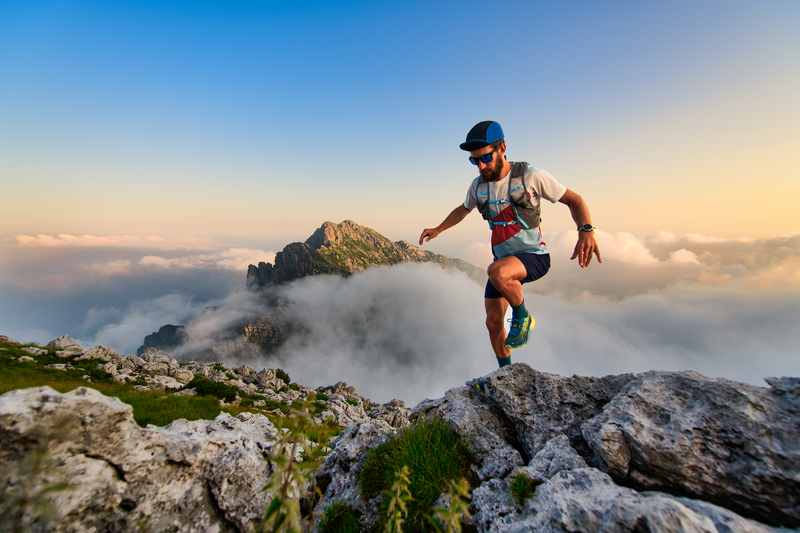
95% of researchers rate our articles as excellent or good
Learn more about the work of our research integrity team to safeguard the quality of each article we publish.
Find out more
ORIGINAL RESEARCH article
Front. Microbiol. , 01 July 2021
Sec. Microbial Physiology and Metabolism
Volume 12 - 2021 | https://doi.org/10.3389/fmicb.2021.700333
Bacterial two-component regulatory systems control the expression of sets of genes to coordinate physiological functions in response to environmental cues. Here, we report a genetically linked but functionally unpaired two-component system (TCS) comprising the sensor kinase GluS (BGLU_1G13350) and the response regulator GluR (BGLU_1G13360), which is critical for cell division in the rice pathogen Burkholderia glumae BGR1. The gluR null mutant, unlike the gluS mutant, formed filamentous cells in Lysogeny Broth medium and was sensitive to exposure to 42°C. Expression of genes responsible for cell division and cell-wall (dcw) biosynthesis in the gluR mutant was elevated at transcription levels compared with the wild type. GluR-His bound to the putative promoter regions of ftsA and ftsZ is involved in septum formation, indicating that repression of genes in the dcw cluster by GluR is critical for cell division in B. glumae. The gluR mutant did not form filamentous cells in M9 minimal medium, whereas exogenous addition of glutamine or glutamate to the medium induced filamentous cell formation. These results indicate that glutamine and glutamate influence GluR-mediated cell division in B. glumae, suggesting that GluR controls cell division of B. glumae in a nutrition-dependent manner. These findings provide insight into how the recognition of external signals by TCS affects the sophisticated molecular mechanisms involved in controlling bacterial cell division.
Two-component systems (TCSs) consisting of a sensor kinase and a cognate response regulator are common in bacteria (Gao and Stock, 2009). They are essential for the responses of bacteria to changes in environmental factors such as pH, osmotic pressure, antibiotics, and quorum-sensing (QS) signals (Gao and Stock, 2009). The sensor kinases are autophosphorylated after sensing an environmental stimulus, followed by phosphotransfer from the phosphorylated sensor kinases to the response regulators (Gao and Stock, 2009). The phosphorylated response regulators then undergo conformational changes to become active, thereby controlling the expression of target genes (Gao and Stock, 2009). The genes encoding sensor kinases and response regulators are often genetically linked in bacterial genomes and functionally paired (Stenson et al., 2005; Coutte et al., 2016). In addition to paired TCSs, sensor kinases and transcriptional regulators can crosstalk, thus modulating multiple biological processes in response to environmental signals irrespective of their genetic linkage (Hellingwerf, 2005; Yamamoto et al., 2005; Coutte et al., 2016; Chen et al., 2017).
We study the social behavior and host interactions of the rice bacterial pathogen Burkholderia glumae, the cause of rice panicle blight (Kim et al., 2004; Goo et al., 2015). A phytotoxin, toxoflavin, is the major virulence factor of B. glumae and exerts a toxic effect on photosynthetic organisms by generating radicals under light (Kim et al., 2004; Koh et al., 2011). The virulence-factor biosynthesis and motility of B. glumae are dependent on QS (Kim et al., 2004, 2007). As well as QS, we are interested in TCSs in B. glumae BGR1 because they coordinate and regulate the expression of genes critical for adaptation to stress, survival, fitness in the host, and virulence (Uhl and Miller, 1996; Perraud et al., 1998; Groisman, 2001; Bronner et al., 2004; Loui et al., 2009; Freeman et al., 2013; Yan et al., 2020). For instance, CpxAR of Actinobacillus pleuropneumoniae (Yan et al., 2020), ArcAB of Escherichia coli (Loui et al., 2009), and KdpDE (Freeman et al., 2013) and PhoPQ (Groisman, 2001) in a variety of bacterial taxa reportedly promote growth, fitness, and survival in the host. In addition, AgrAC, SsrAB, SaeRS, and ArlRS of Staphylococcus aureus and BvgAS of Bordetella pertussis are necessary for virulence (Uhl and Miller, 1996; Perraud et al., 1998; Bronner et al., 2004). Few studies have focused on TCSs in B. glumae, probably because of concern over repeating works on other pathogens. However, Karki et al. reported that the PidS/PidR TCS is essential for the pigmentation and virulence of B. glumae 411gr-6 (Karki et al., 2012).
In this study, we identified a TCS composed of the sensor kinase GluS and the response regulator GluR, which was critical for normal cell division in B. glumae BGR1. gluR and gluS were co-transcribed, but GluR functioned independently of GluS in normal cell division. We report that GluR regulates the gene cluster involved in cell division and cell wall (dcw) biosynthesis and conclude that external nutritional conditions modulate cell division in a TCS-dependent manner in B. glumae.
