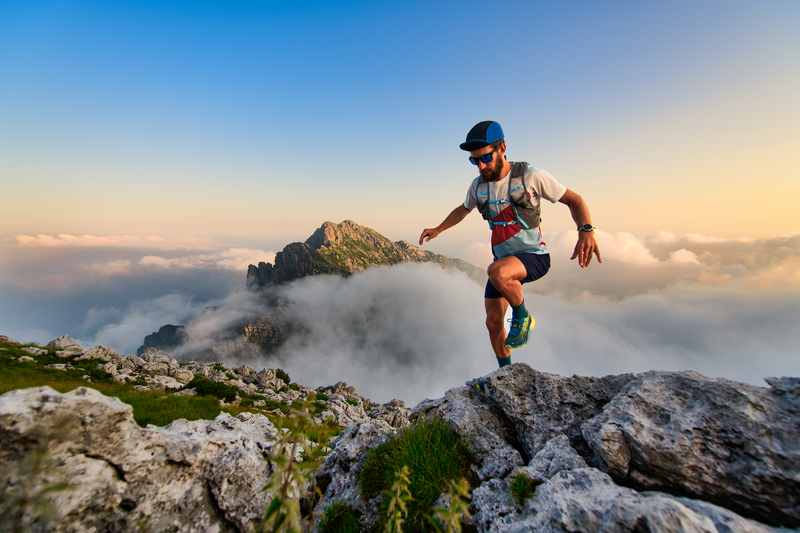
95% of researchers rate our articles as excellent or good
Learn more about the work of our research integrity team to safeguard the quality of each article we publish.
Find out more
REVIEW article
Front. Microbiol. , 01 July 2021
Sec. Microbiological Chemistry and Geomicrobiology
Volume 12 - 2021 | https://doi.org/10.3389/fmicb.2021.690918
This article is part of the Research Topic Interactions Between Microbes, Minerals, and Molecules Across Scales and Time View all 6 articles
Iron (Fe) is the fourth most abundant element in the Earth’s crust where ferrous Fe [Fe(II)] and ferric Fe [Fe(III)] can be used by archaea for energy conservation. In these archaea-Fe interactions, Fe(III) serves as terminal electron acceptor for anaerobic respiration by a variety of archaea, while Fe(II) serves as electron donor and/or energy sources for archaeal growth. As no Fe is incorporated into the archaeal cells, these redox reactions are referred to as dissimilatory Fe(III) reduction and Fe(II) oxidation, respectively. Dissimilatory Fe(III)-reducing archaea (FeRA) and Fe(II)-oxidizing archaea (FeOA) are widespread on Earth where they play crucial roles in biogeochemical cycling of not only Fe, but also carbon and sulfur. To reduce extracellular Fe(III) (oxyhydr)oxides, some FeRA transfer electrons directly to the Fe(III) (oxyhydr)oxides most likely via multiheme c-type cytochromes (c-Cyts). These multiheme c-Cyts may form the pathways similar to those found in bacteria for transferring electrons from the quinone/quinol pool in the cytoplasmic membrane to the Fe(III) (oxyhydr)oxides external to the archaeal cells. Use of multiheme c-Cyts for extracellular Fe(III) reduction by both Domains of Archaea and Bacteria emphasizes an ancient mechanism of extracellular electron transfer, which is well conserved. Other FeRA, however, reduce Fe(III) (oxyhydr)oxides indirectly via electron shuttles. Similarly, it is proposed that FeOA use pathways to oxidize Fe(II) on the surface of the cytoplasmic membrane and then to transfer the released electrons across the cytoplasmic membrane inward to the O2 and NAD+ in the cytoplasm. In this review, we focus on the latest understandings of the molecular mechanisms used by FeRA and FeOA for Fe(III) reduction and Fe(II) oxidation, respectively.
By mass, iron (Fe) is the most abundant element on Earth and the fourth most abundant element in the Earth’s crust (Morgan and Anders, 1980). In the Earth’s biosphere, Fe exists mainly as metal ion of two different oxidation states: divalent ferrous Fe [Fe(II)] and trivalent ferric Fe [Fe(III)]. At the circumneutral pH and under oxic condition, Fe(II) is quickly oxidized to Fe(III) that is readily precipitated to solid Fe(III) (oxyhydr)oxides in the absence of chelator (Emerson et al., 2010). Thus, Fe(III) (oxyhydr)oxides are abundant in a variety of environments (e.g., soils, sediments and subsurface) where they can be used by microorganisms, including archaea, as the terminal electron acceptors for anaerobic respiration (Shi et al., 2016; White et al., 2016; Jiang et al., 2019). For example, the hyperthermophilic archaeon Pyrobaculum islandicum couples H2 oxidation to reduction of Fe(III) (oxyhydr)oxides for growth (Vargas et al., 1998). As Fe is not assimilated into microbial cells, microbial Fe(III) reduction for anaerobic respiration is referred to as dissimilatory Fe(III) reduction. Notably, microbial dissimilatory Fe(III) reduction is believed to be an ancient form of respiration (Vargas et al., 1998). In addition to H2, dissimilatory Fe(III)-reducing archaea (FeRA) also couple oxidation of methane (CH4), acetate and other organic compounds to Fe(III) reduction (Tor and Lovley, 2001; Kashefi et al., 2002; Beal et al., 2009). Moreover, FeRA are found in different environmental settings, ranging from the sediment of freshwater lakes to deep sea hyperthermal vents, where they are involved in biogeochemical cycling of not only Fe, but also carbon (C) (Slobodkina et al., 2009; Yamada et al., 2014; Weber et al., 2017).
