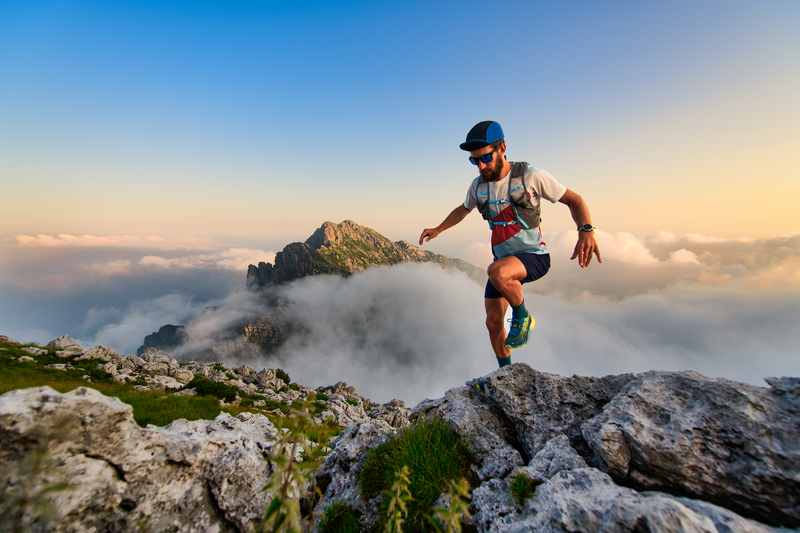
95% of researchers rate our articles as excellent or good
Learn more about the work of our research integrity team to safeguard the quality of each article we publish.
Find out more
ORIGINAL RESEARCH article
Front. Microbiol. , 03 August 2021
Sec. Microbiotechnology
Volume 12 - 2021 | https://doi.org/10.3389/fmicb.2021.696473
This article is part of the Research Topic Microbial Electrogenesis, Microbial Electrosynthesis and Electro-bioremediation View all 5 articles
The efficient delivery of electrochemically in situ produced H2 can be a key advantage of microbial electrosynthesis over traditional gas fermentation. However, the technical details of how to supply large amounts of electric current per volume in a biocompatible manner remain unresolved. Here, we explored for the first time the flexibility of complex 3D-printed custom electrodes to fine tune H2 delivery during microbial electrosynthesis. Using a model system for H2-mediated electromethanogenesis comprised of 3D fabricated carbon aerogel cathodes plated with nickel-molybdenum and Methanococcus maripaludis, we showed that novel 3D-printed cathodes facilitated sustained and efficient electromethanogenesis from electricity and CO2 at an unprecedented volumetric production rate of 2.2 LCH4 /Lcatholyte/day and at a coulombic efficiency of 99%. Importantly, our experiments revealed that the efficiency of this process strongly depends on the current density. At identical total current supplied, larger surface area cathodes enabled higher methane production and minimized escape of H2. Specifically, low current density (<1 mA/cm2) enabled by high surface area cathodes was found to be critical for fast start-up times of the microbial culture, stable steady state performance, and high coulombic efficiencies. Our data demonstrate that 3D-printing of electrodes presents a promising design tool to mitigate effects of bubble formation and local pH gradients within the boundary layer and, thus, resolve key critical limitations for in situ electron delivery in microbial electrosynthesis.
Bioelectrochemical technologies represent a key platform for recycling of CO2 into useful fuels and chemicals to enable a circular carbon economy (Harnisch et al., 2015; Parag and Sovacool, 2016; De Luna et al., 2019). For bioelectrochemical CO2 conversion to operate at industrial scale, two critical performance metrics need to be achieved: (1) high reaction rates, requiring current densities in the range of 100–1,000 mA/cm2 and (2) a high selectivity for production of the target compound (>90%) (Bushuyev et al., 2018; De Luna et al., 2019). In this context, microbial electrosynthesis (MES) is particularly promising as biological syntheses are highly selective, including for complex, multi-carbon compounds. Such high selectivity is achieved when using strictly anaerobic microorganisms that typically utilize H2, HCO2–, or CO as catabolic electron source and convert these electrons at a selectivity of >90% to specific catabolic end products (Daniell et al., 2016). So far, the volumetric electron supply in MES has been limited to current densities in the range of 1 mA/cm2, with rates up to 20 mA/cm2 only reported for projected surface areas that widely underestimate the actual active electrode surface (Prévoteau et al., 2020). Therefore, a key improvement target for transferring this technology from laboratory to larger scale is increasing the current density to maximize the electron feed per volume (Dessì et al., 2020; Jourdin et al., 2020). Hydrogen-driven MES is particularly promising as H2 can be electrochemically produced at high rates and 100% selectivity using inexpensive materials and is used by a wide variety of anaerobic microorganisms (Blanchet et al., 2015; Kracke et al., 2019). In traditional microbial gas fermentation, high volumetric production rates are achieved but the handling and dispersion of sparingly soluble H2 as electron carrier is a major challenge (Liew et al., 2013). MES is similar to H2/CO2-gas fermentation, with the important difference that in MES H2 is formed in situ at the site of microbial consumption and, therefore, circumvents the challenges of introducing bulk H2 gas via extensive purging and mixing. Further, the electrochemical production of H2 as intermediate provides a platform for electricity storage by converting electrical into chemical energy in form of “green methane” (bio-power-to-gas), which can be used in the existing natural gas infrastructure.
Over the past decade, diverse cathodes have been purposely build or modified to enhance MES processes by improved properties such as increasing hydrogen formation (Zhang et al., 2013; Aryal et al., 2017; Kracke et al., 2019). Cathodes with intrinsically high surface area, such as carbon granules, cloth, fiber, or reticulated vitreous carbon, have emerged as particularly useful to improve the process performance by retention of high bacteria loadings on electrodes and improved start up times (Zhang et al., 2013; Aryal et al., 2017; Kracke et al., 2019). However, a systematic variation of surface area and the relationship between surface and current densities has not been explored so far.
