administered to treat E. coli infections in farms to swine via oral, either in feed or in water (Fairbrother et al., 2005). Among a variety of antibiotics used in swine farms, polypeptides and aminoglycosides are most frequently administrated (Sabine et al., 2017).
Colistin is a kind of cationic polypeptides and a member of the polymyxin family, including polymyxins A, B, C, D, and E. Only polymyxin B and polymyxin E (colistin) are currently used clinically. Due to the broad-spectrum activity against a wide range of Gram-negative bacteria (GNB), colistin is widely used in pig production to control intestinal infections caused by Enterobacteriaceae (Landman et al., 2008). The routine use of colistin in human medicine was abandoned in the 1970s due to its major side effects, including nephrotoxicity and neurotoxicity (Landman et al., 2008). However, with the emergence of multidrug-resistant Gram-negative bacteria (MDR-GNB) and the paucity of novel classes of antibiotics entering the clinic, colistin has been reintroduced to human clinical use as a last-line treatment option for severe infections caused by MDR-GNB (Falagas and Kasiakou, 2005). The rapid rise and dissemination of MDR-GNB led to the increased amounts of colistin used in humans and animals with the inevitable risk of accelerating the emergence of colistin resistance (Kempf et al., 2013).
Colistin resistance was commonly thought to be chromosomally mediated, until a novel plasmid-mediated colistin resistance gene mcr-1 was characterized in E. coli isolated from animals and humans in China at the end of 2015 (Liu et al., 2016). Because of the rapid horizontal spread of colistin resistance by plasmids, the discovery of mcr-1 has attracted public attention among physicians and veterinarians. To date, the cases of bacteria harboring mcr-1 gene have been found in 47 different countries across six continents (Asia, Europe, Africa, North America, South America, and Oceania) from humans, animals, and environmental samples (Shi et al., 2020). Due to the high prevalence of mcr-1-positive E. coli originating from food animal than from humans, food animal production, particularly pig production, has been singled out as the major cause of mcr-1 amplification and spread (Rhouma et al., 2016).
In this study, we aimed to investigate the prevalence and characteristics of mcr-1 in swine farms in northeastern China by determining (1) the carriage rate of mcr-1 in colistin-resistant E. coli isolated from swine fecal samples; (2) the antimicrobial resistance profiles of mcr-1-positive E. coli isolates; (3) the presence of additional resistance genes, the relative expression levels of multidrug efflux pump-associated genes, and biofilm formation ability in mcr-1-positive E. coli isolates; (4) the genetic relationship of the mcr-1-positive E. coli isolates by multilocus sequence typing (MLST) and phylogenetic group; and (5) the transferability, conjugation frequency, fitness cost, and plasmid stability of mcr-1.
Between July 2016 and June 2017, a total of 200 fecal swabs were collected from six swine farms in northeastern China, including Heilongjiang (Harbin), Jilin (Changchun), and Liaoning (Shenyang). In each province, two geographically distinct swine farms were selected; 40 fecal swabs were randomly collected from 40 different pigs in each farm in Harbin, and 30 fecal swabs were randomly collected from 30 different pigs in each farm in Changchun and Shenyang. Fecal swabs were collected by placing a wet cotton swab at the animal anus of 2∼5 cm with minor rotation. The samples brought to the laboratory were immediately streaked out on MacConkey agar and incubated at 37°C for 18 h. The putative E. coli isolates on MacConkey agar (bright pink with a dimple) per sample were transferred to eosin methylene blue agar for further purification and were incubated at 37°C for 18 h. Randomly selected colonies with typical E. coli morphology were selected from each sample for PCR detection of 16S rRNA gene and for sequencing (Seurinck et al., 2003). All confirmed E. coli isolates were stored at –80°C for further studies.
To isolate colistin-resistant E. coli, all the strains were screened on the MacConkey agar containing 2 μg/ml of colistin. The DNA templates of all colistin-resistant isolates were extracted using the DNA extraction kit (TIANGEN, Beijing, China) following the instructions of the manufacturer. The presence of mcr-1 in colistin-resistant E. coli was determined by PCR amplification and followed by Sanger sequencing as described previously (Liu et al., 2016).
The susceptibility of all mcr-1-positive strains to 26 antibiotics, namely meropenem, ertapenem, imipenem, ampicillin, ampicillin–sulbactam, amoxicillin/clavulanic acid, cefuroxime, ceftazidime, cefepime, ceftriaxone, cefoxitin, aztreonam, gentamicin, amikacin, kanamycin, streptomycin, ciprofloxacin, levofloxacin, tetracycline, doxycycline, tigecycline, chloramphenicol, florfenicol, fosfomycin, sulfisoxazole, and nitrofurantoin, was determined by the standard disk diffusion method in accordance with the Clinical and Laboratory Standards Institute (CLSI); the interpretation of the susceptibility result was according to CLSI (document M100), 2018, except those for florfenicol and sulfisoxazole which were interpreted according to the CLSI VET01-A4, and tigecycline was interpreted in accordance with the European Committee on Antimicrobial Susceptibility Testing (EUCAST) 2017. The E. coli ATCC 25922 was used as a quality control strain.
The presence of carbapenemase genes (blaKPC, blaNDM, blaOXA–48, and blaIMP) (Doyle et al., 2012), extended spectrum-β-lactamase (ESBL) genes (blaCTX–M) and non-ESBL genes (blaTEM, blaSHV, blaOXA–1) (Dallenne et al., 2010), pAmpC genes (blaCMY, blaFOX, and blaDHA) (Dallenne et al., 2010), tetracycline resistance genes [tet(A), tet(B), tet(C), and tet(M)] (Ng et al., 2001), aminoglycoside resistance genes [rmtA, rmtB, rmtC, rmtD, armA, nmpA, and aac(3)-IV] (Yeganeh Sefidan et al., 2019), fluoroquinolone resistance genes [qnrA, qnrB, qnrC, qnrD, qnrS, oqxAB, qepA, and aac(6′)-Ib-cr] (Ciesielczuk et al., 2013), streptomycin/spectinomycin resistance genes (strA, strB, and aadA) (Srinivasan et al., 2007), fosfomycin resistance genes (fosA and fosA3) (Lee et al., 2012), florfenicol resistance gene (floR) (Li et al., 2015), and sulfonamide resistance genes (sul1, sul2, and sul3) (Hammerum et al., 2006) was examined by PCR. The positive products were validated with Sanger sequencing, then all the obtained sequences were compared using Blast with those published in the NCBI database1.