In addition to oxygen (O2), the stability of Fe(II) in solution is also pH-dependent: the more acidic, the more stable (Emerson et al., 2010). Thus, Fe(II) can be oxidized by bacteria as well as archaea under anoxic condition or at acidic pH (Hafenbradl et al., 1996; Edwards et al., 2000; Valdes et al., 2008). For example, the hyperthermophilic archaeon Ferroglobus placidus oxidizes Fe(II) under anoxic condition and at circumneutral pH (Hafenbradl et al., 1996). The extremely acidophilic archaeon Ferroplasma acidarmanus oxidizes Fe(II) at pH 0–2.5 and the Fe concentration that is as high as 111 g/L (Edwards et al., 2000). Furthermore, the acidophilic dissimilatory Fe(II)-oxidizing archaea (FeOA) are prevalent in the environments of low pH, such as acid mine drainages (AMD) and acidic hot springs, where they play crucial roles in not only AMD formation, but also biogeochemical cycling of Fe, C as well as sulfur (S) (Edwards et al., 2000; Baker and Banfield, 2003; Johnson et al., 2012; Chen et al., 2016; Huang et al., 2016). Notably, the FeOA Ferroplasma spp. are the key members of microbial consortia used in biomining of copper (Cu) and gold (Au) that associate the minerals rich in Fe and S (Dopson et al., 2004; Hawkes et al., 2006).
The electron exchanges between microorganisms and the metal ions or others that are external to microbial cells are termed as microbial extracellular electron transfer (Shi et al., 2016; White et al., 2016; Jiang et al., 2019). FeRA can reduce extracellular Fe(III) (oxyhydr)oxides either directly or indirectly. For example, the hyperthermophilic archaeon Geoglobus ahangari reduces Fe(III) (oxyhydr)oxides most likely via its cell surface-exposed multiheme c-type cytochromes (c-Cyts) (Manzella et al., 2013, 2015). All the currently available data suggest that FeOA oxidize Fe(II) on the exterior side of the cytoplasmic membrane probably via their cell surface-exposed redox proteins, such as b-type cytochromes and the Cu-containing proteins (Kozubal et al., 2011; Castelle et al., 2015).
This review focuses on our current understandings of the molecular mechanisms underlying the ability of FeRA and FeOA to exchange electrons with Fe(III) and Fe(II), respectively.
Thermophilic and hyperthermophilic archaea were among the first groups of archaea demonstrated conclusively to be capable of respiring on Fe(III) (Vargas et al., 1998; Slobodkin et al., 1999; Kashefi and Lovley, 2000; Kashefi et al., 2002, 2008). Among them, Geoglobus acetivorans and Geoglobus ahangari use only Fe(III) as the terminal electron acceptors, of which Fe(III) (oxyhydr)oxides are preferable (Kashefi et al., 2002; Slobodkina et al., 2009). Given that these thermophiles and hyperthermophiles are believed to be the most closely related to the last common ancestors of modern life, discovery of dissimilatory Fe(III) reduction by the thermophilic and hyperthermophilic archaea and bacteria provides microbiological evidences that dissimilatory Fe(III) reduction is an ancient form of microbial respiration (Liu et al., 1997; Pace, 1997; Vargas et al., 1998; Kashefi and Lovley, 2000). Consistent with these microbiological evidences are the geological evidences of abundance of H2 and Fe(III) (oxyhydr)oxides on pre-biotic Earth where microbial dissimilatory Fe(III) reduction occurred earlier than microbial reduction of sulfate (SO42–), nitrate (NO3–) and O2 for respiration (Walker, 1984, 1987; Lovley, 1991).