The bacterial strains and plasmids used are listed in Supplementary Table 1. Unless stated otherwise, the strains were grown in Lysogeny Broth (LB) medium containing 0.1% (w/v) tryptone, 0.5% (w/v) yeast extract, 0.5% (w/v) sodium chloride, and 1.5% agar as required (Affymetrix, Cleveland, OH) with the appropriate antibiotics at 37°C. Antibiotics were used at the following concentrations: rifampicin, 100 μg/ml; ampicillin, 50 μg/ml; tetracycline, 10 μg/ml; kanamycin, 25 and 50 μg/ml; and spectinomycin, 50 μg/ml. 5-Bromo-4-chloro-3-indoyl-β-D-galactopyranoside (X-gal) was added at 40 μg/ml as necessary.
Basic DNA manipulation was conducted following standard protocols (Sambrook et al., 1989). Plasmid DNA from E. coli was isolated using the Biomedic Plasmid DNA Miniprep Kit (Ibiomedic, Bucheon, South Korea) following the manufacturer’s instructions. DNA sequencing was performed by Macrogen Inc. (Seoul, South Korea). The genetic information and gene IDs for DNA construction were obtained from the B. glumae BGR1 genome database (GenBank accession numbers: CP001503–CP001508; kropbase.snu.as.kr/cgi_bg.cg).
We used a previously constructed cosmid library of B. glumae BGR1 (Kim et al., 2004).
With the use of E. coli S17-1 (pRescue mini-Tn5), random mutations were created in B. glumae BGR1 as described previously (De Lorenzo et al., 1990). Successful mutants were isolated by selection on LB agar containing kanamycin. The rescued mini-Tn5 mutants were screened for phenotypic changes under the microscope. Following a previous method (Kwon and Ricke, 2000), the flanking regions were sequenced using the O-end primer (5′-GGTTTTCACCGTCATCACCG-3′), and the TCS genes were disrupted using the identified rescue mini-Tn5 insertions. The selected mini-Tn5rescue mutant, RT271, was complemented by tri-parental mating (Figurski and Helinski, 1979) using pBGH1 plasmid to generate RT271C.
The pLAFR3 derivatives of pBGH1 carrying gluR (BGLU_1G13360) and gluS (BGLU_1G13350) were mutagenized using Tn3-gusA as described previously (Bonas et al., 1989). The Tn3-gusA insertion site and orientation in each mutant were mapped by restriction enzyme digestion analysis, and the plasmid was sequenced using the Tn3gus primer (5′-CCGGTCATCTGAGACCATTAAAAGA-3′). The plasmids carrying Tn3-gusA insertions were marker-exchanged into B. glumae BGR1 via tri-parental mating (Figurski and Helinski, 1979) to generate BGLUR133 and BGLUS35. All marker-exchange mutants were confirmed by Southern hybridization analysis.
We used the pBGH13 plasmid, a derivative of pBGH1, to complement the gluR mutant. First, pBGH1 DNA was digested with ScaI followed by ligation into pBluescript II SK (+). The resulting plasmid DNA was cut with BamHI and HindIII followed by ligation into pLAFR3, resulting in pBGH13. The pBGH13 was introduced into BGLUR133 via tri-parental mating (Figurski and Helinski, 1979) to produce BGLUR133C.
Overnight liquid cultures of the B. glumae strains were adjusted to an OD600 of 0.05 and subcultured into fresh LB medium. The cultures were incubated for 30 h at 37°C with shaking at 250 rpm. At 6-hour intervals, bacterial growth was assayed by spotting 10 μl of serial dilutions in triplicate on LB agar plates. Bacterial growth was expressed as log CFU/ml after 2 days of incubation at 37°C.
Cell viability was assayed using the LIVE/DEAD BacLight Bacterial Viability Kit, which contains SYTO 9 green-fluorescent nucleic acid stain and the red-fluorescent nucleic acid stain, and propidium iodide (Invitrogen, Carlsbad, CA, United States), following the manufacturer’s instructions. Fluorescence images were captured using a confocal laser scanning microscope (Leica SP8X, Wetzlar, Germany) at excitation/emission wavelengths of 483/490–540 and 535/890–680 nm for green and red fluorescence, respectively.
Bacterial cells were harvested from overnight cultures and prepared for observation by transmission electron microscopy (TEM), as reported previously (Kang et al., 2017). Electron micrographs were acquired using a JEM 1010 microscope (JEOL, Tokyo, Japan) with acceleration voltages of 180 and 100 kV from a LIBRA 120 energy-filtration microscope (Carl Zeiss, Oberkochen, Germany).
Total RNA was isolated from B. glumae BGR1, BGLUR133, and BGLUR133C using the RNeasy Mini Kit (Qiagen, Hilden, Germany) following the manufacturer’s instructions. Genomic DNA was removed using DNase I (Thermo Fisher Scientific, Vilnius, Lithuania). From 1 μg of RNA, reverse transcription for cDNA synthesis was performed at 42°C for 1 h with the Recombinant RNasin and M-MLV Reverse transcriptase following manufacturer’s instructions (Promega, Madison, WI, United States). With the use of specific primer sets (Supplementary Table 2), ftsA, ftsB, ftsI, ftsK, ftsL, ftsQ, ftsW, and ftsZ cDNAs were synthesized. Transcription levels were determined using SsoFast EvaGreen Supermix (Bio-Rad, Hercules, CA, United States) under the following conditions: 95°C for 30 s followed by 30 cycles of 95°C for 5 s and 55°C for 5 s. With the use of SensiFast SYBR No-ROX Kit (Bioline, Meridian Bioscience, Cincinnati, OH, United States), PCR was performed in triplicate, and gene expression values were normalized to 16S rRNA using Bio-Rad CFX Manager software.