The genetic relatedness of mcr-1-positive strains was investigated by MLST as previously described for E. coli (Tartof et al., 2005). Furthermore, a two-step multiplex PCR was performed to determine the phylogenetic group, and the primers used (chuA, yiaA, and TspE4.C2) and details were the same as previously described (Clermont et al., 2000). Phylogenetic trees for all sequence types (STs) were constructed using the neighbor-joining method with MEGA software (Kumar et al., 2018). Annotation for each isolate and tree embellishment were visualized using Itol2.
Eleven representative strains were chosen from all mcr-1-positive E. coli for the detection of the relative expression levels of genes encoding efflux pumps (acrA, mdfA, ydhE, acrE, tolC, mdtE, and mdtF), regulators (marA, soxS, fisF, dsrA, and evgA), and porin protein-encoding genes (ompC and ompF). Total RNA of mcr-1-positive strains and a reference strain E. coli ATCC 25922 was extracted using TRIzol reagent (Thermo Fisher Scientific, Waltham, MA, United States), and cDNA was synthesized with 5 × All-In-One MasterMix (ABM, Richmond, Canada) following the instructions of the manufacturer. The mdh gene was used as the housekeeping gene. Quantitative real-time PCR (BioEasy SYBR Green High ROX Master Mix, Bioer, Hangzhou, China) was performed according to the methods described by Vinué et al. (2015). The relative expression levels of the tested genes were calculated using the 2–ΔΔCT method as described by Huang W. et al. (2020).
All mcr-1-positive isolates were inoculated into 15 ml tubes containing 5 ml Luria–Bertani (LB) broth and then cultured overnight in a shaking incubator at 37°C. The biofilm formation assay of these isolates was then conducted in 96-well polystyrene flat-bottom microtiter plates as described previously (Teh et al., 2010). To quantify the biofilm formation ability, the absorbance values of the solution were measured at 590 nm using an automated Multiskan FC reader (Thermo Fisher Scientific). The experiment was repeated independently three times.
The transferability of mcr-1 was tested by conjugation experiment with mcr-1-positive E. coli as donors and rifampicin-resistant E. coli EC600 as recipient strains. The MacConkey agar plates containing rifampicin (256 μg/ml) and colistin (2 μg/ml) were used to select mcr-1-positive transconjugants. PCR analysis and DNA sequencing were carried out to confirm that transconjugants were derivatives of the recipient strain E. coli EC600. The transfer frequency of mcr-1 was determined as described in a previous study (Liu et al., 2016). The replicon types of the transconjugants were determined according to previous studies (Carattoli et al., 2005; Johnson et al., 2012).
To assess the fitness impact of mcr-1 carriage on the host, growth assay and in vitro competition experiment were carried out. Growth curves for the recipient (EC600) and mcr-1-positive E. coli transconjugants were performed in 96-well flat-bottom plates (Corning Inc., Corning, NY, United States) as described previously (Long et al., 2019). In vitro competition experiments were conducted using mcr-1-positive E. coli transconjugants competing with EC600. Twenty-four-hour competition experiments were performed as described previously (He et al., 2017). Growth assay and in vitro competition experiment were performed in triplicate.
To estimate the stability of the plasmid harboring mcr-1, plasmid stability experiments were performed using mcr-1-positive E. coli transconjugants as described previously (Sota et al., 2010).
A total of 176 E. coli strains were isolated from 200 fecal samples collected from six swine farms located in northeastern China, and the E. coli isolates showed high resistance rate to colistin (59.66%, 105/176). Colistin-resistant E. coli colonies were identified in 66.20% (47/71), 54.90% (28/51), and 55.56% (30/54) E. coli strains isolated from swine farms in Heilongjiang, Jilin, and Liaoning, respectively. mcr-PCR and sequencing revealed that 56 E. coli were positive for mcr-1, the carriage rate was extremely high (53.33%, 56/105), and the prevalence of mcr-1 in colistin-resistant E. coli isolated from swine farms in Heilongjiang, Jilin, and Liaoning was 46.81% (22/47), 53.57% (15/28), and 63.33% (19/30), respectively.
The susceptibility of 56 mcr-1-positive E. coli isolates to other antimicrobials was determined. The percentages of resistance rate are presented in Figure 1. There were a high rate of resistance (60–100%) to gentamicin, kanamycin, streptomycin, ciprofloxacin, levofloxacin, tetracycline, chloramphenicol, florfenicol, doxycycline, and sulfisoxazole; a moderate rate of resistance (20–60%) to ampicillin, ampicillin–sulbactam, amoxicillin–clavulanic acid, amikacin, and fosfomycin; and a low rate of resistance (<20%) to meropenem, ertapenem, imipenem, cefuroxime, ceftazidime, cefepime, ceftriaxone, cefoxitin, aztreonam, and nitrofurantoin. There were no strains that were resistant to tigecycline. As shown in Table 1, most of the mcr-1-positive E. coli were multidrug resistant.
Figure 1. The resistant rate of mcr-1-positive E. coli to other antibiotics. MEP, meropenem; ETP, ertapenem; IMP, imipenem; AMP, ampicillin; CXM, cefuroxime; CAZ, ceftazidime; FEP, cefepime; CRO, ceftriaxone; FOX, cefoxitin; ATM, aztreonam; SAM, ampicillin–sulbactam; AMC, amoxicillin–clavulanic acid; GEN, gentamicin; AMK, amikacin; KMC, kanamycin; STP, streptomycin; CIP, ciprofloxacin; LEV, levofloxacin; TEC, tetracycline; DOC, doxycycline; TGC, tigecycline; CMH, chloramphenicol; FFC, florfenicol; FOS, fosfomycin; SFN, sulfisoxazole; NFT, nitrofurantoin.