Methanogens are groups of strictly anaerobic archaea that are directly involved in CH4 production (Thauer et al., 2008). Fe(III) can also be reduced for respiration by the methanogens that are phylogenetically and physiologically diverse (Vargas et al., 1998; Bond and Lovley, 2002; Bodegom et al., 2004; Liu et al., 2011a; Zhang et al., 2012, 2013; Yamada et al., 2014; Sivan et al., 2016; Prakash et al., 2019). For example, Methanosarcina barkeri couples H2 oxidation to reduction of Fe(III) (oxyhydr)oxides and Fe(III)-containing clay minerals, which lower the efficiency of CH4 formation by re-directing the electron originally for CH4 generation to Fe(III) reduction (Bond and Lovley, 2002; Bodegom et al., 2004; Liu et al., 2011a). Furthermore, the acetotrophic methanogen Methanosarcina acetivorans produces CH4 from the methyl group of acetate via fermentation whose Go′ = −36 kJ/mol (Deppenmeier and Muller, 2008; Prakash et al., 2019). Addition of ferrihydrite [Fe(III) (OH)3], however, increases CH4 production by M. acetivorans (Prakash et al., 2019). This increase of CH4 production is directly linked to the ferrihydrite reduction coupled to acetate oxidation whose Go′ = −707 kJ/mol (Roden and Lovley, 1993; Prakash et al., 2019). It is believed that a novel electron bifurcation mechanism facilitates the thermodynamically less favorable acetate fermentation to CH4 with the thermodynamically more favorable respiratory reduction of ferrihydrite (Prakash et al., 2019). This may result in increase of ATP level in the cytoplasm of M. acetivorans, which enhances production of methyl and carbonyl groups from acetate for methanogenesis (Yan and Ferry, 2018; Prakash et al., 2019).
Phylogenetically related to methanogens, anaerobic methane-oxidizing archaea (ANME) can oxidize CH4 in conjunction with reduction of SO42–, NO3–, Mn(IV) and Fe(III) (oxyhydr)oxides under anoxic condition, which play important roles in controlling CH4 emission on modern Earth (Boetius et al., 2000; Beal et al., 2009; Haroon et al., 2013; Timmers et al., 2017; Liang et al., 2019). On the early Earth without O2, this Mn(IV)- and Fe(III)-dependent anaerobic methane oxidation (AMO) is estimated to be capable of oxidizing nearly all available CH4 (Beal et al., 2009). ANME-1 and Methanococcoides/ANME-3 were implicated in CH4 oxidation that was coupled to reduction of Mn(IV) and Fe(III) (oxyhydr)oxides in a sample from the marine methane-seep sediment (Beal et al., 2009). Furthermore, some ANME-2 forms syntrophic relationship with sulfate-reducing bacteria (SRB) to mediate sulfate-dependent AMO via direct interspecies electron transfer (McGlynn et al., 2015). However, soluble molecules such as anthraquinone-2,6-disulphonate (AQDS), humic acids and Fe(III)-citrate, can serve as the terminal electron acceptors to substitute the roles of SRB in AMO by these ANME-2 (Scheller et al., 2016). These results also suggest that ANME-2 may use solid phase Mn(IV) and Fe(III) (oxyhydr)oxides as the terminal electron acceptors for anaerobic respiration (Scheller et al., 2016). Indeed, the ANME “Candidatus Methanoperedens ferrireducens,” “Candidatus Methanoperedens manganicus,” and “Candidatus Methanoperedens manganireducens” can catalyze AMO coupled to reduction of Mn(IV) and/or Fe(III) (oxyhydr)oxides (Ettwig et al., 2016; Cai et al., 2018; Leu et al., 2020a). Collectively, all these results clearly demonstrate the ability of some ANME to reduce Mn(IV) as well as Fe(III) (oxyhydr)oxides for anaerobic respiration. Notably, it is suggested that the syntrophic ANME were originally the free-living FeRA that explored their extracellular electron transfer capability to establish the direct electron transfer-dependent consortia with SRB (Scheller et al., 2016).
Microorganisms may transfer electrons to extracellular Fe(III) (oxyhydr)oxides either directly or indirectly. Direct reduction requires physical contact between the redox proteins on the microbial surfaces, such as multiheme c-Cyts, and the surfaces of Fe(III) (oxyhydr)oxides (Figure 1A; Xiong et al., 2006; Meitl et al., 2009). Indirect reduction may involve electron shuttles and Fe(III) chelators that are secreted by the microorganisms. The electron shuttles are first reduced by FeRA and the reduced shuttles then ferry the electrons to the Fe(III) (oxyhydr)oxides (Figure 1B; Marsili et al., 2008; von Canstein et al., 2008). Different from the electron shuttles, the Fe(III) chelators solubilize the Fe(III) from Fe(III) (oxyhydr)oxides and then bring the chelator-Fe(III) complexes back to the FeRA for reduction (Figure 1C; Gralnick and Newman, 2007; Marsili et al., 2008; Shi et al., 2009).