Additive manufacturing, commonly known as 3D-printing, has been a major driver of innovation in industrial manufacturing and could prove to be revolutionary for the field of MES by offering flexibility in complex and custom design of electrochemically active materials (Barnatt, 2014; Lee et al., 2019). The technology enables the rapid development and testing of customized electrode and reactor prototypes and has recently been successfully applied for improved electrochemical carbon dioxide reduction (Corral et al., 2021). In addition to the fabrication of reactor components, 3D-printing is particularly interesting for the production of next generation electrodes as the technology enables the design of high surface area materials with possible structuring up to nanometer-scale via post-treatment methods (Lee et al., 2019). Complex custom designs can be realized, such as incorporation of flow channels to make high surface area per volume accessible, while high conductivity over large electrodes is provided via incorporation of a metallic core. One option to manufacture conductive metal substrates is the printing of metal powder (Kassym and Perveen, 2020). In addition, the direct electrochemical 3D-printing of metallic materials through electrochemical reduction of metal ions from solutions onto 3D-printed conductive substrates is in development (Chen et al., 2017). First studies in the field of bioelectrochemical technologies have explored the 3D printing of electrodes for microbial fuel cell applications (Chung and Dhar, 2021). This includes the printing of metallic, porous 3D-structured materials (Calignano et al., 2015; Zhou et al., 2017), conductive polymeric materials with subsequent surface modifications or carbonisation to increase conductivity and bio-compatibility (You et al., 2017; Bian et al., 2018; Pumera, 2019), as well as the 3D printing of active bio-electrodes by direct incorporation of living bacteria (Shewanella oneidensis MR-1) into a printable ink (Freyman et al., 2020). Importantly, in a recent study He et al. report the development of a 3D-printed graphene oxide aerogel anode, which achieved record volumetric current output using a pure culture of Geobacter sulfurreducens (He et al., 2021). The authors attribute the performance increase to improved mass transfer properties of the material (compared to carbon felt) enabled by hierarchical pores in their 3D-printed anode (He et al., 2021). These results are a promising indication that 3D-printing presents a suitable tool to address the mass transfer limitation in hydrogen driven microbial CO2 reduction.
Here, we demonstrate, to our knowledge, the first use of cathodes fabricated via 3D-printing for microbial electrosynthesis. We manufactured 3D-electrodes with varying ratios of surface area to volume and used these to study the effect of in situ H2 supply as a function of current density on electromethanogenesis using the methanogen Methanococcus maripaludis as model system.
Carbon aerogel (CA) cathodes coated with a NiMo-alloy were used to enable the fabrication of highly conductive, hydrogen evolving cathodes via additive manufacturing (Chandrasekaran et al., 2018; Kracke et al., 2019). The base CAs were prepared from resorcinol and formaldehyde gels, which were poured into custom 3D-printed acrylonitrile-butadiene-styrene (ABS) molds formed as the inverse of our desired final shape (see Figure 1A). After thermal curing of the gel, the mold was removed by dissolving in acetone, leaving the complex shaped raw organic aerogel, which was carbonized at high temperature in inert atmosphere to yield the CA cathodes. To obtain complex shape casts, acrylonitrile-butadiene-styrene (ABS) molds were printed on a Lulzbot Taz 6 fused filament 3-D printer. Cylindrical lattice electrodes were constructed with 3 mm strut thickness, where the geometric surface area of the electrode was controlled by the density of struts (and thus, the specific surface area) within the lattice. For lattice cylinder electrodes with 55, 89, and 111 cm2 geometric surface area, simple cubic lattices with specific surface area 0.10, 0.12, and 0.15 cm2/cm3 were used, respectively. Electrodes were designed to occupy a cylinder with dimensions 4 cm height by 2.4 cm diameter. Molds based on the electrodes were created by inverting the design; the molds were printed at 135% of the designed size, to account for shrinkage of the material during carbonization of the organic aerogel to form the carbon aerogel.
Figure 1. Additive manufacturing process of the NiMo-plated carbon aerogel cathodes (A). Visualization of computational models of inversed ABS molds for 3D-printing (B) and photograph of one set of finished NiMo-plated carbon aerogel cathodes of varying surface areas (C). The geometric surface areas of the different cathodes are from left to right 39, 55, 86, and 111 cm2.
Carbon aerogels were prepared from resorcinol and formaldehyde (RF) gels. In a typical synthesis, resorcinol (1.23 g) and a 37% formaldehyde solution (1.7 g) in water (1.5 ml) were mixed together, followed by addition of 2N nitric acid (44 μl). The mixture was poured into a custom 3D-printed ABS-mold, which was placed in a sealed glass jar and allowed to cure in an oven at 80°C for 24 h to form wet organic aerogels. After curing, the ABS mold was completely dissolved in a bath of acetone. The acetone bath also served to solvent exchange the organic aerogels. Typically, the acetone was refreshed 2 or 3 times to fully remove the ABS mold. The organic aerogels were then allowed to dry in the fume hood for 24 h. The dried aerogels were carbonized in a tube furnace under nitrogen atmosphere at 1,050°C for 3 h with a heating and cooling rate of 2°C/min to obtain carbon aerogels. The CA electrodes were attached to a copper wire as a current collector using conductive Ag epoxy, which was then sealed with a non-conductive epoxy. The copper wire was insulated to ensure the electrochemical activity would occur only at the electrode.
The CA electrodes were electroplated with a catalyst-layer of nickel-molybdenum for enhanced hydrogen evolution properties under biological conditions. For this, each cathode was placed in NiMo-plating solution at ∼1 cm distance to a surrounding anode (Platinum mesh, PT008720 -350-210-23, Goodfellow), which was separated from the cathode using nylon filter bags (180 micron mesh, SLSON, Amazon.com). NiMo-plating solution contained per liter: 40 g NiCl2⋅6H2O, 25 g Na2MoO4⋅2H2O, 45 g sodium citrate. The pH was adjusted to 10 with NH4OH. Under constant magnetic stirring at 900 rpm, a constant current of 50 mA/cm2 was applied to obtain a deposition of 60 Coulombs/cm2. The cathodes were soaked in deionized water to remove residues from the NiMo-plating before use in the bio-electrochemical system.
