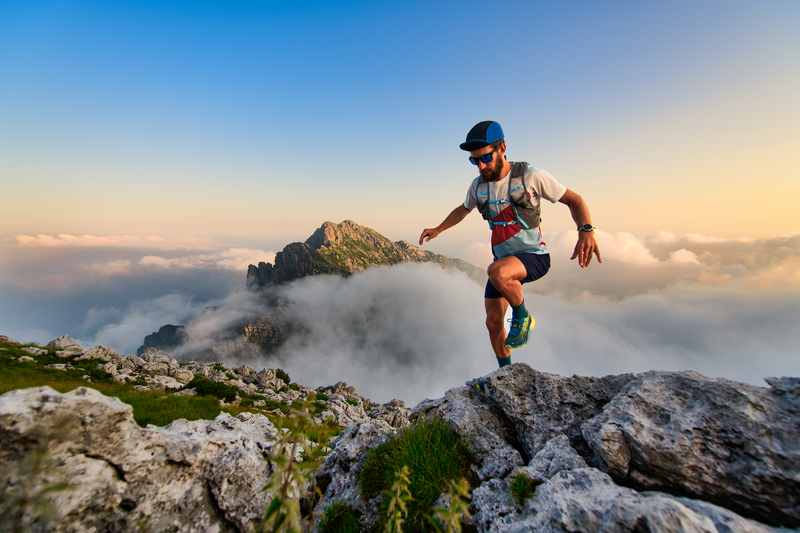
94% of researchers rate our articles as excellent or good
Learn more about the work of our research integrity team to safeguard the quality of each article we publish.
Find out more
ORIGINAL RESEARCH article
Front. Microbiol. , 13 September 2021
Sec. Antimicrobials, Resistance and Chemotherapy
Volume 12 - 2021 | https://doi.org/10.3389/fmicb.2021.700605
The bacterial pathogens Streptococcus agalactiae (GBS) and Staphylococcus aureus (S. aureus) cause serious infections in humans and animals. The emergence of antibiotic-resistant isolates and bacterial biofilm formation entails the urge of novel treatment strategies. Recently, there is a profound scientific interest in the capabilities of non-digestible oligosaccharides as antimicrobial and anti-biofilm agents as well as adjuvants in antibiotic combination therapies. In this study, we investigated the potential of alginate oligosaccharides (AOS) and chitosan oligosaccharides (COS) as alternative for, or in combination with antibiotic treatment. AOS (2–16%) significantly decreased GBS V growth by determining the minimum inhibitory concentration. Both AOS (8 and 16%) and COS (2–16%) were able to prevent biofilm formation by S. aureus wood 46. A checkerboard biofilm formation assay demonstrated a synergistic effect of COS and clindamycin on the S. aureus biofilm formation, while AOS (2 and 4%) were found to sensitize GBS V to trimethoprim. In conclusion, AOS and COS affect the growth of GBS V and S. aureus wood 46 and can function as anti-biofilm agents. The promising effects of AOS and COS in combination with different antibiotics may offer new opportunities to combat antimicrobial resistance.
Among various pathogenic agents, Staphylococcus aureus (S. aureus) and Group B Streptococcus (GBS), alternatively called as Streptococcus agalactiae (S. agalactiae), cause serious infections in both humans and animals at a global scale. These pathogens can cause a wide spectrum of invasive diseases ranging from neonatal sepsis, meningitis, and pneumonia to severe mastitis in cattle (Tong et al., 2015; Lin et al., 2017). Both pathogens produce multiple virulence factors and have the capability to form biofilms (Rosini and Margarit, 2015; Moormeier and Bayles, 2017). An increasing problem in treating these infections is the emergence of strains that are resistant to antimicrobial treatment (Costerton et al., 1999; Jamal et al., 2018). Infections related to the pathogenic form of GBS might occur in utero or with its passage through the birth canal during parturition. The percentage of neonates from GBS-colonized mothers that become transiently colonized with GBS by their mother’s organism is about 30–70% (Melin, 2011). Despite its role as a common intestinal colonizer in infants, how GBS retains its potential virulence and its transition from a commensal to a devastating pathogen remains poorly understood (Kolter and Henneke, 2017). On the other hand, S. aureus causes a wide range of diseases, such as toxic shock syndrome, infective endocarditis, as it is capable to disrupt tissue barriers, entering the bloodstream, and contaminating almost every organ in the body (Brandt et al., 2018). Additionally, S. aureus is the most common causative pathogen of infectious mastitis that might appear at every stage of life but occurs mostly in women during the breast-feeding period. The global incidence of mastitis within lactating women varies from 1 to 10% although some studies indicate that this infection can be observed to reach 33% of lactating women (Boakes et al., 2018). Through breastfeeding, S. aureus can be transferred to the gut microflora of newborns, where it colonizes the gastrointestinal tract (Lindberg et al., 2004).
The high prevalence of pediatric infectious diseases linked with the colonization, and the pathogenicity of GBS and S. aureus in the gastrointestinal tract of infants has increased the attention toward alternative approaches to prevent/reduce the incidence of these infections. Misuse and overuse of broad-spectrum antibiotics has resulted in a situation, wherein bacteria promote the evolution of phenotypes resistant to nearly every antibiotic in clinical use (Craft et al., 2018a). Therefore, there is an urgent need for alternatives that can tackle the problem of antimicrobial resistance. Antibiotic combination therapy, which involves the co-administration of antibiotics with an adjuvant that suppresses the resistance and enhances the antibiotic function and efficacy, offers promising therapeutic perspectives (Wright, 2016). One of the mechanisms that bacteria used to develop resistance to antibiotics is based on the alteration of their physiology through the formation of a biofilm matrix. It is estimated that 65% of all bacterial infections result in bacterial biofilm formation (Jamal et al., 2018), one of the interesting characteristics of many bacteria, including S. aureus and GBS (Rosini and Margarit, 2015; Moormeier and Bayles, 2017). Especially, biofilm formation on implanted materials and medical devices, such as catheters, endotracheal tubes, and prosthetic joints, poses a serious public health problem (Jamal et al., 2018).
The composition and the stability of biofilms is dependent on the structure of their extracellular polymeric substances (EPS), a matrix that is mainly composed of polysaccharides, proteins, lipids, and extracellular DNA (eDNA; Flemming et al., 2016). Different hypotheses have been examined to explain the antimicrobial persistence in the unbreakable structures of biofilms. First, it is believed that antibiotics can be inactivated by antibiotic-degrading enzymes, which are accumulated in the biofilm matrix. Second, the low metabolic activity of microorganisms observed in a biofilm is correlated with antibiotic tolerance (Reffuveille et al., 2017). Additionally, the intrinsic structure of biofilms may prevent the antibiotics to penetrate into the biofilm due to a high abundance of water channels (Bjarnsholt et al., 2013; Reffuveille et al., 2017). Given the complex mechanisms responsible for antibiotic resistance of bacteria in biofilms, a combination of various defensive ways may be needed to successfully combat these bacterial structures.
Non-digestible oligosaccharides (NDOs), complex carbohydrates that resist hydrolysis by salivary and intestinal digestive enzymes and known for their prebiotic properties by stimulating beneficial bacteria in the gut microbiota, These NDOs also exhibit various pathogen reduction capabilities, as reviewed in Asadpoor et al. (2020, 2021a), and thus may represent potential therapeutic candidates against infections. NDOs, such as human milk oligosaccharides (HMOs), chitosan oligosaccharides (COS), and alginate oligosaccharides (AOS), two NDOs that structurally resemble HMOs, can exhibit anti-biofilm activity (Ackerman et al., 2018; Powell et al., 2018; Asadpoor et al., 2021a), not only by preventing biofilm formation but also by decomposing preformed biofilms probably via the disruption of EPS components (Powell et al., 2018). Furthermore, specific NDOs, and especially HMOs, exert a bacteriostatic effect on bacterial growth (Craft et al., 2018b; Asadpoor et al., 2020). Based on different investigations, AOS and COS have already shown anti-virulence and anti-biofilm properties against different bacteria, such as Acinetobacter baumannii and Pseudomonas aeruginosa (Khan et al., 2012; Lu et al., 2014).