Figure 1. The mechanisms for reducing Fe(III) (oxyhydr)oxides by microorganisms. (A) Direct reduction via physical contact. (B) Indirect reduction via electron shuttles. (C) Indirect reduction via Fe(III) chelators. FeRA, Fe(III)-reducing archaea.
The thermophilic FeRA G. ahangari reduces Fe(III) (oxyhydr)oxides directly (Manzella et al., 2013). Although addition of AQDS stimulates the reduction, G. ahangari itself cannot reduce the Fe(III) (oxyhydr)oxides entrapped in the alginate beads of 12-kDa pore size, which is large enough for AQDS to pass through, but not for the archaeal cells (Manzella et al., 2013). Furthermore, compared to the fresh medium, addition of the supernatants of the stationary culture grown with Fe(III) (oxyhydr)oxides does not increase the reduction rate by G. ahangari (Manzella et al., 2013). Finally, addition of artificial chelators has no impact on the reduction of entrapped Fe(III) (oxyhydr)oxides by G. ahangari (Manzella et al., 2013). Thus, G. ahangari produces no electron shuttle or chelator for reducing Fe(III) (oxyhydr)oxides (Manzella et al., 2013). Similarly, neither G. acetivorans nor F. placidus is able to reduce the Fe(III) (oxyhydr)oxides inside the alginate beads (Mardanov et al., 2015; Smith et al., 2015). Thus, all these results show direct contact between Fe(III) (oxyhydr)oxides and G. acetivorans, G. ahangari and F. placidus is required for Fe(III) reduction (Manzella et al., 2013; Mardanov et al., 2015; Smith et al., 2015).
Results from investigating bacterial extracellular electron transfer mechanisms demonstrate the importance of multiheme c-Cyts in direct reduction of Fe(III) (oxyhydr)oxides by bacteria (Shi et al., 2007, 2009, 2016; White et al., 2016; Jiang et al., 2019). Similarly, genomic sequencing results reveal that G. ahangari has 19 putative genes that encode multiheme c-Cyts and some of them are predicted to be on the archaeal cell surface (Manzella et al., 2015). Indeed, some heme-containing proteins can be mechanically sheared off from the cell surface of G. ahangari and this mechanic treatment renders the cells of G. ahangari unable to reduce Fe(III) (oxyhydr)oxides (Manzella et al., 2013). Moreover, transmission electron microscopy results show intimate association of the cells of G. ahangari with Fe(III) (oxyhydr)oxides (Manzella et al., 2013, 2015). Notably, G. ahangari possesses a putative decaheme c-Cyt of NapC/NirT family quinol dehydrogenase (GAH_01256) (Manzella et al., 2015). Investigation with the dissimilatory Fe(III)-reducing bacterium Shewanella oneidensis MR-1 reveals that tetraheme c-Cyt CymA, which is also a member of NapC/NirT family quinol dehydrogenase, is the key member of an electron transfer pathway for extracellular reduction of Fe(III) (oxyhydr)oxides. In this pathway, CymA oxidizes quinol in the cytoplasmic membrane and then transfers the released electrons to other c-Cyts that relay electrons eventually to the Fe(III) (oxyhydr)oxides on the bacterial cell surface (Shi et al., 2007, 2016; Marritt et al., 2012a,b; Mcmillan et al., 2012, 2013). Further analyses also suggest that the c-Cyts GAH_01306, GAH_00286 of GAH_01534, and GAH_01253 maybe the terminal reductases of G. ahangari for extracellular reduction of Fe(III) (Manzella et al., 2015). Although their proposed functions have not been experimentally verified, the c-Cyts GAH_01306, GAH_00286 of GAH_01534, GAH_01253 and GAH_01256 may function like those identified in S. oneidensis MR-1 to form an pathway for transferring electrons from the quinone/quinol pool in the cytoplasmic membrane, across the cell wall to the Fe(III) (oxyhydr)oxides on the cell surface of G. ahangari (Figure 2A).
Figure 2. The proposed molecular mechanisms for reducing Fe(III) by archaea. (A) The proposed electron transfer pathway for extracellular reduction of Fe(III) by the thermophilic archaeon Geoglobus ahangari. (B) The proposed electron transfer mechanisms for extracellular reduction of Fe(III) by the methanogen Methanosarcina acetivorans (Yan et al., 2018). (C) The proposed electron transfer pathway for extracellular reduction of Fe(III) by the anaerobic methane-oxidizing archaea. EX, extracellular; CM, cytoplasmic membrane; CW, cell wall; IN, intracellular; AQDS, oxidized anthraquinone-2,6-disulphonate; AQDSH2, reduced AQDS; HdrDE, heterodisulfide reductase; MmcA, the cytoplasmic membrane multiheme c-type cytochrome A; MP, oxidized methanophenazine; MPH2, reduced MP; Q, quinone; QH2, quinol. The c-type cytochromes are labeled in red; the c-type cytochromes with S-layer protein domains are labeled in blue; the S-layer proteins are labeled in yellow.