The archaeon M. maripaludis was chosen due to its salinity tolerance and its exceptional performance in previous studies (Kracke et al., 2020). M. maripaludis was cultured using chemically defined medium-JD specifically modified for use in bio-electrochemical reactors as reported previously (Kracke et al., 2020). In brief, medium-JD contained per liter: 30 g Na2SO4, 4 g MgSO4 × 7 H2O, 0.2 g KH2PO4, 0.4 g NH4HCO3, 0.6 g KHCO3, 0.04 g CaCl2, 7.2 g morpholinepropanesulfonic (MOPS) acid, 3.4 g MOPS Na-salt, 1 ml selenite-tungstate solution, 1 ml trace element solution SL-10, 1 g NaS2O3, 0.4 g Cysteine-HCl and 30 mL of 1 M NaHCO3 solution. The final medium-JD was prepared from autoclaved anoxic stock solutions under continuous gassing with CO2/N2 (20/80% v/v). The final pH was 6.8.
Methanococcus maripaludis was routinely grown in batch cultures in medium-JD under H2/CO2 (80/20 %v/v) gas atmosphere at 30°C and 250 rpm. Each reactor was inoculated from an exponentially growing culture.
The bioelectrochemical set up was described in detail previously (Kracke et al., 2019). In brief, two-chamber glass H-cells were separated by a Nafion 117 proton-exchange membrane (Fuel Cell Store Inc., College Station, TX, United States, surface area 4.9 cm2). The volume of cathode and anode chamber was 100 mL, respectively. In each reactor, one three-dimensional cathode, fabricated as reported above, was inserted into the cathode compartment and connected by threading the copper wire through a gastight rubber stopper. The anode was a platinized titanium mesh (1′′ × 4′′, TWL, Amazon) and the reference electrode Ag/AgCl (NaCl saturated; BASI). The electrochemical reactors were controlled by applying a constant current using a multichannel potentiostat (VMP3; Bio-Logic Science Instruments, France). For experiment 1 and 2 the current was set to 50 mA per reactor and for experiment 3 the current was increased stepwise in increments of 10 to 60 mA, 70 mA and finally 80 mA. Each time, the system was monitored until steady state conditions were reached, which was defined at a minimum of three consecutive measurements of constant methane production rate (cf. Figure 4). The pH in the catholyte was monitored and remained constant (pH7) at all times.
CO2 was supplied continuously at a flowrate of 0.15 mL min–1 (CO2 100%) controlled via mass flow controllers (EL-Flow F-100D, Bronkhorst) to ensure non CO2-limiting conditions throughout the experiments. The microbial growth medium-JD was continuously supplied via peristaltic pump at a constant feed rate of 0.0676 ± 0.0025 mL min–1. Each chamber was magnetically stirred at 700 rpm. All reactors were operated at ambient pressure in a temperature-controlled room at 30°C. Prior to inoculation the system was operated for 72 h to reach stable pH conditions in the cathode chambers [see detailed description elsewhere (Kracke et al., 2020)].
Liquid samples were taken through rubber side ports at regular intervals. Samples for gas analysis were taken from the respective reactor headspace with a gas syringe. Volumetric gas flow rates of the reactor off gas were measured via Milligas counter (KG MGC-1, Ritter Apparatebau GmbH & Co., Germany) at regular intervals.
Methane and hydrogen were measured using a gas chromatograph (equipped with a thermal conductivity detector and a flame ionization detection detector, Agilent 6890N, Agilent, Santa Clara, CA, United States). Gaseous headspace samples were taken in regular intervals from the cathode chamber with a gastight syringe (VICI). For each sample, 300 μl were injected into a gas chromatograph equipped with a thermal conductivity detector and a flame ionization detection detector. Analysis of methane and was made via the flame ionization detector. Separation of CH4 and H2 was accomplished in a GS-Q capillary column (30 m length, 0.530 μM ID) and helium was used as carrier gas at a flow rate of 7.4 ml min–1. The injector (split ratio 0.1:1) and flame ionization detector temperatures were 250°C, and analyses were isothermal at 100°C column temperature.
Cell densities were measured via Spectroscopy at 600 nm (Ultrospec 2100 pro, Amersham BioSciences, Little Chalfont, United Kingdom).
Coulombic efficiencies were calculated by dividing the electrons recovered in methane by the electrons supplied as current at a certain time point according to equation 1 below. With η(CH4,t) = mol CH4 at time point t; f(e,CH4) = molar conversion factor (8 electrons per mol CH4); F = Faraday constant (96,485 C mol–1 of electron) and I = electric current.
The estimation of the current density at which bubble formation occurs was done using Fick’s law (Eq. 2) and the following assumptions. The diffusion coefficient (D) for hydrogen in water is 5.5∗10–5 cm2 s–1 (atmospheric pressure, 30°C) (Verhallen et al., 1984). The solubility limit for hydrogen at 30°C in water is 0.75 mM (Verhallen et al., 1984). Assuming complete utilization of H2 by hydrogenotrophic microbes in the bulk liquid (∂φ = 0.75 mM) and a diffusive boundary layer of thickness ∂x = 100 μm (Kracke et al., 2019).
Therefore, in good approximation 4.1 nmol s–1 H2 will diffuse away from the electrode per cm2. This corresponds to a maximum current density of around 0.8 mA/cm2 before bubble formation occurs in this system.
To test how the cathodic current density influences process parameters in hydrogen-driven microbial electrosynthesis, we used additive manufacturing to design and fabricate hydrogen-evolving cathode prototypes with identical catalytic properties and overall volume but varying surface areas.
The base material was comprised of carbon aerogel, which exhibits a high electrical conductivity, and good structural stability (Chandrasekaran et al., 2018). After the final synthesis step of carbonization at high temperature in inert atmosphere, the cathodes were electroplated with NiMo-alloy to enhance the hydrogen evolution properties, as described previously (see Figure 1A) (Kracke et al., 2019). Sets of electrodes that share identical overall cylindrical volume of 18 cm3 were fabricated with varying geometric surface areas 55, 86, and 111 cm2 by controlling the density of lattice struts contained within the cylinder (Figures 1B,C). A filled cylinder cathode with the geometric surface area of 39 cm2 was prepared via NiMo-plating of a commercial graphite rod with the same overall cylinder dimensions, as the bulk cylinder CA fabrication did not result in uniform shapes due to uneven shrinkage and cracking during drying and carbonization.