Molecular features revealed that most mcr-1-positive E. coli carried additional resistance genes, as shown in Figure 2. Overall, blaTEM (n = 56, 100%) was the most common non-ESBL gene in our study, followed by blaSHV–1 and blaOXA–1 that were identified in three (5.36%) and five (8.93%) isolates, respectively. In addition, the ESBL gene blaCTX–M was detected in eight (14.86%) mcr-1-positive E. coli isolates. The detected pAmpC genes were blaCMY (n = 10, 17.86%), blaFOX–5 (n = 5, 8.93%), and blaDHA–1 (n = 2, 3.57%). The carbapenemase genes (blaKPC, blaOXA, and blaIMP) were not detected, and only blaNDM–5 was detected in two (3.57%) isolates. Among aminoglycoside resistance genes, only rmtA [7, 12.50%) and aac(3)-IV (25, 44.64%] were detected. As for fluoroquinolone resistance genes, there were 10 (17.86%), 7 (12.50%), 31 (55.36%), 2 (3.57%), and 9 (16.07%) isolates harboring qnrD, qnrS, oqxAB, qepA, and aac(6′)-Ib-cr, respectively, but there were no strains harboring qnrA, qnrB, and qnrC. The number of isolates harboring tet(A), tet(B), and tet(M) was 27 (48.21%), 20 (35.71%), and 31 (55.36%), respectively. The plasmid-encoded floR gene that conferred chloramphenicol resistance was detected in 38 (67.86%) mcr-1-positive strains. The isolates positive for sulfonamide resistance genes comprised 33 (58.93%) strains harboring sul1, followed by 24 (42.86%) and 18 (32.14%) strains harboring sul2 and sul3, respectively. The strA and strB were closely associated with streptomycin resistance, which were detected in 34 (60.71%) and 39 (69.64%) isolates. The fosA (n = 9, 16.07%) and fosA3 (n = 17, 30.36%) were prevalent in fosfomycin-resistant isolates.
Figure 2. Analysis of phylogroup, antimicrobial resistance genes, and relationships among 56 mcr-1-positive E. coli isolates from swine farms in northeastern China. Relationships among 56 mcr-1-positive E. coli isolates are indicated using an unrooted tree based on the alignments of concatenated MLST allelic sequences using the neighbor-joining method. For comparison of resistance genes among the 56 mcr-1-positive E. coli isolates, the red squares represent positivity for antimicrobial resistance genes.
The genotyping results of mcr-1-positive E. coli are summarized in Table 2. The mcr-1-positive isolates were distributed into phylogroups A (n = 26), B1 (n = 20), B2 (n = 3), and D (n = 3), and the phylogroup was undefined for four isolates. MLST differentiated the 56 mcr-1-positive E. coli into 25 STs and five unknown ST (untypable). As shown in Figure 3, the most common ST was ST10 (n = 11), followed by ST48 (n = 4), ST20 (n = 3), ST3944 (n = 3), ST772 (n = 3), ST5229 (n = 3), ST617 (n = 2), ST410 (n = 2), ST93 (n = 2), ST898 (n = 2), and ST1421 (n = 2), and then by single ST isolates, including ST165, ST10580, ST3856, ST1589, ST398, ST1463, ST4379, ST2935, ST156, ST3014, ST131, ST224, ST6730, and ST9159. Moreover, ST10, ST48, and ST617 are different by one or two alleles and they correspond to clonal complex CC10. As shown in Figure 2, phylogenetic analysis of all mcr-1-positive E. coli underlined the evidence for the horizontal transfer of mcr-1.
Figure 3. Minimal spanning tree of mcr-1-positive E. coli. Each circle corresponds to one ST and the size of each circle indicated the number of isolates in this ST type.
According to the results of MLST, 11 STs were predominant among mcr-1-positive E. coli. One representative strain of E. coli was chosen from each ST for subsequent detection. As shown in Figure 4, compared with the E. coli ATCC 25922, the relative expression levels of acrA, mdtE, mdtF, marA, soxS, fisF, ompF, and ompC were increased in all tested mcr-1-positive E. coli. The expression of mdfA, ydhE, acrE, tolC, and dsrA was increased in four, seven, four, five, and four strains, respectively, and the expression of evgA was reduced in all tested mcr-1-positive E. coli. The results indicated that upregulation of the expression of efflux pump-related genes could be used to explain the multidrug resistance of mcr-1-positive E. coli.
Figure 4. Relative expression levels of efflux pumps, porins, and regulators. The different colors indicate the different expression levels.
As shown in Figure 5, among the 56 mcr-1-positive E. coli strains, 28 (50.00%) strains showed significantly increased ability of biofilm formation compared with the E. coli ATCC 25922 (p < 0.05 or p < 0.01), and two (3.57%) strains showed significantly decreased ability of biofilm formation (p < 0.05). However, the remaining strains (26/56, 46.43%) showed no significant changes in their ability of biofilm formation.
Figure 5. Biofilm formation ability of mcr-1-positive E. coli; the red indicates increased biofilm formation ability, and green indicates decreased biofilm formation ability compared with E. coli ATCC 25922. ∗p < 0.05; $p < 0.01.
The transferability of mcr-1 and conjugation frequencies are exhibited in Table 3. Among 30 representative mcr-1-positive E. coli, majority of the strains (n = 26) were capable of transferring mcr-1 to the recipient rifampicin-resistant E. coli EC600. The conjugation frequencies of the isolates lay between 2.68 × 10–6 and 3.73 × 10–3. The detected plasmid replicon types in the transconjugants included IncI2 (n = 8), IncX4 (n = 5), IncHI2 (n = 3), IncN (n = 3), and IncP (n = 1).
Table 3. MLST, transferability, conjugation efficiencies, and plasmid replicon types of 30 mcr-1-positive E. coli.
The combinations of IncN/IncX4 (n = 2), IncP/IncHI2 (n = 2), and IncI2/IncX4/IncHI2 (n = 1) were detected, indicating some transconjugants harbored several replicon types.
