- 1Instituto de Investigaciones Químico-Biológicas, Universidad Michoacana de San Nicolás de Hidalgo, Morelia, Mexico
- 2Instituto Tecnológico de Sonora, Ciudad Obregón, Mexico
In recent decades, various bacterial species have been characterized as biocontrol agents for plant crop diseases; however, only a few genera have been predominantly reported in the literature. Therefore, the identification of new antagonists against phytopathogens is essential for boosting sustainable food production systems. In this study, we evaluated the role of strain SER3 from the recently discovered Rouxiella badensis as a biocontrol agent. SER3 was isolated from the phyllosphere of decaying strawberry fruit (Fragaria × ananassa) and showed different grades of antagonism against 20 fungal pathogens of berries, based on confrontation assays, due to the action of its diffusible and volatile compounds. These fungal pathogens were isolated from decayed strawberry, blackberry, and blueberry fruit and were characterized through internal transcribed spacer (ITS) sequencing and homology searches, exhibiting similarity with well-known postharvest pathogens such as Botrytis, Fusarium, Geotrichum, Mucor, Penicillium, Alternaria, and Botryosphaeria. Koch’s postulates were confirmed for most pathogens by reinfecting berry fruit. SER3 showed good capacity to inhibit the growth of Botrytis cinerea and Fusarium brachygibbosum in strawberry fruit, affecting mycelial development. To gain better understanding of the genetic and metabolic capacities of the SER3 strain, its draft genome was determined and was found to comprise a single chromosome of 5.08 Mb, 52.8% G + C content, and 4,545 protein-coding genes. Phylogenetic analysis indicated that the SER3 strain is affiliated with the R. badensis species, with an average nucleotide identity >96% and a genome-to-genome distance >70%. A comparison of the genomic properties of R. badensis SER3 and other close bacterial relatives showed several genes with potential functions in biocontrol activities, such as those encoding siderophores, non-ribosomal peptide synthetases, and polyketide synthases. This is the first study to demonstrate a novel role of the recently discovered R. badensis species (and any other species of the genus Rouxiella) as a biocontrol agent against postharvest fungal pathogens.
Introduction
The demand for food is increasing worldwide, resulting in the requirement to produce it under eco-friendly systems to ensure food security (Allen and Prosperi, 2016). However, constant attack by fungal and oomycete phytopathogens reduces the yield and quality of crops, causing huge losses at different stages of the agricultural cycle (Dean et al., 2012; Kamoun et al., 2015). For example, Botrytis cinerea has been reported to infect more than 200 plant species and cause losses of more than €1 billion/annum globally (Romanazzi and Feliziani, 2014). Similarly, several species of the genus Fusarium, together with Botrytis, are among the top 10 pathogens worldwide that can cause serious yield losses in agriculture (Magan et al., 2010; Dean et al., 2012). Likewise, invasion by non-native species of phytopathogens owing to transportation and storage of vegetables and fruit is another factor that affects products postharvest (Fried et al., 2017).
Thus, an efficient alternative against crop infestation, which includes the use of antagonistic biological agents, has been developed to eliminate or reduce the use of pesticides in agriculture (Compant et al., 2005; Backer et al., 2018). One of the advantages of biological agents such as Trichoderma or bacteria is that they are safe and environment friendly (Elad, 2000; Wang et al., 2020). This group of beneficial microorganisms associated with plants has emerged as a viable, economical, and efficient alternative to control various pre- and postharvest diseases (Morales-Cedeño et al., 2021). Even antagonism in plant growth-promoting bacteria (PGPB) toward phytopathogens is considered an indirect mechanism to stimulate plant growth (Glick, 2012). Their mechanisms of antifungal action against fungal pathogens include the production of diffusible compounds [e.g., hydrolytic enzymes, siderophores, lipopeptides, phenazines, 1-aminocyclopropane-1-carboxylate (ACC) deaminase] or antibiotics and volatiles compounds (e.g., dimethyl disulfide, hydrogen cyanide, and others) (Hernández-León et al., 2015; Khan et al., 2018; Rojas-Solís et al., 2018). Multiple species of PGPB have been isolated and characterized based on their antagonism toward phytopathogens, including Pseudomonas spp. and Bacillus spp., among few other genera that are predominantly reported (Höfte and Altier, 2010; Santoyo et al., 2012; Islam et al., 2017). Thus, the search for new antagonistic bacterial species is essential to increase the possibility of developing new biofungicides for commercial application (Córdova-Albores et al., 2020).
In this study, we propose a novel ecological role for Rouxiella badensis strain SER3 as an antagonist of postharvest pathogens of berries. R. badensis, together with Rouxiella silvae, was recently proposed as a new bacterial species by Le Fléche-Matéos et al. (2017). Some genera phylogenetically close to Rouxiella, such as Serratia and Rahnella, have previously been described as antagonists and PGPB. For example, Koo and Cho (2009) isolated and characterized a strain of Serratia sp. SY5, which had the ability to stimulate the growth of maize seedlings under stressful conditions. In addition, Sun et al. (2020) observed that Rahnella aquatilis strain MEM40, isolated from the rhizosphere of a rice plant, showed plant growth promoter effects and antagonism against phytopathogens such as Magnaporthe oryzae and F. graminearum. The only report on the genus Rouxiella described its role as an inhibitor of human pathogenic bacterial growth, but this strain has not been fully characterized, and its taxonomic assignation remains at the genus level (Nam et al., 2020). The isolation of a possible R. badensis strain 70 (among other 43 isolated endophytic strains) as an antagonist of pathogenic bacteria and fungi has also been reported. However, the characterization of strain 70 was based only on a partial 16S rDNA sequence (1,023 bp); thus, elucidation of its taxonomic affiliation requires further analysis (Wang et al., 2019). Herein, we present the isolation and characterization of a novel ecological role of R. badensis as a biocontrol agent against 20 fungal phytopathogens of berries (Fragaria × ananassa, Vaccinium spp. var. Biloxi, Rubus subgenus Eubatus), also isolated and characterized in this study. Furthermore, the R. badensis SER3 genome was sequenced to support its taxonomic affiliation and mined for detecting biosynthetic gene clusters that could be involved in its biocontrol capabilities.
Materials and Methods
Isolation and Characterization of Postharvest Fungal Pathogens
Endophytic fungal pathogens were isolated from berries, including strawberries (n = 33), blackberries (n = 36), and blueberries (n = 49), which were collected from commercial markets. Berry fruits were surface sterilized according to a previous study (Contreras et al., 2016). Briefly, berries were immersed in 70% ethanol for 30 s, then washed with sodium hypochlorite (NaOCI) solution (2.5% available Cl–) for 5 min, and then rinsed with ethanol (70% v/v) for 30 s. Finally, the fruits were washed five times with sterile distilled water. Aliquots of sterile distilled water used in the final rinse were cultured on plates containing nutrient agar (NA) medium (Merck). The plates were examined for bacterial growth after incubation at 28°C for 4 days. The sterilized fruits were used in decaying experiments to isolate potentially endophytic fungal pathogens. Briefly, groups of 5–10 fruits (strawberries, blackberries, and blueberries) were placed in disinfected containers, closed, and kept in the dark at room temperature. Fruit weight and firmness were measured on days 1, 5, and 10 (until the growth of fungal mycelium was detected) with an analytical balance (Benchmark Scientific, Inc., Sayreville, NJ, United States) and a penetrometer (Model GY-1, Hangzhou Scientific Instruments), respectively. Koch’s postulates of fungal endophytes were confirmed for most of the pathogens (except Trichoderma) as follows: berry fruits were sterilized as described above, placed inside sterile glass bottles, and inoculated with the spores obtained from each fungal culture (∼1 × 105 spores/ml). Mycelial growth of fungi on the fruit was visualized after 5–10 days. Further characterization was performed to confirm fungal identity.
Genomic DNA was extracted from fungal isolates as per the protocol by Mahuku (2004), followed by polymerase chain reaction analysis to amplify the intergenic spacer (ITS) regions with the following primers: ITS4 (5′-TCCTCCGCTTATTGATATGC-3′) and ITS5 (5′-GGAAGTAAAAGTCGTAACAAGG-3′) (Hernández-León et al., 2015). The amplified ITS regions of each of the 20 fungal isolates were sequenced at Macrogen, Seoul, South Korea. Sequences and most probable taxonomic affiliation were deposited in GenBank, and the accession numbers are shown in Table 1.
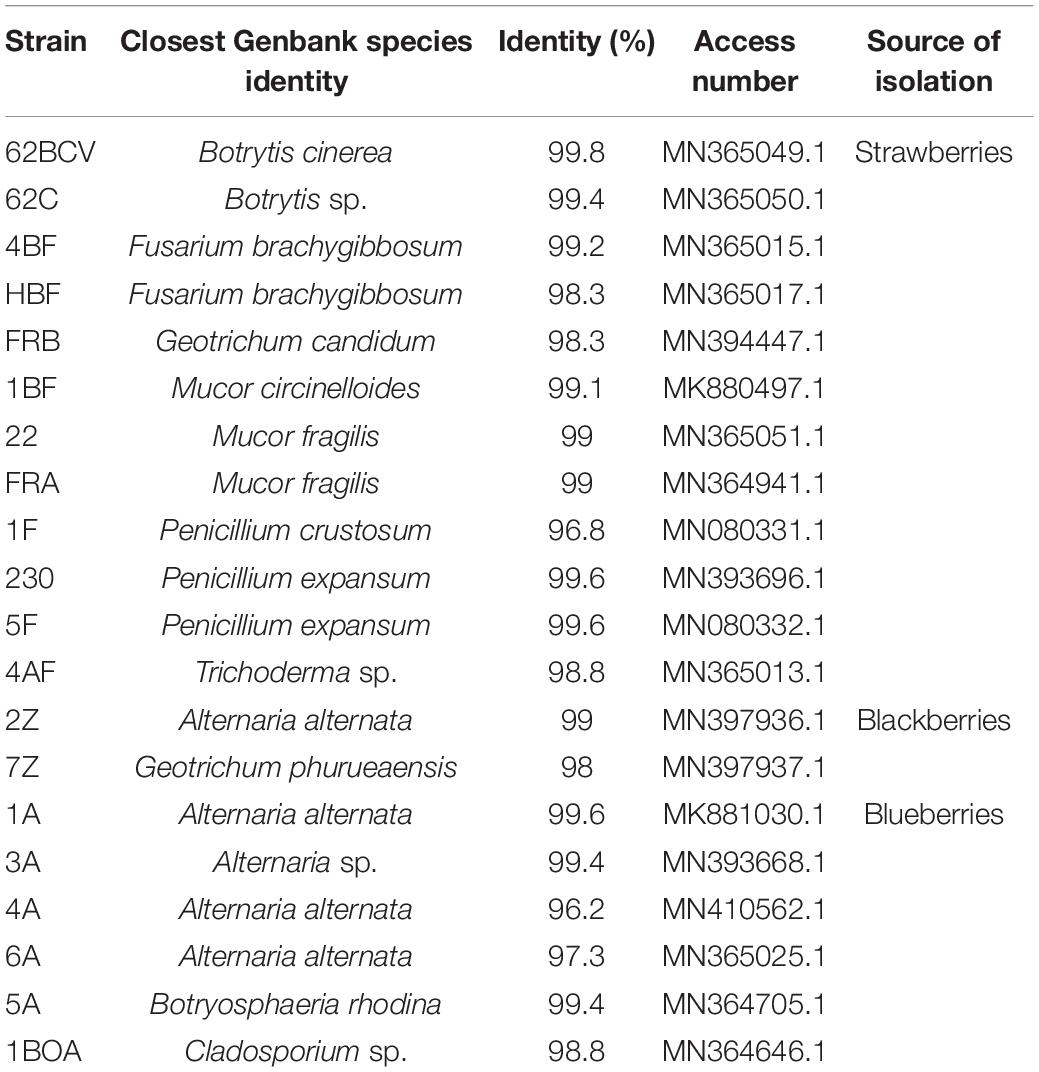
Table 1. Fungal strains isolated from strawberries, blackberries and blueberries, with the closest identity based on the ITS sequence homology searches.
