Corrigendum: The selective advantage of the lac operon for Escherichia coli is conditional on diet and microbiota composition
- 1CEDOC, Chronic Diseases Research Centre, NOVA Medical School, Faculdade de Ciências Médicas, Universidade NOVA de Lisboa, Lisbon, Portugal
- 2Department of Medical Sciences, Institute of Biomedicine (iBiMED), University of Aveiro, Aveiro, Portugal
- 3Instituto Gulbenkian de Ciência, Oeiras, Portugal
The lac operon is one of the best known gene regulatory circuits and constitutes a landmark example of how bacteria tune their metabolism to nutritional conditions. It is nearly ubiquitous in Escherichia coli strains justifying the use of its phenotype, the ability to consume lactose, for species identification. Lactose is the primary sugar found in milk, which is abundant in mammals during the first weeks of life. However, lactose is virtually non-existent after the weaning period, with humans being an exception as many consume dairy products throughout their lives. The absence of lactose during adulthood in most mammals and the rarity of lactose in the environment, means that the selective pressure for maintaining the lac operon could be weak for long periods of time. Despite the ability to metabolize lactose being a hallmark of E. coli’s success when colonizing its primary habitat, the mammalian intestine, the selective value of this trait remains unknown in this ecosystem during adulthood. Here we determine the competitive advantage conferred by the lac operon to a commensal strain of E. coli when colonizing the mouse gut. We find that its benefit, which can be as high as 11%, is contingent on the presence of lactose in the diet and on the presence of other microbiota members in the gut, but the operon is never deleterious. These results help explaining the pervasiveness of the lac operon in E. coli, but also its polymorphism, as lac-negative E. coli strains albeit rare can naturally occur in the gut.
Introduction
The lac operon, first described by Jacob and Monod (1961), codes for the cellular machinery to transport and metabolize lactose. It is constituted by three structural genes: lacZ codes for the lactose-degrading enzyme β-galactosidase, that breaks down lactose into glucose and galactose; lacY encodes the β-galactoside permease, that facilitates lactose transport into the cell; and lacA encodes galactoside transacetylase, whose function in lactose metabolism remains unknown (Müller-Hill, 1996b; Roderick, 2005). The lac operon is expressed under the presence of lactose and low levels of glucose (Müller-Hill, 1996a), being the textbook example of gene regulation at the transcriptional level in prokaryotes. As it is present in the vast majority of Escherichia coli strains (Stoebel, 2005), the phenotype of lactose consumption was used to identify E. coli among environmental samples (Hartl and Dykhuizen, 1984).
Lactose is the primary sugar in milk (Holsinger, 1988) and abundant in the mammalian gut during breastfeeding. However, lactose is virtually absent after that period. In fact, mammals become lactose-intolerant in adulthood, except for about a third of the human population, which exhibits lactase persistence into adulthood resulting from selection in dairy farming cultures (Storhaug et al., 2017). Thus, given the absence of lactose during adulthood in most mammals and also its scarcity in the environment, the selective pressure for preserving the lac operon could be weak. Yet, β-galactosidase can also degrade other compounds, including galactosylglycerols, which result from the pancreatic degradation of galactolipids (Egel, 1979, 1988). As galactolipids are main components of the chloroplast membranes, the frequent ingestion of green leaves could act as a selective pressure to maintain the lac operon in E. coli beyond the breastfeeding period. Studies on the origin of the operon were not able to trace its occurrence to a horizontal gene transfer event (Stoebel, 2005), further supporting a continuous pressure to keep the operon and the hypothesis of it being an important component of the species identity.
E. coli’s primary habitat is the mucus layer of the mammalian intestinal tract, where it grows in a multi-species biofilm and accounts for around 0.1% of the human gut microbiota population (Raman et al., 2005). E. coli, together with streptococci, is one of the first bacteria to colonize the intestinal track, being established as soon as 48 h after birth (Mackie et al., 1999). The large amounts of lactose present in the gut at this stage might confer E. coli an advantage for colonizing this environment (Ochman et al., 2000; Blount, 2015).
Still, the actual selective value of the lac operon for E. coli in this niche, whether it increases in the presence of lactose, and how it changes according to microbiota composition, remains undetermined. To tackle these questions, we determined the competitive advantage given by the lac operon to a commensal strain of E. coli when colonizing the mouse intestine both in the presence and absence of lactose. We found that the ability to consume lactose is mainly beneficial in the presence of lactose and that this benefit can have an individualized effect, possibly reflecting differences in microbiota composition.
To disentangle the effect of lactose from the effect of the microbiota we next performed similar competitions in animals devoid of microbiota [germ free (GF) mice]. To our surprise, the lac operon was neutral in this scenario, irrespectively of the presence of lactose. We hypothesized that the overabundance of nutritional sources and the lack of interspecies competition could be responsible for this result. We corroborate this hypothesis via a series of competitions varying the level of inter and intraspecies competition and show that the selective benefit of the lac operon depends on the presence of lactose and also gradually increases with the number of different species present in the gut.
Materials and Methods
Mice
All experiments were conducted using female 6–8 weeks old C57BL6/6J mice under germ free (GF) or specific pathogen-free (SPF) conditions at the Instituto Gulbenkian de Ciência (IGC) animal facilities. Mice were individually caged and had access to water and food ad libitum. GF mice were bred and raised at the IGC gnotobiology facility in dedicated axenic isolators (La Calhene/ORM). Young adults were transferred into sterile ISOcages (Tecniplast) before the competition experiments. This research project was approved by both the Ethics Committee and the IGC Animal Welfare Body (license reference: A009.2018), and by the Portuguese National Entity that regulates the use of laboratory animals (DGAV—Direção Geral de Alimentacão e Veterinária; license reference: 009676). All experiments were performed following the Portuguese (Decreto-Lei n° 113/2013) and European (Directive 2010/63/EU) legislations concerning animal welfare.