Given the antimicrobial capacity of AOS and COS, the current study investigated the potential of these two promising NDOs to inhibit bacterial growth and biofilm formation of S. aureus and GBS. In addition, the effect of the NDOs in combination with antibiotics was tested to evaluate possible synergistic effects that may diminish the bacterial resistance to antimicrobials.
S. aureus strain wood 46 (ATCC 10832; de Vor et al., 2021) and wild-type (WT) GBS clinical isolate NCTC 10/84 (1169-NT1; ATCC 49447; serotype V; Sheen et al., 2011) were gifts from Suzan Rooijakkers (UMC, University Medical Center, Utrecht, Netherlands) and Nina van Sorge (AMC, AcademicMedical Center, Amsterdam, Netherlands), respectively. Bacteria were stored at −80°C. For all experiments, bacteria were first grown on blood agar plates (Biotrading, Mijdrecht, Netherlands) at 37°C for 24h and subsequently, the colonies were sub-cultured in tryptic soy broth (TSB) and incubated overnight at 37°C under shaking conditions (160rpm). After incubation, bacterial growth (OD600) was measured and bacterial density was adjusted according to OD600=0.5 (determination of the minimum inhibitory concentration) or at OD600=1 (biofilm formation assay).
The NDOs, AOS (purity>85%) and COS (purity>90%), were purchased from BZ Oligo Biotech Co., Ltd. (Qingdao, Shandong, China). AOS was produced by the degradation of algin and COS originated from marine biological sources (shrimp and crab shells). Their structures are depicted in Figure 1A. NDO (AOS and COS) solutions were freshly dissolved in TSB before each experiment and the pH of the solution was adjusted to 7.2–7.4. The chemical NDO structures were drawn using ChemDraw Professional 15.0.
Figure 1. Structures of oligosaccharides and antibiotics used for antimicrobial activity against GBS V and S. aureus wood 46. (A) Structures of AOS and COS. (B) Structures of bacteriostatic (TMP, CLI, and TET) and bactericidal (AMP and CIP) antibiotics.
Ampicillin (AMP), Ciprofloxacin (CIP), Clindamycin (CLI), Tetracycline (TET), and Trimethoprim (TMP) were purchased from Sigma-Aldrich (Steinheim, Germany). These five common-used antibiotics were chosen based on a variety of chemical structures (Figure 1B) and mechanisms of action to assess whether in combination with AOS and COS can sensitize bacterial strains (S. aureus strain wood 46 and GBS V) to these antibiotics. Sterile stock concentrations of each antibiotic were made in TSB and used for serial dilutions and prior to each experiment fresh stocks were prepared. The chemical antibiotic structures were drawn using ChemDraw Professional 15.0.
The antibacterial capacity of AOS and COS against GBS V and S. aureus wood 46 was determined via analyzing the minimum inhibitory concentration following the method as described previously (Asadpoor et al., 2021b). AOS and COS were serially diluted in 96-well U-bottom polypropylene plates (Corning Costar, Cambridge, MA, United States) to reach 100μl final volume with concentrations ranging from 16 to 0.25%. Subsequently, 100μl of bacterial inoculums (S. aureus strain wood 46 and GBS V) with OD600=0.5 (approximately 10+8colony forming units [CFU]/ml) were added to serially diluted NDOs (Uriarte et al., 2017; Pinna et al., 2020). The plates were covered with sterile breathable film (VWR International, Amsterdam, Netherlands) and incubated overnight at 37°C under shaking conditions (160rpm). After the incubation period, 100μl of culture medium was transferred to 96-well F-bottom polystyrene microtiter plates (Corning Costar, Cambridge, MA, United States) and the signal was measured at 600nm with a FLUOstar Omega microplate reader (BMG Labtech GmbH, Ortenberg, Germany). Bacteria growth in TSB without treatment served as positive control and TSB alone was used as negative control. The minimum inhibitory concentration was considered as the lowest concentration that inhibits bacterial growth by more than 90% in comparison to positive control groups, as IC90 value correlated well with the minimum inhibitory concentration of a compound as described by Sun et al. (2016) and Zheng et al. (2018).
The ability of AOS to sensitize the GBS strain V to specific antibiotics was determined using the antibiotic sensitization assay as described before (Marks et al., 2013). AOS were serially diluted in 96-well U-bottom polypropylene plates with concentrations ranging from 16 to 2%. Subsequently, AOS was combined with different concentrations of AMP, CLI, TET, and TMP. The concentration range of the studied antibiotics was chosen based on the determination of the minimum inhibitory concentration. Concentrations below the minimum effective concentration were selected in order to create a wide range, in which the additional effect of AOS could be visible. Thereafter, 100μl bacterial inoculums with OD=0.5 was added to the treatments. Plates were covered with sterile breathable film (VWR International, Amsterdam, Netherlands) and incubated for 24h at 37°C under shaking conditions (160rpm). After the incubation period, the optical density was measured with a FLUOstar Omega microplate at 600nm. Bacteria growth in TSB without any treatment served as positive control, while wells with TSB (no treatment) were considered as negative control. For determining statistical significance, the outcome of the combinational treatment was compared to both the results of corresponding antibiotic concentration and corresponding AOS concentration. Therefore, the final effect was considered statistically significant when all two conditions were significant different compared to the combination therapy, suggesting sensitization was achieved.
The biofilm formation assay was performed for the evaluation of the effect of AOS and COS on the biofilms produced by S. aureus wood 46 and GBS V. As described above, for the biofilm formation assay, bacteria strains were grown in TSB at 37°C under shaking conditions (160rpm). Afterward, different procedures were carried out for the growth of streptococci and staphylococci biofilms.
For the development of streptococci biofilm, a biofilm formation assay was adapted from methods described previously (Ruppen et al., 2017). Briefly, NDO treatments of serially diluted concentrations (16–0.5%) were prepared in 96-well F-bottom polystyrene microtiter plates. For the preparation of the serial dilutions of AOS and COS, the biofilm medium (BM) composed of TSB supplemented with 0.5% glucose and 3% NaCl was used. Thereafter, the optical density of GBS V was adjusted at OD600=0.5 and 100μl of the inoculated medium was transferred into the 96-well plates in the presence of increasing NDO concentrations. The biofilms were grown for 24h at 37°C with 5% CO2 under static conditions. Furthermore, bacteria grown in BM in the absence of any intervention served as positive control, representing the maximum biofilm growth. Uninoculated culture media (BM: TSB, 1:1) was considered as negative control.
For the biofilm formation by staphylococci, a different procedure was followed as described by Kang et al. (2019). Briefly, the OD of grown bacteria was adjusted at OD600=1. To prepare the working bacterial solution (WBS), 10μl of bacterial solution was added to 10ml of BM (1:1000). NDO treatments were prepared in 96-well F-bottom plates of serial diluted concentrations (16–0.5%). Thereafter, 100μl WBS was added in 96-well F-bottom plates in absence or presence of NDOs and incubated for 24h at 37°C with 5% CO2 under static conditions. For quantifying the biofilm inhibitory effect of NDOs, full-formed biofilms without any additional treatment were used as positive control and uninoculated culture media (BM: TSB, 1:1) was used as negative control.
Subsequently, supernatants of both streptococci and staphylococci biofilms were gently removed, wells were washed with 200μl of phosphate-buffered saline (PBS), and the bacterial biofilms were fixed at 60°C for 30min. The fixed biofilms were stained with 160μl of crystal violet (CV) solution (0.1%) for 5min. Excess stain was discarded and wells were washed twice with tap water. Stained biofilms were solubilized in 160μl of acetic acid (33%), and 100μl was gently transferred to a 96-well F-bottom plate. The biofilm formation was measured at 595nm using a FLUOstar Omega microplate reader.
Minimum biofilm inhibitory concentration (MBIC) of NDOs against GBS V and S. aureus wood 46 was determined as the lowest concentration of NDOs that inhibit biofilm formation by more than 90% in comparison to control groups (Sun et al., 2016; Zheng et al., 2018).
The checkerboard biofilm formation assay was performed in order to identify the type of interaction (synergistic, additive, indifferent, or antagonistic) between AOS and COS with the five antibiotics against bacterial biofilm formation by S. aureus (Mataraci and Dosler, 2012). Concentration ranges of antibiotics are depicted in Table 1.