Isolation of SER3 and Confrontation Bioassays
Strain SER3 was isolated from the phyllosphere of strawberry fruit and selected for antagonism against the fungal pathogen F. brachygibbosum in a prescreening assay in dual culture (Supplementary Figure 1). The strain was grown at 30°C for 24 h on NA medium and maintained at 4°C.
Fungal antagonism by strain SER3 was evaluated as previously reported for Petri dish-based bioassays (Hernández-León et al., 2015). Briefly, SER3 was streaked onto potato dextrose agar (PDA) plates in a cross shape, and then, four mycelial plugs (6-mm diameter) from each of the 20 fungal isolates were deposited in the center of each quadrant. The plates were incubated in the dark at 30°C, and the mycelial growth diameter was measured on day 6.
The antifungal effects of the volatile compounds produced by strain SER3 were also evaluated in Petri plates. SER3 [100 μl, at ∼1 × 106 colony forming units (CFU)] was inoculated on one side of the divided Petri plates, and in the other sections, mycelial plugs of each studied fungus (6-mm diameter) were inoculated. The inoculated plates were incubated, and mycelial growth was measured as described above. Both experiments were independently performed in triplicate, and the inhibition percentage was calculated using the following formula:% growth inhibition = [(Ac − Ab)/Ac] × 100, where Ac is the control mycelial area, and Ab is the mycelial area under treatment.
Fungal Growth Inhibition Bioassay on Strawberry Fruit and Microscopy Visualization
The strawberries were washed with running water and subsequently placed in a container with 70% ethanol for 1 min. The ethanol was decanted, and then, the berries were washed with 2.5% sodium hypochlorite for 1 min. This process was repeated three times, and finally, the strawberries were rinsed thrice with sterile deionized water.
Following the above procedure, strawberries were allowed to dry in a laminar flow hood, and an incision of approximately 3 mm length, width, and depth was made on each fruit with the tip of a sterile scalpel. Four treatments were performed, using the following: (i) sterile distilled water as the negative control; (ii) a mycelium plug, 7 mm in diameter, of the phytopathogen B. cinerea 62BCV or F. brachygibbosum 4BF as a positive control; and (iii) a bacterial suspension of SER3 (100 μl, ∼1 × 106 CFU) and a mycelium plug, 7 mm in diameter, of each phytopathogen; and (iv) the supernatants (100 μl) of strain SER3 obtained from nutrient broth after an overnight culture and the mycelium of the two studied phytopathogens grown for 24 h at 29°C. After treatment, the strawberries were placed in closed sterile plastic containers and maintained at room temperature for 3 days.
For microscopy visualization, strain SER3 was simultaneously striated with phytopathogenic fungi (B. cinerea 62BCV or F. brachygibbosum 4BF) in separate Petri dishes containing PDA. The bacteria were streaked on the cross-shaped dishes, and a 7-mm portion of the mycelium was deposited in the center of each quadrant, as previously mentioned. Subsequently, a mycelium sample was stained with lactophenol blue and safranin and visualized under a Velab VE-BC3 Plus optical microscope.
SER3 Genome Sequencing and Analysis
A single colony of strain SER3 was picked from a streaked NA plate (BD Bioxon), which was maintained at 30°C overnight. SER3 genomic DNA was extracted following standard protocols (Mahuku, 2004) and further purified using a Wizard® Genomic DNA Purification Kit (Promega, Fitchburg, WI, United States). The quality and quantity of the extracted DNA were evaluated with agarose gel electrophoresis and using a NanoDrop spectrophotometer (Thermo Scientific, Waltham, MA, United States), respectively. Genomic DNA from SER3 was sequenced commercially (MR DNA, Shallowater, TX, United States) by using the Illumina HiSeq technologies platform (2 × 300 bp). FastQC analysis, version 0.11.5, of the raw reads was employed to perform quality control (Andrews, 2010). Trimmomatic, version 0.32, was used to remove bases of low quality and adapter sequences (Bolger et al., 2014). Genome assembly was performed with contigs obtained through the PATRIC1 genome service and SPAdes assembler version 3.10.0 (Bankevich et al., 2012). The draft genome of SER3 was reordered according to the reference genome of Rahnella aquatilis KM12 (NCBI project accession number: ASM395610v2). PLACNETw was used to explore the presence of plasmids in the SER3 genome (Vielva et al., 2017).
Taxonomic Affiliation of Strain SER3
The 16S rRNA gene sequence was obtained from the genome and used in basic local alignment search tool (BLAST) homology searches to assign the possible taxonomic affiliation of strain SER3. After that, a genome-level approach was adopted, employing average nucleotide identity (ANI) > 95–96% (Yoon et al., 2017) and a genome-to-genome distance calculator (GGDC) > 70% (Meier-Kolthoff et al., 2013). This genome-level approach was based on strains having cutoff values for species delimitation established for the 16S rRNA gene (>98.7%) (Chun et al., 2018).
Phylogenomic Analysis of SER3
Phylogenomic relationships of R. badensis SER3 and the bacterial strains with high similarity according to ANI and GGDC values were analyzed using the REALPHY pipeline (Bertels et al., 2014). The neighbor-joining method was used for tree construction, and the nucleotide distance was measured using the Jukes–Cantor model. Furthermore, bootstrap analysis with 1,000 replications was performed.
Genome Annotation and Mining for Plant Growth-Promoting and Biocontrol Traits
The assembled genome was annotated using the Rapid Annotation of the Subsystem Technology (RAST) server2. Genome mining was performed by biosynthetic gene cluster (BGC) prediction using antiSMASH 4.0 (Blin et al., 2017) for R. badensis SER3 and other close bacterial genomes and manually inspected from the annotations generated by the RAST server3 (Aziz et al., 2008), specifically the RASTtk pipeline.
Results
Isolation and Characterization of Postharvest Phytopathogens
In this study, the decay of berries over time showed a reduction in fresh weight and fruit firmness between days 5 and 10, consistent with the appearance of decaying symptoms caused by fungal pathogens (Figure 1). Following the decay, 20 berry fungi were isolated. Figure 2 shows the morphological appearance of the isolated fungal strains. Sequencing of the ITS from the isolated fungi showed high homology with B. cinerea, Botrytis sp., F. brachygibbosum, Geotrichum candidum, Geotrichum phurueaesis, Mucor circinelloides, Mucor fragilis, Penicillium crustosum, Penicillium expansum, Trichoderma sp., Alternaria alternata, Alternaria sp., Botryosphaeria rhodina, and Cladosporium sp. (Table 1). To determine the infection rates of fungi, including those not reported as the main postharvest phytopathogens of berries, Koch’s postulates were confirmed, thus corroborating their role in postharvest fungal infections (Supplementary Figure 2).
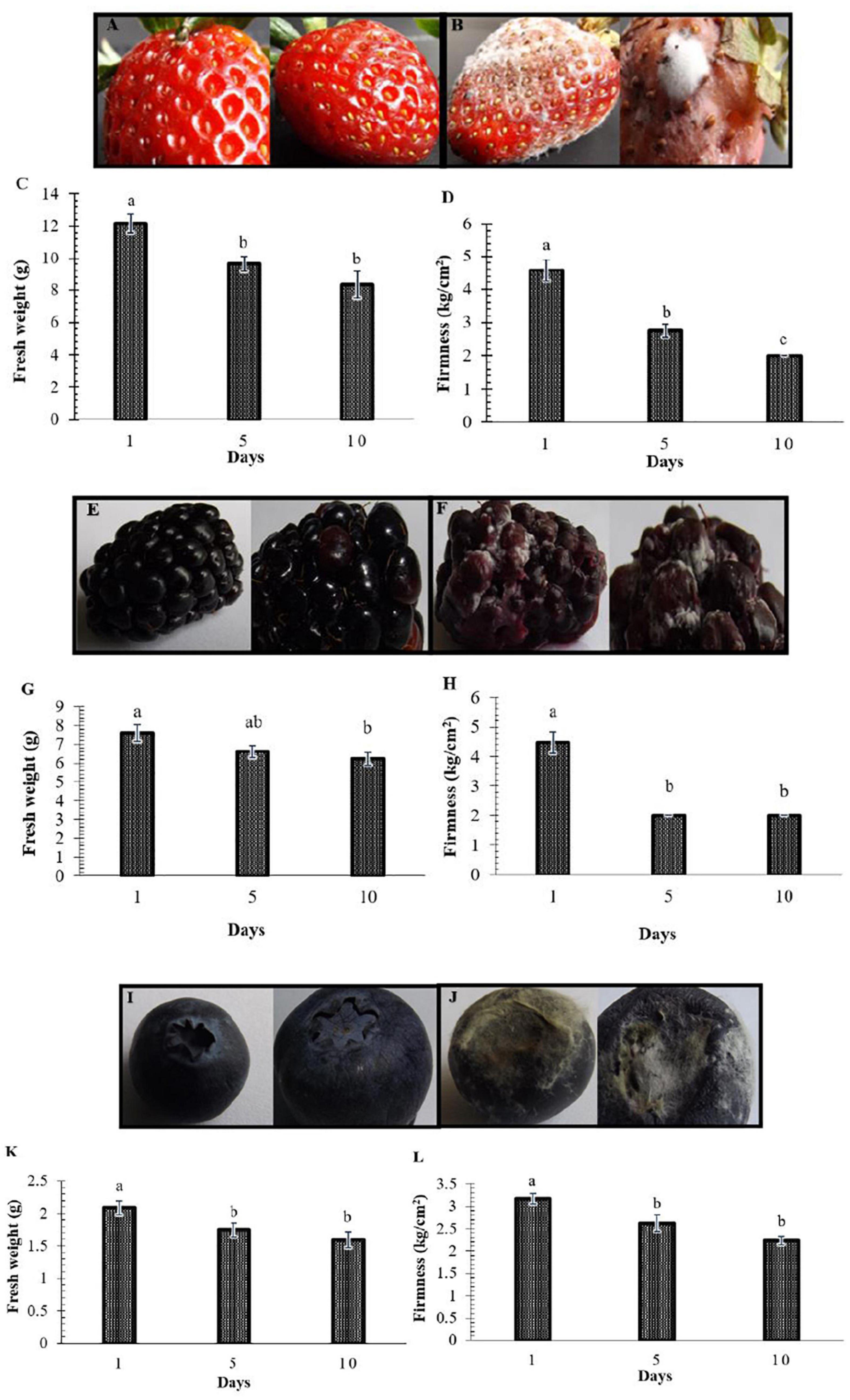
Figure 1. Postharvest decay in berries represented by loss of weight and firmness. Representative fruit are shown in Panels (A,B,E,F,I,J). (A,E,I) Fruit at the beginning of the study (control). (B,F,J) Fruit in decay on days 5 and 10. Panels (C,G,K) show the decrease in the weight of strawberries, blackberries, and blueberries, respectively. Panels (D,H,L) show the decline in firmness. Bars represent means ± standard deviation (n = 12). The letters indicate that the means differ significantly according to Duncan’s multiple range test (p < 0.05).