The B. glumae BGR1, TCS null mutants, and BGLUR133C strains were cultured overnight at 37°C, and the optical density at 600 nm (OD600) was adjusted to 0.05. The strains were incubated at 42°C with shaking at 250 rpm for 24 h in LB and M9 minimal media (6 g of Na2HPO4, 3 g of KH2PO4, 0.5 g of NaCl, and 1 g of NH4Cl in 1 L of deionized water containing 1 mM of MgSO4 and 0.1 mM of CaCl2, supplemented with 0.2% glucose), and the cell density was measured at 6-hour intervals.
We cultured the wild type, gluR mutant BGLUR133, and BGLUR133C in M9 minimal medium. To evaluate whether amino acids are required for GluR activity, M9 minimal medium was supplemented with 10% Bacto Casamino Acids (Becton, Dickson and Co., Franklin Lakes, NJ, United States) that comprise 20 essential amino acids. Individual amino acids (Sigma Aldrich, St. Louis, MI, United States) were analyzed at the concentrations in LB medium (Sezonov et al., 2007).
Overnight liquid cultures of the wild-type BGR1 were adjusted to an optical density of OD600 of 0.05 and subcultured in LB medium for 24 h at 37°C with shaking at 250 rpm. At 3-hour intervals, the cultures were centrifuged (14,000 rpm, 4°C, 10 min), and the supernatants were collected. Glutamate analysis was carried out by liquid chromatography–mass spectrometry (LCMS-2000, Shimadzu, Kyoto, Japan) at the National Instrumentation Center and Environment Management (Seoul National University, Seoul, South Korea).
B. glumae strains cultured overnight in LB or M9 minimal medium with/without amino acids were harvested, fixed with Karnovsky’s fixative [2% glutaraldehyde, 2% paraformaldehyde in 0.05 M of sodium cacodylate buffer (pH 7.4)], and post-fixed with 1% sodium tetroxide in 0.1 M of sodium cacodylate buffer for 1 h at 4°C as described previously (Morris, 1965). Before imaging, the samples were coated with platinum at 10 mA for 270 s using a G20 Ion Sputter Coater (GSEM Co., Suwon, South Korea), and electron micrographs were acquired using a Carl Zeiss microscope (Auriga, Zeiss Germany).
The coding region of gluR was amplified from BGR1 chromosomal DNA with the primers, gluR_Nde1-F and gluR_BamH1-R (Supplementary Table 2), and then cloned into the NdeI and BamHI sites of pET21b (Invitrogen) to produce pGluR-His. GluR-His was overexpressed in E. coli strain BL21 (DE3) followed by purification using a Ni-NTA spin column in a buffer containing 50 mM of Tris–HCl (pH 8.0) and 100 mM of NaCl as described by the manufacturer (Qiagen). With primer sets ftsAp-F/R and ftsZ-F/R (Supplementary Table 2), the promoter regions of the putative GluR targets, ftsAp and ftsZp, respectively, were amplified. The resulting PCR products were labeled with biotin using LightShift Chemiluminescent Electrophoretic Mobility Shift Assay Kits, as described by the manufacturer (Pierce, Appleton, WI, United States). We used 329 bp upstream of katE1 as a non-specific competitor DNA amplified using KatE1-F and KatE1-R primers (Supplementary Table 2). Purified GluR-His (0.75 μM) was incubated in binding buffer (10 mM of Tris–HCl (pH 7.5), 100 mM NaCl, and 5% (v/v) glycerol) containing 1 nM biotin-labeled DNA as described previously (Kim et al., 2007). For competition assays, unlabeled target DNA at 20-fold molar excess was added to each reaction with the labeled DNA. With the use of 4% (w/v) polyacrylamide gels, the reactions were separated and transferred to nitrocellulose membranes. The bands were detected using streptavidin/horseradish peroxidase-derived chemiluminescence kits, as described by the manufacturer (Pierce) and visualized using ChemiDoc XRS + and Image Lab Software (Bio-Rad).
All experiments were conducted in triplicate with the appropriate controls. One-way analysis of variance (ANOVA) followed by Tukey’s honestly significant difference post hoc analysis in SPSS software (ver. 25 × 86-x64; IBM Corp., Armonk, NY, United States) were conducted to detect significant differences. A value of p < 0.05 was considered indicative of statistical significance.
All the figures presented in this manuscript were prepared using Adobe illustrator 2020 v. 24.3, available at: https://dobe.com/products/illustrator.
To identify a key TCS important for normal cell division of B. glumae BGR1, we first mutagenized it with mini-Tn5 and examined the morphology of the mutants. The mutant RT271 formed filamentous cells when grown in LB medium (Figure 1A); and its respective mutant complementation, RT271C, restored the rod-shaped cells similar to those in the wild-type BGR1 (Figure 1A). To determine the insertion site of mini-Tn5 in the RT271 mutant, a mini-Tn5 insertion along with flanking sequences was rescued by digestion of its genomic DNA with EcoRI, self-ligation, and transformation into E. coli DH5α. Flanking sequences of mini-Tn5 from the rescued plasmid pRT271E revealed that an annotated gene BGLU_1G13360 had an insertional mutation (Figure 1B). This gene, gluR, encoded a 27.7-kDa protein that exhibited 99.6% similarity to known OmpR-type response regulators such as BURPS305_7006 in Burkholderia pseudomallei 305, RisA (BMA10247_1253) in Burkholderia mallei NCTC 10247, and BCENMCO3_1962 of Burkholderia cenocepacia MCO-3 (Supplementary Figure 1A). Downstream of gluR was a putative sensor kinase, gluS (BGLU_1G13350) (Figure 1B) that showed 96.7%, 94.1%, and 90.0% identities with known sensor kinases such as Envz1 (BGL_1C23830) in Burkholderia plantarii, BGLA_1G24110 in Burkholderia gladioli BSR3, and RisS (BMA1486) in B. mallei ATCC 23344, respectively (Supplementary Figure 1B).