Table 1. Minimum biofilm inhibitory concentration (MBIC) of S. aureus wood 46 and ranges of antibiotic solutions performed in the checkerboard biofilm formation assay.
Serial dilutions of NDOs were prepared with concentrations ranging from 16 to 0.25% and were added to the different rows of the flatbottom 96 well plate. The antibiotic dilutions were added to the different columns of the 96 well plate. In this regard, a variety of mixtures of different concentrations of the assessed compounds (NDOs and antibiotics) was created with controls for NDOs (far-right column) and antibiotics (bottom row). The well at the bottom right corner was used as positive control, in which only inoculated medium was present in order to indicate the maximum biofilm growth. Subsequently, biofilms were prepared and biofilm formation was measured as described in section Antibiotic Sensitization Assay. Briefly, 100μl of the WBS was added to the combined treatments to reach 200μl final volume per well. The plate was incubated at 37°C with 5% CO2 for 24h at static conditions. Following the incubation period, the medium was gently aspirated and the wells were washed once with 200μl PBS to remove free-floating “planktonic” bacteria. After the washing procedure, the biofilm was fixed at 60°C for 30min. For measuring the biofilm biomass, the fixed biofilm was stained with 160μl of CV solution (0.1%) for 5min, and the excess stain was eliminated by two washes with tap water. For solubilizing the bound CV, 160μl of acetic acid (33%) was added to the wells, and the optical absorbance was determined at 595nm using a FLUOstar Omega microplate reader.
In this study, the nature of the interaction of the combinational agents was evaluated by determination of the anti-biofilm capacity of the agents and using the fractional biofilm inhibitory concentration (FBIC). For calculating FBIC index, the observing equation was used: ΣFBIC=FBIC A+FBIC B=(MBIC of drug A in the combination/MBIC of drug A alone)+(MBIC of drug B in the combination/MBIC of drug B alone; Abdi Ali et al., 2015; Feldman et al., 2020). The lowest ΣFBIC index was chosen for the strongest interaction between two agents. The effect of two agents is considered as synergy when the ΣFBIC index is 0.5 or less, additive when the ΣFBIC index is between 0.5 and 1, indifferent when the ΣFBIC index is between 1 and 4, and antagonistic when the ΣFBIC index is 4 or more (Ramezani Ali Akbari and Abdi Ali, 2017; Feldman et al., 2020). Synergy means the interaction or cooperation of two or more organizations, substances, or other agents to produce a combined effect greater than the sum of their separate effects. Additive means the overall consequence, which is the result of two agents acting together and which is the simple sum of the effects of the agents acting independently. Indifferent means the combination has no increase in inhibitory activity of both agents. Antagonistic means the effect produced by the contrasting actions of two (or more) agents (Pillai et al., 2005).
To assess the effectivity of the most optimal combination (COS and CLI) on bacterial growth inhibition, the supernatant of the combination of treatments that acquired the best inhibitory effect and the lowest ΣFBIC was collected and grown on blood agar plates. One loopful (approximately 10μl) of the corresponding well was transferred into a separate blood agar plate. The transferred amount was evenly spread over the surface and the agar plate was incubated at 37°C for 24h. The bacterial growth of the combinational treatment was optically compared with the growth of bacteria with each agent separately as well as with the positive control (without treatment).
Data were reported as mean values ± SEM of at least three independent experiments (n=3) routinely performed in triplicate (three wells/condition). Results were analyzed using Prism 8.0 GraphPad Software (GraphPad, San Diego, CA, United States). Statistical significance was determined using ANOVA followed by Bonferroni post-hoc test. Differences were considered as statistically significant when p<0.05.
Το evaluate whether AOS and COS can inhibit the growth of the two pathogenic strains, GBS V and S. aureus wood 46, bacterial growth in TSB in the absence and presence of increasing concentrations of AOS and COS was investigated. As shown in Figure 2, the addition of AOS caused a significant concentration-dependent reduction in the growth of GBS V. At AOS concentrations below 2% (0.25, 0.5, and 1%) no significant inhibition of GBS V growth was observed (Figure 2A). Although higher AOS concentrations decreased the growth of GBS V, the minimum inhibitory concentration was not identified, since the growth inhibition did not reach 90% or higher. Maximum inhibition of growth (81%) was achieved at concentration of 8% AOS. Unlike AOS, COS treatment (0.25–8%) did not inhibit, but even enhanced GBS V growth by up to 2-fold. Using the highest COS concentration (16%), a slight but not significant reduction in GBS V growth was measured (Figure 2B). Similar measurements of the effects of AOS and COS on the growth of S. aureus demonstrated unaltered growth of S. aureus in the presence of AOS (Figure 2C), whereas COS (0.25–8%) again caused an increase in bacterial (S. aureus) growth (Figure 2D).
Figure 2. Effect of AOS and COS on bacterial growth of GBS V and S. aureus wood 46 strains in TSB. In order to identify the MIC of AOS and COS against GBS V (A,B) and S. aureus wood 46 (C,D), seven 2-fold serial dilutions of each NDO were examined, as described in the Material and Methods section. Control represents the percentage of the maximum growth of bacteria without any intervention. Results are expressed as the percentage of bacterial growth (relative to control) as mean±SEM of three independent experiments each performed in triplicate. Statistical differences *(p<0.05) and **(p<0.01) compared to positive control were obtained using one-way ANOVA test. AOS, alginate oligosaccharides and COS, chitosan oligosaccharides.
In order to investigate whether AOS is able to sensitize GBS V to antibiotics (AMP, CLI, TET, and TMP), an antibiotic sensitization assay was conducted. Hereto, GBS V growth in the absence and presence of increasing concentrations of AOS and antibiotics was followed over time. A downward trend in GBS V growth is observed by all the AOS-antibiotic combinations tested, however, AOS-AMP and AOS-TET did not provoke any GBS sensitization to the antimicrobial agents, since any of combinational concentrations did not significantly decrease the bacterial growth comparing with corresponding concentration of AOS as well as with the antibiotic (Figures 3A,C). Interestingly, a striking reduction in the minimum inhibitory concentration of CLI was visible when AOS was combined. When 4% AOS was added to 0.0313μg/ml CLI, the same reduction of GBS growth was observed as obtained with three times higher CLI concentration (0.125μg/ml) without AOS supplementation (Figure 3B). Furthermore, AOS sensitized GBS V to the highest resistance concentrations of TMP (8–64μg/ml; Figure 3D). More specifically, 2 and 4% AOS induced a significant reduction of TMP at concentrations more than 8μg/ml, and from 8μg/ml to 32μg/ml, respectively (Supplementary Figure 1).
Figure 3. Effect of AOS in combination with AMP, CLI, TET and TMP on the growth of GBS V strain. To examine whether AOS has the feasibility to sensitize GBS V to antibiotics, a sensitization assay was performed as described in the material and method section. AOS (2, 4 and 8%) were combined with different concentrations of AMP (A), CLI (B), TET (C) and TMP (D). Sensitization was only achieved when 2 and 4% AOS were combined with TMP. Star (for 2% AOS) or dollar (for 4% AOS) are representing a significant reduction of the combinational treatments comparing with both corresponding antimicrobial agents (AOS and antibiotics). Positive control represents the percentage of the absolute growth of bacteria (100% growth) without the presence of any treatment. The results are expressed as the percentage of bacterial growth as mean±SEM of three independent experiments each performed in a minimum of three replicates. Statistical differences *, $ (p<0.05) compared to control and the corresponding concentrations of antibiotics and AOS, were obtained using a two-way ANOVA test.