The novel 3D cathodes were employed in an integrated bioelectrochemical reactor as described previously (Kracke et al., 2020). In brief, four identical H-cell reactors were operated in chemostat-mode under continuous supply of gaseous CO2 and fresh, sterile growth medium. The supply of catabolic electrons was controlled by applying a constant current of 50 mA per reactor. Cathodes evolved H2 at 100% selectivity (Kracke et al., 2019) and, therefore, supplied equal amounts of H2 to each reactor. The current density varied based on the available cathode geometric surface area; the tested specific current densities were 0.5, 0.6, 0.9, and 1.3 mA/cm2. After an abiotic equilibration period of 72 h, each reactor was inoculated with the anaerobic archaeon M. maripaludis to an initial optical density of OD600, start = 0.02.
The methane production profiles of the different reactors during this first experiment indicated that efficient electromethanogenesis in this system strongly depended on the current density of the cathode (see Figure 2, Exp1, left column). At the lowest current density of 0.5 mA/cm2 (high surface area), stable electromethanogenesis was observed within 24 h reaching an average coulombic efficiency of 98 ± 0.2%. With increasing current density, steady state operation was delayed and H2 concentration in the reactor off-gas increased. At 0.6 mA/cm2 highly efficient methane production was established only after 145 h (CE = 97 ± 0.4%), while the reactor operating at 0.9 mA/cm2 did not reach stable methane production rate within the monitored timeframe of 1 week. At the highest current density of 1.3 mA/cm2 tested, substantial underutilization of H2 was observed, which resulted in an overall low coulombic efficiency of 20 ± 4% for methane (averaged between 50 and 190 h). These data demonstrate that the cathodes fabricated with 3D-printing technology are suitable for direct integration with microbial electrosynthesis; however, the overall start-up time and coulombic efficiency strongly depended on the cathodic current density.
Figure 2. Methane production rate, unused hydrogen in the reactor off gas and microbial growth during Exp1 (initial OD = 0.02, left column) and Exp2 (initial OD = 0.1, right column).
To investigate whether the observed effect of current density on start-up times during experiment 1 was dependent on the initial cell density, we repeated the experiment (identical set-up, unused set of cathodes) and increased the initial cell density by a factor of 5 to an OD600, start = 0.1 (Exp2). At a higher initial cell density, start-up times were significantly shortened but lower current densities still resulted in improved overall process performance (see Figure 2, Exp2, right column). For current densities of 0.5 and 0.6 mA/cm2, highly efficient methane production was achieved within less than 7 h (for coulombic efficiencies see Figure 3). At a current density of 0.9 mA/cm2, steady state methane production was reached after 55 h, while the low-inoculum culture of Exp1 did not reach stable production rates within 7 days at this current density. Apparently, electromethanogenesis at the highest current density of 1.3 mA/cm2 tested did not significantly benefit from a high initial cell density. Again, significant amounts of H2 were lost in the reactor off gas and the average coulombic efficiency for methane was only 12 ± 2% (cf. Figure 3).
Figure 3. Coulombic efficiencies achieved with the 3D printed cathodes of different surface areas under low cell density (Exp1, left) and high cell density conditions (Exp2, right). The given values are averages of 4–12 individual measurements taken after reaching steady state, with error bars displaying the standard deviation between individual measurements.
While increasing cell density significantly shortened start-up times, the achieved coulombic efficiency in steady state conditions was dependent on the cathodic current density.
Based on the above experiments, we hypothesized that the specific current density on the cathodes is crucial for efficiently delivering H2 in situ into the microbial metabolism. To test this hypothesis, we increased the total current per reactor and, therefore, the current density in three consecutive steps in the following experiment 3 (Figure 4). With increasing current densities, the efficiency of bioelectrochemical methane production decreased as the H2 concentration in the off-gas increased. At 60 mA total current, the reactors with the three highest surface area cathodes enable methane production at almost 100% coulombic efficiency. Increasing the current to 70 mA led to an increase in methane production rate, except in the reactor with the second smallest surface area of 55 cm2, where the methane production rate dropped slightly instead (cf., Figure 4). A further increase in total current to 80 mA resulted in a similar effect for the next larger cathode at 89 cm2. Under these conditions only the cathode with the largest surface area (111 cm2) resulted in a further increase in methane production rate, corresponding to a volumetric methane production rate of 2.2 LCH4 /Lcatholyte/day at a coulombic efficiency of 99%. These observations suggest that after exceeding a critical current density the performance ceases to increase further and, more significantly, drops as highly efficient electromethanogenesis is no longer observed (see Figure 5). For the here tested system using NiMo cathodes, magnetic stirring (700 rpm) and M. maripaludis as microbial catalyst and at the cell densities tested, this critical current density was found to be around ∼1.0–1.5 mA/cm2 (cf. Figure 5). At lower current densities, methane production rates per electrode area increase linearly with increasing current densities (Figure 5A), which indicates highly efficient conversion of the supplied electrical current into methane under these conditions resulting in near 100% coulombic efficiencies (Figure 5B). When current densities between 1.0 and 1.5 mA/cm2 were applied, the product spectrum shifted from methane to hydrogen, which resulted in a drop of coulombic efficiency (Figures 5A,B). At current densities exceeding 1.5 mA/cm2, hydrogen was the major product, increasing linearly per area with the applied current density (Figure 5A). Under these conditions, the cell concentration in the reactor dropped significantly (Figure 5C), despite the increasing amount of hydrogen being produced. These data demonstrate that the efficient in situ delivery of H2 in the bio-electrochemical system strongly depends on the cathodic current density. Large surface area cathodes that enabled current densities to remain below the observed critical current density threshold were successful to facilitate continuous electromethanogenesis via in situ H2 production at record volumetric production rate. The underlying mechanism for this observed behavior is likely a combination of increased loss of gaseous H2 due to increased bubble formation and decreased biocompatibility due to locally high pH at the cathode surface at higher current densities and is discussed in detail in the following section.