Bacterial Strains
The two E. coli strains used in this work were derived from E. coli K-12 MG1655 and were resistant to streptomycin due to the point mutation rpsL K43T (StrR). The two strains are isogenic except for the deletion of the lacZ (ΔlacZ, lac−), differing only in the ability to metabolize lactose. L. murinus was isolated from feces of SPF mice and species identity was confirmed by sequencing the whole 16S rRNA gene. Bacteroides thetaiotaomicron strain (VPI-5482) was acquired from the German Collection of Microorganisms and Cell Cultures (DSMZ 2079).
Mouse Gut Colonizations
To colonize the mouse intestine, we used a streptomycin treated colonization model (Conway et al., 2004) under two antibiotic regimes, i.e., treatment lasted for 1 or 7 days. GF animals were treated continuously with streptomycin. SPF animals were colonized with E. coli, while GF mice were either mono-colonized (E. coli), double-colonized (E. coli + L. murinus), or triple-colonized (E. coli + L. murinus + B. thetaiotaomicron). In all colonization conditions, mice were given autoclaved drinking water supplemented with streptomycin (5 g/L) for 24 h.
After 4 h of starvation for water and food, animals were gavaged with 100 μL of a suspension of 108 colony forming units (CFUs) of a mixture (1:1) of lac− and lac + (SPF and mono-colonization). Double and triple colonizations started with the gavage of 100 μL of a suspension of 108 CFU containing equal amounts of E. coli and each of the other species.
Bacteria for gavage were prepared as follows: E. coli was grown in LB broth overnight with aeration followed by a 1:100 dilution and grown in BHI broth until reaching an optical density of 2. L. murinus was inoculated in De Man, Rogosa and Sharpe (MRS) broth and incubated in anaerobiosis. B. thetaiotaomicron was inoculated in Chopped Meat Medium with Carbohydrates and grown in anaerobiosis. All bacteria were incubated overnight at 37°C and 1 mL of each culture was centrifuged and resuspended in PBS 1x + 0.1% cysteine.
Water with streptomycin was maintained throughout the 6 days of experiment and replaced after 3 days, except for the colonization where streptomycin was only administered for 24 h before gavage. In half of the animals, the water was also supplemented with 2% lactose. This percentage was chosen to mimic to the concentration found in mice milk (Görs et al., 2009). Fecal samples were collected and weighed daily. Samples were then suspended in PBS 1x and appropriate dilutions were then inoculated in MacConkey agar supplemented with 100 μg/mL of streptomycin (for E. coli) and MRS agar (for L. murinus) or in LB agar with X-Gal and 100 μg/mL of streptomycin. E. coli and L. murinus were grown at 37°C for 18 and 24 h, respectively in aerobiosis. When grown in MacConkey, E. coli lac + and lac− strains were discriminated by colony color (red colonies are lac +, white colonies are lac−).
Competitive Fitness Assays in vivo to Measure the Selective Coefficient of the lac Operon
To assess if the lac operon confers an advantage to E. coli in the tested conditions, total numbers and relative frequencies of the bacteria were determined by counting the number of colonies of each strain. The selection coefficient (fitness gain) per generation of the lac + strain in vivo was calculated as the slope of the linear regression of ln (freqlac+/freqlac–) divided by the number of generations for E. coli per day. The number of days considered to calculate the selection coefficient in the colonization of SPF mice (1 day of streptomycin) was 2 (n = 3), 3 (n = 1), 4 (n = 3), or 5 (n = 1), to use a time interval with a linear tendency of selection. For the remaining colonizations, the first 3 days were considered. As for the number of generations per day, we considered this value to be 18 based on the previous estimation of 80-min generation time for E. coli in streptomycin-treated mice (Rang et al., 1999).
Microbiota Analysis
To characterize the effect of different streptomycin regimes in the microbiota, we analyzed the V4 region of 16S rRNA gene sequenced from fecal samples from young animals before and after antibiotic treatment (days 7/8 of the evolution experiment). 16S sequence data from animals treated with streptomycin for only 1 day is available from Frazão et al. (2019) and 16S sequence data from animals treated with continuous streptomycin is available from Barreto et al. (2020).
Raw reads were processed using QIIME2 version 2020.8 with default parameters (Bolyen et al., 2019). Deblur was used for quality filtering and denoising (Amir et al., 2017). The generated table of ASVs was then used in the R package phyloseq (McMurdie and Holmes, 2013) to determine the Bray-Curtis dissimilarity index, using rarefaction based on the sample with the lowest sequencing depth. Within-group distance based on Bray-Curtis was calculated in QIIME2.
The observed ASVs were also calculated in QIIME2 with diversity core-metrics-phylogenetic and diversity alpha-group-significance, also using rarefaction based on the sample with the lowest sequencing depth.
Statistical Analysis
All graphical representations were performed in GraphPad Prism 8.2.1 for Mac1 (GraphPad Software, San Diego, California, United States) and statistical analysis in Prism or in R version 3.6.3 (R Core Team, 2020). Normal data distribution was assessed using the Shapiro-Wilk test and QQ plots observation, and the homogeneity of variances using Levene’s test. Statistical significance was defined for p < 0.05. Significance levels were defined as: ∗p < 0.05, ∗∗p < 0.01, ∗∗∗p < 0.001, ****p < 0.0001. The detailed statistics and sample size for each experiment are described in the figure legends.
Results
The Selective Advantage of the lac Operon Is Stronger on the Presence of Lactose and Reflects the Amount of Competition
The selective advantage conferred by the ability to metabolize a given nutrient is expected to change with its relative abundance, its energetic potential, and the amount of competition for that nutrient.