Figure 1. The GluS-GluR two-component system (TCS) of Burkholderia glumae BGR1. (A) Microscopic observation of the cell morphology of BGR1 (wild type), RT271 (BGR1, gluR:mini-Tn5rescue), and mutant complemented RT271C (BGR1, gluR:mini-Tn5rescue carrying pBGH1) strains in Lysogeny Broth (LB) medium. (B) Organization scheme of the GluS sensor kinase and GluR response regulator in pBGH1. Vertical bars above the restriction map indicate the position of the Tn5 (271) and Tn3-gusA insertions (35 and 133). E, EcoRI; H, HindIII; B, BamHI; S, SacI. The map below pBGH1 represents pBGH13 plasmid of a 6.3-kb fragment containing gluS and gluR genes. (C) RT-PCR analysis showing that gluS and gluR genes are co-transcribed in BGR1. Primers were designed to amplify a 237-bp (*) product encompassing the gluR and gluS genes in the wild type. Lane G, PCR product using genomic DNA as a template; Lane R, PCR product using RNA as a template; Lane C, PCR product using cDNA as a template. (D) No polar effect resulting from Tn3-gusA insertion. Lane bp, marker; Lane G1, PCR product from gluR chromosomal DNA as a template; Lane G2, PCR product from gluS chromosomal DNA as a template; Lane R, PCR product from total RNA as a template; Lane C1, PCR product from gluR cDNA as a template; Lane C2, PCR product from gluS cDNA as a template. The thick bars below the gene map indicate the position that was amplified for cDNA synthesis. Primers were designed to amplify 200 bp (1) and 216 bp (2) product of gluS and gluR, respectively. Full gel images are presented in Supplementary Figures 6, 7.
Due to the proximity of gluS and gluR in the BGR1 genome, we reasoned that these two genes might be co-transcribed into a polycistronic RNA. Therefore, we performed reverse transcription–polymerase chain reaction (RT-PCR) with specific primers, GluSR-F/R (Figure 1B and Supplementary Table 2). We found that gluR and gluS were indeed co-transcribed (Figure 1C). We next mutagenized pBGH1, a cosmid carrying gluS and gluR, with Tn3-gusA to generate mutants of gluR and gluS followed by marker exchange into B. glumae BGR1, resulting in BGLUR133 (BGR1 gluR:Tn3-gusA133) and BGLUS35 (BGR1 gluS:Tn3-gusA35) (Figure 1B). In the gluR mutant, the expression of gluS was decreased, suggesting the possibility of polar effects due to the transposon insertion in gluR (Figure 1D).
To determine whether the insertion of Tn3-gusA in gluR or gluS conferred a similar cell morphology to the RT217 mutant, we observed the morphology of the gluR and gluS mutants under a light microscope. The gluR mutant BGLUR133 showed extensive filamentous cells in LB medium (Figure 2), consistent with the initial phenotype of the gluR:min-Tn5 mutant RT271 in LB (Figure 1A). However, the gluS mutant BGLUS35 formed normal cells in LB medium (Figure 2), implying that the polar effect seen in Figure 1D was not significant. The gluR mutant BGLUR133 maintained a normal rod-shaped cell morphology similar to that of the gluS mutant BGLUS35 in M9 minimal medium supplemented with glucose (Figure 2). Evaluating the expression of gluS and gluR in LB and M9 minimal medium showed that gluR was more abundant in LB than M9 medium, while gluS showed the opposite pattern (Supplementary Figures 2A,B), suggesting that nutrient conditions may differentially regulate the two genes. TEM of ultrathin sections of the gluR mutant BGLUR133 revealed characteristic features of filamentous cells with multiple nuclei and indents along the cell membrane at points where the septum would have formed to separate dividing cells (Figure 3A). The genetically complemented strain of the gluR mutant BGLUR133 with pBGH13, BGLUR133C, had morphologically uniform rod-shaped cells (Figure 3A). The growth of the gluR mutant BGLUR133 and the wild-type BGR1 for 30 h in LB medium at 37°C was similar (Supplementary Figure 3 and Figure 3B). Although filamentous cells of the gluR mutant BGLUR133 remained viable for 30 h, their abundance decreased after 18 h, as observed under the microscope (Figure 3B).
Figure 2. Tn3-gusA mutations in gluR resulted in nutrient-dependent cell filamentation. In Lysogeny Broth (LB) medium, the gluR mutant BGLUR133 formed filamentous cells, but a normal rod-shaped cell morphology was observed in M9 minimal medium. No morphological defects were observed in the gluS mutant BGLUS35 in the different culture media.
Figure 3. The gluR mutant forms a heterogeneous population of viable filamentous and normal rod-shaped cells. (A) The indicated bacterial strains were grown to the early stationary phase, and the morphological phenotypes of ultrathin sections were observed by transmission electron microscopy (TEM). BGLUR133-M shows that the filamentous cells formed by the gluR mutant contained multiple nuclei (arrows) with indents (arrowhead) along the cell wall, symbolizing failed septum formation. (B) Cell viability of the wild type, BGLUR133, and complemented BGLUR133C strains assessed by combination staining with propidium iodide (PI) and SYTO 9 green. Fluorescence images were obtained by confocal laser scanning microscopy. Dead cells stained with PI are red, and SYTO 9-stained viable cells are green.
Because TEM suggested the involvement of GluR in cell division, we determined whether GluR influences the expression of genes in the dcw cluster involved in cell division. In B. glumae, there were 15 annotated genes: e.g., ftsA, ftsI, ftsL, ftsQ, ftsW, and ftsZ, in the dcw cluster and ftsB and ftsK in other regions (Figures 4A,B). The expression levels of ftsA, ftsB, ftsI, ftsK, ftsL, ftsQ, ftsW, and ftsZ in the gluR mutant BGLUR133 were significantly increased compared with those in the wild-type BGR1 (Figure 4C). The expression levels of the eight genes in BGLUR133C were similar to those in the wild type (Figure 4C). To determine whether GluR directly controls their expression, we performed electrophoresis mobility shift assays (EMSA) on the putative promoter regions of ftsA and ftsZ and purified His-tagged GluR (GluR-His). The binding of GluR-His to the putative promoter regions of ftsA and ftsZ confirmed that GluR-His directly represses the expression of cell division genes in B. glumae (Figure 4D). In the upstream regions of ftsA and ftsZ, we found a conserved inverted repeat sequence, indicating a potential GluR binding site (Supplementary Figure 4).