As shown in the growth curves of Figure 6A, compared with the recipient (EC600), the growth rates at growth phase and cell densities at stationary phase were decreased slightly in mcr-1-positive E. coli transconjugants. The results of the in vitro competition experiment (Figure 6B) showed that the relative fitness values of all selected mcr-1-positive E. coli transconjugants were below 1. These results revealed that the acquisition of mcr-1-bearing plasmid could place an energy burden on the bacterial host and incur fitness cost. A total of five mcr-1-positive E. coli transconjugants were randomly selected and were passaged daily for 10 days in the absence of antibiotic selection. The results (Figure 6C) showed that mcr-1 could be detected in transconjugants after a series of passages, suggesting that the plasmid harboring mcr-1 remains stable in the hosts.
Figure 6. (A) Growth kinetics of transconjugants harboring mcr-1; (B) relative fitness of transconjugants harboring mcr-1, a relative fitness of 1 indicates that the transcoujugants undergo no fitness cost; (C) stability of plasmid harboring mcr-1 in transconjugants.
In the 1960s, several countries permitted the use of colistin in food animal production (Rhouma et al., 2016). However, the regular use of colistin in food animal is recognized as one of the major contributors to the emergence of colistin-resistant Enterobacteriaceae in humans (Maamar et al., 2018). The discovery of a novel stable plasmid-mediated gene mcr-1 in E. coli contributed to our understanding of potential colistin resistance transmission between animals and humans (Liu et al., 2016). Moreover, livestock and poultry have been described as the major reservoir for colistin resistance (Rhouma et al., 2016). A survey has been performed to investigate the prevalence of colistin resistance in E. coli isolated from farms in different geographic areas of China during 2013–2014, which revealed that colistin resistance rates in E. coli from pigs, chickens, and cattle were 26.5, 14.0, and 0.9%, respectively (Zhang et al., 2019). The results demonstrated that colistin resistance was extremely serious in food animals, particularly in pigs.
In this study, E. coli strains isolated from swine farms in northeastern China showed significantly higher frequency of colistin resistance (52.5%). This result supports a previous finding that colistin resistance in E. coli occurred widely in pigs (54.25%) in intensive breeding farms of Jiangsu Province from 2015 to 2016 (Zhang et al., 2019). The high frequency of colistin resistance in the E. coli isolates recovered from food production animals could be explained by the increasing amount of colistin administrated in animal husbandry in the past few years, especially in swine (Zhang et al., 2019). It has been reported that colistin was used in massive quantities in the swine industry for the treatment of gastrointestinal disease worldwide, including France, Belgium, Spain, Austria, Germany, and China (Rhouma et al., 2016). Moreover, the amount of colistin used in agriculture was 11,942 tons per year by the end of 2015 in China, which was predominant all over the world (Liu et al., 2016).
The rapid horizontal spread of mcr-1 by plasmids is one of the major reasons for the increasing prevalence of colistin resistance. Several studies have reported that many countries and regions found the presence of GNB carrying mcr-1 in humans, animals, and the environment (Fernandes et al., 2016; Hadjadj et al., 2017). In this study, 56 (53.33%) E. coli strains were positive for mcr-1 among 105 colistin-resistant E. coli isolated from swine farms. Similar to our result, a surveillance of colistin resistance performed in Jiangsu Province revealed that the mcr-1 prevalence was 68.86% in pigs (Zhang et al., 2019). A previous study showed a high mcr-1-positive rate (79.2%) in swine-origin E. coli isolated from nine provinces in China. Further testing showed that most mcr-1-positive bacteria were identified as E. coli, demonstrating that E. coli was the predominant bacterial host of the mcr-1 gene (Zhang et al., 2018). With the purpose of promoting growth, colistin had been widely used as a feed additive in farms for many years in China before 2017. The excessive use of colistin potentially increases the selection pressure which can promote the spread of mcr-1, finally leading to an exceedingly high prevalence of mcr-1 in food animals (Tong et al., 2018). Fortunately, the Chinese government has banned the use of colistin as food additive for growth promotion in farms since April 1, 2017.
It has been reported that plasmids harboring mcr-1 usually carry other resistance genes, encoded for aminoglycosides, quinolones, etc. (Rozwandowicz et al., 2018). Furthermore, the resistance genes can be horizontally transferred via plasmids, which is recognized as one of the major reasons for the extensive resistance profiles of the mcr-1-positive bacteria (Fan et al., 2020). In the present study, mcr-1-positive E. coli isolates displayed high resistance rates to antibiotics that are commonly used in veterinary medicine, including florfenicol, doxycycline, ciprofloxacin, chloramphenicol, streptomycin, gentamicin, kanamycin, and ampicillin. They showed low rates of resistance to some important antibiotics in human medicine, such as tigecycline, nitrofurantoin, ertapenem, meropenem, and imipenem. The usage of different antibiotics may lead to various resistance profiles, and antibiotics commonly used in food animals can form selection pressure on bacteria to become resistant. The antimicrobial resistance profiles of mcr-1-positive E. coli in this study were similar to the large-scale investigation performed in China (Huang et al., 2017).
The emergence of a superbug resistant to all last-line antibiotics (carbopenems, colistin, and tigecycline) was rare in swine farms, and a similar result was also obtained in a previous study about E. coli of food-animal origin in China (Tong et al., 2018). However, co-carriage of mcr-1 and blaNDM–5 was detected in this study which has been found in Enterobacteriaceae isolated from animals and humans (Du et al., 2016; Paveenkittiporn et al., 2020). Notably, the one isolate harboring mcr-1 and blaNDM–5 belongs to phylogroup D, indicating the possibility of two isolates being pathogenic E. coli responsible for extraintestinal infection (Khanawapee et al., 2020). The extensive resistance profiles of mcr-1-positive E. coli could be explained by the high frequencies of the presence of other resistance genes, including blaTEM, blaCTX–M, aac3-IV, tet(A), tet(M), floR, sul1, sul2, and oqxAB. Multidrug efflux pump in bacteria is a ubiquitous mechanism leading to cross-resistance with several antimicrobial agents and can increase the resistance level by interacting synergistically with other resistance mechanisms (Baron and Rolain, 2018). It has been demonstrated that β-lactams, fluoroquinolones, tetracycline, and chloramphenicol could be the substrates of efflux pumps. In the present study, the relative expression levels of some genes associated with multidrug efflux pumps were increased in mcr-1-positive E. coli. When the same plasmid carries mcr-1 and various resistance genes, the frequent use of other antibiotics, such as aminoglycosides, tetracyclines, or sulfonamides, also can promote the selection of colistin resistance (Sabine et al., 2017). Therefore, we cannot ignore the effect of the high prevalence of mcr-1 in swine-origin E. coli, increasing the number of multidrug-resistant bacteria.