Here we evaluated the selective advantage conferred by the lac operon to an E. coli strain (lac +), by allowing for its competition with a lac− strain in the intestine of mice after a short treatment (1 day) with streptomycin. This procedure maintains a complex microbiota while allowing for E. coli colonization (Frazão et al., 2019). We started by colonizing 8 animals with a mixture of 1:1 (lac + and lac−) isogenic strains, except for the deletion of the lacZ in the lac- strain, by oral gavage. We further supplemented the diet of 4 animals with 2% lactose whereas the remaining 4 animals were fed regular chow. The frequencies of lac +/lac− strains were assessed by daily collecting fecal samples and plating appropriate dilutions for 6 days.
During this period, in the absence of lactose, the frequency of lac + remained close to the initial 50% in two animals and increased slightly in the other two (Figures 1A,B), showing a mean advantage of 1 ± 0.6% (SEM) (Figure 2).
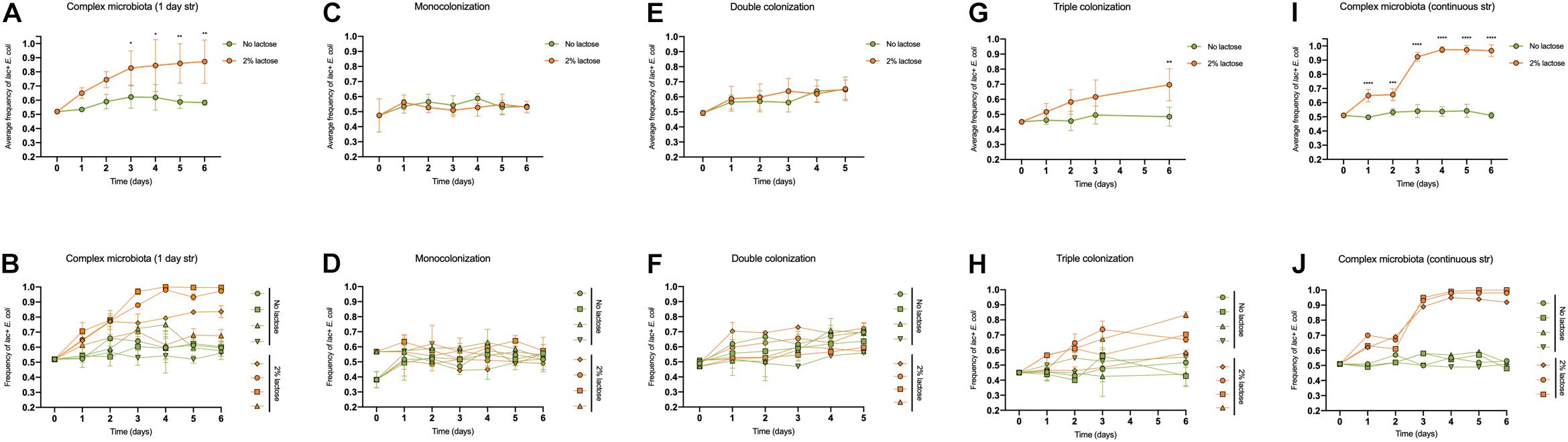
Figure 1. The advantage of the lac operon is dependent on diet and the microbiota composition. Frequency of lac + E. coli when the diet is supplemented or not with lactose, during the first 6 days of colonization of the mouse gut, with microbiotas of different complexity: (A,B) complex microbiota (SPF mice) after 1 day of streptomycin (n = 4 per group); (C,D) monocolonization with E. coli (n = 4 per group); (E,F) double colonization with E. coli and L. murinus (n = 4 per group); (G,H) triple colonization with E. coli, L. murinus and B. thetataiomicron (n = 4 per group); (I,J) complex microbiota (SPF mice) with continuous streptomycin treatment (n = 3–4 per group). In the top panels (A,C,E,G,I), values are represented as mean ± SD. In the bottom panels, each animal is individually represented, and the error bars correspond to experimental replicates (n = 3). The effects of the microbiota composition and time on the lac + E. coli frequency were tested by a linear mixed model followed by a type III ANOVA and post hoc contrasts with Bonferroni’s correction.