Similar to G. ahangari, G. acetivorans and F. placidus also possess numerous genes for multiheme c-Cyts (Mardanov et al., 2015; Smith et al., 2015). Some of these c-Cyts of G. acetivorans contain the binding motifs for Fe(III) (oxyhydr)oxides, which were originally identified in the decaheme c-Cyts MtrC and OmcA. MtrC and OmcA are the terminal reductases used by S. oneidensis MR-1 for extracellular reduction of Fe(III) (oxyhydr)oxides (Xiong et al., 2006; Lower et al., 2008; Johs et al., 2010; Edwards et al., 2014, 2015, 2020; Mardanov et al., 2015). Furthermore, expressions of some of the c-Cyts with binding motifs for Fe(III) (oxyhydr)oxides are up-regulated when G. acetivorans is cultured under Fe(III)-reducing conditions and some of them are on the cell surface. Thus, G. acetivorans and F. placidus may reduce Fe(III) (oxyhydr)oxides via their cell surface-exposed multiheme c-Cyts (Mardanov et al., 2015; Smith et al., 2015). Given numerous c-Cyts found in these archaea, some of them may also form the electron transfer pathway similar to the proposed one of G. ahangari for extracellular reduction of Fe(III) (oxyhydr)oxides (Figure 2A; Mardanov et al., 2015).
The methyl-coenzyme M reductase (Mcr) is believed to catalyzes the key reaction of methane activation during anaerobic methane oxidation. The mcr genes are widespread in ANME. Addition of a mcr gene from an uncultivated ANME-1 enables the methanogen M. acetivorans to couple methane oxidation to Fe(III) reduction (Soo et al., 2016). Further characterizations show that the cytoplasmic membrane multiheme c-Cyt A (MmcA), heterodisulfide reductase (HdrDE), and methanophenazine (MP) in the cytoplasmic membrane are all capable of reducing the soluble Fe(III)-citrate either directly or indirectly (Figure 2B; Yan et al., 2018). Recent results suggested that MmcA was probably the terminal reductase for ferrihydrite (Prakash et al., 2019).
Multiheme c-Cyts are also believed to be crucial in direct electron transfer from ANME to SRB as well as in electron transfer from ANME to Mn(IV) and Fe(III) (oxyhydr)oxides (McGlynn et al., 2015; Wegener et al., 2015; Skennerton et al., 2017; Cai et al., 2018; Leu et al., 2020a,b). All these ANME genomes possess numerous multiheme c-Cyt-encoding genes. Some of these putative c-Cyts also contain S-layer domain, indicating that they are cell surface-exposed. Further chemical imaging analyses indeed detect cytochromes on these archaeal cell surface (McGlynn et al., 2015). Moreover, some of these multiheme c-Cyt-encoding genes are acquired by the ANME from the Fe(III)-reducing bacteria via lateral gene transfer (Skennerton et al., 2017; Leu et al., 2020b). Although their direct involvements in extracellular reduction of Fe(III) still remain to be demonstrated, the multiheme c-Cyts of these ANME may also form the pathways similar to those found in the bacteria and that proposed in other FeRA (Figure 2A) to transfer electrons from the quinone/quinol pool in the cytoplasmic membrane, across the cell wall to the Fe(III) (oxyhydr)oxides or the bacterial cells contacted on the archaeal cell surface (Figure 2C; McGlynn et al., 2015; Shi et al., 2016; Jiang et al., 2019; Leu et al., 2020b).
The crucial roles of multiheme c-Cyts in extracellular reduction of Fe(III) (oxyhydr)oxides and other extracellular substrates by both Domains of Archaea and Bacteria emphasizes that the multiheme c-Cyts-mediated pathway is an ancient and well conserved extracellular electron transfer mechanism.
The hyperthermophilic FeRA P. islandicum reduces Fe(III) (oxyhydr)oxides and elemental sulfur [S(0)] directly as it is unable to reduce the Fe(III) (oxyhydr)oxides and S(0) inside the dialysis bags of 1.2–1.4 kDa pore size and produces no electron shuttle (Feinberg and Holden, 2006). It should be noted that P. islandicum lacks c-Cyt. Thus, P. islandicum must employ c-Cyt-independent mechanisms for direct reduction of Fe(III) (oxyhydr)oxides (Feinberg et al., 2008). Indeed, molybdopterin oxidoreductases of Pyrodictium delaneyi are suggested to transfer electrons directly to Fe(III) (oxyhydr)oxides (Kashyap and Holden, 2021).