To assess the behavior of AOS and COS during biofilm formation, the biofilm-forming capacity of GBS V and S. aureus was conducted in presence and absence of AOS or COS by staining with CV. As shown in Figure 4A, the highest concentration of AOS (16%) induced a significant reduction (71%) of biofilm formation by GBS V compared to the positive control (without treatment). This cannot be considered as MBIC since this inhibition did not reach 90% or higher. COS treatment did not affect the GBS V biofilm formation (Figure 4B). In contrast, both AOS and COS showed an inhibitory effect on S. aureus biofilm formation. AOS showed an inhibitory effect in a concentration-dependent manner, although only at 8% AOS and 16% AOS the effects were statistically significant, as depicted in Figure 4C. With the highest AOS concentration (16%) even a 90% inhibition of biofilm formation was achieved (MBIC). COS (2–16%) treatment significantly reduced the biofilm formation of S. aureus wood 46. Precisely, COS 16% and COS 8% (MBIC) exhibited 97 and 90% inhibition of S. aureus biofilm formation, respectively. At concentrations below 2% COS no effect was observed (Figure 4D).
Figure 4. Anti-biofilm activity of AOS and COS against GBS V and S. aureus wood 46 strains. For the biofilm formation assay, six 2-fold serial dilutions of AOS and COS were tested after 24h of exposure, targeting the MBIC against GBS V (A,B) and S. aureus wood 46 (C,D), as described in the Material and Methods section. Control (−) represents the negative control (uninoculated culture media without NDO treatment) and control (+) represents full-formed biofilms without any additional treatment 16% AOS and 8% COS are considered as MBIC against S. aureus wood 46. Results are expressed as the percentage of biofilm formation (relative to positive control) as mean±SEM of three independent experiments each performed in triplicate. Statistical differences *(p<0.05) and **(p<0.01) compared to positive control were obtained using one-way ANOVA test. AOS, alginate oligosaccharides and COS, chitosan oligosaccharides.
To test the possible additive effects of NDOs on the action of conventional antibiotics, a biofilm formation checkerboard assay was performed (Table 2). The different interactions were measured using the FBIC index as described in the material and methods section. Among different combinations tested, COS with CLI obtained a synergistic anti-biofilm effect. As shown in Figure 5A, a simulation of the checkerboard biofilm assay is depicted, demonstrating the synergistic effect of 2% COS in combination with 0.0625μg/ml CLI (FBIC value, 0.5) on S. aureus biofilm formation. This specific combination resulted in the lowest FBIC index among the different combinations that exhibited full inhibition of S. aureus biofilm formation. Along with the FBIC value related to synergy, the FBIC values of the wells, in which an additive effect was reported, were also calculated and mentioned in Figure 5A. Concerning the other antibiotics, an additive effect was reported with the treatments of AOS and COS in conjunction with TET, and the FBIC values were 0.54 and 0.75, respectively. Additive interaction was also observed when AOS was combined with CLI (FBIC value, 0.73). The nature of interaction of both AOS and COS with CIP (FBIC value, 1.01 and 1.03, respectively) or TMP (FBIC value, 1.01 for both) was characterized as indifferent. Finally, antagonism interaction was reported when AMP was combined with COS (FBIC value>4) and indifference when combined with AOS (FBIC value, 1.42).
Table 2. MBIC values, FBIC index and the nature of interaction between AOS and COS with AMP, CIP, CLI, TET, and TMP against S. aureus wood 46.
Figure 5. A simulated checkerboard assay for the combination of COS with CLI against biofilm formation of S. aureus and the subsequent growth of the synergistic combination on blood agar plates. As depicted in (A; Pillai et al., 2005), both COS and CLI were 2-fold serial diluted starting from 16% COS (2×MBIC) and 2μg/ml CLI (8×MBIC). Wells without color refer to full inhibition of S. aureus biofilm formation (≥ 90% biofilm inhibition) while in purple wells the S. aureus biofilm formation was not fully inhibited. The FBIC values of the wells that showed synergistic or additive effect were calculated as described in the material and methods section using the following equation: ΣFBIC=FBIC A+FBIC B=(MBIC of drug A in the combination/MBIC of drug A alone)+(MBIC of drug B in the combination/MBIC of drug B alone) and depicted in the figure for each well separately. Partial synergy was identified when 2% COS and 0.0625μg/ml CLI were combined with a corresponding FBIC value of 0.5. This specific FBIC value is considered as the lowest FBIC index among the combination wells that had full biofilm formation inhibition. The far-right column represents the control of COS treatment (without CLI treatment) while the bottom row, in which COS is absent, represents the control of CLI treatment. Finally, the well on the bottom right corner, in which both COS and CLI are absent, is used as positive control for biofilm production. The combination of the antimicrobial agents that provoke the synergistic effect, 2% COS with 0.0625μg/ml CLI were grown on blood agar FIGURE 2plates for 24h in order to determine whether the observed effect was attributed to their abilities to reduce the number of bacteria (E). To identify the outcome of their interaction on bacterial growth, each agent was also grown separately, positive control which represents the absolute growth of bacteria without the presence of any treatment (B), CLI 0.0625μg/ml (C) and COS 2% (D). Finally, on a separate plate, the supernatant of the well without any intervention was grown, representing the positive control.
Furthermore, the supernatant of the combinational treatment that achieved the most optimal effect (COS with CLI, FBIC value, 0.5) was collected and cultured on blood agar plates in order to identify whether the two agents could also reduce the bacterial growth in addition to the full inhibition of S. aureus biofilm formation. As depicted in Figure 5E, the S. aureus colonies treated with the COS-CLI combination were almost eliminated in comparison with the number of S. aureus bacteria in positive control group (Figure 5B), treated with 0.0625μg/ml CLI (Figure 5C) or 2% COS (Figure 5D).
In the present study, the anti-growth and anti-biofilm activities of AOS and COS against two pathogenic bacterial strains, GBS V and S. aureus wood 46, were evaluated. Furthermore, their combination with different antibiotics was tested to determine whether NDOs could enhance the function of antibiotics.
The antibacterial data revealed that AOS induce a strong inhibitory effect on the growth of GBS V, even at a low concentration (AOS 1%). One possible interpretation of such an effect is the anionic nature of AOS. Craft et al. (2019) showed that sialylated HMOs, which are negatively charged at homeostatic pH due to the sialic acid residues, exert antimicrobial activity against GBS III and Ia strains. In addition, the same group presented in another study that neutral fucosylated HMOs (2’-FL) did not have any substantial activity (Craft and Townsend, 2019). Despite the fact that the negative charge is assumed to play a substantial role in the antimicrobial abilities of AOS, further investigations are needed to confirm this mechanism of action. AOS significantly reduced the growth of S. aureus wood 46, and therefore may act in a strain-dependent manner. Interestingly, a depolymerized product of alginate (a mannuronic acid derivative) demonstrated an inhibition and high inhibitory activity against S. aureus (Hu et al., 2005). The differences in the anti-pathogenic effects of AOS might be related to the match or mismatch between the structural features of AOS (negatively charged) and the strain-specific bacterial target structures.
While COS increased the growth of both bacterial strains, this increase was only significant in the case of S. aureus wood 46. Concerning the observed bacterial growth, it can be hypothesized that instead of exerting antimicrobial effects, COS was utilized as a beneficial source for the growth and survival of GBS and S. aureus. These results concur with an earlier study (Yildirim-Aksoy et al., 2019), in which chitosan exhibit the capacity to stimulate growth. This could be related to the positively charged amino groups of chitosan, which bind to surface components and cell debris instead of cell surfaces of the related pathogen (Yildirim-Aksoy et al., 2019). Moreover, it is possible that GBS could reproduce and utilize degraded chitosan as the sole carbon source to benefit their growth (Yildirim-Aksoy et al., 2019). These observations were in contrast with previous reports indicating that the antimicrobial activities of COS might relate to the interaction between the positively-charged COS and the negatively-charged membrane residues (e.g., carbohydrate, proteins, and lipids; Jeon et al., 2001; Zheng and Zhu, 2003; Moon et al., 2007; Lin et al., 2009; Benhabiles et al., 2012). This effect can lead to cytoplasmic leakage and subsequently to cell death (Liu et al., 2004). For example, Benhabiles et al. (2012), confirmed the inhibitory effects of N-acetyl COS (NAc-COS) and COS on the growth of S. aureus ATCC 25923 and S. aureus ATCC 43300. COS with a higher molecular weight (MW; MW≥10kDa) are more effective in inhibition of different microorganisms, such as S. aureus, compared to fractions with lower MW (Jeon et al., 2001). These discrepancies might be attributable to methodological and experimental differences such as bacterial strains and structural characteristics of COS. In this regard, it has previously been shown that the antibacterial effect of COS is greatly dependent on their degree of polymerization or their MW (Jeon and Kim, 2000).