Figure 4. GluR represses cell division and septation genes in Burkholderia glumae. (A) Genetic organization of the dcw cluster in BGR1. Dark arrows represent genes involved in septation during cell division, and light arrows are genes involved in cell-wall synthesis or with no known function. The section below the gene map indicates the positions and size of the respective putative promoters in this study. (B) Gene maps of additional cell-division genes outside the dcw cluster. (C) Expression levels of eight cell-division genes in the wild type (BGR1), gluR mutant, and complemented BGLUR133C strains compared by qRT-PCR. mRNA levels were normalized to 16S rRNA, and the fold expressions are relative to those of the wild type. Data are means ± standard error (SE) of triplicates; statistical analysis was performed by one-way ANOVA/Tukey’s correlation for multiple comparisons. *p < 0.05; [F (48,71) = 1,536.273; p = 0.00]. (D) Electrophoresis mobility shift assay (EMSA) showed direct control of ftsA and ftsZ by GluR-His binding to the respective putative promoter regions; 0.75 μM of GluR-His, 1 nM of labeled target DNA, 1 nM of unlabeled katE non-competitor DNA, and 20 nM of unlabeled target promoter DNA were used for EMSA. Full blot images with multiple contrasts are shown in Supplementary Figure 8.
Because the gluR mutant BGLUR133 formed filamentous cells in LB medium but not in M9 minimal medium, we reasoned that the amino acids in LB medium might be the cause of filamentous cell formation. Therefore, we added 10% casamino acids to M9 minimal medium containing glucose to evaluate their influence on the morphology of the gluR mutant BGLUR133. Adding casamino acids to M9 minimal medium transformed the morphologically normal cells of the gluR mutant BGLUR133 into filamentous cells (Figure 5). To identify the amino acids(s) responsible for triggering filamentous cells in the gluR mutant BGLUR133, 20 amino acids were individually added to M9 minimal medium. Of the 20 amino acids, only glutamine and glutamate individually triggered cells of the gluR mutant BGLUR133 to become filamentous in M9 minimal medium (Figures 6A,B). These results suggested that glutamine and glutamate play a role in gluR-mediated cell division in B. glumae.
Figure 5. Extracellular amino acids promote filamentation in response to GluR mutation. The indicated bacterial strains were cultured overnight in M9 minimal medium with or without 10% casamino acids (CA) and processed for SEM analysis, and their morphology was observed using a Carl Zeiss GmbH Auriga microscope.
Figure 6. Extracellular glutamine and glutamate are required for GluR-mediated cell division. The gluR mutant BGLUR133 formed filamentous cells in glutamine (A) and glutamate (B)-rich M9 minimal medium. (C,D) Differences in the expression levels of septation genes in plain M9 (C) and glutamine-rich M9 minimal medium (D), analyzed by qRT-PCR using the wild-type BGR1 as the baseline. Data are means ± standard error (SE) of triplicates.
Because environmental glutamine affected the cell morphology of the gluR mutant BGLUR133 in M9 minimal medium, we examined the expression levels of seven fts genes in M9 minimal medium with or without glutamine. In the absence of glutamine, the expression levels of the seven fts genes were significantly lower in the gluR mutant BGLUR133 than in the wild type, or the BGLUR133C complemented strain (Figure 6C). However, the addition of glutamine to M9 minimal medium increased the expression levels of the seven fts genes in the gluR mutant BGLUR133 (Figure 6D).
Because fts genes were identified in a temperature-sensitive filamenting mutant, we assessed whether the filamenting gluR mutant BGLUR133 is heat sensitive. Despite having no effect at 37°C (Supplementary Figure 3), the number of cells of the gluR mutant BGLUR133 decreased significantly after 6 h at 42°C, and they were entirely non-viable after 12 h in LB medium (Figure 7A). The wild-type BGR1, the gluS mutant BGLUS35, and the complemented strain BGLUR133C showed no growth but prolonged survival at 42°C (Figure 7A). In M9 minimal medium at 42°C, the gluR mutant BGLUR133 retained viability for 18 h and subsequently lost viability (Figure 7B). By contrast, the wild-type BGR1 and the complemented strain BGLUR133C increased in cell number during the static period of gluR mutant BGLUR133 in M9 medium (Figure 7B).
Figure 7. Exponential population decline at 42°C as a result of mutations in GluR. At 6-h intervals, the indicated strains’ population densities in Lysogeny Broth (LB) medium (A) and M9 medium (B) were quantified by colony-forming unit (CFU) counting, and the results are expressed as log CFU/ml. Data are means ± standard error (SE) of triplicates.
In addition to QS systems, pathogens also respond to environmental factors using TCSs. Here, we investigated the response regulator, GluR, which is crucial for normal cell division in B. glumae. Although gluR and gluS were co-transcribed, GluS may not be a bona fide counterpart of GluR because a mutation in gluS did not affect the normal cell division of B. glumae. Such a genetically linked but functionally independent TCS was reported for risS and risA, which encode a sensor kinase and a response regulator, respectively, in B. pertussis (Stenson et al., 2005). risS and risA were genetically linked but functionally independent (Stenson et al., 2005). Phosphorylation of RisA was mediated by crosstalk with a non-operonic histidine kinase, RisK (Chen et al., 2017). Therefore, an as-yet-unidentified sensor kinase may be responsible for the phosphorylation of GluR in B. glumae.