Figure 4. Effect of increasing current density on electromethanogenesis performance in reactors with different cathodic surface areas. Methane production rate and unused hydrogen in the reactor off gas during Exp3. The total supplied current was increased stepwise as indicated per dashed vertical lines. Initial OD = 0.1.
Figure 5. Summary of process parameters during electromethanogenesis in dependency of the applied cathodic current density: (A) The rates of methane (diamonds, left axis) and H2 (squares, right axis) produced per cathode area, (B) coulombic efficiencies for methane (circles) and (C) steady state biomass concentrations (triangles). Eight individual electrodes of four different surface areas 39, 55, 86, and 111 cm2 were tested under constant current conditions of 50, 60, 70, and 80 mA. The given values are averages of 4–12 individual measurements under steady state conditions with error bars displaying the standard deviation between individual measurements.
Similar observations of optimum performance in MES at relatively low current densities have been reported for other systems (Bajracharya et al., 2015; Blanchet et al., 2015). In an electromethanogenesis system, Geppert et al. (2019) increased the current density from 0.5 to 3.5 mA/cm2 and found optimum conditions at around 2.5 mA/cm2, while at higher current densities the fraction of electrons lost as unused hydrogen in the off-gas increased. This was interpreted by others as a “metabolic limitation of the biocatalyst” (Dessì et al., 2020). However, microbial production of methane as well as acetate from H2/CO2 is possible at rates exceeding the rates achieved in bioelectrochemical systems today by about one order of magnitude (Rittmann et al., 2012; Kantzow et al., 2015; Asimakopoulos et al., 2018). This demonstrates that the metabolic capacity of the microorganism is not limiting. Instead, our data show that the mode of H2 delivery ultimately determines the fraction of electrons available for microbial metabolism, which, therefore, determines the maximum achievable production rate under the given conditions. For gas fermentation, it is well known that efficient delivery of dissolved H2 is a key limiting factor for maximizing production rates, and, therefore in some cases, requires expensive pressurization to increase H2 dissolution (Phillips et al., 2011, 2017; Asimakopoulos et al., 2018). Thus, the electrochemical production of H2 in situ can provide substantial advantage over introducing and dispersing bulk H2 gas if engineered well (cf. Figure 6).
Figure 6. Conceptual representation of the fate of H2 in a (A) single-phase system at low current density and (B) gas-evolving system at high current density on a hydrogen producing cathode during electrosynthesis. The concentration of dissolved H2 in the bulk aqueous phase is lower than in the boundary layer because of microbial consumption. If the cathodic current density produces dissolved H2 at a rate that exceeds its diffusion out of the boundary layer and microbial consumption, bubble formation will occur (B). Hydrogen gas bubbles can passivate the active catalytic surface area of the cathode and, thus, reduce electric efficiencies. If mass transfer from gaseous H2 in bubbles to dissolved H2 in the bulk electrolyte is limited, there is a net loss of H2 from the reaction space which reduces coulombic efficiency as well as volumetric production rates. When the electrocatalytic H2 production exceeds the rate at which protons diffuse into the boundary layer, the local pH will rise to alkaline levels (indicated red, see B) potentially toxic to the microorganisms. With increasing current density, number and size of bubbles will increase and with that the intensity of all associated effects.
Hydrogen-driven electrosynthesis is mainly performed by cells in suspension in the reactor bulk liquid, where turbulent mixing enhances mass transfer of cathode-derived H2 while p(H2) is effectively drawn close to zero by microbial metabolism (Conrad and Wetter, 1990; Kotsyurbenko et al., 2001; Feldewert et al., 2020). The electrode surface presents a liquid-solid interface, around which a diffusive boundary layer exists. In a magnetically stirred (200 rpm) electromethanogenesis reactor, we previously determined the boundary layer around a graphite rod cathode to be ∼50–100 μm (Kracke et al., 2019). Therefore, electrocatalytically produced H2 needs to pass through this diffusion-limited boundary layer before it is available to the microbial culture in bulk liquid (see Figure 6). At low current density, the rate of formation of dissolved H2 is slower than microbial consumption in and diffusion out of the boundary layer, and therefore no H2 gas bubbles form (Figure 6A). When the current density increases and the rate of hydrogen formation exceeds H2 consumption and diffusion out of the boundary layer, the solubility limit of H2 is reached and gas bubbles start to form (Figure 6B). Such bubble formation in electrosynthesis processes is generally undesirable as they can (i) passivate the active sites of the electrocatalyst reducing the electric efficiency and (ii) escape the reactor unused and, therefore, reduce coulombic efficiencies and lower the purity of output gas products (Figure 6B) (Angulo et al., 2020). While H2 present in bubbles can still be available for metabolic conversion in the reactor’s bulk liquid depending on the geometry and operation, as previously noted for gas fermentation, the size and number of bubbles increases with current density. A video documentation of this process demonstrates the difference in hydrogen bubble formation under constant current conditions on the here tested cathodes with varying surface areas (see Supplementary Information to this article). It can be seen that at identical overall H2 production rate (identical current), the cathodes with lower surface areas lead to significantly increased bubble formation compared to higher surface area cathodes (cf. Supplementary Video 1–3). Based on our calculation using Fick’s law and the constraints of our system (see section “Materials and Methods” for details), formation of H2 bubbles is predicted to start at currents densities exceeding 0.8 mA/cm2. This can explain the observation of significant H2 loss at current densities above 1.0 mA/cm2 in the here tested system (cf. Figure 5).