The potentiation of antibiotic activity in the therapy of multidrug-resistant organisms is a major goal of an anti-infective cure. In the present study, we investigated the ability of AOS to potentiate the activity of conventional antibiotics against the GBS V strain. AOS was selected since it exhibited the capacity to inhibit GBS V. Results obtained from the antibiotic sensitization assay indicated that AOS (2 and 4%) sensitizes GBS V to TMP by significantly decreasing the effective TMP concentration to observe a similar effect as obtained with more than eight times higher TMP concentration. Although sensitization of GBS V occurred only in the case of TMP, 4% AOS decreased the lowest effective concentration of CLI up to 4-fold (from 0.125 to 0.0313μg/ml CLI). Overall, a downward trend is observed in the growth of GBS V from all the combinations tested, however, this trend is mostly attributed to the effectivity of AOS starting from 8% AOS with the most potent anti-growth ability observed with 4 and 2% AOS. Therefore, these insights into the effects of AOS might provide new opportunities to develop treatments of GBS-associated infections for which effective treatment is currently extremely limited.
Biofilms are one of the most challenging resistant mechanisms of bacteria that secrete various enzymes and virulence factors (Graf et al., 2019). Different mechanisms related to the inhibition of biofilm formation are modification of cell-surface charge, inhibition of bacterial growth, and prevention of microbial adhesion (Roy et al., 2018).
In our study, AOS significantly reduced the biofilm formation of both GBS V (4, 8, and 16% AOS) and S. aureus wood 46 (8 and 16% AOS). So far, anti-biofilm activities of AOS were only identified against Gram-negative bacteria such as P. aeruginosa (Powell et al., 2013, 2018; He et al., 2014). To the best of our knowledge, this is the first study indicating the anti-biofilm effects of AOS against gram-positive bacteria. We hypothesized that the negative charges of AOS interact with positively charged components of the biofilm matrix. Indeed, concerning staphylococci biofilms, one of the most important positively charged polymers is called as polysaccharide intercellular adhesin (PIA). PIA is involved in at least the majority of the staphylococcal biofilm-associated infections and constitutes the main molecule responsible for intercellular adhesion. The cationic PIA polymer (at neutral or basic pH) interacts with the negatively charged bacterial cell surface (e.g., with negatively charged teichoic acids) through multivalent electrostatic interactions (Arciola et al., 2015). Since PIA plays such a significant role in staphylococci biofilm formation, its inhibition by AOS could lead to obstructing the adhesive role of PIA and consequently inhibiting biofilm formation. There is limited information available related to the formation of streptococci biofilms such as the composition of the GBS biofilm matrix. However, recent studies showed that this type of biofilm is mainly composed of proteins and eDNA, while polysaccharides represent a minor proportion (D’Urzo et al., 2014; Alvim et al., 2019). The anti-biofilm effect of AOS against GBS V can be attributed to the disruption of the intramolecular interactions in EPS that might occur due to the negative charge of AOS. These assumptions are in line with the results found by Taylor Nordgård and Draget (2011), who reported that alginates can disrupt intramolecular interaction in EPS (e.g., mucus), and competitively inhibit the interpolymer cross-links, weakening the biofilm structures. Anti-biofilm potential of AOS on the biofilms of GBS has not been identified so far. Therefore, further research is needed to identify the underlying mechanisms.
In contrast with AOS, the effect of COS on biofilm formation differs among the two bacterial species. COS treatment was ineffective against biofilm formation of GBS V, which is in line with the antibacterial data of COS in this study. On the other hand, there was a strong anti-biofilm inhibitory effect of different COS concentration (2, 4, 8, and 16% COS) against S. aureus wood 46. Interestingly, the strong effect of COS against the biofilm formation of S. aureus wood 46 is in contrast with the observed (non-existing) antibacterial effect since a significant increase in bacterial growth was observed. This contradiction might be attributed to the interference of COS with the compartments of the biofilm matrix that leads to the inhibition of biofilm formation, instead of adherence to the bacterial surface that has been previously proved to cause growth reduction (Felipe et al., 2019). Our results supported the finding that chitosan displays an anti-biofilm activity against S. aureus strains of bovine origin (Asli et al., 2017). Additionally, a significant inhibitory effect of LMW chitosan on S. aureus V329 biofilm formation was demonstrated (Felipe et al., 2019). Anti-biofilm properties of COS most likely rely on the polycationic nature due to its protonated amino groups, which interact electrostatically with the negatively charged biofilm components (e.g., proteins and eDNA; Khan et al., 2020). Hence, this electrostatic interaction may inhibit the formation of biofilm (Jiang et al., 2014). The observed inhibitory effect of COS on biofilm formation of S. aureus wood 46 in the current study can be attributed to the prevention of biofilm formation rather than on the destruction of preformed biofilms, since no changes were observed when COS was added for 24h after formation of established biofilms (Supplementary Figure 2A). Therefore, it can be hypothesized that COS interferes biofilm formation and development in early stages.
In summary, based on the anti-biofilm effects displayed by AOS and COS against S. aureus wood 46, a proposed mechanism of action is schematized in Figure 6. In comparison with the untreated bacteria that are able to form a mature biofilm, biofilm formation with treatment of AOS and COS is inhibited. This inhibitory anti-biofilm formation might be attributed to the charge of those two NDOs. AOS might electrostatically interact with the positively charged PIA while COS might interact with the negatively charged proteins and the eDNA of the extracellular matrix.
Figure 6. Representation of the proposed mechanism underlying the inhibitory effect of AOS and COS on biofilm formation of S. aureus. Concerning the untreated biofilm, a mature biofilm is formed by S. aureus in the absence of any treatment mainly composed of PIA, eDNA, and proteins (A). On the other hand, administration of AOS and COS may inhibit the formation of biofilm through the electrostatic interaction of oligosaccharides with the charged components of the extracellular matrix (B). This figure was created with BioRender.com.
In support of this assumption: neutral fructo-oligosaccharides did not exert any anti-biofilm activity against S. aureus wood 46 (Supplementary Figure 2B).
The development of bacterial resistance is one of the major concerns nowadays that affects the efficacy of several antibiotics for the treatment of severe infections. Combination therapy is considered as an effective approach to increase the potency of existing antibiotics and thereby combat antimicrobial resistance.
First, a checkerboard biofilm formation assay was performed testing the nature of the interactions of AOS and COS with five common-used antibiotics against S. aureus wood 46. The combination of COS and AOS with several antibiotics was investigated before, however, these studies was mainly focused on the reduction of bacterial growth rather than on the inhibition of biofilm formation (Tin et al., 2009; Khan et al., 2012; Asli et al., 2017; Kim et al., 2017). In the present study, the combination of COS with CLI and TET (targeting ribosomal protein 50S and 30S subunits, respectively) showed synergistic and additive activity against S. aureus wood 46 biofilm formation, respectively. Additive interaction also occurred when AOS was combined with both antibiotics. COS can inhibit the biofilm formation through ionic interactions (Khan et al., 2020), therefore it can enhance the accumulation of CLI into the biofilm and inhibition of protein synthesis, showing a synergistic effect. Since CLI inhibits bacterial protein synthesis by binding to the 50S subunit of the bacterial rRNA inside the cell, it is expected that it can also reduce exoprotein production in S. aureus biofilms. Hu et al. (2019) studied the effects of sub-inhibitory CLI on the production of S. aureus exoproteins and demonstrated that subinhibitory concentrations of CLI considerably decrease the S. aureus biofilm exoprotein. The additive effect observed by the interaction of AOS and COS with TET might be attributed to a similar mechanism of action, as TET also inhibits protein synthesis, although binding to the 30S subunit.