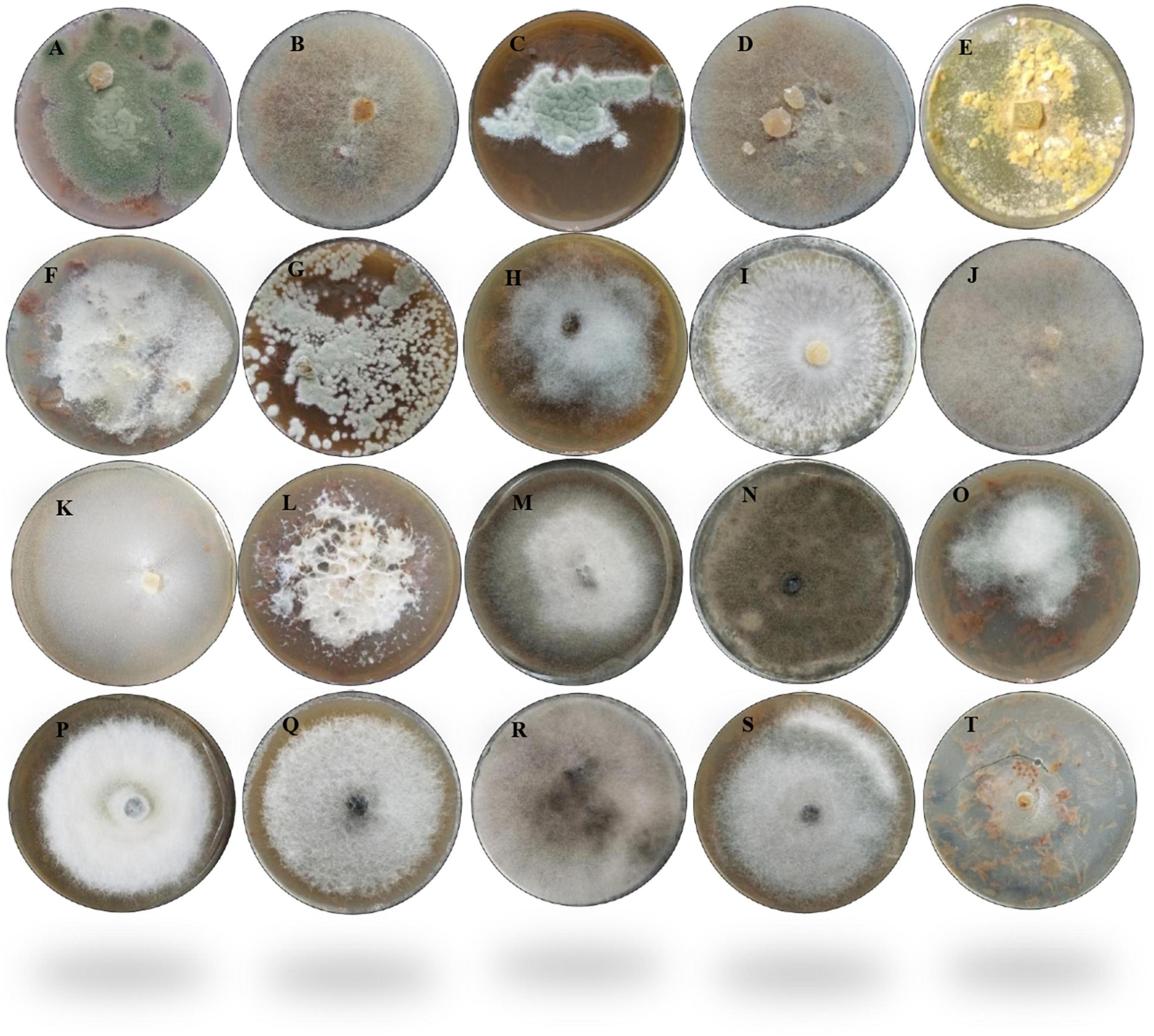
Figure 2. Morphological appearance of the 20 fungal strains isolated from fruit berries. (A) Penicillium crustosum1F. (B) Mucor circinelloides 1BF. (C) Penicillium expansum 230. (D) Mucor fragilis 22. (E) Trichoderma sp. 4AF. (F) Fusarium brachygibbosum 4BF. (G) Penicillium expansum 5F. (H) Botrytis sp. 62C. (I) Botrytis cinerea 62BCV. (J) Mucor fragilis FRA. (K) Geotrichum candidum FRB. (L) Fusarium brachygibbosum HBF. (M) Alternaria alternata 1A. (N) Cladosporium sp. 1BOA. (O) Alternaria alternata 2Z. (P) Alternaria sp. 3A. (Q) Alternaria alternata 4A. (R) Botryosphaeria rhodina 5A. (S) Alternaria alternata 6A. (T) Geotrichum phurueaensis 7Z. The fungal growth was observed at different times, ranging from 5 to 8 days.
Confrontation Assays
Once the growth and infection capacity of fungal phytopathogens was confirmed in strawberry and blueberry fruit, confrontation tests were performed using strain SER3. SER3 remarkably inhibited mycelial growth through the action of diffusible compounds against eight phytopathogens, such as Alternaria alternata, Botryosphaeria rhodina, Mucor circinelloides, Botrytis spp., and Fusarium spp. (Figure 3A). Although an inhibitory trend was observed in the growth of some phytopathogens by the action of volatile compounds from SER3, results showed that the inhibition was not significant (Figure 3B).
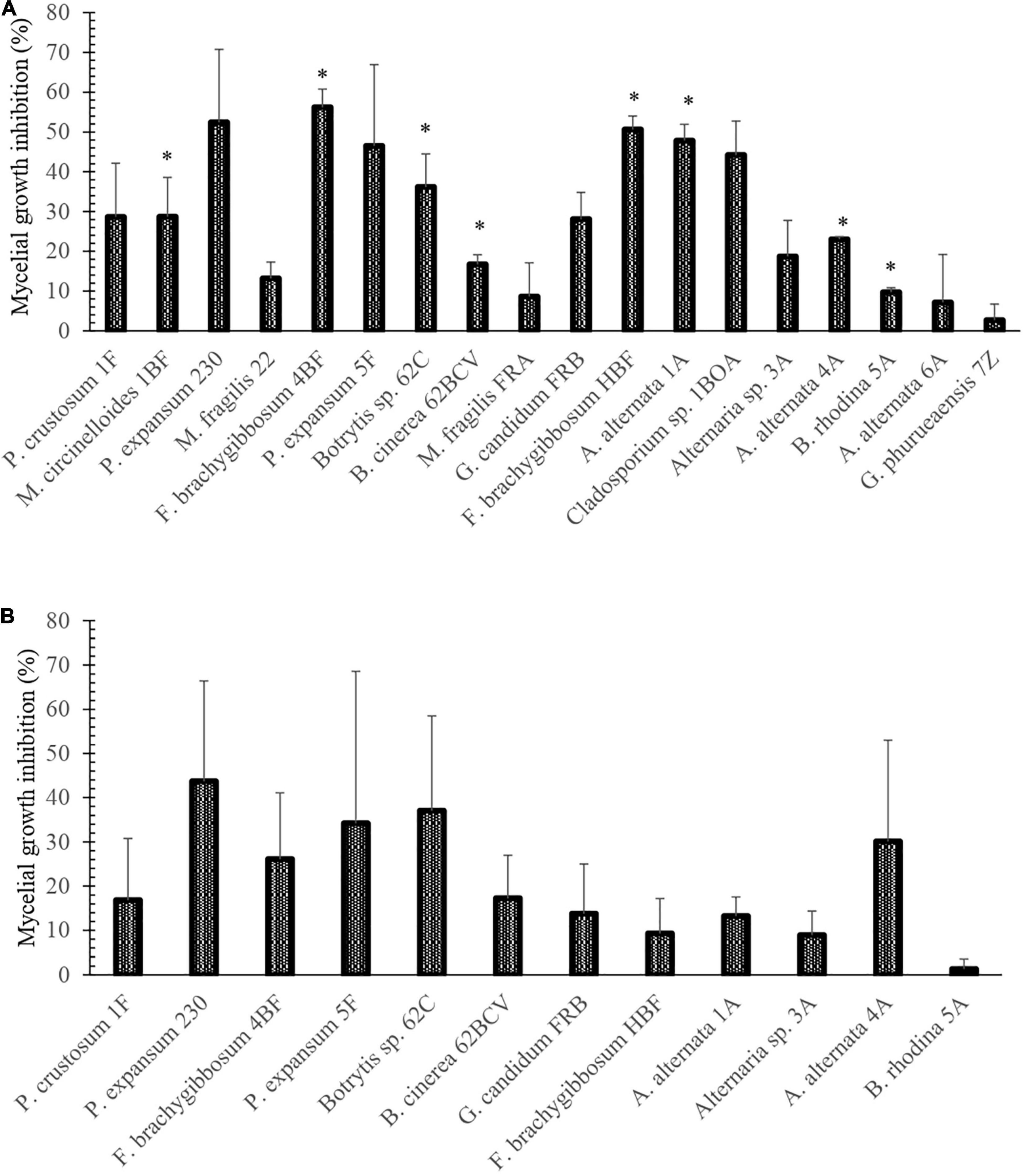
Figure 3. Percentages of inhibition of fungi isolated from berries postharvest caused by (A) diffusible and (B) volatile compounds of the SER3 strain. The experiments were carried out independently three times. Bars represent mean ± SE. Asterisks indicate significant difference compared to the respective controls, using Student’s t-test (p < 0.05).
In vivo Phytopathogen Inhibition Assay Using Strain SER3
To evaluate the potential antagonism of strain SER3 against phytopathogens on fruit, two important postharvest phytopathogens (B. cinerea 62BCV and Fusarium brachyggibosum 4BF) were selected. Figure 4 shows that SER3 produced significant mycelium growth inhibition of B. cinerea 62BCV through direct interaction (42.66%), while the cell-free supernatant (CFS) inhibited only 5.55% of phytopathogen growth. With F. brachyggibosum 4BF, mycelial growth was inhibited by 75.68%, while CFS restricted mycelial growth by 57.37%. Microscopic analysis of the mycelia of each fungal phytopathogen showed deformations and protrusions in their hyphae on application of the bacterial strain or the cell-free supernatant, whereas typical hyphae were observed in the control in the absence of strain SER3 or its CFS (Figure 5).