Biofilm formation is commonly relied on regarding the cooperation of different bacterial strains and species for a common goal. Biofilm shows as bacteria form dense surface-associated communities, which could allow them to prosper and protect each other; bacteria within a biofilm showed enhanced tolerance to harsh environmental conditions and increased antibiotic resistance (Rabin et al., 2015). It has been suspected that biofilm could play a significant role in the persistence of bacterial infections in both clinical and food industries (Bridier et al., 2015). Unfortunately, most of the mcr-1-positive E. coli isolated from swine in this study were biofilm producers. The result suggested that biofilm formation is one of the strategies used by these bacteria against antibiotics and environmental stress. The prevalence of biofilm in swine-origin mcr-1-positive E. coli maybe associated with the excessive use of antibiotics in swine farms. A similar idea has been reported that the improper use of antibiotics may select for and further accumulate bacteria with a strong or moderate biofilm formation ability (Ma et al., 2020).
Many studies have demonstrated that the mobile genetic element of mcr-1 could promote colistin resistance dissemination between animals and humans and result in the high prevalence of mcr-1 worldwide (Liu et al., 2016; Wang et al., 2018). In this study, the transferability and the dissemination risk of mcr-1 were assessed among 30 representative strains. The results were in line with previous findings which showed that majority of the reported mcr encoded by plasmids were transferable (Wang et al., 2018). Among the reported mcr-1, majority of them were mediated by plasmids, but there were some studies that reported the emergence of mcr-1 on chromosome, or the plasmids harboring mcr-1 were inconjugative, which could lead to failure of horizontal transfer (Lu et al., 2019).
The plasmid replicon types IncI2 (n = 9), IncX4 (n = 5), IncHI2 (n = 3), IncN (n = 3), and IncP (n = 1) were detected in the transconjugants. Among the already reported plasmids harboring the mcr-1 gene, they belong to different replicon types, including IncI2, IncHI1, IncHI2, IncFIB, IncFII, IncP, IncX4, and IncY (Huang H. et al., 2020). With the use of colistin in clinical settings, the type of plasmids carrying mcr-1 became more diverse which was reported by a survey performed in China to investigate the carriage of mcr-1 among hospital patients, suggesting that colistin administration could promote the dissemination of diverse resistance plasmids among E. coli isolates (Huang H. et al., 2020). Moreover, the combinations of IncN/IncX4 (n = 2), IncP/IncHI2 (n = 2), and IncI2/IncX4/IncHI2 (n = 1) were detected, indicating that some transconjugants harbored several replicon types. This could be explained by the co-transfer of mcr-1 and other resistance genes. The results of growth assay analysis and in vitro competition experiment showed that the acquisition of mcr-1-harboring plasmids could reduce the fitness of the bacterial host, but plasmid stability testing revealed that mcr-1-harboring plasmids remained stable in the recipient strain, which was consistent with a previous study (He et al., 2017). These results indicated that bacterial fitness cost could not cause plasmid loss.
The genetic relationship of the mcr-1-positive E. coli isolates was analyzed by MLST, which revealed that the most common ST was ST10, followed by ST48, ST20, ST3944, ST772, ST5229, ST617, ST410, ST93, ST898, and ST1421, and then by single ST isolates. More importantly, three predominant STs (ST10, ST48, and ST617) identified in the current study are different by one or two alleles and they correspond to clonal complex CC10. This result supported the previous finding that the most prevalent ST was ST10 in an investigation of mcr-positive E. coli isolated from diseased food animals in Europe (Garch et al., 2016). As we all know, ST10 is described as one of the predominant E. coli lineages, which is widespread among humans and animals, especially in livestock animals (Manges et al., 2015). By phylogenetic group classification, a total of 46 (82.14%) mcr-1-positive E. coli belong to groups A and B1 in this study, indicating that most of the swine-origin mcr-1-positive E. coli were non-pathogenic or commensal strains, consistent with a previous study (Khanawapee et al., 2020).
The findings of this study demonstrated the high prevalence of mcr-1 in swine farms in northeastern China. mcr-1-positive E. coli showed extensive antimicrobial resistance profiles with the presence of additional resistance genes, increased expression of efflux pump-associated genes, and increased biofilm formation ability. The high diversity of clones and the results of the conjugation experiment underlined the evidence for the horizontal transfer of mcr-1. The mcr-1-harboring plasmids could reduce the fitness of bacterial hosts but remained stable in the recipient strain. Due to the last-line role of colistin in the treatment option against infection caused by MDR GNB, and livestock production has been described as one of the greatest reservoirs of mcr-1, careful monitoring of the spread of mcr-1 gene in food animals is urgently needed, particularly in swine.
The original contributions presented in the study are included in the article/supplementary material, further inquiries can be directed to the corresponding author/s.
XZ, YY, and PC conceived and designed the experiments. PC, SC, HL, XL, JS, FL, and MI collected the samples and performed the experiments. PC and MI analyzed the data and wrote the manuscript. All authors have read and agreed to the published version of the manuscript.
This work was supported by the National Science and Technology Project and National 13th 5-Year Science and Technology Project under Grant: 2018YFD0500306.
The authors declare that the research was conducted in the absence of any commercial or financial relationships that could be construed as a potential conflict of interest.
We would like to thank the six swine farms located in Heilongjiang, Jilin, and Liaoning provinces for giving permission and help during the collection of fecal samples.