Interestingly, two bactericidal antibiotics (AMP and CIP) used in this study showed indifferent or antagonistic effects in combination with AOS and COS. Yang et al. (2015) demonstrated that two bactericidal antibiotics (vancomycin and CIP) were tested on Staphylococcus epidermidis biofilms and found that the combinational treatment with these two antibiotics can reduce the efficacy of these individual treatments. These observations might be related to the formation of persister cells induced by stress from antibiotics (Dörr et al., 2010). Moreover, the antagonistic effect deriving from the combination of COS with AMP can be partly explained by the fact that both agents target extracellular cell compartments. So, the positively charged group of COS can bind to the negatively charged compartments of the cell membrane, leading to the death of bacteria (Liu et al., 2004), while AMP inhibits cell wall synthesis (Epand et al., 2016), leading to competition among the two agents.
In the present study, we have shown that AOS and COS modulate both bacterial growth and biofilm production of GBS V and S. aureus wood 46, respectively. In addition to the observed anti-growth and anti-biofilm properties of both NDOs, the anti-biofilm and anti-growth potential in combination with different antibiotics was evaluated. The synergistic effect of COS with CLI against S. aureus and the ability of AOS to sensitize GBS V to TMP were the most promising results offering new perspectives to help combat antimicrobial resistance. Given the increasing need for antimicrobial alternatives and the capability of NDOs to serve as antibacterial agents, future efforts should focus on assessing the antimicrobial effects of AOS and COS against additional species of both Gram-positive and Gram-negative pathogens and evaluating combination therapy with different antibiotics. Moreover, investigating the mechanisms underlying the antimicrobial, and anti-biofilm capacity of NDOs, as well as the interaction with antibiotics is necessary to further establish the therapeutic potential of NDOs.
The original contributions presented in the study are included in the article/Supplementary Material, further inquiries can be directed to the corresponding author.
MA, GF, RP, JP, and SB: conceptualization. MA and G-NI: methodology, software, investigation, data curation, writing – original draft preparation, and visualization. MA, G-NI, and SB: validation. MA: formal analysis. JP and GF: resources. JP, SB, GF, and RP: writing – review and editing. GF and SB: supervision. GF, RP, JP, and SB: project administration. GF, SB, and RP: funding acquisition. All authors have read and agreed to the published version of the manuscript. All authors contributed to the article and approved the submitted version.
The authors declare that the research was conducted in the absence of any commercial or financial relationships that could be construed as a potential conflict of interest.
All claims expressed in this article are solely those of the authors and do not necessarily represent those of their affiliated organizations, or those of the publisher, the editors and the reviewers. Any product that may be evaluated in this article, or claim that may be made by its manufacturer, is not guaranteed or endorsed by the publisher.
We would like to acknowledge Suzan Rooijakkers and Nina Van Sorge from Department of Medical Microbiology, Utrecht Medical Center, Netherlands, for their generous gift of pathogens and Lisanne de Vor from the same department for her technical assistance with the biofilm formation assay. We appreciated the help that we received from Soheil Varasteh in designing the experiments. We would also like to thank Ebrahim Rezazadeh Zarandi from Immunology of Infectious Diseases Research Center, Research Institute of Basic Medical Sciences, Rafsanjan University of Medical Sciences, Rafsanjan, Iran, for his assistance during the data analysis of the checkerboard biofilm formation assay.
The Supplementary Material for this article can be found online at: https://www.frontiersin.org/articles/10.3389/fmicb.2021.700605/full#supplementary-material
Abdi Ali, A., Shafiei, M., Shahcheraghi, F., Saboora, A., and Ghazanfari, T. (2015). The study of synergistic effects of n.butanolic cyclamen coum extract and ciprofloxacin on inhibition of Pseudomonas aeruginosa biofilm formation. Biol. J. Microorganisms 3, 25–32.
Ackerman, D. L., Craft, K. M., Doster, R. S., Weitkamp, J. H., Aronoff, D. M., Gaddy, J. A., et al. (2018). Antimicrobial and antibiofilm activity of human mMilk oligosaccharides against Streptococcus agalactiae, Staphylococcus aureus, and Acinetobacter baumannii. ACS Infect. Dis. 4, 315–324. doi: 10.1021/acsinfecdis.7b00183
Alvim, D. C. S. S., Ferreira, A. F. M., Leal, M. A., Oliveira, L. M. A., Botelho, A. M. N., Botelho, A. C. N., et al. (2019). Biofilm production and distribution of pilus variants among Streptococcus agalactiae isolated from human and animal sources. Biofouling 35, 938–944. doi: 10.1080/08927014.2019.1678592
Arciola, C. R., Campoccia, D., Ravaioli, S., and Montanaro, L. (2015). Polysaccharide intercellular adhesin in biofilm: structural and regulatory aspects. Front. Cell. Infect. Microbiol. 5:7. doi: 10.3389/fcimb.2015.00007
Asadpoor, M., Ithakisiou, G. N., Henricks, P. A. J., Pieters, R., Folkerts, G., and Braber, S., (2021a). Non-digestible oligosaccharides and short chain fatty acids and their toxins. Toxins 13:175. doi: 10.3390/toxins13030175
Asadpoor, M., Peeters, C., Henricks, P. A. J., Varasteh, S., Pieters, R. J., Folkerts, G., et al. (2020). Anti-pathogenic functions of non-digestible oligosaccharides in vitro. Nutrients 12:1789. doi: 10.3390/nu12061789
Asadpoor, M., Varasteh, S., Pieters, R., Folkerts, G., and Braber, S. (2021b). Differential effects of oligosaccharides on the effectiveness of ampicillin against Escherichia coli in vitro. PharmaNutrition 16:100264. doi: 10.1016/j.phanu.2021.100264
Asli, A., Brouillette, E., Ster, C., Ghinet, M. G., Brzezinski, R., Lacasse, P., et al. (2017). Antibiofilm and antibacterial effects of specific chitosan molecules on Staphylococcus aureus isolates associated with bovine mastitis. PLoS One 12:e0176988. doi: 10.1371/journal.pone.0176988
Benhabiles, M. S., Salah, R., Lounici, H., Drouiche, N., Goosen, M. F. A., and Mameri, N. (2012). Antibacterial activity of chitin, chitosan and its oligomers prepared from shrimp shell waste. Food Hydrocoll. 29, 48–56. doi: 10.1016/j.foodhyd.2012.02.013
Bjarnsholt, T., Ciofu, O., Molin, S., Givskov, M., and Høiby, N. (2013). Applying insights from biofilm biology to drug development-can a new approach be developed? Nat. Rev. Drug Discov. 12, 791–808. doi: 10.1038/nrd4000
Boakes, E., Woods, A., Johnson, N., and Kadoglou, N. (2018). Breast infection: a review of diagnosis and management practices. Eur. J. Breast Health 14, 136–143. doi: 10.5152/ejbh.2018.3871
Brandt, S. L., Putnam, N. E., Cassat, J. E., and Serezani, C. H. (2018). Innate immunity to Staphylococcus aureus: evolving paradigms in soft tissue and invasive infections. J. Immunol. 200, 3871–3880. doi: 10.4049/jimmunol.1701574
Costerton, J. W., Stewart, P. S., and Greenberg, E. P. (1999). Bacterial biofilms: a common cause of persistent infections. Science 284, 1318–1322. doi: 10.1126/science.284.5418.1318
Craft, K. M., Gaddy, J. A., and Townsend, S. D. (2018a). Human milk oligosaccharides (HMOs) sensitize group B Streptococcus to clindamycin, erythromycin, gentamicin, and minocycline on a strain specific basis. ACS Chem. Biol. 13, 2020–2026. doi: 10.1021/acschembio.8b00661
Craft, K. M., Thomas, H. C., and Townsend, S. D. (2018b). Interrogation of human milk oligosaccharide fucosylation patterns for antimicrobial and antibiofilm trends in group B Streptococcus. ACS Infect. Dis. 4, 1755–1765. doi: 10.1021/acsinfecdis.8b00234