Pyrobaculum aerophilum and Pyrobaculum arsenaticum reduce Fe(III) (oxyhydr)oxides indirectly as they can still reduce the Fe(III) (oxyhydr)oxides inside the dialysis bags of 1.2–1.4 kDa pore size that separate the archaeal cells and the (oxyhydr)oxides (Feinberg and Holden, 2006; Feinberg et al., 2008). Given that no chelated Fe(II) or Fe(III) is detected outside the bags, P. aerophilum is suggested to use electron shuttles to reduce the Fe(III) (oxyhydr)oxides inside the dialysis bags (Feinberg and Holden, 2006).
The FeRA F. placidus was originally isolated from a shallow submarine hydrothermal system at Vulcano, Italy as an Fe(II) oxidizer that could couple Fe(II) oxidation to NO3– reduction with a pH optimum of 7 (Hafenbradl et al., 1996). Notably, F. placidus can fix CO2 and reduce NO3– to N2O for chemolithoautotrophic growth when H2 is used as an electron donor (Vorholt et al., 1997). However, whether F. placidus can chemolithoautotrophically grow with Fe(II) and NO3– still needs to be experimentally tested.
Nearly all FeOA isolated so far are the acidophiles. They are abundant in the acidic and extremely acidic environments rich in Fe and S, such as acidic hot springs, AMD sites and bioleaching plants. These acidophilic FeOA are the key players in regulating biogeochemical cycling of C, Fe, and S, in AMD formation as well as in biomining of Cu, Au and other metals associated with the minerals that contain Fe and S (Baker and Banfield, 2003; Chen et al., 2016; Golyshina et al., 2016; Huang et al., 2016; Quatrini and Johnson, 2018). For example, Metallosphaera yellowstonensis was isolated from the Fe(III) oxide microbial mats in the acidic geothermal springs in Yellowstone National Park. It grows autotrophically with pyrite (FeS2) and Fe(II) sorbed on ferrihydrite at the optimal pH 2–3 and temperature 65–75°C (Kozubal et al., 2008). Notably, the Fe(III) oxide microbial mats also contain other microorganisms, such as Marsarchaeota and Geoarchaeota that reside under microaerobic condition and beneath the more oxic zone where M. yellowstonensis is abundant (Kozubal et al., 2012, 2013; Bernstein et al., 2013; Jay et al., 2018). Furthermore, the Fe(III)-reducing archaea are also found in these microbial mats (Kozubal et al., 2012). Thus, an active archaea-mediated Fe(II)/Fe(III) cycling is believed to occur in the mats (Kozubal et al., 2012). Moreover, Metallosphaera sedula was isolated from an acidic drain of a hot spring near Naples, Italy. It can grow chemolithoautotrophically on FeS2, sphalerite [(Zn,Fe)S], chalcopyrite (CuFeS2) and S(0) at pH 1–4.5 and temperature 50–80°C (Huber et al., 1989). Other Metallosphaera spp. that are isolated from the acidic hot springs of different locations can also grow by oxidizing Fe(II) and other metal ions (Kozubal et al., 2008, 2011; Liu et al., 2011b,c; Wheaton et al., 2019). Notably, Metallosphaera spp. belong to the order of Sulfolobales, among which Sulfolobus metallicus, Sulfolobus tokodaii, and Acidianus brierleyi (formerly known as Sulfolobus brierleyi) are all isolated from acidic hot springs and are all able to oxidize Fe(II) for growth (Brierley and Brierley, 1973; Segerer et al., 1986; Huber and Stetter, 1991; Suzuki et al., 2002; Bathe and Norris, 2007).
In addition to AMD sites, Ferroplasma spp. is also found in the bioleaching pilot plants globally. For example, F. acidiphilum was isolated from the bioreactor of a pilot plant at Tula, Russia for bioleaching the arsenopyrite (FeAsS) and FeS2 ores that contain Au. F. acidiphilum is an aerobe that oxidizes Fe(II), including FeS2, with an optimum pH of 1.7 for growth (Golyshina et al., 2000). Originally identified as F. cupricumulans, Acidiplasma cupricumulans was isolated from an industrial-scale CuFeS2 bioleach heap in Myanmar and it grew on Fe(II) at optimum pH 1–1.2 (Hawkes et al., 2006; Golyshina et al., 2009). It should be noted that all the tested Ferroplasma and Acidiplasma spp. are facultative anaerobes that can couple oxidation of yeast extract to Fe(III) reduction, which demonstrate that these Ferroplasma and Acidiplasma spp. are both FeOA and FeRA, depending on the ambient environmental conditions (Dopson et al., 2004; Zhou et al., 2008; Golyshina et al., 2009).