Baron, S. A., and Rolain, J. M. (2018). Efflux pump inhibitor CCCP to rescue colistin susceptibility in mcr-1 plasmid-mediated colistin-resistant strains and Gram-negative bacteria. J. Antimicrob. Chemother. 73, 1862–1871. doi: 10.1093/jac/dky134
Bridier, A., Sanchez-Vizuete, P., Guilbaud, M., Piard, J. C., Naïtali, M., and Briandet, R. (2015). Biofilm-associated persistence of food-borne pathogens. Food Microbiol. 45, 167–178. doi: 10.1016/j.fm.2014.04.015
Carattoli, A., Bertini, A., Villa, L., Falbo, V., Hopkins, K. L., and Threlfall, E. J. (2005). Identification of plasmids by PCR-based replicon typing. J. Microbiol. Methods 63, 219–228. doi: 10.1016/j.mimet.2005.03.018
Ciesielczuk, H., Hornsey, M., Choi, V., Woodford, N., and Wareham, D. (2013). Development and evaluation of a multiplex PCR for eight plasmid-mediated quinolone resistance determinants. J. Med. Microbiol. 62, 1823–1827. doi: 10.1099/jmm.0.064428-0
Clermont, O., Bonacorsi, S., and Bingen, E. (2000). Rapid and simple determination of the Escherichia coli phylogenetic group. Appl. Environ. Microbiol. 66, 4555–4558. doi: 10.1128/aem.66.10.4555-4558.2000
Dallenne, C., Da Costa, A., Decre, D., Favier, C., and Arlet, G. (2010). Development of a set of multiplex PCR assays for the detection of genes encoding important beta-lactamases in Enterobacteriaceae. J. Antimicrob. Chemother. 65, 490–495. doi: 10.1093/jac/dkp498
Doyle, D., Peirano, G., Lascols, C., Lloyd, T., Church, D. L., and Pitout, J. D. (2012). Laboratory detection of Enterobacteriaceae that produce carbapenemases. J. Clin. Microbiol. 50, 3877–3880. doi: 10.1128/JCM.02117-12
Du, H., Chen, L., Tang, Y.-W., and Kreiswirth, B. N. (2016). Emergence of the mcr-1 colistin resistance gene in carbapenem-resistant Enterobacteriaceae. Lancet Infect. Dis. 16, 287–288. doi: 10.1016/S1473-3099(16)00056-6
Fairbrother, J. M., Nadeau, E., and Gyles, C. L. (2005). Escherichia coli in postweaning diarrhea in pigs: an update on bacterial types, pathogenesis, and prevention strategies. Anim. Health Res. Rev. 6, 17–39. doi: 10.1079/ahr2005105
Falagas, M. E., and Kasiakou, S. K. (2005). Colistin: the revival of polymyxins for the management of multidrug-resistant gram-negative bacterial infections. Clin. Infect. Dis. 40, 1333–1341. doi: 10.1086/429323
Fan, R., Li, C., Duan, R., Qin, S., Liang, J. R., Xiao, M., et al. (2020). Retrospective screening and analysis of mcr-1 and blaNDM in gram-negative bacteria in China, 2010–2019. Front. Microbiol. 11:121. doi: 10.3389/fmicb.2020.00121
Fernandes, M. R., Moura, Q., Sartori, L., Silva, K. C., Cunha, M. P., Esposito, F., et al. (2016). Silent dissemination of colistin-resistant Escherichia coli in South America could contribute to the global spread of the mcr-1 gene. Euro Surveill. 21:30214. doi: 10.2807/1560-7917.ES.2016.21.17.30214
Garch, F. E., Sauget, M., Hocquet, D., Lechaudee, D., and Bertrand, X. (2016). mcr-1 is borne by highly diverse Escherichia coli isolates since 2004 in food-producing animals in Europe. Clin. Microbiol. Infect. 23, 51.e1–51.e4. doi: 10.1016/j.cmi.2016.08.033
Graham, J. P., Evans, S. L., Price, L. B., and Silbergeld, E. K. (2009). Fate of antimicrobial-resistant enterococci and staphylococci and resistance determinants in stored poultry litter. Environ. Res. 109, 682–689. doi: 10.1016/j.envres.2009.05.005
Hadjadj, L., Riziki, T., Zhu, Y., Li, J., Diene, S. M., and Rolain, J. M. (2017). Study of mcr-1 gene-mediated colistin resistance in Enterobacteriaceae isolated from humans and animals in different countries. Genes (Basel) 8:394. doi: 10.3390/genes8120394
Hammerum, A. M., Sandvang, D., Andersen, S. R., Seyfarth, A. M., Porsbo, L. J., Frimodt-Molller, N., et al. (2006). Detection of sul1, sul2 and sul3 in sulphonamide resistant Escherichia coli isolates obtained from healthy humans, pork and pigs in Denmark. Int. J. Food Microbiol. 106, 235–237. doi: 10.1016/j.ijfoodmicro.2005.06.023
He, T., Wei, R. C., Zhang, L. L., Sun, L. C., Pang, M. D., Wang, R., et al. (2017). Characterization of NDM-5-positive extensively resistant Escherichia coli isolates from dairy cows. Vet. Microbiol. 207, 153–158. doi: 10.1016/j.vetmic.2017.06.010
Huang, H., Dong, N., Shu, L., Lu, J., Sun, Q. L., Chan, E. W. C., et al. (2020). Colistin-resistance gene mcr in clinical carbapenem-resistant Enterobacteriaceae strains in China, 2014-2019. Emerg. Microbes Infect. 9, 237–245. doi: 10.1080/22221751.2020.1717380
Huang, W., Cao, Z., Yao, Q. C., Ji, Q., Zhang, J., and Li, Y. F. (2020). Mitochondrial damage are involved in Aflatoxin B(1)-induced testicular damage and spermatogenesis disorder in mice. Sci. Total Environ. 701:135077. doi: 10.1016/j.scitotenv.2019.135077
Huang, X. H., Yu, L. F., Chen, X. J., Zhi, C. P., Yao, X., Liu, Y. Y., et al. (2017). High prevalence of colistin resistance and mcr-1 gene in Escherichia coli isolated from food animals in China. Front. Microbiol. 8:562. doi: 10.3389/fmicb.2017.00562
Johnson, T. J., Bielak, E. M., Fortini, D., Hansen, L. H., Hasman, H., Debroy, C., et al. (2012). Expansion of the IncX plasmid family for improved identification and typing of novel plasmids in drug-resistant Enterobacteriaceae. Plasmid 68, 43–50. doi: 10.1016/j.plasmid.2012.03.001
Kempf, I., Fleury, M. A., Drider, D., Bruneau, M., Sanders, P., Chauvin, C., et al. (2013). What do we know about resistance to colistin in Enterobacteriaceae in avian and pig production in Europe? Int. J. Antimicrobial. Agents 42, 379–383. doi: 10.1016/j.ijantimicag.2013.06.012
Khanawapee, A., Kerdsin, A., Chopjitt, P., Boueroy, P., Hatrongjit, R., Akeda, Y., et al. (2020). Distribution and molecular characterization of Escherichia coli harboring mcr genes isolated from slaughtered pigs in Thailand. Microb. Drug Resist. doi: 10.1089/mdr.2020.0242 [Epub ahead of print].