In rod-shaped bacteria such as B. glumae, cell division involves ingrowth of the cell wall and membrane, forming a septum between two replicated chromosomes (Harry, 2001). To ensure equal partitioning of chromosomes into daughter cells, the expression of genes involved in cell division must be properly regulated (Dai and Lutkenhaus, 1992; Harry, 2001). In most bacteria, cell division and cell-wall synthesis are regulated by a series of genes in the dcw cluster (Dai and Lutkenhaus, 1992). Within bacterial groups of the same class and cell shape, the order and regulation of genes in the dcw cluster are highly conserved (Pilhofer et al., 2008). Therefore, it was not surprising that in B. glumae, the dcw cluster displayed significant similarities to that of E. coli (Vicente et al., 1998). Pioneer studies of the dcw cluster genes in E. coli spotlighted ftsZ as the key element in cell division (Bi and Lutkenhaus, 1991; De Boer et al., 1992). It was later noted that FtsZ is not sufficient to drive septation, leading to the discovery of, for instance, ftsA, ftsQ, and ftsI (Vicente et al., 1998). In a hierarchical order initiated by the assembly of FtsZ at the division site, the dcw proteins are coordinately involved in cell division and the synthesis of the peptidoglycan precursors (Vicente et al., 1998; Lutkenhaus and Du, 2017).
The mechanisms of regulation of the dcw cluster are unclear, despite the presence therein of several regulatory elements, e.g., internal promoters, transcript stabilizers, and protein ratios (Vicente et al., 1998; Francis et al., 2000). Studies on the control of cell division have concentrated on FtsZ. Multiple promoter regions have been reported upstream of ftsZ in the dcw cluster, indicating regulation at the transcriptional level (Vicente et al., 1998; Francis et al., 2000; Margolin, 2000). We found that GluR binds to the upstream promoter regions of ftsZ and ftsA located in the ftsA and ftsQ coding regions, respectively. A conserved inverted repeat sequence was also found in the upstream sequences of ftsA and ftsZ. This suggested a possible GluR binding sequence, albeit mutational analysis of the putative promoter regions of both genes and DNase footprinting experiments are needed to validate it. Unlike positive regulators in E. coli, such as SdiA (Sitnikov et al., 1996), the phase-specific sigma factor (Ballesteros et al., 1998), and RcsB (Carballès et al., 1999), GluR negatively regulates cell division in B. glumae. Biased expression of the dcw cluster genes resulting from a mutation in gluR-induced aberrant cell division suggested that the GluR-controlled expression of dcw cluster genes was essential for normal cell division in B. glumae. Similar findings have been reported in other bacteria species, such as E. coli, where high levels of dcw genes expression impeded cell division, as seen by the production of filamentous cells in some cases (Dai and Lutkenhaus, 1992).
Because LB medium is rich in amino acids, and filamentation of the gluR mutant was facilitated by supplementation of extracellular glutamine or glutamate in M9 medium, the glutamine-dependent filamentous cell formation at an early stage of growth in LB was explicable. However, the number of filamentous cells of the gluR mutant BGLUR133 decreased over time, possibly as a result of depletion of amino acids, including glutamine and glutamate, 12 h after incubation (Supplementary Figure 5). It is clear that GluR and glutamine/glutamate are involved in normal cell division of B. glumae; however, it is not conclusive how these and other factors work together for the gluR mutant phenotypes in M9 minimal medium. Extracellular glutamine and glutamate reportedly alter the expression of genes involved in cell division and cell-wall synthesis of Bacillus subtilis (Ye et al., 2009). Beuria et al. reported an increased FtsZ polymerization rate and extent in E. coli that resulted from extracellular glutamine (Beuria et al., 2003). It was noted that FtsZ showed optimal polymerization as large, bundled filamentous structures in E. coli in the presence of 1 M of glutamine (Beuria et al., 2003). Interestingly, FtsZ polymers formed in the absence of glutamine were 9-fold less stable than those in its presence, emphasizing the roles of these amino acids in the stability of FtsZ polymers (Beuria et al., 2003).
Connections between TCS and glutamine metabolism have been reported in other bacteria. For example, GlnK-GlnL of B. subtilis (Satomura et al., 2005), GluR-GluK of Streptomyces coelicolor (Li et al., 2017), and AauR-AauS of Pseudomonas putida (Sonawane et al., 2006) reportedly sense and control glutamate uptake. In other bacteria, the TCSs involved in glutamine sensing and uptake are located close to the glutamine ABC transporter (Satomura et al., 2005; Sonawane et al., 2006; Li et al., 2017). However, GluR is not likely to be involved in glutamine uptake because we reported that GltI is responsible for glutamine uptake in B. glumae (Kang et al., 2017). A bona fide sensor kinase responsible for glutamine sensing and GluR phosphorylation is yet to be identified in B. glumae.
Physiological experiments in E. coli demonstrated that mutated septation genes resulted in elongated cells and an exponential population decrease at high temperatures, giving the mutants the name filamentous temperature sensitive (Ricard and Hirota, 1973). While we did not specifically modify the septation genes of B. glumae in the gluR mutant BGLUR133, we observed identical phenotypes of cell elongation and sensitivity to heat treatment at 42°C as in E. coli with mutated septation genes (Ricard and Hirota, 1973). These findings further support the hypothesis that GluR is crucial for cell division and an optimum gene expression profile. Taken together, our findings indicate that GluR is key for maintaining the gene expression profile required for glutamine- or glutamate-dependent control of cell division in B. glumae BGR1.
The original contributions presented in the study are included in the article/Supplementary Material, further inquiries can be directed to the corresponding author/s.
JM and IH designed the experiments. JM and EG performed the experiments. JM, EG, YK, and IH analyzed the data. JM and IH contributed reagents/materials, and analysis tools, and wrote the manuscript. All authors contributed to the article and approved the submitted version.
This work was supported by a National Research Foundation of Korea grant (2020R1F1A1062829) funded by the Ministry of Science and ICT of the Korean government.
The authors declare that the research was conducted in the absence of any commercial or financial relationships that could be construed as a potential conflict of interest.
We thank Woon Jeong Kim and Bongsoo Lee for the generation of gluS and gluR mutants.
The Supplementary Material for this article can be found online at: https://www.frontiersin.org/articles/10.3389/fmicb.2021.700333/full#supplementary-material
Ballesteros, M., Kusano, S., Ishihama, A., and Vicente, M. (1998). The ftsQ1p gearbox promoter of Escherichia coli is a major sigma S-dependent promoter in the ddlB–ftsA region. Mol. Microbiol. 30, 419–430.