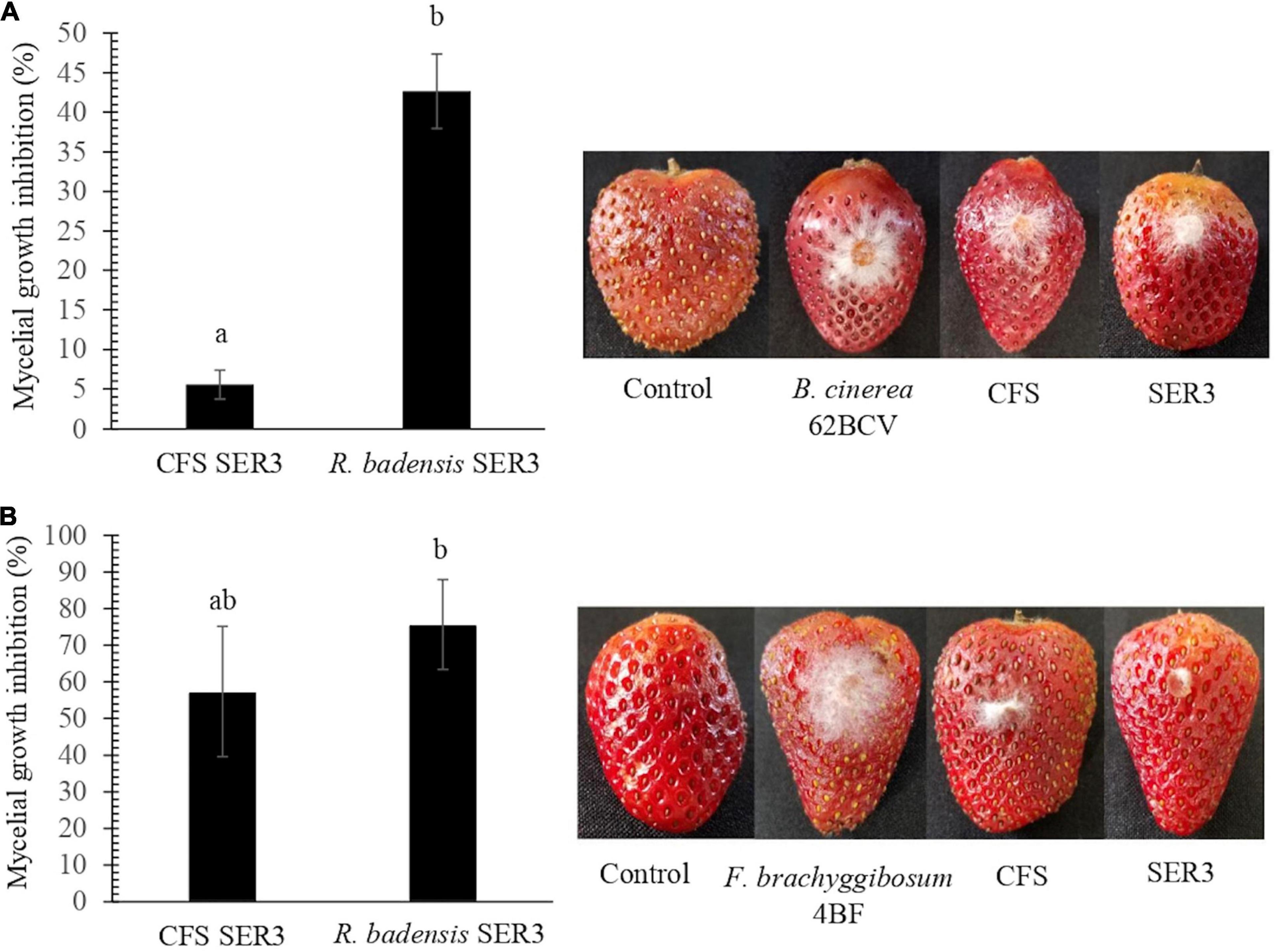
Figure 4. Biocontrol effects of SER3 and its supernatant on strawberries. (A) Mycelial growth inhibition of Botrytis cinerea by the cell-free supernatant (CFS) and SER3. (B) Mycelial growth inhibition of Fusarium brachyggibosum by the CFS and SER3. Treatments consisted of sterile distilled water (control) and inoculation with pathogens, CFS from SER3, and cell suspensions of SER3. Experiments were independently performed three times, and bars represent mean ± SE (n = 9). Letters indicate significant difference, based on Duncan’s multiple range test (p < 0.05).
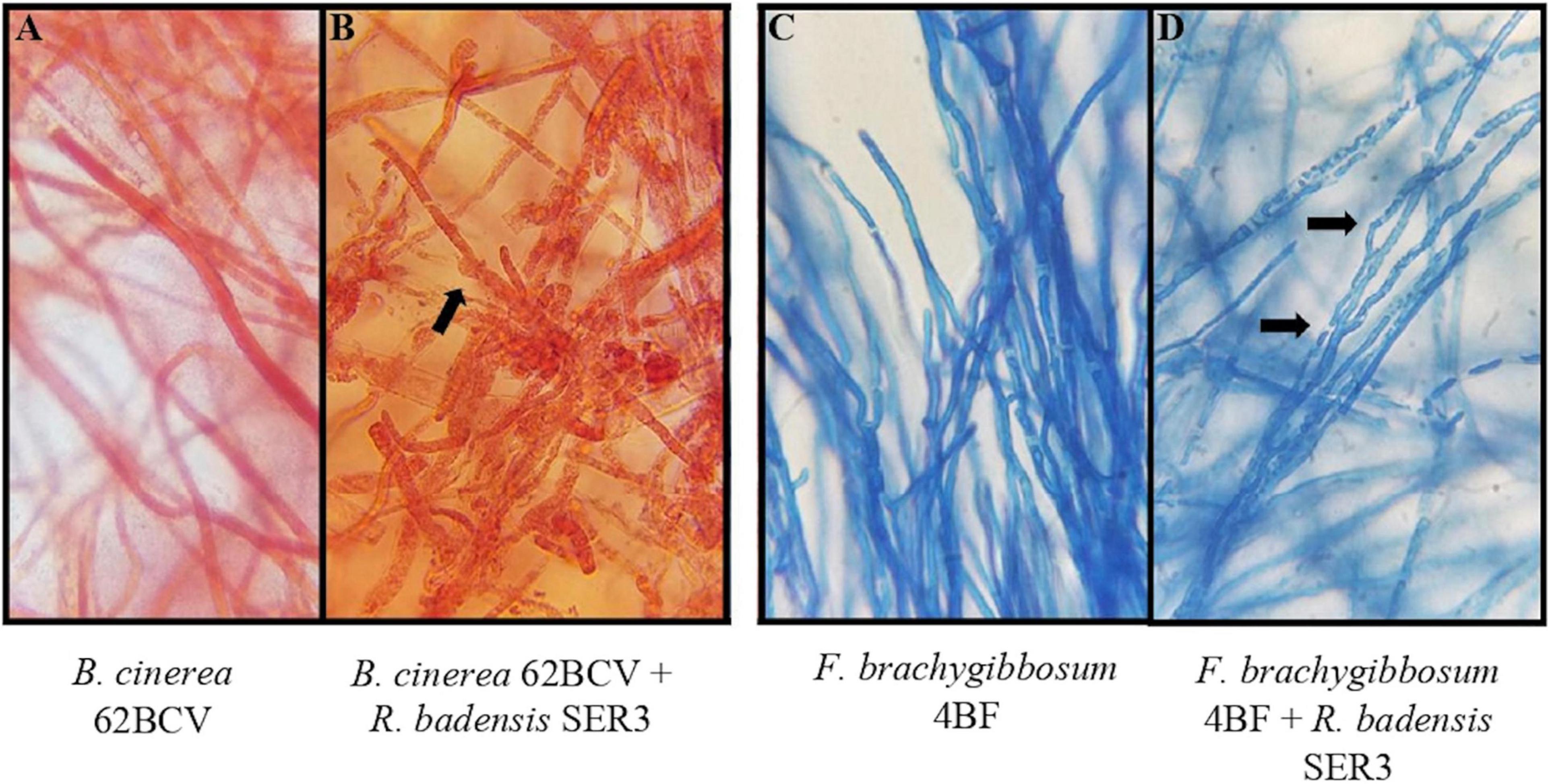
Figure 5. Effect of SER3 on mycelial morphology of (A,B) B. cinerea and (C,D) F. brachyggibosum. Panels (A,C) represent the controls, Panels (B,D) show the interaction between the bacterial strain and each of the pathogens. Arrows indicate distortion of hyphae (×100 magnification).
Genome Features of Strain SER3
To gain better understanding of the potential traits of strain SER3 involved in postharvest phytopathogen biocontrol, its genome was sequenced. The SER3 genome consisted of 47 contigs, and the quality of the assembly was evaluated with Quast4, with approximately 5.08 Mb, a GC content of 52.8%, and 4,545 open reading frames, among other genes that code for ribosomal genes (Table 2 and Figure 6). Similar numbers are also found in other Rouxiella genomes. The genome sequences were deposited in GenBank under the following accession numbers: NZ_CP049603.1; BioProject, PRJNA224116; and BioSample, SAMN14066751.