Kumar, S., Stecher, G., Li, M., Knyaz, C., and Tamura, K. (2018). MEGA X: molecular evolutionary genetics analysis across computing platforms. Mol. Biol. Evol. 35, 1547–1549. doi: 10.1093/molbev/msy096
Landman, D., Georgescu, C., and Martin, D. A. (2008). Polymyxins revisited. Clin. Microbiol. Rev. 21, 449–465. doi: 10.1128/CMR.00006-08
Lee, S. Y., Park, Y. J., Yu, J. K., Jung, S., Kim, Y., Jeong, S. H., et al. (2012). Prevalence of acquired fosfomycin resistance among extended-spectrum β-lactamase-producing Escherichia coli and Klebsiella pneumoniae clinical isolates in Korea and IS26-composite transposon surrounding fosA3. J. Antimicrob. Chemother. 67, 2843–2847. doi: 10.1093/jac/dks319
Li, B. B., Zhang, Y., Wei, J. C., Shao, D. H., Liu, K., Shi, Y. Y., et al. (2015). Characterization of a novel small plasmid carrying the florfenicol resistance gene floR in Haemophilus parasuis. J. Antimicrob. Chemother. 70, 3159–3161. doi: 10.1093/jac/dkv230
Liu, Y. Y., Wang, Y., Walsh, T. R., Yi, L. X., Zhang, R., Spencer, J., et al. (2016). Emergence of plasmid-mediated colistin resistance mechanism MCR-1 in animals and human beings in China: a microbiological and molecular biological study. Lancet Infect. Dis. 16, 161–168. doi: 10.1016/S1473-3099(15)00424-7
Long, D., Zhu, L. L., Du, F. L., Xiang, T. X., Wan, L. G., Wei, D. D., et al. (2019). Phenotypical profile and global transcriptomic profile of Hypervirulent Klebsiella pneumoniae due to carbapenemase-encoding plasmid acquisition. BMC Genomics 20:480. doi: 10.1186/s12864-019-5705-2
Lu, X., Xiao, X., Liu, Y., Li, Y., Li, R., and Wang, Z. Q. (2019). Chromosome-mediated mcr-1 in Escherichia coli strain L73 from a goose. Int. J. Antimicrob. Agents 54, 99–101. doi: 10.1016/j.ijantimicag.2019.03.003
Ma, Y. B., Xu, X. B., Gao, Y., Zhan, Z. Q., Xu, C. G., Qu, X. Y., et al. (2020). Antimicrobial resistance and molecular characterization of Salmonella enterica serovar Corvallis isolated from human patients and animal source foods in China. Int. J. Food Microbiol. 335:108859. doi: 10.1016/j.ijfoodmicro.2020.108859
Maamar, E., Alonso, C. A., Hamzaoui, Z., Dakhli, N., Abbassi, M. S., Ferjani, S., et al. (2018). Emergence of plasmid-mediated colistin-resistance in CMY-2-producing Escherichia coli of lineage ST2197 in a Tunisian poultry farm. Int. J. Food Microbiol. 269, 60–63. doi: 10.1016/j.ijfoodmicro.2018.01.017
Manges, A. R., Harel, J., Masson, L., Edens, T. J., Portt, A., Reid-Smith, R. J., et al. (2015). Multilocus sequence typing and virulence gene profiles associated with Escherichia coli from human and animal sources. Foodborne Pathog. Dis. 12, 302–310. doi: 10.1089/fpd.2014.1860
Ng, L. K., Martin, I., Alfa, M., and Mulvey, M. (2001). Multiplex PCR for the detection of tetracycline resistant genes. Mol. Cell. Probes 15, 209–215. doi: 10.1006/mcpr.2001.0363
Paveenkittiporn, W., Kamjumphol, W., Ungcharoen, R., and Kerdsin, A. (2020). Whole-Genome sequencing of clinically isolated carbapenem-resistant enterobacterales harboring mcr genes in Thailand, 2016-2019. Front. Microbiol. 11:586368. doi: 10.3389/fmicb.2020.586368
Rabin, N., Zheng, Y., Opoku-Temeng, C., Du, Y., Bonsu, E., and Sintim, H. O. (2015). Biofilm formation mechanisms and targets for developing antibiofilm agents. Future Med. Chem. 7, 493–512. doi: 10.4155/fmc.15.6
Rhouma, M., Beaudry, F., and Letellier, A. (2016). Resistance to colistin : what is the fate for this antibiotic in pig production? Int. J. Antimicrob. Agents 48, 119–126. doi: 10.1016/j.ijantimicag.2016.04.008
Rozwandowicz, M., Brouwer, M. S. M., Fischer, J., Wagenaar, J. A., Gonzalez-Zorn, B., Guerra, B., et al. (2018). Plasmids carrying antimicrobial resistance genes in Enterobacteriaceae. J. Antimicrob. Chemother. 73, 1121–1137. doi: 10.1093/jac/dkx488
Sabine, D., Le, D. L., Eric, J., Patrick, F., Djamel, D., and Isabelle, K. (2017). Characterization of colistin-resistant Escherichia coli isolated from diseased pigs in France. Front. Microbiol. 8:2278. doi: 10.3389/fmicb.2017.02278
Seurinck, S., Verstraete, W., and Siciliano, S. D. (2003). Use of 16S-23S rRNA intergenic spacer region PCR and repetitive extragenic palindromic PCR analyses of Escherichia coli isolates to identify nonpoint fecal sources. Appl. Environ. Microbiol. 69, 4942–4950. doi: 10.1128/aem.69.8.4942-4950.2003