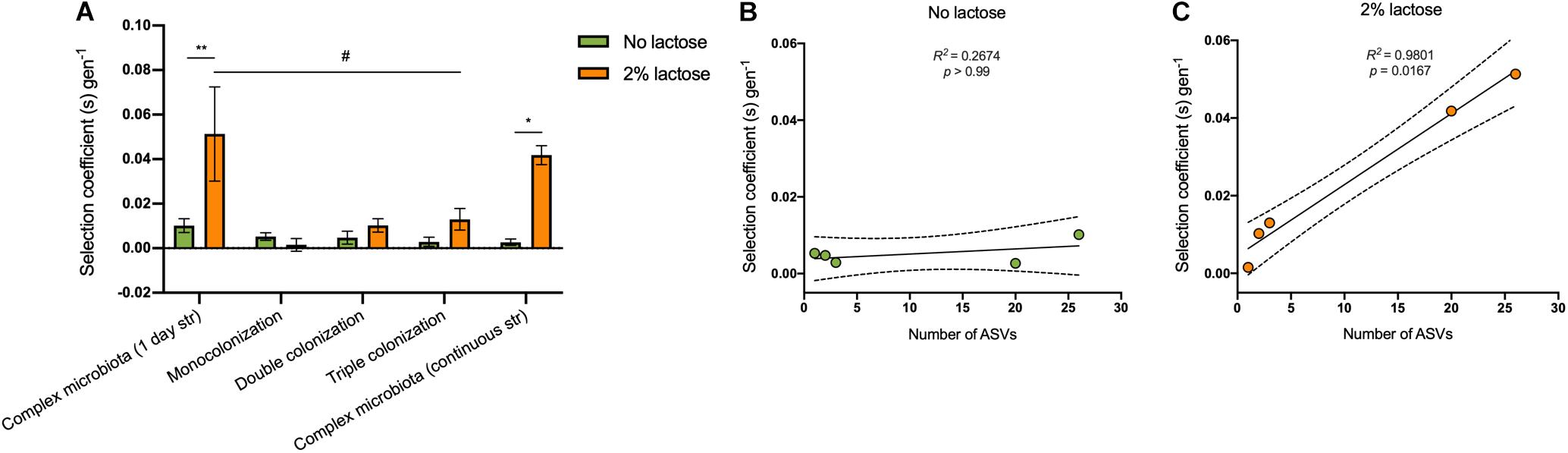
Figure 2. The selective advantage provided by the lac operon in the presence of lactose increases with microbiota complexity. (A) Selection coefficient per generation of the lac + E. coli strain when the diet is supplemented or not with lactose, with microbiotas of different complexity: Complex microbiota (SPF mice) after 1 day of streptomycin (n = 4 per group); Monocolonization with E. coli (n = 4 per group); double colonization with E. coli and L. murinus (n = 4 per group); triple colonization with E. coli, L. murinus and B. thetataiomicron (n = 4 per group); complex microbiota (SPF mice) with continuous streptomycin treatment (n = 3–4 per group). Values are represented as mean ± SEM. The effects of the microbiota composition and lactose on the selection coefficient were tested by a Two-Way ANOVA followed by post hoc selected contrasts with Bonferroni’s correction. (B,C) Linear regression of the number of ASVs vs. the selection coefficient per generation in the (B) absence and (C) presence of lactose. P-values refer to the significance of the Spearman’s correlation. The dashed lines represent the 95% confidence intervals. *p < 0.05, **p < 0.01 refers to comparisons between the presence or absence of lactose within each microbiota composition. #p < 0.05 refers to comparisons between the different microbiota compositions in the presence of lactose.
The presence of lactose had a significant effect in the lac + frequency over time [Figures 1A,B, F(6, 36) = 5.63, p = 0.0003] and a more variable effect was observed for the selective advantage of the lac + strain, ranging from 1.4 to 11%, with a mean of 5 ± 4% (SEM) (Figure 2).
Besides the presence of lactose, differences between measurements likely reflect the common variation in microbiota composition between individuals which is known to further increase with the antibiotic treatment (Leónidas Cardoso et al., 2020). The effect of the chosen antibiotic regime was assessed by estimating the Bray-Curtis beta diversity, a measure of group heterogeneity, before and after antibiotic treatment in an independent set of 5 animals. A mean increase of ∼30% in Bray-Curtis diversity was observed after antibiotic treatment (Supplementary Figures 1A,B) possibly contributing to the variation in the fitness effect of the lac operon in the gut of different mice.
These results led us to conclude that the lac operon can afford a significant advantage to an E. coli strain while colonizing the intestine, but its benefit is influenced by lactose and possibly microbiota composition.
To isolate the effect of lactose and test for the influence of a microbiota diversity to the selective coefficient of the lac operon, we engaged in a series of colonizations within a range of microbiota diversities. These comprised a mono, double and a triple colonization. A group of animals continuously treated with antibiotic (7 days) was further included, representing a complex yet simpler microbiota than the group treated only for a short period (1 day).
Although not statistically significant, after antibiotic treatment the two regimes differed in microbiota richness, with an average of 20 (continuous regime) and 26 (1 day regime) ASVs (Supplementary Figure 1C and Supplementary Table 1).
These microbiota compositions likely represent different levels of competition to E. coli, which could influence the abundance of lactose and other nutritional sources.
To reduce competition to a minimum we monoassociated GF animals with a mixture of 1:1 (lac +/lac−) E. coli and followed its frequency for a period of 6 days. As before, a group of animals received a lactose supplemented diet (n = 4) whereas the remaining animals were fed regular chow. Interestingly we observed that when E. coli is the only inhabitant of the gut, the ability to metabolize lactose, irrespectively of the presence of its cognate substrate, is a neutral trait (Figures 1C,D, 2).
Though it could be hypothesized that in this simple environment the available glucose could preclude the expression of the lac operon by catabolite repression this seems unlikely since glucose is less abundant in the cecum of monocolonized than in SPF mice (Barroso-Batista et al., 2020), where the lac operon is presumably expressed.
We proceeded by co-colonizing GF animals with E. coli and similar numbers of a representative species of Firmicutes, Lactobacilus murinus, which belongs to lactic acid bacteria, thus can compete for lactose (de Vos and Vaughan, 1994). As before, the lac operon conferred no particular advantage both in the presence or absence of lactose (Figures 1E,F, 2).
Therefore, to further increase the competition level, we performed a triple colonization of GF animals with equal amounts of E. coli, L. murinus, and Bacteroides thetaiotaomicron, which is a representative species of the phylum Bacteroidetes, usually present in large numbers in the gut microbiota and that can also compete for lactose (Chia et al., 2020).
The addition of a third species was enough to restore the advantage of the lac operon in the presence of lactose [Figures 1G,H, F(4, 24) = 4.41, p = 0.0082], providing a selective advantage around 1.3 ± 1% (SEM) per generation (Figure 2). Nevertheless, we cannot rule out the possibility that B. thetaiotaomicron per se, and not just the number of species, was responsible for the increase in the effect of the lac operon since we did not perform a colonization including only E. coli and B. thetaiotaomicron.