A second, often underexplored aspect of the boundary layer in bioelectrochemical systems is the formation of a pH gradient across the boundary layer and its effect on microbial metabolism. In circumneutral microbial growth medium the concentration of H+ and OH– ions is low at 0.1 μM (pH = 7). Therefore, electrochemical proton reduction to H2 at even moderate current density can lead to high local pH in the diffusive boundary layer (Jayathilake et al., 2018). While the presence of a buffer in mM concentration can significantly mitigate this effect (Auinger et al., 2011; Shinagawa et al., 2019), the rate of diffusion of the protonated buffer into the boundary layer from the bulk liquid becomes limiting for a sustained formation of dissolved, bubble-free H2 at medium or high current densities (Figure 6B). An alkaline pH in the boundary layer in combination with bubble formation presumably precludes the formation of biofilms at high current densities (Blanchet et al., 2015). Although the largest fraction of microbial activity during H2-driven MES is in the bulk liquid, metabolically active microbes do come in contact with the high pH environment in the cathodic boundary layer. Exposure to alkaline pH environments can compromise microbial organisms like M. maripaludis (Schauer and Whitman, 1989). In fact, in a previous study we found slightly increased cell lysis of M. maripaludis cells grown with in situ electrochemically evolved hydrogen (at 1 mA/cm2) compared to cultures supplied with a gaseous mix of H2/CO2 (Kracke et al., 2020).
In combination, the increased H2 loss through bubble formation and decreased biocompatibility due to high local pH can explain the here observed decline in biomass and methane formation rates after exceeding a critical current density. The drop in methane formation rate seems to slightly precede the decrease in biomass concentration (cf. Figure 5), indicating that in the here investigated system, H2-loss via bubble formation is the dominating effect before bio-incompatible pH conditions are reached at further increased current densities. The specific value of this current density threshold depends for each MES system on its specific properties, including reactor configurations, operating conditions, boundary layer and mixing, and microorganisms chosen. To maximize the microbial production rate per catalytic electrode area, it would be beneficial to operate a microbial electrosynthesis reactor close to its critical current density (cf. Figure 5A). Thus, for designing advanced MES systems, it is of critical importance to consider the above discussed molecular basis of bubble formation and local pH gradients. Because these gradient effects increase with the thickness of the diffusive boundary layer (Vogt, 1980), reducing the size of this layer will mitigate these limitations and maximize the supply of electrons per reactor volume while maintaining biocompatibility. 3D-printing is a particularly promising platform to effectively decrease the boundary layer via advanced electrode and reactor designs that combine high surface areas with improved fluid dynamic properties (Lee et al., 2019) and, therefore, presents a critical opportunity to further improve the performance of MES.
We demonstrated here for the first time electrocatalytically active cathodes fabricated via 3D-printing as a new platform for efficient in situ delivery of H2 in an integrated microbial electrosynthesis system. With this tool, we identified the specific current density on the cathode surface as being critical for (i) fast start-up times of the microbial culture, (ii) stable steady-state performance, and (iii) high coulombic efficiencies via minimizing H2 escape, favoring efficient microbial H2 utilization, and maintaining biocompatible pH conditions near the electrode surface. Moreover, we showed that it is not the metabolic capacity of a microorganism per se that limits product formation rate in a MES, but the physical/chemical conditions in the boundary layer.
This proof-of concept demonstrates that advanced manufacturing of cathodes is a suitable tool for bioelectrochemical technologies, which provides major advances for the rapid development of electrode-prototypes and enables advanced custom design of innovative bio-electrochemical reactors. Even in our non-optimized H-cell reactor the cathodes with highest surface area enabled highly efficient electromethanogenesis at record volumetric rate of 2.2 LCH4 /Lcatholyte/day (111 cm2, – 80 mA, CE = 99%). Future research efforts will show how this new platform can be used to develop microbial electrosynthesis systems via in situ H2 production that provide superior mass transfer conditions compared to a two-step process of water electrolysis and subsequent gas fermentation.
The original contributions presented in the study are included in the article/Supplementary Material, further inquiries can be directed to the corresponding authors.
FK designed and performed the experiments in the integrated bio-electrochemical system, analyzed the data, and drafted the manuscript. BJ and SC fabricated the electrodes. SP designed the electrodes and molds. FK, JD, BJ, SP, SC, SB, and AS conceived and designed the study and contributed in regular data analyzes and discussions. All authors edited, read, and approved the final manuscript.
The authors gratefully acknowledge the support for this research provided by the U.S. Department of Energy through the Bioenergy Technologies Office (BETO) and a cooperative research and development agreement among Lawrence Livermore National Laboratory, Stanford University, and the Southern California Gas Company under agreement number TC02293. Work at Lawrence Livermore National Laboratory was performed under the auspices of the U.S. Department of Energy under Contract DE-AC52-07NA27344, LLNL release number LLNL-JRNL-821387.
The authors declare that the research was conducted in the absence of any commercial or financial relationships that could be construed as a potential conflict of interest.
All claims expressed in this article are solely those of the authors and do not necessarily represent those of their affiliated organizations, or those of the publisher, the editors and the reviewers. Any product that may be evaluated in this article, or claim that may be made by its manufacturer, is not guaranteed or endorsed by the publisher.
The Supplementary Material for this article can be found online at: https://www.frontiersin.org/articles/10.3389/fmicb.2021.696473/full#supplementary-material
Supplementary Video 1 | 39 cm2 at –50 mA.
Supplementary Video 2 | 55 cm2 at –50 mA.
Supplementary Video 3 | 111 cm2 at –50 mA.