Fe(II) oxidation is a bioenergetic challenge for microorganisms, especially for autotrophic microorganisms. At pH 2, the redox potentials for Fe(II)/Fe(III) and O2/H2O couples are +0.77 V and +1.2 V, respectively. Thus, little energy can be derived from Fe(II) oxidation coupled to O2 reduction (Bird et al., 2011). Furthermore, the redox potential for NAD+/NADH couple is −0.32 V at pH 6.5, which is the pH found in the microbial cytoplasm. Thus, without any energy input, Fe(II) oxidation coupled to NAD+ reduction is thermodynamically unfeasible (Bonnefoy and Holmes, 2012). To overcome this thermodynamic barrier, the acidophilic FeOA use the energy from proton motive force to drive the electron transfer from Fe(II) oxidation to NAD+ reduction (i.e., uphill electron transfer). Although energy generation is limited, the electron transfer from Fe(II) oxidation to O2 reduction is thermodynamically feasible (i.e., downhill electron transfer) and thus can be catalyzed by microorganisms (Figure 3A; Bird et al., 2011; Bonnefoy and Holmes, 2012; Ilbert and Bonnefoy, 2013; Nitschke and Bonnefoy, 2016; Jiang et al., 2019).
Figure 3. The proposed molecular mechanisms for oxidizing Fe(II) by acidophilic archaea. (A) Standard redox potentials (E0′) of NAD+/NADH couple at pH 6.5, Fe(II)/Fe(III) and O2/H2O couples at pH 2. Modified with permission from Bonnefoy and Holmes (2012)© (2012) Society for Applied Microbiology and John Wiley and Sons Ltd. (B) The proposed electron transfer pathway for oxidation of Fe(II) by Metallosphaera yellowstonensis. MCO, multicopper oxidase. Modified with permission from Kozubal et al. (2011)© (2011) American Society for Microbiology. (C) The proposed electron transfer pathway for oxidation of Fe(II) by Ferroplasma acidiphilum. Q, quinone; QH2, quinol. Modified with permission from Castelle et al. (2015)© (2015) Elsevier.
Sequencing results revealed that the genome of M. yellowstonensis contained a gene cluster that was also found in other FeOA, such as M. sedula and S. metallicus, in which this gene cluster was implicated in Fe(II) oxidation (Bathe and Norris, 2007; Auernik and Kelly, 2008; Kozubal et al., 2008). In this gene cluster, two of its protein products FoxCD are proposed to oxidize Fe(II) on the surface of the cytoplasmic membrane of M. yellowstonensis and transfer the released electrons in the cytoplasmic membrane to either O2 via the proposed downhill pathway or NAD+ via the proposed uphill pathway in the cytoplasmic membrane (Kozubal et al., 2011). The downhill electron transfer pathway may include multicopper oxidase (MCO) and terminal heme-copper oxidase FoxAB. The uphill electron transfer pathway may include MCO, Cytochrome bc complex, quinone/quinol pool and NADH dehydrogenase (Figure 3B; Kozubal et al., 2011). Given that they also have the fox gene cluster, the acidophilic FeOA Acidianus copahuensis, M. sedula, and S. metallicus are also suggested to oxidize Fe(II) via the mechanism similar to that of M. yellowstonensis (Bathe and Norris, 2007; Auernik and Kelly, 2008; Auernik et al., 2008; Bonnefoy and Holmes, 2012; Ilbert and Bonnefoy, 2013; Nitschke and Bonnefoy, 2016; Urbieta et al., 2017).
Sequencing results reveal no fox homolog in the genomes of Ferroplasma spp. (Tyson et al., 2004; Allen et al., 2007; Golyshina et al., 2017). Protein purification and characterization demonstrate that the blue copper protein sulfocyanin of F. acidiphilum, which is suggested to be on the exterior side of cytoplasmic membrane, oxidizes Fe(II) directly (Castelle et al., 2015). Sulfocyanin is part of an 850 kDa protein complex that also contains the aa3-type cytochrome oxidase. Thus, this cytoplasmic membrane protein complex is proposed to couple Fe(II) oxidation to O2 reduction (Figure 3C; Castelle et al., 2015). A 150 kDa protein complex is also isolated from the membrane fraction. This complex is believed to be the Rieske–cytochrome b-type complex that is suggested to mediate electron transfer from sulfocyanin to quinone in the cytoplasmic membrane (Figure 3C). The similar Fe(II) oxidation mechanism may also exist in other Ferroplasma spp. (Castelle et al., 2015). Thus, F. acidiphilum and most likely other Ferroplasma spp. oxidize Fe(II) via a mechanism different from that of M. yellowstonensis. Given that they are also different from those of bacteria (Bird et al., 2011; Bonnefoy and Holmes, 2012; Shi et al., 2012, 2016; Ilbert and Bonnefoy, 2013; Jiang et al., 2019), the molecular mechanisms for Fe(II) oxidation by FeOA must have evolved independently.