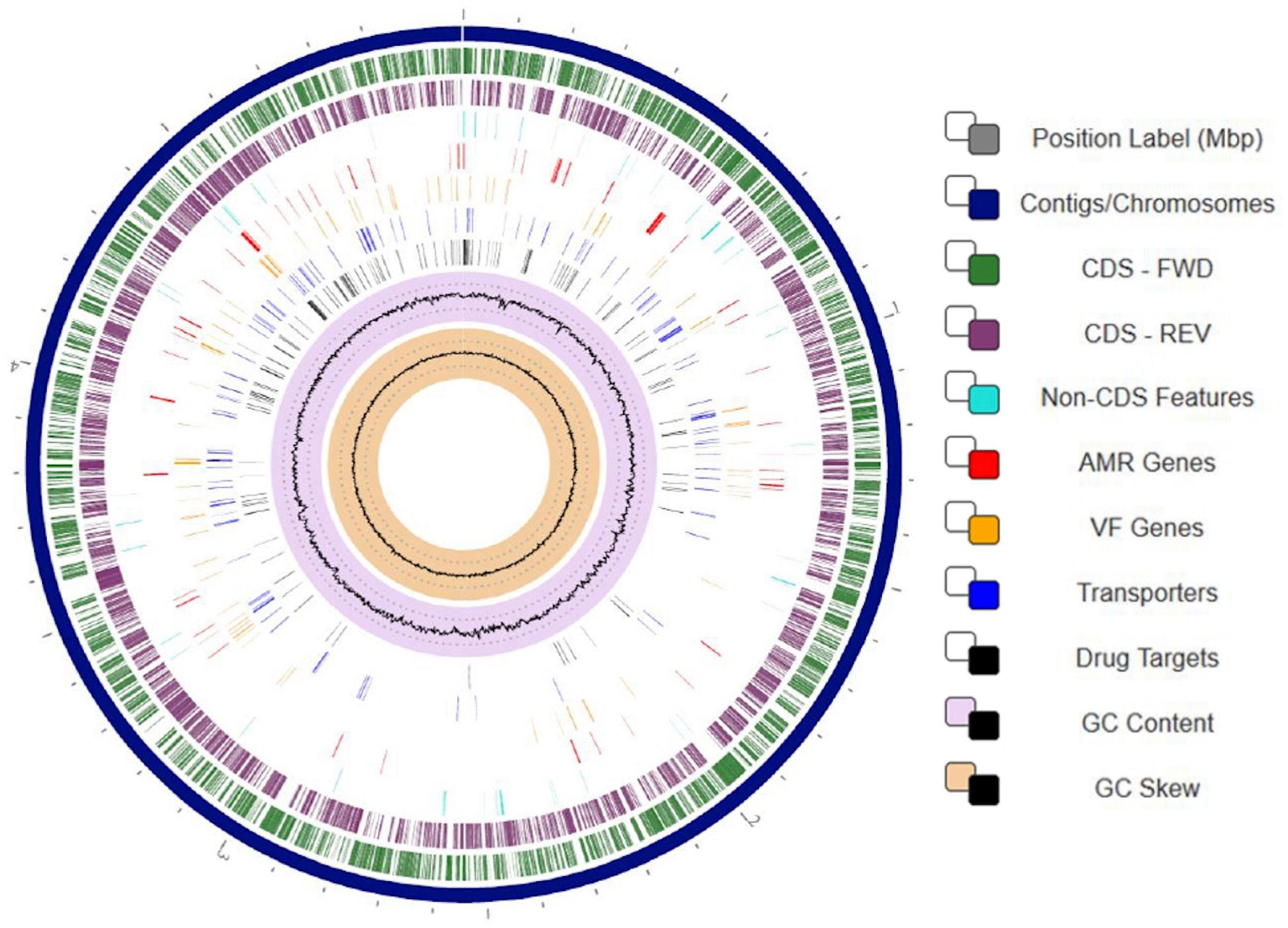
Figure 6. Circular visualization of the Rouxiella badensis strain SER3 genome. Numbers represent megabases (Mbp). From outside to the center: 47 contigs were assembled to form a scaffold representing the chromosome (navy blue track). Genes on forward strand (green), genes on reverse strand (purple), non-CDS features (turquoise), resistance genes (red), virulence factor genes (orange), transporters (blue), drug targets (black), GC content (lilac and black), and GC skew (khaki and black). To display the circular viewer, we used genome annotation service from PATRIC (RAST tool kit).
Taxonomic Affiliation of SER3
Based on the sequences of the 16S rRNA gene, SER3 showed 100% identity with the type strains of R. badensis DSM 100043T (Supplementary Figure 3). A phylogenomic approach confirmed the close relationship with the R. badensis DSM 100043 type strain (Figure 7). Moreover, a comparison at the genomic level of strain SER3 through ANI > 95–96% and the GGDC > 70% also showed that it is strongly affiliated with R. badensis (Table 3).
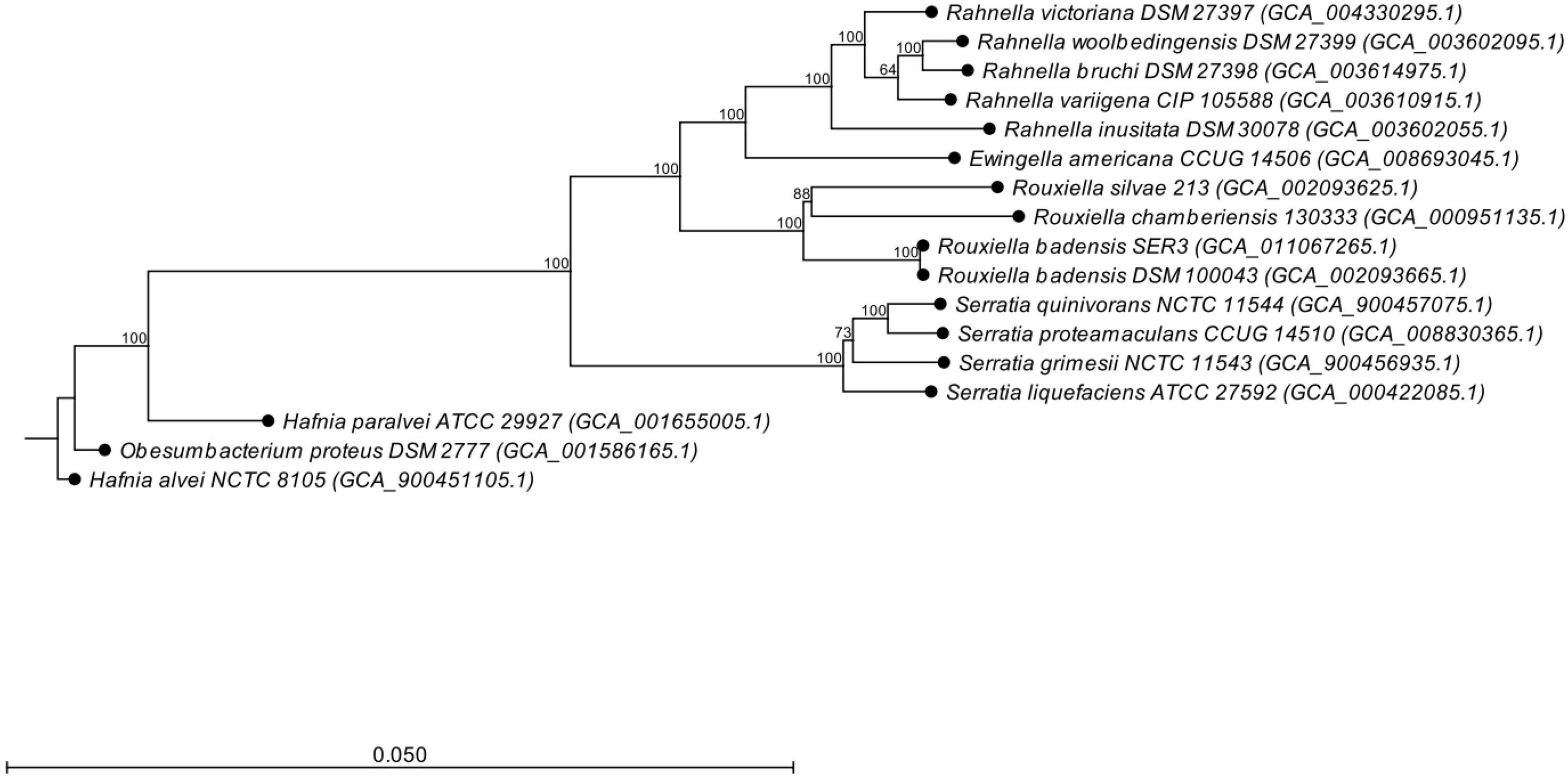
Figure 7. Phylogenomic tree based on the whole genome sequence data of Rouxiella badensis strain SER3, including the relationship with other bacterial species. The phylogenetic tree was constructed using the neighbor-joining algorithm. Bootstrap analysis of 1,000 replications was performed and is expressed as a percentage. Hafnia alvei NCTC 8,105 was used as an outgroup.
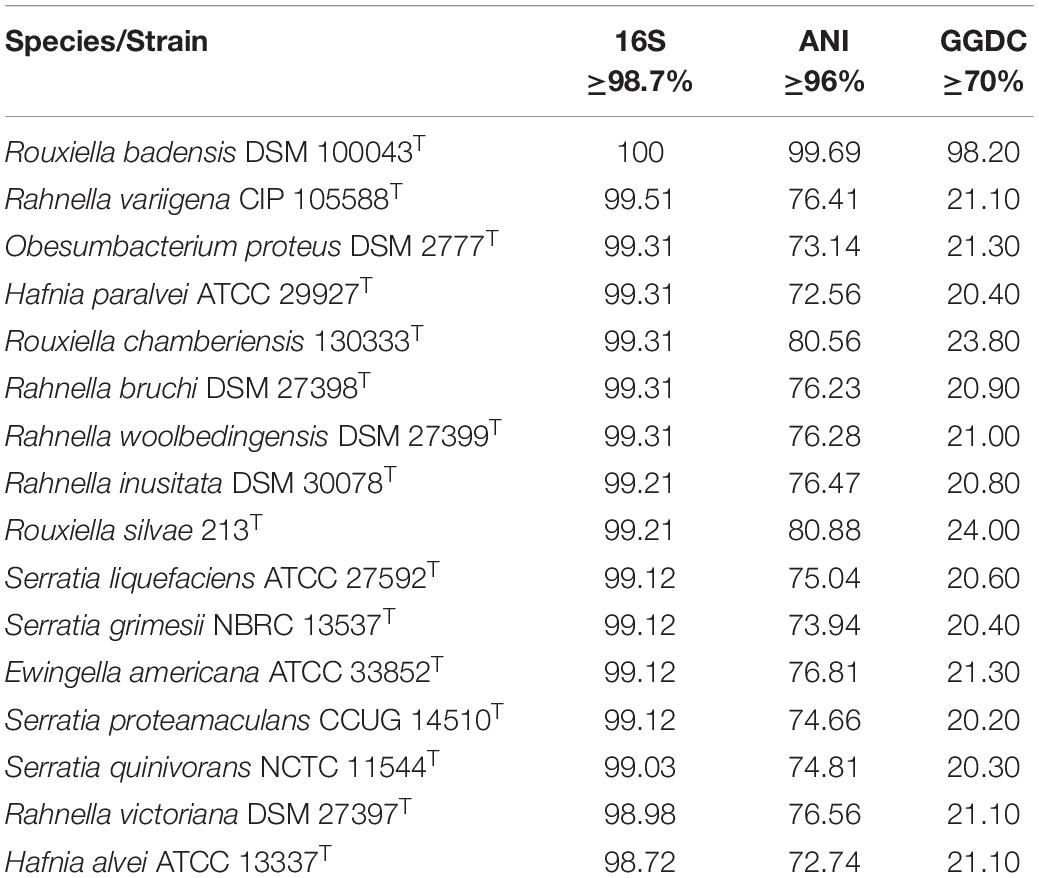
Table 3. OGRIs values obtained from the genome comparison of strain SER3 and closely related species.
Search for Biocontrol Gene Clusters in SER3 and Related Genomes
The antiSMASH program was used to determine the potential compounds involved in the postharvest biocontrol of phytopathogens by R. badensis SER3 and other closely related species, including two R. badensis strains (DSM 100043 and WG36), Rhanella spp., and Serratia spp. In the SER3 genome, biosynthetic gene clusters involved in siderophores (100%) and polyenes (77%) were observed. In addition, compounds such as thiopeptides, non-ribosomal peptide synthetases (NRPS), and polyketide synthases (PKS) were identified (Table 4). Similar biosynthetic clusters and percentages were observed in the other two R. badensis genomes analyzed, corroborating their close phylogenomic similarity. A 100% similarity was observed for siderophore biosynthetic clusters in Rhanella spp. and Ewingella americana CCUG 14506, and in Obesumbacterium proteus DSM 2777 and Hafnia spp. with a good similarity score (75%) in their respective genomes.