Angulo, A., van der Linde, P., Gardeniers, H., Modestino, M., and Rivas, D. F. (2020). Influence of bubbles on the energy conversion efficiency of electrochemical reactors. Joule 4, 555–579. doi: 10.1016/j.joule.2020.01.005
Aryal, N., Ammam, F., Patil, S. A., and Pant, D. (2017). An overview of cathode materials for microbial electrosynthesis of chemicals from carbon dioxide. Green Chem. 19, 5748–5760. doi: 10.1039/c7gc01801k
Asimakopoulos, K., Gavala, H. N., and Skiadas, I. V. (2018). Reactor systems for syngas fermentation processes: a review. Chem. Eng. J. 348, 732–744. doi: 10.1016/j.cej.2018.05.003
Auinger, M., Katsounaros, I., Meier, J. C., Klemm, S. O., Ulrich Biedermann, P., Topalov, A. A., et al. (2011). Near-surface ion distribution and buffer effects during electrochemical reactions. Phys. Chem. Chem. Phys. 13, 16384–16394. doi: 10.1039/c1cp21717h
Bajracharya, S., ter Heijne, A., Dominguez Benetton, X., Vanbroekhoven, K., Buisman, C. J., Strik, D. P., et al. (2015). Carbon dioxide reduction by mixed and pure cultures in microbial electrosynthesis using an assembly of graphite felt and stainless steel as a cathode. Bioresour. Technol. 195, 14–24. doi: 10.1016/j.biortech.2015.05.081
Bian, B., Wang, C., Hu, M., Yang, Z., Cai, X., Shi, D., et al. (2018). Application of 3D printed porous copper anode in microbial fuel cells. Front. Energy Res. 6:50. doi: 10.3389/fenrg.2018.00050
Blanchet, E., Duquenne, F., Rafrafi, Y., Etcheverry, L., Erable, B., and Bergel, A. (2015). Importance of the hydrogen route in up-scaling electrosynthesis for microbial CO 2 reduction. Energy Environ. Sci. 8, 3731–3744. doi: 10.1039/c5ee03088a
Bushuyev, O. S., De Luna, P., Dinh, C. T., Tao, L., Saur, G., van de Lagemaat, J., et al. (2018). What should we make with CO2 and how can we make it? Joule 2, 825–832. doi: 10.1016/j.joule.2017.09.003
Calignano, F., Tommasi, T., Manfredi, D., and Chiolerio, A. (2015). Additive manufacturing of a microbial fuel cell—a detailed study. Sci. Rep. 5, 1–10.
Chandrasekaran, S., Yao, B., Liu, T., Xiao, W., Song, Y., Qian, F., et al. (2018). Direct ink writing of organic and carbon aerogels. Mater. Horiz. 5, 1166–1175. doi: 10.1039/c8mh00603b
Chen, X., Liu, X., Childs, P., Brandon, N., and Wu, B. (2017). A low cost desktop electrochemical metal 3D printer. Adv. Mater. Technol. 2:1700148. doi: 10.1002/admt.201700148
Chung, T. H., and Dhar, B. R. (2021). A mini-review on applications of 3D printing for microbial electrochemical technologies. Front. Energy Res. 9:679061. doi: 10.3389/fenrg.2021.679061
Conrad, R., and Wetter, B. (1990). Influence of temperature on energetics of hydrogen metabolism in homoacetogenic, methanogenic, and other anaerobic bacteria. Arch. Microbiol. 155, 94–98. doi: 10.1007/bf00291281
Corral, D., Feaster, J. T., Sobhani, S., DeOtte, J. R., Lee, D. U., Wong, A. A., et al. (2021). Advanced manufacturing for electrosynthesis of fuels and chemicals from CO 2. Energy Environ. Sci. 14, 3064–3074. doi: 10.1039/d0ee03679j
Daniell, J., Nagaraju, S., Burton, F., Köpke, M., and Simpson, S. D. (2016). Low-carbon fuel and chemical production by anaerobic gas fermentation. Adv. Biochem. Eng. Biotechnol. 156, 293–321.
De Luna, P., Hahn, C., Higgins, D., Jaffer, S. A., Jaramillo, T. F., and Sargent, E. H. (2019). What would it take for renewably powered electrosynthesis to displace petrochemical processes? Science 364:eaav3506. doi: 10.1126/science.aav3506
Dessì, P., Rovira-Alsina, L., Sánchez, C., Dinesh, G. K., Tong, W., Chatterjee, P., et al. (2020). Microbial electrosynthesis: towards sustainable biorefineries for production of green chemicals from CO2 emissions. Biotechnol. Adv. 46:107675. doi: 10.1016/j.biotechadv.2020.107675
Feldewert, C., Lang, K., and Brune, A. (2020). The hydrogen threshold of obligately methyl-reducing methanogens. FEMS Microbiol. Lett. 367:fnaa137.
Freyman, M. C., Kou, T., Wang, S., and Li, Y. (2020). 3D printing of living bacteria electrode. Nano Res. 13, 1318–1323. doi: 10.1007/s12274-019-2534-1
Geppert, F., Liu, D., Weidner, E., and ter Heijne, A. (2019). Redox-flow battery design for a methane-producing bioelectrochemical system. Int. J. Hydrogen Energy 44, 21464–21469. doi: 10.1016/j.ijhydene.2019.06.189
Harnisch, F., Rosa, L. F., Kracke, F., Virdis, B., and Krömer, J. O. (2015). Electrifying white biotechnology: engineering and economic potential of electricity-driven bio-production. ChemSusChem 8, 758–766. doi: 10.1002/cssc.201402736
He, Y.-T., Fu, Q., Pang, Y., Li, Q., Li, J., Zhu, X., et al. (2021). Customizable design strategies for high-performance bioanodes in bioelectrochemical systems. Iscience 24:102163. doi: 10.1016/j.isci.2021.102163
Jayathilake, B., Plichta, E., Hendrickson, M., and Narayanan, S. (2018). Improvements to the coulombic efficiency of the iron electrode for an all-iron redox-flow battery. J. Electrochem. Soc. 165:A1630.
Jourdin, L., Sousa, J., van Stralen, N., and Strik, D. P. (2020). Techno-economic assessment of microbial electrosynthesis from CO2 and/or organics: an interdisciplinary roadmap towards future research and application. Appl. Energy 279:115775. doi: 10.1016/j.apenergy.2020.115775
Kantzow, C., Mayer, A., and Weuster-Botz, D. (2015). Continuous gas fermentation by Acetobacterium woodii in a submerged membrane reactor with full cell retention. J. Biotechnol. 212, 11–18. doi: 10.1016/j.jbiotec.2015.07.020
Kassym, K., and Perveen, A. (2020). Atomization processes of metal powders for 3D printing. Mater. Today Proc. 26, 1727–1733. doi: 10.1016/j.matpr.2020.02.364
Kotsyurbenko, O. R., Glagolev, M. V., Nozhevnikova, A. N., and Conrad, R. (2001). Competition between homoacetogenic bacteria and methanogenic archaea for hydrogen at low temperature. FEMS Microbiol. Ecol. 38, 153–159. doi: 10.1111/j.1574-6941.2001.tb00893.x
Kracke, F., Deutzmann, J. S., Gu, W., and Spormann, A. M. (2020). In situ electrochemical H2 production for efficient and stable power-to-gas electromethanogenesis. Green Chem. 22, 6194–6203. doi: 10.1039/d0gc01894e
Kracke, F., Wong, A. B., Maegaard, K., Deutzmann, J. S., Hubert, M. A., Hahn, C., et al. (2019). Robust and biocompatible catalysts for efficient hydrogen-driven microbial electrosynthesis. Commun. Chem. 2:45.