Craft, K. M., Thomas, H. C., and Townsend, S. D. (2019). Sialylated variants of lacto-: N-tetraose exhibit antimicrobial activity against group B Streptococcus. Org. Biomol. Chem. 17, 1893–1900. doi: 10.1039/C8OB02080A
Craft, K. M., and Townsend, S. D. (2019). 1-Amino-2'-fucosyllactose inhibits biofilm formation by Streptococcus agalactiae. J. Antibiot. 72, 507–512. doi: 10.1038/s41429-019-0151-6
de Vor, L., van Dijk, B., van Kessel, K. P. M., Kavanaugh, J. S., de Haas, C. J. C., Aerts, P. C., et al. (2021). Human monoclonal antibodies against Staphylococcus aureus surface antigens recognize in vitro biofilm and in vivo implant infections. bioRxiv [Preprint]. doi: 10.1101/2021.02.09.429966
Dörr, T., Vulić, M., and Lewis, K. (2010). Ciprofloxacin causes persister formation by inducing the TisB toxin in Escherichia coli. PLoS Biol. 8:e1000317. doi: 10.1371/journal.pbio.1000317
D’Urzo, N., Martinelli, M., Pezzicoli, A., De Cesare, V., Pinto, V., Margarit, I., et al. (2014). Acidic pH strongly enhances in vitro biofilm formation by a subset of hypervirulent ST-17 Streptococcus agalactiae strains. Appl. Environ. Microbiol. 80, 2176–2185. doi: 10.1128/AEM.03627-13
Epand, R. M., Walker, C., Epand, R. F., and Magarvey, N. A. (2016). Molecular mechanisms of membrane targeting antibiotics. BBA-Biomembranes 1858, 980–987. doi: 10.1016/j.bbamem.2015.10.018
Feldman, M., Smoum, R., Mechoulam, R., and Steinberg, D. (2020). Potential combinations of endocannabinoid/endocannabinoid-like compounds and antibiotics against methicillin-resistant Staphylococcus aureus. PLoS One 15:e0231583. doi: 10.1371/journal.pone.0231583
Felipe, V., Breser, M. L., Bohl, L. P., Rodrigues da Silva, E., Morgante, C. A., Correa, S. G., et al. (2019). Chitosan disrupts biofilm formation and promotes biofilm eradication in Staphylococcus species isolated from bovine mastitis. Int. J. Biol. Macromol. 126, 60–67. doi: 10.1016/j.ijbiomac.2018.12.159
Flemming, H. C., Wingender, J., Szewzyk, U., Steinberg, P., Rice, S. A., and Kjelleberg, S. (2016). Biofilms: an emergent form of bacterial life. Nat. Rev. Microbiol. 14, 563–575. doi: 10.1038/nrmicro.2016.94
Graf, A. C., Leonard, A., Schäuble, M., Rieckmann, L. M., Hoyer, J., Maass, S., et al. (2019). Virulence factors produced by Staphylococcus aureus biofilms have a moonlighting function contributing to biofilm integrity. Mol. Cell. Proteomics 18, 1036–1053. doi: 10.1074/mcp.RA118.001120
He, X., Hwang, H.-M., Aker, W. G., Wang, P., Lin, Y., Jiang, X., et al. (2014). Synergistic combination of marine oligosaccharides and azithromycin against Pseudomonas aeruginosa. Microbiol. Res. 169, 759–767. doi: 10.1016/j.micres.2014.01.001
Hu, X., Jiang, X., Gong, J., Hwang, H., Liu, Y., and Guan, H. (2005). Antibacterial activity of lyase-depolymerized products of alginate. J. Appl. Phycol. 17, 57–60. doi: 10.1007/s10811-005-5524-5
Hu, H., Ramezanpour, M., Hayes, A. J., Liu, S., Psaltis, A. J., Wormald, P.-J., et al. (2019). Sub-inhibitory clindamycin and azithromycin reduce S. aureus exoprotein induced toxicity, inflammation, barrier disruption and invasion. J. Clin. Med. 8:1617. doi: 10.3390/jcm8101617
Jamal, M., Ahmad, W., Andleeb, S., Jalil, F., Imran, M., Nawaz, M. A., et al. (2018). Bacterial biofilm and associated infections. J. Chin. Med. Assoc. 81, 7–11. doi: 10.1016/j.jcma.2017.07.012
Jeon, Y. J., and Kim, S. K. (2000). Production of chitooligosaccharides using an ultrafiltration membrane reactor and their antibacterial activity. Carbohydr. Polym. 41, 133–141. doi: 10.1016/S0144-8617(99)00084-3
Jeon, Y. J., Park, P. J., and Kim, S. K. (2001). Antimicrobial effect of chitooligosaccharides produced by bioreactor. Carbohydr. Polym. 44, 71–76. doi: 10.1016/S0144-8617(00)00200-9
Jiang, F., Deng, Y., Yeh, C. K., and Sun, Y. (2014). Quaternized chitosans bind onto preexisting biofilms and eradicate pre-attached microorganisms. J. Mater. Chem. B 2, 8518–8527. doi: 10.1039/C4TB01131G
Kang, J., Jin, W., Wang, J., Sun, Y., Wu, X., and Liu, L. (2019). Antibacterial and anti-biofilm activities of peppermint essential oil against Staphylococcus aureus. LWT 101, 639–645. doi: 10.1016/j.lwt.2018.11.093
Khan, F., Pham, D. T. N., Oloketuyi, S. F., Manivasagan, P., Oh, J., and Kim, Y. M. (2020). Chitosan and their derivatives: antibiofilm drugs against pathogenic bacteria. Colloids Surf. B: Biointerfaces 185:110627. doi: 10.1016/j.colsurfb.2019.110627
Khan, S., Tøndervik, A., Sletta, H., Klinkenberg, G., Emanuel, C., Onsøyen, E., et al. (2012). Overcoming drug resistance with alginate oligosaccharides able to potentiate the action of selected antibiotics. Antimicrob. Agents Chemother. 56, 5134–5141. doi: 10.1128/AAC.00525-12
Kim, J.-H., Yu, D., Eom, S.-H., Kim, S.-H., Oh, J., and Jung, W.-K. (2017). et al, Synergistic antibacterial effects of chitosan-caffeic acid conjugate against antibiotic-resistant acne-related bacteria. Mar. Drugs 15:167. doi: 10.3390/md15060167
Kolter, J., and Henneke, P. (2017). Codevelopment of microbiota and innate immunity and the risk for group B streptococcal disease. Front. Immunol. 8:1497. doi: 10.3389/fimmu.2017.01497
Lin, A. E., Autran, C. A., Szyszka, A., Escajadillo, T., Huang, M., Godula, K., et al. (2017). Human milk oligosaccharides inhibit growth of group B Streptococcus. J. Biol. Chem. 292, 11243–11249. doi: 10.1074/jbc.M117.789974
Lin, S.-B., Chen, S.-H., and Peng, K.-C. (2009). Preparation of antibacterial chito-oligosaccharide by altering the degree of deacetylation of β-chitosan in a Trichoderma harzianum chitinase-hydrolysing process. J. Sci. Food Agric. 89, 238–244. doi: 10.1002/jsfa.3432
Lindberg, E., Adlerberth, I., Hesselmar, B., Saalman, R., Strannegård, I. L., Åberg, N., et al. (2004). High rate of transfer of Staphylococcus aureus from parental skin to infant gut Flora. J. Clin. Microbiol. 42, 530–534. doi: 10.1128/JCM.42.2.530-534.2004
Liu, H., Du, Y., Wang, X., and Sun, L. (2004). Chitosan kills bacteria through cell membrane damage. Int. J. Food Microbiol. 95, 147–155. doi: 10.1016/j.ijfoodmicro.2004.01.022
Lu, Y., Slomberg, D. L., and Schoenfisch, M. H. (2014). Nitric oxide-releasing chitosan oligosaccharides as antibacterial agents. Biomaterials 35, 1716–1724. doi: 10.1016/j.biomaterials.2013.11.015
Marks, L. R., Clementi, E. A., and Hakansson, A. P. (2013). Sensitization of Staphylococcus aureus to methicillin and other antibiotics In vitro and In vivo in the presence of HAMLET. PLoS One 8:e63158. doi: 10.1371/journal.pone.0063158
Mataraci, E., and Dosler, S. (2012). In vitro activities of antibiotics and antimicrobial cationic peptides alone and in combination against methicillin-resistant Staphylococcus aureus biofilms. Antimicrob. Agents Chemother. 56, 6366–6371. doi: 10.1128/AAC.01180-12
Melin, P. (2011). Neonatal group B streptococcal disease: from pathogenesis to preventive strategies. Clin. Microbiol. Infect. 17, 1294–1303. doi: 10.1111/j.1469-0691.2011.03576.x
Moon, J. S., Kim, H. K., Koo, H. C., Joo, Y. S., Nam, H. M., Park, Y. H., et al. (2007). The antibacterial and immunostimulative effect of chitosan-oligosaccharides against infection by Staphylococcus aureus isolated from bovine mastitis. Appl. Microbiol. Biotechnol. 75, 989–998. doi: 10.1007/s00253-007-0898-8
Moormeier, D. E., and Bayles, K. W. (2017). Staphylococcus aureus biofilm: a complex developmental organism. Mol. Microbiol. 104, 365–376. doi: 10.1111/mmi.13634
Pillai, S. K., Moellering, R. C. Jr., and Eliopoulos, G. M. (2005). “Antimicrobial combinations,” in Antibiotics in Laboratory Medicine. 5th Edn. ed. V. Lorian (Philadelphia, PA, USA: Lippincott Williams and Wilkins), 365–440.