Coherently, in the context of continuously antibiotic treated mice and in the presence of lactose, the effect of the lac operon was further increased showing a mean advantage of 4.2 ± 1% per generation (Figure 2) which allowed it to reach a frequency close to fixation by day 4 (Figures 1I,J). Interestingly, the variation in lac + selective coefficient between animals was also reduced in comparison to the 1 day antibiotic regime (Figure 2), in agreement with the smaller effect of the antibiotic in group heterogeneity in the continuous regime [Supplementary Figures 1A,B, p = 0.0007 (Kruskal-Wallis test, post hoc comparisons with Dunn’s correction)].
Globally, these experiments demonstrate that the benefit of the lac operon is potentiated by the presence of lactose and increases with the number of co-colonizers (Figures 2B,C).
The Absolute Success of E. coli in the Gut Depends on the Net Result Between Synergism and Competition
Increasing microbiota diversity likely promotes competition but it also raises the probability of synergism, e.g., via cross-feeding.
Here we observe that E. coli’s abundance (measured by its loads—CFU/g of feces) increases with the number of co-colonizers in ex-GF mice, but then it drops when the gut environment reaches the complexity of a regular microbiota (Figure 3). Specifically, when comparing the double with the mono colonization we observed a non-significant but sustained increase in the average loads of E. coli in the double colonization in 4 out of the 5 days of the experiment (Figure 3). Upon 5 days of colonization E. coli was on average 3 times more abundant in the double (∼4.4 × 109 CFU/g) than in the mono colonization (∼1.3 × 109 CFU/g).
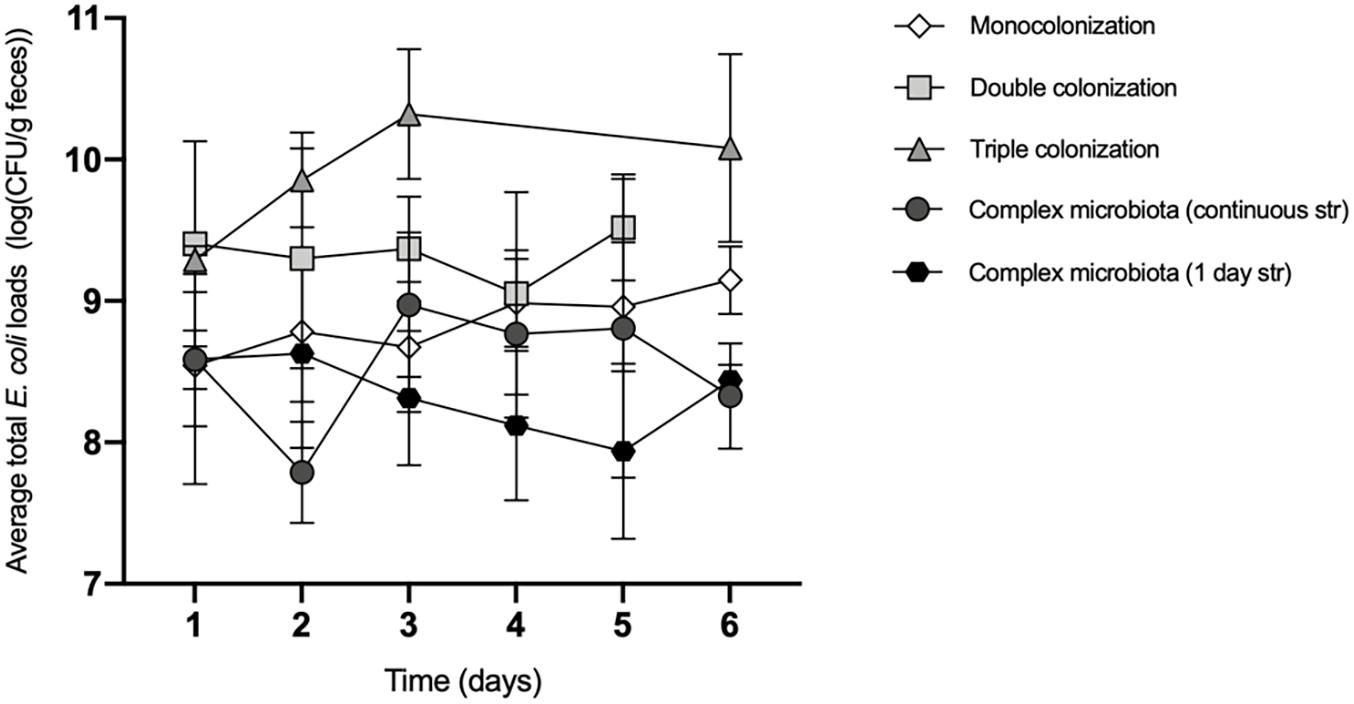
Figure 3. The total E. coli loads vary with the composition of the microbiota. Average E. coli total loads (log10 transformed) during the first 6 days of colonization of the mouse gut, with increasing microbiota complexity: Monocolonization with E. coli, n = 8; Double colonization with E. coli and L. murinus, n = 6; Triple colonization with E. coli, L. murinus and B. thetataiomicron, n = 8; Complex microbiota with continuous streptomycin treatment, n = 7; Complex microbiota after 1 day of streptomycin, n = 8. Values are represented as mean ± SD. The effects of the microbiota composition and time on the total E. coli loads were tested by a linear mixed model followed by type III ANOVA. Post hoc comparisons were performed between all the levels of microbiota complexity for day 5 and 6 (Bonferroni’s correction). In day 5, E. coli loads in the complex microbiota (continuous streptomycin) are significantly lower than: the monocolonization (p = 0.0036); double colonization (p < 0.0001); and the complex microbiota (continuous str) (p = 0.038). In day 6, E. coli loads in the triple colonization are higher than the monocolonization (p = 0.0117) and the complex microbiota (both 1 day and continuous str) (p < 0.0001).