Lee, C.-Y., Taylor, A. C., Nattestad, A., Beirne, S., and Wallace, G. G. (2019). 3D printing for electrocatalytic applications. Joule 3, 1835–1849. doi: 10.1016/j.joule.2019.06.010
Liew, F. M., Köpke, M., and Simpson, S. D. (2013). “Gas fermentation for commercial biofuels production,” in Liquid, Gaseous and Solid Biofuels-Conversion Techniques, ed. Z. Fang (Rijeka: InTech), 125–173.
Parag, Y., and Sovacool, B. K. (2016). Electricity market design for the prosumer era. Nat. Energy 1, 1–6.
Phillips, J. R., Atiyeh, H. K., Lewis, R. S., and Huhnke, R. L. (2011). “Mass transfer and kinetic limitations during synthesis gas fermentation by acetogenic bacteria”, Proceedings of the American Society of Agriculturaland Biological Engineers Annual International Meeting 2011. Louisville, KY: American Society of Agricultural and Biological Engineers.
Phillips, J. R., Huhnke, R. L., and Atiyeh, H. K. (2017). Syngas fermentation: a microbial conversion process of gaseous substrates to various products. Fermentation 3:28. doi: 10.3390/fermentation3020028
Prévoteau, A., Carvajal-Arroyo, J. M., Ganigué, R., and Rabaey, K. (2020). Microbial electrosynthesis from CO2: forever a promise? Curr. Opin. Biotechnol. 62, 48–57. doi: 10.1016/j.copbio.2019.08.014
Pumera, M. (2019). Three-dimensionally printed electrochemical systems for biomedical analytical applications. Curr. Opin. Electrochem. 14, 133–137. doi: 10.1016/j.coelec.2019.02.001
Rittmann, S., Seifert, A., and Herwig, C. (2012). Quantitative analysis of media dilution rate effects on Methanothermobacter marburgensis grown in continuous culture on H2 and CO2. Biomass Bioenergy 36, 293–301. doi: 10.1016/j.biombioe.2011.10.038
Schauer, N. L., and Whitman, W. B. (1989). Formate growth and pH control by volatile formic and acetic acids in batch cultures of methanococci. J. Microbiol. Methods 10, 1–7. doi: 10.1016/0167-7012(89)90041-9
Shinagawa, T., Obata, K., and Takanabe, K. (2019). Switching of kinetically relevant reactants for the aqueous cathodic process determined by mass-transport coupled with protolysis. ChemCatChem 11, 5961–5968. doi: 10.1002/cctc.201901459
Verhallen, P., Oomen, L., Elsen, A., Kruger, J., and Fortuin, J. (1984). The diffusion coefficients of helium, hydrogen, oxygen and nitrogen in water determined from the permeability of a stagnant liquid layer in the quasi-s. Chem. Eng. Sci. 39, 1535–1541. doi: 10.1016/0009-2509(84)80082-2
Vogt, H. (1980). On the supersaturation of gas in the concentration boundary layer of gas evolving electrodes. Electrochim. Acta 25, 527–531. doi: 10.1016/0013-4686(80)87052-6
You, J., Preen, R. J., Bull, L., Greenman, J., and Ieropoulos, I. (2017). 3D printed components of microbial fuel cells: towards monolithic microbial fuel cell fabrication using additive layer manufacturing. Sustain. Energy Technol. Assess. 19, 94–101. doi: 10.1016/j.seta.2016.11.006
Zhang, T., Nie, H., Bain, T. S., Lu, H., Cui, M., Snoeyenbos-West, O. L., et al. (2013). Improved cathode materials for microbial electrosynthesis. Energy Environ. Sci. 6, 217–224. doi: 10.1039/c2ee23350a
Keywords: microbial electrosynthesis, gas fermentation, bioelectrochemical system, hydrogen mass transfer, current density, 3D-printing, additive manufacturing (3D printing)
Citation: Kracke F, Deutzmann JS, Jayathilake BS, Pang SH, Chandrasekaran S, Baker SE and Spormann AM (2021) Efficient Hydrogen Delivery for Microbial Electrosynthesis via 3D-Printed Cathodes. Front. Microbiol. 12:696473. doi: 10.3389/fmicb.2021.696473
Received: 16 April 2021; Accepted: 09 July 2021;
Published: 03 August 2021.
Edited by:
Sebastià Puig, University of Girona, SpainReviewed by:
Krishnaveni Venkidusamy, University of South Australia, AustraliaCopyright © 2021 Kracke, Deutzmann, Jayathilake, Pang, Chandrasekaran, Baker and Spormann. This is an open-access article distributed under the terms of the Creative Commons Attribution License (CC BY). The use, distribution or reproduction in other forums is permitted, provided the original author(s) and the copyright owner(s) are credited and that the original publication in this journal is cited, in accordance with accepted academic practice. No use, distribution or reproduction is permitted which does not comply with these terms.
*Correspondence: Frauke Kracke, ZnJhdWtlLmtyYWNrZUBnbWFpbC5jb20=; Alfred M. Spormann, c3Bvcm1hbm5Ac3RhbmZvcmQuZWR1
Disclaimer: All claims expressed in this article are solely those of the authors and do not necessarily represent those of their affiliated organizations, or those of the publisher, the editors and the reviewers. Any product that may be evaluated in this article or claim that may be made by its manufacturer is not guaranteed or endorsed by the publisher.
Research integrity at Frontiers
Learn more about the work of our research integrity team to safeguard the quality of each article we publish.