Beuria, T. K., Krishnakumar, S. S., Sahar, S., Singh, N., Gupta, K., Meshram, M., et al. (2003). Glutamate-induced assembly of bacterial cell division protein FtsZ. J. Biol. Chem. 278, 3735–3741. doi: 10.1074/jbc.M205760200
Bi, E., and Lutkenhaus, J. (1991). FtsZ ring structure associated with division in Escherichia coli. Nature 354, 161–164.
Bonas, U., Stall, R. E., and Staskawicz, B. (1989). Genetic and structural characterization of the avirulence gene avrBs3 from Xanthomonas campestris pv. vesicatoria. Mol. Gen. Genet. 218, 127–136.
Bronner, S., Monteil, H., and Prévost, G. (2004). Regulation of virulence determinants in Staphylococcus aureus: complexity and applications. FEMS Microbiol. Rev. 28, 183–200. doi: 10.1016/j.femesre.2003.09.003
Carballès, F., Bertrand, C., Bouché, J. P., and Cam, K. (1999). Regulation of Escherichia coli cell division genes ftsA and ftsZ by the two-component system rcsC–rcsB. Mol. Microbiol. 34, 442–450.
Chen, Q., Ng, V., Warfel, J. M., Merkel, T. J., and Stibitz, S. (2017). Activation of Bvg-repressed genes in Bordetella pertussis by RisA requires cross talk from noncooperonic histidine kinase RisK. J. Bacteriol. 199, e475–e417. doi: 10.1128/JB.00475-17
Coutte, L., Huot, L., Antoine, R., Slupek, S., Merkel, T. J., Chen, Q., et al. (2016). The multifaceted RisA regulon of Bordetella pertussis. Sci. Rep. 6:32774. doi: 10.1038/srep32774
Dai, K., and Lutkenhaus, J. (1992). The proper ratio of FtsZ to FtsA is required for cell division to occur in Escherichia coli. J. Bacteriol. 174, 6145–6151.
De Boer, P., Crossley, R., and Rothfield, L. (1992). The essential bacterial cell-division protein FtsZ is a GTPase. Nature 359, 254–256.
De Lorenzo, V., Herrero, M., Jakubzik, U., and Timmis, K. N. (1990). Mini-Tn5 transposon derivatives for insertion mutagenesis, promoter probing, and chromosomal insertion of cloned DNA in gram-negative eubacteria. J. Bacteriol. 172, 6568–6572.
Figurski, D. H., and Helinski, D. R. (1979). Replication of an origin-containing derivative of plasmid RK2 dependent on a plasmid function provided in trans. Proc. Natl. Acad. Sci. U S A. 76, 1648–1652.
Francis, F., Ramirez-Arcos, S., Salimnia, H., Victor, C., and Dillon, J.-A. R. (2000). Organization and transcription of the division cell wall (dcw) cluster in Neisseria gonorrhoeae. Gene 251, 141–151. doi: 10.1016/S0378-1119(00)00200-6
Freeman, Z. N., Dorus, S., and Waterfield, N. R. (2013). The KdpD/KdpE two-component system: integrating K+ homeostasis and virulence. PLoS Pathog. 9:e1003201. doi: 10.1371/journal.ppat.1003201
Gao, R., and Stock, A. M. (2009). Biological insights from structures of two-component proteins. Annu. Rev. Microbiol. 63, 133–154. doi: 10.1146/annurev.micro.091208.073214
Goo, E., An, J. H., Kang, Y., and Hwang, I. (2015). Control of bacterial metabolism by quorum sensing. Trends Microbiol. 23, 567–576. doi: 10.1016/j.tim.2015.05.007
Groisman, E. A. (2001). The pleiotropic two-component regulatory system PhoP-PhoQ. J. Bacteriol. 183, 1835–1842. doi: 10.1128/JB.183.6.1835-1842.2001
Harry, E. (2001). Bacterial cell division: regulating Z-ring formation. Mol. Microbiol. 40, 795–803.
Hellingwerf, K. J. (2005). Bacterial observations: a rudimentary form of intelligence? Trends Microbiol. 13, 152–158. doi: 10.1016/j.tim.2005.02.001
Kang, Y., Goo, E., Kim, J., and Hwang, I. (2017). Critical role of quorum sensing-dependent glutamate metabolism in homeostatic osmolality and outer membrane vesiculation in Burkholderia glumae. Sci. Rep. 7:44195. doi: 10.1038/srep33195
Karki, H. S., Barphagha, I. K., and Ham, J. H. (2012). A conserved two-component regulatory system, PidS/PidR, globally regulates pigmentation and virulence-related phenotypes of Burkholderia glumae. Mol. Plant Pathol. 13, 785–794. doi: 10.1111/j.1364-3703.2012.00787.x
Kim, J., Kang, Y., Choi, O., Jeong, Y., Jeong, J. E., Lim, J. Y., et al. (2007). Regulation of polar flagellum genes is mediated by quorum sensing and FlhDC in Burkholderia glumae. Mol. Microbiol. 64, 165–179. doi: 10.1111/j.1365-2958.2007.05646.x
Kim, J., Kim, J. G., Kang, Y., Jang, J. Y., Jog, G. J., Lim, J. Y., et al. (2004). Quorum sensing and the LysR-type transcriptional activator ToxR regulate toxoflavin biosynthesis and transport in Burkholderia glumae. Mol. Microbiol. 54, 921–934. doi: 10.1111/j.1365-2958.2004.04338.x
Koh, S., Kim, H., Kim, J., Goo, E., Kim, Y. J., Choi, O., et al. (2011). A novel light-dependent selection marker system in plants. Plant Biotechnol. J. 9, 348–358. doi: 10.1111/j.1467-7652.2010.00557.x
Kwon, Y., and Ricke, S. (2000). Efficient amplification of multiple transposon-flanking sequences. J. Microbiol. Methods 41, 195–199. doi: 10.1016/S0167-7012(00)00159-7
Li, L., Jiang, W., and Lu, Y. (2017). A novel two-component system, GluR-GluK, involved in glutamate sensing and uptake in Streptomyces coelicolor. J. Bacteriol. 199, e97–e17. doi: 10.1128/JB.00097-17
Loui, C., Chang, A. C., and Lu, S. (2009). Role of the ArcAB two-component system in the resistance of Escherichia colito reactive oxygen stress. BMC Microbiol. 9:183. doi: 10.1186/147-2180-9-183
Margolin, W. (2000). Themes and variations in prokaryotic cell division. FEMS Microbiol. Rev. 24, 531–548. doi: 10.1111/j.1574-6976.2000.tb00554.x
Morris, J. K. (1965). A formaldehyde glutaraldehyde fixative of high osmolality for use in electron microscopy. J. Cell Biol. 27, 1A–149A.