The sharpest increase was observed when comparing the mono with the triple colonization where a significant difference (∼10 times) in the average loads of E. coli was measured (2 × 1010 and 1.6 × 109 CFU/g at the 6th day of the experiment, in the triple and mono colonization, respectively (p = 0.012, post hoc comparison with Bonferroni’s correction).
These data suggest that E. coli’s niche is expanded by the presence of L. murinus and B. thetaiotaomicron, hinting at a synergistic relationship between these bacteria and E. coli.
In contrast, the expansion of E. coli in an environment with multiple species of bacteria, such as the microbiota of streptomycin-treated mice, regardless of the antibiotic regime, was limited to ∼108 CFU/g upon 6 days of colonization, likely due to the predominance of interspecies competition.
These differences are similar for the lac + and lac− strains separately (Supplementary Figure 2) and are independent of the presence of lactose in the mouse diet (Supplementary Figure 3).
Discussion
The nearly ubiquitous presence of the lac operon in E. coli and the restricted timeframe of lactose availability in its natural environment, the mammalian gut, has for long raised questions about the adaptive value of the lac operon in E. coli. Breastfeeding represents only a brief period in mammalians’ life, but it is particularly influential in determining the first stages of microbiota establishment (Milani et al., 2017), possibly with long-term effects. E. coli represents a small fraction of the adult microbiota (Raman et al., 2005), but is among the first colonizers of the gut (Milani et al., 2017) and therefore, consuming lactose, could in principle, contributes to its success. In contrast, it has it has been shown that lactose consumption is not essential for E. coli to colonize the intestine of streptomycin-treated SPF CD-1 mice, while essential sugars for either the initiation or maintenance of gut colonization are arabinose, fucose, gluconate, N-acetyl-glucosamine, and N-acetyl-neuraminic acid (Fabich et al., 2008).
In our work, by allowing for direct competition between lac + and lac− E. coli strains in the adult mammalian gut we found that, in the presence of lactose, the lac operon confers a selective advantage that can be as high as 11%, while being close to neutral in its absence. Therefore, our results suggest that the strong interspecies competition for nutritional resources that occurs in these settings (Coyte et al., 2015) considerably increases the advantage for lactose consumers, particularly in the presence of lactose.
The emergence of constitutive mutants for lactose consumption during the first days of life was observed in a controlled experiment (Ghalayini et al., 2019), further supporting the hypothesis that the lac operon is under strong selection during this period, which in the long term could help E. coli to secure a place in the complex adult microbiota.
Besides the presence of lactose, the fitness advantage of the lac operon for E. coli was also shown to depend on microbiota composition, particularly on the number of species present. One way to interpret this result is to consider that an increasing number of species increases the opportunity for more competitive interactions. Thus, the ability to consume lactose would become a bigger advantage as other nutritional sources get depleted by the growing number of competitors. On the other hand, as the number of species increases, so does the opportunity for synergism, with the absolute success of E. coli reflecting the net result between these two forms of ecological interactions.
The observation that the population size of E. coli substantially increased in the triple-colonization, in comparison to the situation where it is alone (mono-colonization), showed us that, from the perspective of E. coli, synergism dominated in this scenario. This is consistent with the notion that E. coli consumes the byproducts of complex nutrients’ degraded by B. thetaiotaomicron, such as maltose and fucose which result from the breakdown of dietary glycans (Chang et al., 2004; Li et al., 2015; Baümler and Sperandio, 2016). This seems to occur regardless of the ability to metabolize lactose, and it is independent of the availability of that additional carbohydrate.
In contrast to our findings, in a scenario of DSS-induced colitis E. coli was antagonized by B. thetaiotaomicron and another species of Lactobacillus (L. johnsonii). Here the oral administration of these species was enough to reduce the overgrowth of E. coli (Charlet et al., 2020). The higher microbiota complexity of the animals in the referred study when compared to ours, as well as potentially different strains, may account for this difference.
Conversely, in the presence of a complex microbiota (several tens of species), its absolute success decreased and the most likely scenario was one dominated by competition (Coyte et al., 2015). A scenario where the population size of E. coli in the gut is inversely correlated with microbiota diversity has been previously observed in more than one occasion (Sousa et al., 2017).
Surprisingly, when comparing the mono with the triple colonization, lactose showed to be more advantageous in the situation where E. coli’s absolute success was higher, suggesting that the fitness effect of the lac operon may be density dependent. Future work should address this issue, possibly through investigating the geographic distribution of bacteria abundances and possible differences in the lac operon selective advantage along the intestinal tract. Since lactose is mainly absorbed in the small intestine, this could be the place where lac + is positively selected with the cecum being the intestinal compartment where E. coli expands irrespectively of its ability to consume lactose. Such a hypothetical situation would simultaneously explain the larger advantage of a lac + strain and the higher absolute success of the species.
Another interesting observation was the correlation between the variance of the lac operon selective effect and microbiota heterogeneity. This was unraveled by the two antibiotic regimes but probably best resemble the natural diversity of the microbiota of animals which are not genetically homogenous or eat the same controlled diet as in our experimental setup.
Overall, the results indicate that the specificity of interactions established by E. coli, and, therefore, the balance between beneficial and antagonistic interactions, determines its niche size and location. The adaptive value of the lac operon for E. coli is dependent on the competitors and the availability of nutritional resources and might be especially useful in highly competitive environments and for exploring new niches.
Data Availability Statement
The original contributions presented in the study are included in the article/Supplementary Material, further inquiries can be directed to the corresponding author/s.