Shi, X. M., Li, Y. M., Yang, Y. Y., Shen, Z. Q., Wu, Y. N., and Wang, S. L. (2020). Global impact of mcr-1-positive Enterobacteriaceae bacteria on “one health”. Crit. Rev. Microbiol. 46, 565–577. doi: 10.1080/1040841x.2020.1812510
Sota, M., Yano, H., Hughes, J. M., Daughdrill, G. W., Abdo, Z., Forney, L. J., et al. (2010). Shifts in the host range of a promiscuous plasmid through parallel evolution of its replication initiation protein. ISME J. 4, 1568–1580. doi: 10.1038/ismej.2010.72
Srinivasan, V., Gillespie, B. E., Lewis, M. J., Nguyen, L. T., Headrick, S. I., Schukken, Y. H., et al. (2007). Phenotypic and genotypic antimicrobial resistance patterns of Escherichia coli isolated from dairy cows with mastitis. Vet. Microbiol. 124, 319–328. doi: 10.1016/j.vetmic.2007.04.040
Tartof, S. Y., Solberg, O. D., Manges, A. R., and Riley, L. W. (2005). Analysis of a uropathogenic Escherichia coli clonal group by multilocus sequence typing. J. Clin. Microbiol. 43, 5860–5864. doi: 10.1128/jcm.43.12.5860-5864.2005
Teh, K. H., Flint, S., and French, N. (2010). Biofilm formation by Campylobacter jejuni in controlled mixed-microbial populations. Int. J. Food Microbiol. 143, 118–124. doi: 10.1016/j.ijfoodmicro.2010.07.037
Tong, H. X., Liu, J. X., Yao, X. H., Jia, H. Y., Wei, J. C., Shao, D. H., et al. (2018). High carriage rate of mcr-1 and antimicrobial resistance profiles of mcr-1-positive Escherichia coli isolates in swine faecal samples collected from eighteen provinces in China. Vet. Microbiol. 225, 53–57. doi: 10.1016/j.vetmic.2018.09.018
Vinué, L., Corcoran, M. A., Hooper, D. C., and Jacoby, G. A. (2015). Mutations That Enhance the Ciprofloxacin Resistance of Escherichia coli withqnrA1. Antimicrob. Agents Chemother. 60, 1537–1545. doi: 10.1128/AAC.02167-15
Wang, R. B., Dorp, L. V., Shaw, L. P., Bradley, P., and Balloux, F. (2018). The global distribution and spread of the mobilized colistin resistance gene mcr-1. Nat. Commun. 9:1179. doi: 10.1038/s41467-018-03205-z
Worthington, R. J., and Melander, C. (2013). Combination approaches to combat multidrug-resistant bacteria. Trends Biotechnol. 31, 177–184. doi: 10.1016/j.tibtech.2012.12.006
Yeganeh Sefidan, F., Mohammadzadeh-Asl, Y., and Ghotaslou, R. (2019). High-Level Resistance to Aminoglycosides due to 16S rRNA Methylation in Enterobacteriaceae Isolates. Microb. Drug Resist. 25, 1261–1265. doi: 10.1089/mdr.2018.0171
You, Y., and Silbergeld, E. K. (2014). Learning from agriculture: understanding low-dose antimicrobials as drivers of resistome expansion. Front. Microbiol. 5:284. doi: 10.3389/fmicb.2014.00284
Zhang, J. L., Chen, L., Wang, J. W., Yassin, A. K., Butaye, P., Kelly, P., et al. (2018). Molecular detection of colistin resistance genes (mcr-1, mcr-2 and mcr-3) in nasal/oropharyngeal and anal/cloacal swabs from pigs and poultry. Sci. Rep. 8:3705. doi: 10.1038/s41598-018-22084-4
Keywords: colistin resistance, mcr-1 gene, swine, Escherichia coli, prevalence, characteristics
Citation: Cheng P, Yang Y, Cao S, Liu H, Li X, Sun J, Li F, Ishfaq M and Zhang X (2021) Prevalence and Characteristic of Swine-Origin mcr-1-Positive Escherichia coli in Northeastern China. Front. Microbiol. 12:712707. doi: 10.3389/fmicb.2021.712707
Received: 21 May 2021; Accepted: 23 June 2021;
Published: 20 July 2021.
Edited by:
Azucena Mora Gutiérrez, University of Santiago de Compostela, SpainReviewed by:
Xiang-Dang Du, Henan Agricultural University, ChinaCopyright © 2021 Cheng, Yang, Cao, Liu, Li, Sun, Li, Ishfaq and Zhang. This is an open-access article distributed under the terms of the Creative Commons Attribution License (CC BY). The use, distribution or reproduction in other forums is permitted, provided the original author(s) and the copyright owner(s) are credited and that the original publication in this journal is cited, in accordance with accepted academic practice. No use, distribution or reproduction is permitted which does not comply with these terms.
*Correspondence: Xiuying Zhang, emhhbmd4aXV5aW5nQG5lYXUuZWR1LmNu
Disclaimer: All claims expressed in this article are solely those of the authors and do not necessarily represent those of their affiliated organizations, or those of the publisher, the editors and the reviewers. Any product that may be evaluated in this article or claim that may be made by its manufacturer is not guaranteed or endorsed by the publisher.
Research integrity at Frontiers
Learn more about the work of our research integrity team to safeguard the quality of each article we publish.