Perraud, A. L., Kimmel, B., Weiss, V., and Gross, R. (1998). Specificity of the BvgAS and EvgAS phosphorelay is mediated by the C-terminal HPt domains of the sensor proteins. Mol. Microbiol. 27, 875–887.
Pilhofer, M., Rappl, K., Eckl, C., Bauer, A. P., Ludwig, W., Schleifer, K.-H., et al. (2008). Characterization and evolution of cell division and cell wall synthesis genes in the bacterial phyla Verrucomicrobia, Lentisphaerae, Chlamydiae, and Planctomycetes and phylogenetic comparison with rRNA genes. J. Bacteriol. 190, 3192–3202. doi: 10.1128/JB.01797-07
Ricard, M., and Hirota, Y. (1973). Process of cellular division in Escherichia coli: physiological study on thermosensitive mutants defective in cell division. J. Bacteriol. 116, 314–322.
Sambrook, J., Fritsch, E. F., and Maniatis, T. (1989). Molecular cloning: A laboratory manual, 2nd Edn. New York, NY: Cold Spring Harbor Laboratory Press.
Satomura, T., Shimura, D., Asai, K., Sadaie, Y., Hirooka, K., and Fujita, Y. (2005). Enhancement of glutamine utilization in Bacillus subtilis through the GlnK-GlnL two-component regulatory system. J. Bacteriol. 187, 4813–4821. doi: 10.1128/JB.187.14.4813-4821.2005
Sezonov, G., Joseleau-Petit, D., and D’ari, R. (2007). Escherichia coli physiology in Luria-Bertani broth. J. Bacteriol. 189, 8746–8749. doi: 10.1128/JB.01368-07
Sitnikov, D. M., Schineller, J. B., and Baldwin, T. O. (1996). Control of cell division in Escherichia coli: regulation of transcription of ftsQA involves both rpoS and SdiA-mediated autoinduction. Proc. Natl. Acad. Sci. U S A. 93, 336–341.
Sonawane, A. M., Singh, B., and Röhm, K.-H. (2006). The AauR-AauS two-component system regulates uptake and metabolism of acidic amino acids in Pseudomonas putida. Appl. Environ. Microbiol. 72, 6569–6577. doi: 10.1128/AEM.00830-06
Stenson, T. H., Allen, A. G., Al-Meer, J. A., Maskell, D., and Peppler, M. S. (2005). Bordetella pertussis risA, but not risS, is required for maximal expression of Bvg-repressed genes. Infect. Immun. 73, 5995–6004. doi: 10.1128/IAI.73.9.5995-6004.2005
Uhl, M., and Miller, J. (1996). Integration of multiple domains in a two-component sensor protein: the Bordetella pertussis BvgAS phosphorelay. EMBO J. 15, 1028–1036.
Vicente, M., Gomez, M., and Ayala, J. (1998). Regulation of transcription of cell division genes in the Escherichia coli dcw cluster. Cell. Mol. Life Sci. 54, 317–324.
Yamamoto, K., Hirao, K., Oshima, T., Aiba, H., Utsumi, R., and Ishihama, A. (2005). Functional characterization in vitro of all two-component signal transduction systems from Escherichia coli. J. Biol. Chem. 280, 1448–1456. doi: 10.1074/jbc.M410104200
Yan, K., Liu, T., Duan, B., Liu, F., Cao, M., Peng, W., et al. (2020). The CpxAR Two-Component System Contributes to Growth, Stress Resistance, and Virulence of Actinobacillus pleuropneumoniae by Upregulating wecA Transcription. Front. Microbiol. 11:1026. doi: 10.3389/fmicb.01026
Keywords: Burkholderia glumae, two-component regulatory system, cell division, GluR, rice panicle blight
Citation: Marunga J, Goo E, Kang Y and Hwang I (2021) Identification of a Genetically Linked but Functionally Independent Two-Component System Important for Cell Division of the Rice Pathogen Burkholderia glumae. Front. Microbiol. 12:700333. doi: 10.3389/fmicb.2021.700333
Received: 26 April 2021; Accepted: 04 June 2021;
Published: 01 July 2021.
Edited by:
Hari S. Misra, Bhabha Atomic Research Centre (BARC), IndiaReviewed by:
Jong Hyun Ham, Louisiana State University, United StatesCopyright © 2021 Marunga, Goo, Kang and Hwang. This is an open-access article distributed under the terms of the Creative Commons Attribution License (CC BY). The use, distribution or reproduction in other forums is permitted, provided the original author(s) and the copyright owner(s) are credited and that the original publication in this journal is cited, in accordance with accepted academic practice. No use, distribution or reproduction is permitted which does not comply with these terms.
*Correspondence: Ingyu Hwang, aW5neXVAc251LmFjLmty
Disclaimer: All claims expressed in this article are solely those of the authors and do not necessarily represent those of their affiliated organizations, or those of the publisher, the editors and the reviewers. Any product that may be evaluated in this article or claim that may be made by its manufacturer is not guaranteed or endorsed by the publisher.
Research integrity at Frontiers
Learn more about the work of our research integrity team to safeguard the quality of each article we publish.