Ethics Statement
The animal study was reviewed and approved by Direção Geral de Alimentacão e Veterinária (license reference: 009676) and Instituto Gulbenkian de Ciência Animal Welfare Body (license reference: A009.2018).
Author Contributions
CP, IG, and AS conceived and designed the experiments. CP performed the experiments. CP, RM-M, and AS analyzed the data. IG and AS contributed reagents, materials, and analysis tools. RM-M and AS wrote the manuscript. CP and IG contributed to the writing of the manuscript. All authors contributed to the article and approved the submitted version.
Funding
This work was funded by Fundação para a Ciência e Tecnologia PTDC/BIA-EVF/3247/2012, PTDC/BIA-EVL/30212/2017, and Programa Operacional Regional do Centro, through Fundo Europeu de Desenvolvimento Regional-FEDER (CENTRO-01-0145-FEDER-30212), and by iBiMED (UID/BIM/04501/2019, project POCI-01-0145-FEDER-007628).
Conflict of Interest
The authors declare that the research was conducted in the absence of any commercial or financial relationships that could be construed as a potential conflict of interest.
Acknowledgments
We would like to acknowledge Karina Bivar Xavier for providing the strain Bacteroides thetaiotaomicron.
Supplementary Material
The Supplementary Material for this article can be found online at: https://www.frontiersin.org/articles/10.3389/fmicb.2021.709259/full#supplementary-material
Footnotes
References
Amir, A., Daniel, M., Navas-Molina, J., Kopylova, E., Morton, J., Xu, Z. Z., et al. (2017). Deblur rapidly resolves single-nucleotide community sequence patterns. Am. Soc. Microbiol. 2, 1–7.
Barreto, H. C., Sousa, A., and Gordo, I. (2020). The landscape of adaptive evolution of a gut commensal bacteria in aging Mice. Curr. Biol. 30, 1102.e5–1109.e5. doi: 10.1016/j.cub.2020.01.037
Barroso-Batista, J., Pedro, M. F., Sales-Dias, J., Pinto, C. J. G., Thompson, J. A., Pereira, H., et al. (2020). Specific eco-evolutionary contexts in the mouse gut reveal Escherichia coli metabolic versatility. Curr. Biol. 30, 1049.e7–1062.e7. doi: 10.1016/j.cub.2020.01.050
Baümler, A. J., and Sperandio, V. (2016). Interactions between the microbiota and pathogenic bacteria in the gut. Nature 535, 85–93. doi: 10.1038/nature18849
Bolyen, E., Rideout, J. R., Dillon, M. R., Bokulich, N. A., Abnet, C. C., Al-Ghalith, G. A., et al. (2019). Reproducible, interactive, scalable and extensible microbiome data science using QIIME 2. Nat. Biotechnol. 37, 852–857. doi: 10.1038/s41587-019-0209-9
Chang, D. E., Smalley, D. J., Tucker, D. L., Leatham, M. P., Norris, W. E., Stevenson, S. J., et al. (2004). Carbon nutrition of Escherichia coli in the mouse intestine. Proc. Natl. Acad. Sci. U.S.A. 101, 7427–7432. doi: 10.1073/pnas.0307888101
Charlet, R., Bortolus, C., Sendid, B., and Jawhara, S. (2020). Bacteroides thetaiotaomicron and Lactobacillus johnsonii modulate intestinal inflammation and eliminate fungi via enzymatic hydrolysis of the fungal cell wall. Sci. Rep. 10, 1–13. doi: 10.1038/s41598-020-68214-9
Chia, L. W., Mank, M., Blijenberg, B., Aalvink, S., Bongers, R. S., Stahl, B., et al. (2020). Bacteroides thetaiotaomicron fosters the growth of butyrate-producing Anaerostipes caccae in the presence of lactose and total human milk carbohydrates. Microorganisms 8:1513.
Conway, T., Krogfelt, K. A., and Cohen, P. S. (2004). The life of commensal Escherichia coli in the mammalian intestine. EcoSal Plus 1, 1–16. doi: 10.1128/ecosalplus.8.3.1.2
Coyte, K. Z., Schluter, J., and Foster, K. R. (2015). The ecology of the microbiome: networks, competition, and stability. Science 350, 663–666. doi: 10.1126/science.aad2602
de Vos, W. M., and Vaughan, E. E. (1994). Genetics of lactose utilization in lactic acid bacteria. FEMS Microbiol. Rev. 15, 217–237. doi: 10.1016/0168-6445(94)90114-7
Egel, R. (1979). The lac-operon for lactose degradation, or rather for the utilization of galactosylglycerols from galactolipids? J. Theor. Biol. 79, 117–119. doi: 10.1016/0022-5193(79)90260-1
Fabich, A. J., Jones, S. A., Chowdhury, F. Z., Cernosek, A., Anderson, A., Smalley, D., et al. (2008). Comparison of carbon nutrition for pathogenic and commensal Escherichia coli strains in the mouse intestine. Infect. Immun. 76, 1143–1152. doi: 10.1128/IAI.01386-07
Frazão, N., Sousa, A., Lässig, M., and Gordo, I. (2019). Horizontal gene transfer overrides mutation in Escherichia coli colonizing the mammalian gut. Proc. Natl. Acad. Sci. U.S.A. 116, 17906–17915. doi: 10.1073/pnas.1906958116
Ghalayini, M., Magnan, M., Dion, S., Zatout, O., Bourguignon, L., Tenaillon, O., et al. (2019). Long-term evolution of the natural isolate of Escherichia coli 536 in the mouse gut colonized after maternal transmission reveals convergence in the constitutive expression of the lactose operon. Mol. Ecol. 28, 4470–4485. doi: 10.1111/mec.15232