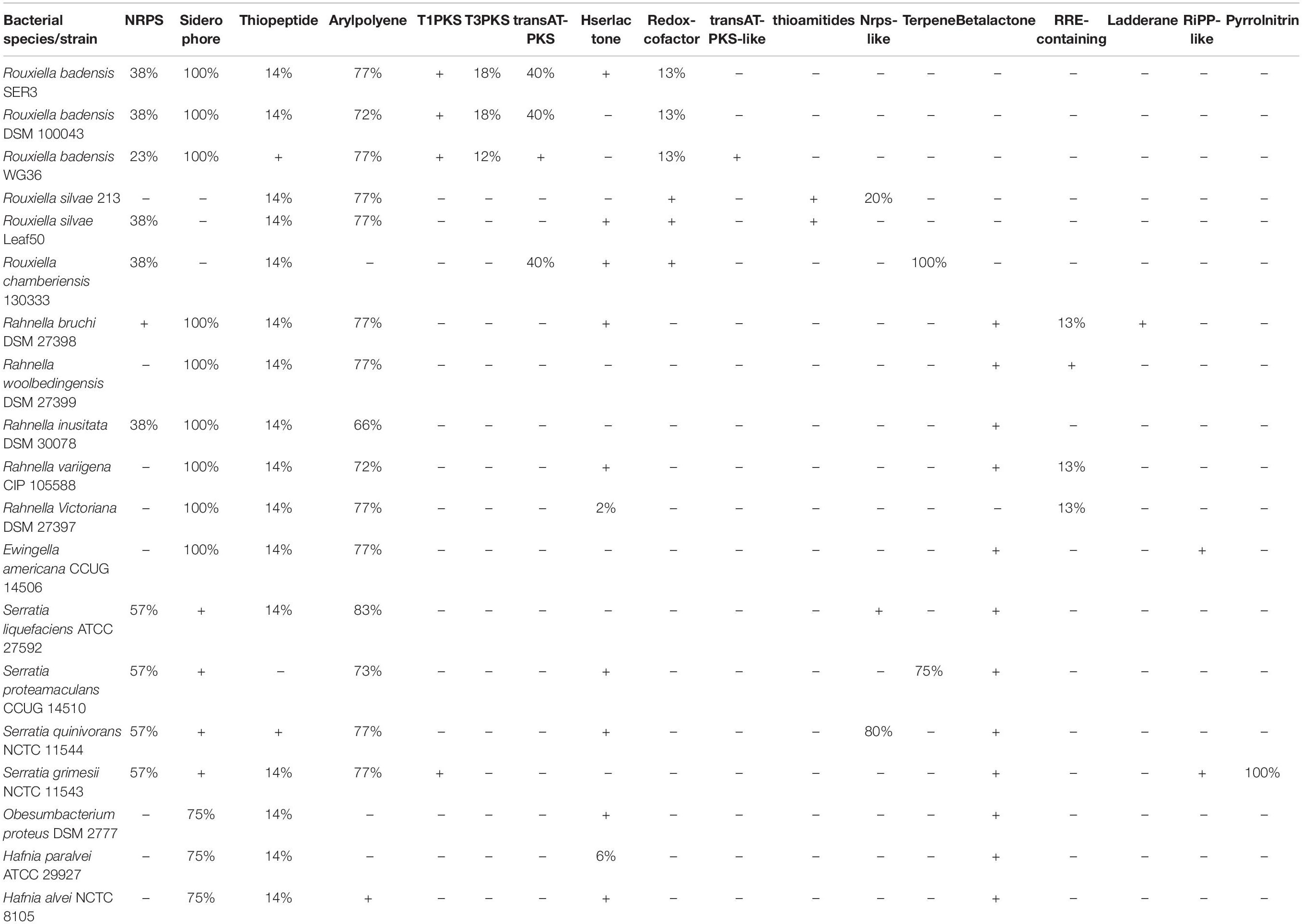
Table 4. AntiSMASH analysis and prediction of biosynthetic compounds in R. badensis SER3 and related bacterial genomes.
Discussion
Berries (strawberries, blackberries, and blueberries) have a very short shelf life after harvest. Therefore, they must be immediately distributed for use, preferably under cold chain, which considerably hinders their international commercialization. High postharvest fruit losses due to phytopathogenic fungal diseases are related to high humidity levels, increased nutrients, low pH values, and low intrinsic resistance to postharvest decomposition and fungal diseases (Dukare et al., 2019). During the decomposition process, the fruit loses weight and decreases in firmness and quality, resulting in economic losses. In many instances, synthetic chemical compounds are used as coatings to avoid pathogen infections and extend their shelf life; however, toxic residues can be hazardous to human health, in addition to restricting the global commercialization of berries. Therefore, it is important to describe the phytopathogens that affect postharvest berries as well as to develop sustainable alternatives for their biological control (Abeer et al., 2013).
Here, 20 fungal strains were isolated from berries and characterized by ITS sequencing and homology searches. They showed similarity to Botrytis or Fusarium, among others. Previous reports have shown that several of these genera are phytopathogens that cause pre- and postharvest diseases in various crops, including strawberries (Dukare et al., 2019). In particular, B. cinerea can easily infect berries such as strawberry, blueberry, blackberry, raspberry, cranberry, and bilberry fruit, causing drastic losses after harvest (Leroux et al., 2002; Romanazzi and Feliziani, 2014; Petrasch et al., 2019). Another type of ascomycete fungus that causes damage to various crops is Fusarium, which is best known for affecting the roots and some aerial parts of plants, such as stems, and causes vascular browning, leaf epinasty, stunting, progressive wilting, defoliation, floral damage, and subsequent plant death (Dean et al., 2012). Herein, two strains with highly similar identity (98.3 and 99.2%) to F. brachygibbosum were isolated from strawberry fruit (Table 1). To our knowledge, F. brachygibbosum has not been reported as a fruit phytopathogen; therefore, this would be the first report as a postharvest pathogen in fruit such as strawberries. Further studies on the morphology of F. brachygibbosum and analysis of other molecular markers are being conducted by our research group to corroborate this hypothesis.
Other fungal genera found in berries were Alternaria, Cladosporium, Geotrichum, Mucor, and Penicillium, which have already been reported as causative agents of postharvest disease in these fruit (Koike et al., 2003; Tournas and Katsoudas, 2005; Gordon et al., 2016; López et al., 2016; Pastrana et al., 2017; Petrasch et al., 2019). It should be noted that fungi belonging to beneficial species such as Trichoderma have also been found in berries (Santoyo et al., 2021). In the present work, the strain Trichoderma sp. AF4 did not produce any apparent damage when reinoculated in strawberries and showed similar results as the uninoculated controls. Moreover, preliminary studies performed in our laboratory suggest that AF4 restricts the growth of some postharvest berry pathogens.
In agreement with the aforementioned studies, the fruit microbiome has been reported to contain not only pathogenic species but also microorganisms that can naturally help fight postharvest diseases, thus reducing losses through increased shelf life and fruit quality (Droby and Wisniewski, 2018). Consequently, we isolated the SER3 strain from the surface of a strawberry fruit, and it showed antifungal activity against Fusarium (Supplementary Figure 1). Furthermore, during activity evaluation, SER3 exhibited significant antagonism against the postharvest pathogens isolated herein. Moreover, the volatile compounds of SER3 also exhibited inhibition of mycelial growth, although to a lesser extent, with significant inhibition being observed only against two species, viz., P. expansum and F. brachygibbosum. These results suggested that SER3 antagonizes the phytopathogens through the action of diffusible (mainly) and volatile compounds, which is consistent with other studies showing similar mechanisms of action in other bacterial species (Hernández-León et al., 2015; Wallace et al., 2017). The inhibition of mycelial growth of postharvest phytopathogens was corroborated by in vivo tests on strawberry fruit using B. cinerea and F. brachygibbosum. Following the coinoculation of the SER3 strain and B. cinerea or F. brachygibbosum, the hyphae presented deformations and protrusions on the surface. This type of damage in the fungal pathogen hyphae has been observed in other studies and is associated with a reduction in fungal pathogenicity (Wallace et al., 2017; Emanuel et al., 2020).
Given the relevant biocontrol properties of strain SER3, its genome was sequenced, and its taxonomic affiliation was assigned based on ANI and GGDC. Based on these parameters, SER3 was established to belong to the R. badensis species. R. badensis is a relatively new species described in 2017; it is a Gram-negative bacillus that forms whitish colonies, can grow optimally at 37°C, reduces nitrates, and produces acid from different sugars (Le Fléche-Matéos et al., 2017). To investigate the possible antifungal mechanism of R. badensis SER3, its genome was analyzed using the antiSMASH server (Blin et al., 2017), which led to the prediction of various antibiotic compounds and antifungal compounds such as siderophores, NRPS, and PKS. These three compounds are extracellular and are produced by a wide range of biocontrol bacterial species, such as Bacillus and Pseudomonas, and close relatives of R. badensis, such as Rahnella aquatilis, which have been characterized as antifungals (Calvo et al., 2007; Chen et al., 2007; Santoyo et al., 2012; Carmona-Hernandez et al., 2019). Likewise, NRPS and PKS are not exclusive to bacterial strains but can also be synthesized by phytopathogenic and beneficial fungi, such as Trichoderma (Mukherjee et al., 2012). Other compounds reported to have been found in the R. badensis SER3 genome were a cluster of desferrioxamine-type siderophores (100% similarity), which are iron-chelating compounds (Boiteau et al., 2019) and can restrict the growth of pathogens (Kloepper et al., 1980; de los Santos-Villalobos et al., 2012). They have been reported in a wide range of biocontrol and plant growth promoter species (Crowley, 2006; Wang et al., 2020). Interestingly, the same compounds, such as siderophores, NRPS, and arylpolyene compounds, with similar percentages of identity were detected using antiSMASH in two other R. badesis genomes. Similarly, close relatives of R. badensis, such as Rahnella, also presented good similarity percentages with clusters for the synthesis of siderophores, thiopeptides, and arylpolyenes in their respective genomes. Other bacterial species, including those belonging to genera such as Ewingella (Roy Chowdhury et al., 2007), Obesumbacterium (Amin et al., 2014), and Hafnia, contain highly similar clusters for the synthesis of potential compounds, such as siderophores (100%). These results support the proven role of SER3 in the biocontrol of fungal pathogens and similar roles reported by Calvo et al. (2007) and Chen et al. (2007) in Rahnella and Serratia genera. To our knowledge, the potential role in the biocontrol of plant fungal pathogens has not been described for the rest of the bacterial species analyzed here with antiSMASH (Table 4).