Pinna, A., Donadu, M. G., Usai, D., Dore, S., D’Amico-Ricci, G., Boscia, F., et al. (2020). In vitro antimicrobial activity of a new ophthalmic solution containing povidone-iodine 0.6% (IODIM®). Acta Ophthalmol. 98, e178–e180. doi: 10.1111/aos.14243
Powell, L. C., Pritchard, M. F., Ferguson, E. L., Powell, K. A., Patel, S. U., Rye, P. D., et al. (2018). Targeted disruption of the extracellular polymeric network of Pseudomonas aeruginosa biofilms by alginate oligosaccharides. NPJ Biofilms Microbi. 4, 1–10. doi: 10.1038/s41522-018-0056-3
Powell, L. C., Sowedan, A., Khan, S., Wright, C. J., Hawkins, K., Onsøyen, E., et al. (2013). The effect of alginate oligosaccharides on the mechanical properties of Gram-negative biofilms. Biofouling 29, 413–421. doi: 10.1080/08927014.2013.777954
Ramezani Ali Akbari, K., and Abdi Ali, A. (2017). Study of antimicrobial effects of several antibiotics and iron oxide nanoparticles on biofilm producing pseudomonas aeruginosa. Nanomed. J. 4, 37–43. doi: 10.22038/nmj.2017.8051
Reffuveille, F., Josse, J., Vallé, Q., Mongaret, C., and Gangloff, S. C. (2017). “Staphylococcus aureus biofilms and their impact on the medical field,” in The Rise of Virulence and Antibiotic Resistance in Staphylococcus aureus. eds. S. Enany and L. E. Crotty Alexander (London, UK: InTech Open).
Rosini, R., and Margarit, I. (2015). Biofilm formation by Streptococcus agalactiae: influence of environmental conditions and implicated virulence factor. Front. Cell. Infect. Microbiol. 5:6. doi: 10.3389/fcimb.2015.00006
Roy, R., Tiwari, M., Donelli, G., and Tiwari, V. (2018). Strategies for combating bacterial biofilms: a focus on anti-biofilm agents and their mechanisms of action. Virulence 9, 522–554. doi: 10.1080/21505594.2017.1313372
Ruppen, C., Hemphill, A., and Sendi, P. (2017). In vitro activity of gentamicin as an adjunct to penicillin against biofilm group B Streptococcus. J. Antimicrob. Chemother. 72, 444–447. doi: 10.1093/jac/dkw447
Sheen, T. R., Jimenez, A., Wang, N.-Y., Banerjee, A., van Sorge, N. M., and Doran, K. S. (2011). Serine-rich repeat proteins and Pili promote Streptococcus agalactiae colonization of the vaginal tract. J. Bacteriol. 193:6834. doi: 10.1128/JB.00094-11
Sun, W., Weingarten, R. A., Xu, M., Southall, N., Dai, S., Shinn, P., et al. (2016). Rapid antimicrobial susceptibility test for identification of new therapeutics and drug combinations against multidrug-resistant bacteria. Emerg. Microbes Infect. 5:e116. doi: 10.1038/EMI.2016.123
Taylor Nordgård, C., and Draget, K. I. (2011). Oligosaccharides as modulators of rheology in complex mucous systems. Biomacromolecules 12, 3084–3090. doi: 10.1021/bm200727c
Tin, S., Lim, C., Sakharkar, M., and Sakharkar, K. (2009). Synergistic combinations of chitosans and antibiotics in Staphylococcus aureus. Lett. Drug Des. Discov. 7, 31–35. doi: 10.2174/157018010789869406
Tong, S. Y. C., Davis, J. S., Eichenberger, E., Holland, T. L., and Fowler, V. G. (2015). Staphylococcus aureus infections: epidemiology, pathophysiology, clinical manifestations, and management. Clin. Microbiol. Rev. 28, 603–661. doi: 10.1128/CMR.00134-14
Uriarte, S. M., Randis, T., Behnsen, J., Gaddy, J. A., Kothary, V., Doster, R. S., et al. (2017). Group B Streptococcus induces neutrophil recruitment to gestational tissues and elaboration of extracellular traps and nutritional immunity. Front. Cell. Infect. Microbiol. 7:19. doi: 10.3389/fcimb.2017.00019
Wright, G. D. (2016). Antibiotic adjuvants: rescuing antibiotics from resistance. Trends Microbiol. 24, 862–871. doi: 10.1016/j.tim.2016.06.009
Yang, S., Hay, I. D., Cameron, D. R., Speir, M., Cui, B., Su, F., et al. (2015). Antibiotic regimen based on population analysis of residing persister cells eradicates Staphylococcus epidermidis biofilms. Sci. Rep. 5:18578. doi: 10.1038/srep18578
Yildirim-Aksoy, M., Beck, B. H., and Zhang, D. (2019). Examining the interplay between Streptococcus agalactiae, the biopolymer chitin and its derivative. MicrobiologyOpen 8:e00733. doi: 10.1002/mbo3.733
Zheng, W., Sun, W., and Simeonov, A. (2018). Drug repurposing screens and synergistic drug-combinations for infectious diseases. Br. J. Pharmacol. 175, 181–191. doi: 10.1111/bph.13895
Zheng, L. Y., and Zhu, J. F. (2003). Study on antimicrobial activity of chitosan with different molecular weights. Carbohydr. Polym. 54, 527–530. doi: 10.1016/j.carbpol.2003.07.009
Keywords: alginate oligosaccharides, chitosan oligosaccharides, Staphylococcus aureus, group B Streptococcus, bacterial growth, anti-biofilm, synergy, sensitization
Citation: Asadpoor M, Ithakisiou G-N, van Putten JPM, Pieters RJ, Folkerts G and Braber S (2021) Antimicrobial Activities of Alginate and Chitosan Oligosaccharides Against Staphylococcus aureus and Group B Streptococcus . Front. Microbiol. 12:700605. doi: 10.3389/fmicb.2021.700605
Received: 26 April 2021; Accepted: 17 August 2021;
Published: 13 September 2021.
Edited by:
Eduardo Costa, Escola Superior de Biotecnologia - Universidade Católica Portuguesa, PortugalReviewed by:
Tim Maisch, University of Regensburg, GermanyCopyright © 2021 Asadpoor, Ithakisiou, van Putten, Pieters, Folkerts and Braber. This is an open-access article distributed under the terms of the Creative Commons Attribution License (CC BY). The use, distribution or reproduction in other forums is permitted, provided the original author(s) and the copyright owner(s) are credited and that the original publication in this journal is cited, in accordance with accepted academic practice. No use, distribution or reproduction is permitted which does not comply with these terms.
*Correspondence: Saskia Braber, cy5icmFiZXJAdXUubmw=
Disclaimer: All claims expressed in this article are solely those of the authors and do not necessarily represent those of their affiliated organizations, or those of the publisher, the editors and the reviewers. Any product that may be evaluated in this article or claim that may be made by its manufacturer is not guaranteed or endorsed by the publisher.
Research integrity at Frontiers
Learn more about the work of our research integrity team to safeguard the quality of each article we publish.