Görs, S., Kucia, M., Langhammer, M., Junghans, P., and Metges, C. C. (2009). Technical note: milk composition in mice - Methodological aspects and effects of mouse strain and lactation day. J. Dairy Sci. 92, 632–637. doi: 10.3168/jds.2008-1563
Hartl, D. L., and Dykhuizen, D. E. (1984). The population genetics of Escherichia coli. Annu. Rev. Genet. 18, 31–68. doi: 10.1146/annurev.ge.18.120184.000335
Holsinger, V. H. (1988). “Lactose,” in Fundamentals of Dairy Chemistry, eds N. P. Wong, R. Jenness, M. Keeney, and E. H. Marth (New York, NY: Van Nostrand Reinhold Company Inc), 583–607. doi: 10.1007/978-1-4615-7050-9_11
Jacob, F., and Monod, J. (1961). Genetic regulatory mechanisms in the synthesis of proteins. J. Mol. Biol. 3, 318–356. doi: 10.1016/S0022-2836(61)80072-7
Leónidas Cardoso, L., Durão, P., Amicone, M., and Gordo, I. (2020). Dysbiosis individualizes the fitness effect of antibiotic resistance in the mammalian gut. Nat. Ecol. Evol. 4, 1268–1278. doi: 10.1038/s41559-020-1235-1
Li, H., Limenitakis, J. P., Fuhrer, T., Geuking, M. B., Lawson, M. A., Wyss, M., et al. (2015). The outer mucus layer hosts a distinct intestinal microbial niche. Nat. Commun. 6:8292. doi: 10.1038/ncomms9292
Mackie, R. I., Sghir, A., and Gaskins, H. R. (1999). Developmental microbial ecology of the neonatal gastrointestinal tract. Am. J. Clin. Nutr. 69, 1035S–1045S. doi: 10.1093/ajcn/69.5.1035s
McMurdie, P. J., and Holmes, S. (2013). Phyloseq: an R package for reproducible interactive analysis and graphics of microbiome census data. PLoS One 8:e0061217. doi: 10.1371/journal.pone.0061217
Milani, C., Duranti, S., Bottacini, F., Casey, E., Turroni, F., Mahony, J., et al. (2017). The first microbial colonizers of the human gut: composition, activities, and health implications of the infant gut microbiota. Microbiol. Mol. Biol. Rev. 81, 1–67. doi: 10.1128/mmbr.00036-17
Müller-Hill, B. (1996a). “Activation of the lac promoter by CAP protein,” in The Lac Operon: A Short History of a Genetic Paradigm, ed. Berlin (New York, NY: Walter de Gruyter), 137–141.
Müller-Hill, B. (1996b). “ß-Galactosidase, lac permease and transacetylase,” in The Lac Operon: A Short History of a Genetic Paradigm, ed. Berlin (New York, NY: Walter de Gruyter), 181.
Ochman, H., Lawrence, J. G., and Groisman, E. A. (2000). Lateral gene transfer and the nature of bacterial innovation. Nature 405, 299–304. doi: 10.1038/35012500
Raman, R., Thomas, R. G., Weiner, M. W., Jack, C. R., Ernstrom, K., Aisen, P. S., et al. (2005). Diversity of the human intestinal microbial Flora. Science 308, 1635–1638.
Rang, C. U., Licht, T. R., Midtvedt, T., Conway, P. L., Chao, L., Krogfelt, K. A., et al. (1999). Estimation of growth rates of Escherichia coli BJ4 in streptomycin- treated and previously germfree mice by in situ rRNA hybridization. Clin. Diagn. Lab. Immunol. 6, 434–436. doi: 10.1128/cdli.6.3.434-436.1999
Roderick, S. L. (2005). The lac operon galactoside acetyltransferase. Compt. Ren. Biol. 328, 568–575. doi: 10.1016/j.crvi.2005.03.005
Sousa, A., Ramiro, R. S., Barroso-Batista, J., Güleresi, D., Lourenčo, M., and Gordo, I. (2017). Recurrent reverse evolution maintains polymorphism after strong bottlenecks in commensal gut bacteria. Mol. Biol. Evol. 34, 2879–2892. doi: 10.1093/molbev/msx221
Stoebel, D. M. (2005). Lack of evidence for horizontal transfer of the lac operon into Escherichia coli. Mol. Biol. Evol. 22, 683–690. doi: 10.1093/molbev/msi056
Keywords: lac operon, fitness effect, Escherichia coli, Lactobacillus murinus, Bacteroides thetaiotaomicron, lactose, gut microbiota, microbe–microbe interactions
Citation: Pinto C, Melo-Miranda R, Gordo I and Sousa A (2021) The Selective Advantage of the lac Operon for Escherichia coli Is Conditional on Diet and Microbiota Composition. Front. Microbiol. 12:709259. doi: 10.3389/fmicb.2021.709259
Received: 13 May 2021; Accepted: 28 June 2021;
Published: 21 July 2021.
Edited by:
Maria Elena Martino, University of Padua, ItalyReviewed by:
Åsa Sjöling, Karolinska Institutet (KI), SwedenScott Miller, University of Montana, United States
Copyright © 2021 Pinto, Melo-Miranda, Gordo and Sousa. This is an open-access article distributed under the terms of the Creative Commons Attribution License (CC BY). The use, distribution or reproduction in other forums is permitted, provided the original author(s) and the copyright owner(s) are credited and that the original publication in this journal is cited, in accordance with accepted academic practice. No use, distribution or reproduction is permitted which does not comply with these terms.
*Correspondence: Ana Sousa, YW1zb3VzYUB1YS5wdA==
†These authors have contributed equally to this work