Conclusion
In this study, a functional analysis of the biocontrol activities of the novel strain SER3 against postharvest pathogenic fungi of berries was performed, which showed a high genomic and phylogenetic identity with R. badensis. Thus, we propose a new ecological role for this species and other species of the genus Rouxiella. Notably, SER3 genome provides some indications of the antifungal modes of action; however, other mechanisms of biocontrol by R. badensis SER3 cannot be excluded, since other antifungal activities, such as the activity of lytic enzymes, have not been explored. In addition, antiSMASH analysis for other species analyzed in this study provides some clues of possible antagonistic action toward plant pathogens, although this hypothesis requires further investigation. Lastly, isolation of SER3 presents a new option in the biocontrol of postharvest pathogens of berries and provides new opportunities to investigate its role as a promoter of plant growth through direct mechanisms.
Data Availability Statement
The datasets presented in this study can be found in online repositories. The names of the repository/repositories and accession number(s) can be found below: https://www.ncbi.nlm.nih.gov/genbank/, NZ_CP049603.1.
Author Contributions
LRM-C conducted the experiments, analyzed the data, and prepared the figures and tables. SS-V and GS conceived and designed the experiments and analyzed the data. GS wrote the first draft of the manuscript. All authors contributed to manuscript revision, read, and approved the submitted version.
Funding
This work was supported by CONACYT-México (Propuesta A1-S-15956). LRM-C received a Ph.D. scholarship from CONACYT-México.
Conflict of Interest
The authors declare that the research was conducted in the absence of any commercial or financial relationships that could be construed as a potential conflict of interest.
Publisher’s Note
All claims expressed in this article are solely those of the authors and do not necessarily represent those of their affiliated organizations, or those of the publisher, the editors and the reviewers. Any product that may be evaluated in this article, or claim that may be made by its manufacturer, is not guaranteed or endorsed by the publisher.
Acknowledgments
We thank Julie Hernandez-Salmerón for excellent technical assistance with the genome assembly.
Supplementary Material
The Supplementary Material for this article can be found online at: https://www.frontiersin.org/articles/10.3389/fmicb.2021.709855/full#supplementary-material
Supplementary Figure 1 | Effect of diffusible compounds of SER3 following direct co-inoculation with the Fusarium pathogen. The bacterial strain was streaked onto plates in a cross shape, and mycelial plugs 4 mm in diameter were deposited at the center of the quadrants formed. Experiments were independently performed a minimum of three times. The plates were incubated, and mycelial growth was measured on day 3. The percentage of growth inhibition was measured as follows:% growth inhibition = [(Ac – Ab)/Ac] × 100, where Ac is the control mycelial area, and Ab is the mycelial area with treatment.
Supplementary Figure 2 | Koch’s postulates. Berries were infected (n = 18) with spore solutions (1 × 105 spores/mL). Panel (A) shows blueberries inoculated with Cladosporium sp. 1BOA spores, while panel (B) shows strawberries inoculated with Penicillium expansum 230 spores, and panel (C) shows strawberries inoculated with Mucor circinelloides 1BF spores.
Supplementary Figure 3 | Phylogenetic tree based on the 16S ribosomal gene sequence of Rouxiella badensis strain SER3, including the relationship with other bacterial species (nucleotide sequence can be accessed in GenBank: CP049603). A phylogenetic tree was constructed using the maximum-likelihood algorithm. Bootstrap analysis of 1000 replications was performed and expressed as a percentage, and the most common enterobacteria were used as an outgroup.
Footnotes
- ^ https://www.patricbrc.org/
- ^ http://rast.theseed.org/FIG/rast.cgi
- ^ http://rast.nmpdr.org
- ^ http://quast.sourceforge.net/quast
References
Abeer, H., Abd-Allah, E. F., Al-Obeed, R. S., Mridha, M. A. U., and Al-Huqail, A. A. (2013). Non-chemical strategies to control postharvest losses and extend the shelf life of table grape fruits. Biol. Agric. Hortic. 29, 82–90. doi: 10.1080/01448765.2013.763735
Allen, T., and Prosperi, P. (2016). Modeling Sustainable Food Systems. Environ. Manage. 57, 956–975. doi: 10.1007/s00267-016-0664-8
Amin, A. W., Anter, A. A., Ashoub, A. H., and El-Nuby, A. S. (2014). Evaluation of rhizobacteria as resistance inducers or bio-control agents for the control of Meloidogyne incognita in tomato. Pak. J. Nematol. 32, 211–221.
Andrews, S. (2010). FastQC A Quality Control tool for High Throughput Sequence Data. Available online at: http://www.bioinformatics.babraham.ac.uk/projects/fastqc
Aziz, R. K., Bartels, D., Best, A., DeJongh, M., Disz, T., Edwards, R. A., et al. (2008). The RAST Server: rapid annotations using subsystems technology. BMC Genomics 9:75. doi: 10.1186/1471-2164-9-75
Backer, R., Rokem, J. S., Ilangumaran, G., Lamont, J., Praslickova, D., Ricci, E., et al. (2018). Plant growth-promoting rhizobacteria: context, mechanisms of action, and roadmap to commercialization of biostimulants for sustainable agriculture. Front. Plant Sci. 871:1473. doi: 10.3389/fpls.2018.01473
Bankevich, A., Nurk, S., Antipov, D., Gurevich, A. A., Dvorkin, M., Kulikov, A. S., et al. (2012). SPAdes: a new genome assembly algorithm and its applications to single-cell sequencing. J. Comput. Biol. 19, 455–477. doi: 10.1089/cmb.2012.0021
Bertels, F., Silander, O. K., Pachkov, M., Rainey, P. B., and Van Nimwegen, E. (2014). Automated reconstruction of whole-genome phylogenies from short-sequence reads. Mol. Biol. Evol. 31, 1077–1088. doi: 10.1093/molbev/msu088
Blin, K., Wolf, T., Chevrette, M. G., Lu, X., Schwalen, C. J., Kautsar, S. A., et al. (2017). AntiSMASH 4.0 - improvements in chemistry prediction and gene cluster boundary identification. Nucleic Acids Res. 45, W36–W41. doi: 10.1093/nar/gkx319
Boiteau, R. M., Fansler, S. J., Farris, Y., Shaw, J. B., Koppenaal, D. W., Pasa-Tolic, L., et al. (2019). Siderophore profiling of co-habitating soil bacteria by ultra-high resolution mass spectrometry. Metallomics 11, 166–175. doi: 10.1039/c8mt00252e
Bolger, A. M., Lohse, M., and Usadel, B. (2014). Trimmomatic: a flexible trimmer for Illumina sequence data. Bioinformatics 30, 2114–2120. doi: 10.1093/bioinformatics/btu170
Calvo, J., Calvente, V., de Orellano, M. E., Benuzzi, D., and Sanz de Tosetti, M. I. (2007). Biological control of postharvest spoilage caused by Penicillium expansum and Botrytis cinerea in apple by using the bacterium Rahnella aquatilis. Int. J. Food Microbiol. 113, 251–257. doi: 10.1016/j.ijfoodmicro.2006.07.003
Carmona-Hernandez, S., Reyes-Pérez, J. J., Chiquito-Contreras, R. G., Rincon-Enriquez, G., Cerdan-Cabrera, C. R., and Hernandez-Montiel, L. G. (2019). Biocontrol of postharvest fruit fungal diseases by bacterial antagonists: a review. Agronomy 9:121. doi: 10.3390/agronomy9030121
Chen, F., Guo, Y. B., Wang, J. H., Li, J. Y., and Wang, H. M. (2007). Biological control of grape crown gall by Rahnella aquatilis HX2. Plant Dis. 91, 957–963. doi: 10.1094/PDIS-91-8-0957
Chun, J., Oren, A., Ventosa, A., Christensen, H., Arahal, D. R., da Costa, M. S., et al. (2018). Proposed minimal standards for the use of genome data for the taxonomy of prokaryotes. Int. J. Syst. Evol. Microbiol. 68, 1498–1513. doi: 10.1099/ijsem.0.002516
Compant, S., Duffy, B., Nowak, J., Clément, C., and Barka, E. A. (2005). Use of plant growth-promoting bacteria for biocontrol of plant diseases: principles, mechanisms of action, and future prospects. Appl. Environ. Microbiol. 71, 4951–4959. doi: 10.1128/AEM.71.9.4951-4959.2005
Contreras, M., Loeza, P. D., Villegas, J., Farias, R., and Santoyo, G. (2016). A glimpse of the endophytic bacterial diversity in roots of blackberry plants (Rubus fruticosus). Genet. Mol. Res. 15:gmr.15038542. doi: 10.4238/gmr.15038542
Córdova-Albores, L. C., Zelaya-Molina, L. X., Ávila-Alistac, N., Valenzuela-Ruíz, V., Cortés-Martínez, N. E., Parra-Cota, F. I., et al. (2020). Omics sciences potential on bioprospecting of biological control microbial agents: the case of the Mexican agro-biotechnology. Rev. Mex. Fitopatol. 39, 147–185. doi: 10.18781/r.mex.fit.2009-3
Crowley, D. E. (2006). “Microbial Siderophores in the plant rhizosphere,”in Iron Nutrition in Plants and Rhizospheric Microorganisms, eds L. L. Barton., and J. Abadia (Dordrecht: Springer), 169–198. doi: 10.1007/1-4020-4743-6_8
de los Santos-Villalobos, S., Barrera-Galicia, G. C., Miranda-Salcedo, M. A., and Peña-Cabriales, J. J. (2012). Burkholderia cepacia XXVI siderophore with biocontrol capacity against Colletotrichum gloeosporioides. World J. Microbiol. Biotechnol. 28, 2615–2623. doi: 10.1007/s11274-012-1071-9
Dean, R., Van Kan, J. A. L., Pretorius, Z. A., Hammond-Kosack, K. E., Di Pietro, A., Spanu, P. D., et al. (2012). The Top 10 fungal pathogens in molecular plant pathology. Mol. Plant Pathol. 13, 414–430. doi: 10.1111/j.1364-3703.2011.00783.x
Droby, S., and Wisniewski, M. (2018). The fruit microbiome: a new frontier for postharvest biocontrol and postharvest biology. Postharvest Biol. Technol. 140, 107–112. doi: 10.1016/j.postharvbio.2018.03.004
Dukare, A. S., Paul, S., Nambi, V. E., Gupta, R. K., Singh, R., Sharma, K., et al. (2019). Exploitation of microbial antagonists for the control of postharvest diseases of fruits: a review. Crit. Rev. Food Sci. Nutr. 68, 1498–1513. doi: 10.1080/10408398.2017.1417235
Elad, Y. (2000). Biological control of foliar pathogens by means of Trichoderma harzianum and potential modes of action. Crop Prot. 19, 709–714. doi: 10.1016/S0261-2194(00)00094-6
Emanuel, R. V., César Arturo, P. U., Lourdes Iveth, M. R., de la Cruz Homero, R., and Mauricio Nahuam, C. A. (2020). In vitro growth of Colletotrichum gloeosporioides is affected by butyl acetate, a compound produced during the co-culture of Trichoderma sp. and Bacillus subtilis. 3 Biotech 10, 1–14. doi: 10.1007/s13205-020-02324-z
Fried, G., Chauvel, B., Reynaud, P., and Sache, I. (2017). “Decreases in Crop Production by Non-native Weeds, Pests, and Pathogens,”in Impact of Biological Invasions on Ecosystem Services. Invading Nature - Springer Series in Invasion Ecology, eds M. Vilà., and P. Hulme (Cham: Springer). doi: 10.1007/978-3-319-45121-3
Glick, B. R. (2012). Plant Growth-Promoting Bacteria : mechanisms and Applications. Scientifica. 2012:963401.
Gordon, T. R., Daugovish, O., Koike, S. T., Islas, C. M., Kirkpatrick, S. C., Yoshisato, J. A., et al. (2016). Options for Management of Fusarium Wilt of Strawberry in California. Int. J. Fruit Sci. 16, 160–168. doi: 10.1080/15538362.2016.1219294
Hernández-León, R., Rojas-Solís, D., Contreras-Pérez, M., del Orozco-Mosqueda, M. C., Macías-Rodríguez, L. I., Reyes-de la Cruz, H., et al. (2015). Characterization of the antifungal and plant growth-promoting effects of diffusible and volatile organic compounds produced by Pseudomonas fluorescens strains. Biol. Control 81, 83–92. doi: 10.1016/j.biocontrol.2014.11.011
Höfte, M., and Altier, N. (2010). Fluorescent pseudomonads as biocontrol agents for sustainable agricultural systems. Res. Microbiol. 161, 464–471. doi: 10.1016/j.resmic.2010.04.007
Islam, M. T., Rahman, M., Pandey, P., Jha, C. K., and Aeron, A. (2017). Bacilli and Agrobiotechnology. Berlin: Springer International Publishing. doi: 10.1007/978-3-319-44409-3.
Kamoun, S., Furzer, O., Jones, J. D. G., Judelson, H. S., Ali, G. S., Dalio, R. J. D., et al. (2015). The Top 10 oomycete pathogens in molecular plant pathology. Mol. Plant Pathol. 16, 413–434. doi: 10.1111/mpp.12190
Khan, N., Martínez-Hidalgo, P., Ice, T. A., Maymon, M., Humm, E. A., Nejat, N., et al. (2018). Antifungal activity of bacillus species against fusarium and analysis of the potential mechanisms used in biocontrol. Front. Microbiol. 9:2363. doi: 10.3389/fmicb.2018.02363
Kloepper, J. W., Leong, J., Teintze, M., and Schroth, M. N. (1980). Pseudomonas siderophores: a mechanism explaining disease-suppressive soils. Curr. Microbiol. 4, 317–320. doi: 10.1007/BF02602840
Koike, S. T., Vilchez, M. S., and Paulus, A. O. (2003). Fungal ecology of strawberry flower anthers and the saprobic role of Cladosporium cladosporioides in relation to fruit deformity problems. HortScience 38, 246–250. doi: 10.21273/hortsci.38.2.246
Koo, S. Y., and Cho, K. S. (2009). Isolation and characterization of a plant growth-promoting rhizobacterium, Serratia sp. SY5. J. Microbiol. Biotechnol. 19, 1431–1438.
Le Fléche-Matéos, A., Kügler, J. H., Hansen, S. H., Syldatk, C., Hausmann, R., Lomprez, F., et al. (2017). Rouxiella badensis sp. nov. and Rouxiella silvae sp. nov. isolated from peat bog soil and emendation description of the genus Rouxiella. Int. J. Syst. Evol. Microbiol. 67, 1255–1259. doi: 10.1099/ijsem.0.001794
Leroux, P., Fritz, R., Debieu, D., Albertini, C., Lanen, C., Bach, J., et al. (2002). Mechanisms of resistance to fungicides in field strains of Botrytis cinerea. Pest Manag. Sci. 58, 876–888. doi: 10.1002/ps.566
López, S. N., Sangorrín, M. P., and Pildain, M. B. (2016). Fruit rot of sweet cherries and raspberries caused by Penicillium crustosum and Mucor piriformis in South Patagonia, Argentina. Can. J. Plant Pathol. 38, 511–516. doi: 10.1080/07060661.2016.1243582
Magan, N., Aldred, D., Mylona, K., and Lambert, R. J. W. (2010). Limiting mycotoxins in stored wheat. Food Addit. Contam. Part A Chem. Anal. Control Expo. Risk Assess. 27, 644–650. doi: 10.1080/19440040903514523
Mahuku, G. S. (2004). A simple extraction method suitable for PCR-based analysis of plant, fungal, and bacterial DNA. Plant Mol. Biol. Report. 22: 71–81. doi: 10.1007/bf02773351
Meier-Kolthoff, J. P., Auch, A. F., Klenk, H. P., and Göker, M. (2013). Genome sequence-based species delimitation with confidence intervals and improved distance functions. BMC Bioinformatics 14:60. doi: 10.1186/1471-2105-14-60
Morales-Cedeño, L. R., del Carmen Orozco-Mosqueda, M., Loeza-Lara, P. D., Parra-Cota, F. I., de los Santos-Villalobos, S., and Santoyo, G. (2021). Plant growth-promoting bacterial endophytes as biocontrol agents of pre- and post-harvest diseases: fundamentals, methods of application and future perspectives. Microbiol. Res. 242:126612. doi: 10.1016/j.micres.2020.126612
Mukherjee, P. K., Buensanteai, N., Moran-Diez, M. E., Druzhinina, I. S., and Kenerley, C. M. (2012). Functional analysis of non-ribosomal peptide synthetases (NRPSs) in Trichoderma virens reveals a polyketide synthase (PKS)/NRPS hybrid enzyme involved in the induced systemic resistance response in maize. Microbiology 158, 155–165. doi: 10.1099/mic.0.052159-0
Nam, Y. H., Hwang, B. S., Choi, A., and Chung, E. J. (2020). Isolation and characterization of strain Rouxiella sp. S1S-2 producing antibacterial compound. Korean J. Microbiol. 56, 152–159. doi: 10.7845/kjm.2020.0040
Pastrana, A. M., Kirkpatrick, S. C., Kong, M., Broome, J. C., and Gordon, T. R. (2017). Fusarium oxysporum f. sp. mori, a new forma specialis causing fusarium wilt of blackberry. Plant Dis. 101, 2066–2072. doi: 10.1094/PDIS-03-17-0428-RE
Petrasch, S., Knapp, S. J., van Kan, J. A. L., and Blanco-Ulate, B. (2019). Grey mould of strawberry, a devastating disease caused by the ubiquitous necrotrophic fungal pathogen Botrytis cinerea. Mol. Plant Pathol. 20, 877–892. doi: 10.1111/mpp.12794
Rojas-Solís, D., Zetter-Salmón, E., Contreras-Pérez, M., Rocha-Granados, M., del, C., Macías-Rodríguez, L., et al. (2018). Pseudomonas stutzeri E25 and Stenotrophomonas maltophilia CR71 endophytes produce antifungal volatile organic compounds and exhibit additive plant growth-promoting effects. Biocatal. Agric. Biotechnol. 13, 46–52. doi: 10.1016/j.bcab.2017.11.007
Romanazzi, G., and Feliziani, E. (2014). Botrytis cinerea (Gray Mold). Amsterdam: Elsevier, doi: 10.1016/B978-0-12-411552-1.00004-1
Roy Chowdhury, P., Pay, J., and Braithwaite, M. (2007). Isolation, identification and ecology of Ewingella americana (the causal agent of internal stipe necrosis) from cultivated mushrooms in New Zealand. Australas Plant Pathol. 36, 424–428. doi: 10.1071/AP07045
Santoyo, G., del Orozco-Mosqueda, M. C., and Govindappa, M. (2012). Mechanisms of biocontrol and plant growth-promoting activity in soil bacterial species of Bacillus and Pseudomonas: a review. Biocontrol Sci. Technol. 22, 855–872. doi: 10.1080/09583157.2012.694413
Santoyo, G., Guzmán-Guzmán, P., Parra-Cota, F. I., de los Santos-Villalobos, S., del Carmen Orozco-Mosqueda, C., and Glick, B. R. (2021). Plant Growth Stimulation by Microbial Consortia. Agronomy 11:219. doi: 10.3390/AGRONOMY11020219
Sun, X., Ma, W., Xu, Y., Jin, X., and Ni, H. (2020). Complete Genome Sequence of Rahnella aquatilis MEM40, a Plant Growth-Promoting Rhizobacterium Isolated from Rice Rhizosphere Soil, with Antagonism against Magnaporthe oryzae and Fusarium graminearum. Microbiol. Resour. Announc. 9, e651–e620.
Tournas, V. H., and Katsoudas, E. (2005). Mould and yeast flora in fresh berries, grapes and citrus fruits. Int. J. Food Microbiol. 105, 11–17. doi: 10.1016/j.ijfoodmicro.2005.05.002
Vielva, L., De Toro, M., Lanza, V. F., and De La Cruz, F. (2017). PLACNETw: a web-based tool for plasmid reconstruction from bacterial genomes. Bioinformatics 33, 3796–3798. doi: 10.1093/bioinformatics/btx462
Wallace, R. L., Hirkala, D. L., and Nelson, L. M. (2017). Postharvest biological control of blue mold of apple by Pseudomonas fluorescens during commercial storage and potential modes of action. Postharvest Biol. Technol. 133, 1–11. doi: 10.1016/j.postharvbio.2017.07.003
Wang, S. S., Liu, J. M., Sun, J., Sun, Y. F., Liu, J. N., Jia, N., et al. (2019). Diversity of culture-independent bacteria and antimicrobial activity of culturable endophytic bacteria isolated from different Dendrobium stems. Sci. Rep. 9:10389. doi: 10.1038/s41598-019-46863-9
Wang, Z., Mei, X., Du, M., Chen, K., Jiang, M., Wang, K., et al. (2020). Potential modes of action of Pseudomonas fluorescens ZX during biocontrol of blue mold decay on postharvest citrus. J. Sci. Food Agric. 100, 744–754. doi: 10.1002/jsfa.10079
Keywords: genomic analysis, sustainable agriculture, fungal antagonism, postharvest disease, volatile organic compound
Citation: Morales-Cedeño LR, de los Santos-Villalobos S and Santoyo G (2021) Functional and Genomic Analysis of Rouxiella badensis SER3 as a Novel Biocontrol Agent of Fungal Pathogens. Front. Microbiol. 12:709855. doi: 10.3389/fmicb.2021.709855
Received: 14 May 2021; Accepted: 12 July 2021;
Published: 05 August 2021.
Edited by:
Khamis Youssef, Agricultural Research Center, EgyptReviewed by:
Samir Jaoua, Qatar University, QatarChunpeng (Craig) Wan, Jiangxi Agricultural University, China
Copyright © 2021 Morales-Cedeño, de los Santos-Villalobos and Santoyo. This is an open-access article distributed under the terms of the Creative Commons Attribution License (CC BY). The use, distribution or reproduction in other forums is permitted, provided the original author(s) and the copyright owner(s) are credited and that the original publication in this journal is cited, in accordance with accepted academic practice. No use, distribution or reproduction is permitted which does not comply with these terms.
*Correspondence: Gustavo Santoyo, Z3VzdGF2by5zYW50b3lvQHVtaWNoLm14