The phylogenetically and physiologically diverse groups of FeRA and FeOA have been isolated from a variety of ecosystems worldwide. They are directly involved in biogeochemical cycling of Fe, Mn, C, and S. Furthermore, FeRA reduce Fe(III) (oxyhydr)oxides either directly or indirectly and multiheme c-Cyts are believed to be involved in the direct reduction of extracellular Fe(III) (oxyhydr)oxides most likely by forming the extracellular electron transfer pathways. Finally, FeOA are proposed to oxidize Fe(II) on the surface of the cytoplasmic membrane via the b-type cytochromes and Cu-containing proteins that maybe the part of the electron transfer pathways.
In bacteria, the extracellular electron transfer pathways connect extracellular redox transformation of Fe with intracellular metabolic activities. These pathways consist of redox and structural proteins as well as redox molecules (Table 1) [for reviews, see Bird et al. (2011), Bonnefoy and Holmes (2012), Ilbert and Bonnefoy (2013), Shi et al. (2016), White et al. (2016), Jiang et al. (2019)]. Some of these pathways are rigorously characterized (Shi et al., 2016; White et al., 2016; Jiang et al., 2019). In addition to the extracellular electron transfer pathways, extracellular extensions, such as Geobacter conductive pili (G-pili) and multiheme c-Cyt-based nanowires, have also been identified and characterized for transferring electrons to the Fe(III) (oxyhydr)oxides distant from bacterial surfaces (Table 1; Filman et al., 2019; Lovley and Walker, 2019; Reguera and Kashefi, 2019; Wang et al., 2019; Yalcin et al., 2020).
Table 1. Comparison of different types of proteins and redox molecules that are or are suggested to be involved in Fe(III) reduction or Fe(II) oxidation by bacteria and archaea.
Similar to those found in bacteria, multiheme c-Cyts and quinone/quinol pool are suggested to be the key components of the electron transfer pathways involved in extracellular reduction of Fe(III) by archaea. Likewise, Cu-containing proteins, NADH dehydrogenases, aa3 oxidase, bc1 complex, and quinone/quinol pool are implicated as the key components of the electron transfer pathways for Fe(II) oxidation by both bacteria and archaea (Table 1). Thus, archaea and bacteria may share these redox proteins and molecules for Fe(III) reduction and/or Fe(II) oxidation. However, identification of the redox proteins and molecules unique for archaeal Fe(III) reduction, such as molybdopterin oxidoreductases, HdrDE and MP, and those unique for bacterial Fe(III) reduction (e.g., flavins, flavin-binding proteins, G-pili and c-Cyt-based nanowires) demonstrate the diverse mechanisms that have evolved independently among different groups of archaea and bacteria for exchanging electrons with Fe(III) and Fe(II) (Table 1).
Compared to those in bacteria, the molecular mechanisms for reduction of Fe(III) (oxyhydr)oxides and oxidation of Fe(II) by archaea have been much less characterized and remain speculative, which is mainly attributed to the lack of genetically tractable model FeRA and FeOA. Thus, development of the representative model systems is crucial for further understanding these electron transfer mechanisms at molecular level. Currently, M. acetivorans and M. barkeri are the two genetically tractable methanogens for investigating molecular mechanisms for Fe(III) reduction. Although it is demonstrated to reduce soluble Fe(III), whether MmcA of M. acetivorans can reduce Fe(III) (oxyhydr)oxides remains uncharacterized. Furthermore, a novel c-Cyt-independent extracellular electron transfer mechanism has recently been identified and characterized in Gram-positive bacteria (Light et al., 2018, 2019). Whether the similar mechanism also exists in archaea remains uninvestigated. Thus, future research should focus on bridging these knowledge gaps.
Identification and characterization of archaeal electron transfer mechanisms at molecular level will not only substantially advance our understanding of how microorganisms exchange electrons with Fe(III) and Fe(II), but also lay solid foundations for further developments of FeRA- and FeOA-based biotechnology. For example, molecular understanding of electron transfer mechanisms from archaeal cell surface to their cytoplasmic membranes will certainly help develop electromethanogenesis via the synthetic biology approach (Cheng et al., 2009).
All authors listed have made a substantial, direct and intellectual contribution to the work, and approved it for publication.
This work was supported by the National Key Research and Development Program of China (2018YFA0901303) and the National Natural Science Foundation of China (NSFC91851211 and 41772363).
The authors declare that the research was conducted in the absence of any commercial or financial relationships that could be construed as a potential conflict of interest.
Allen, E. E., Tyson, G. W., Whitaker, R. J., Detter, J. C., Richardson, P. M., and Banfield, J. F. (2007). Genome dynamics in a natural archaeal population. Proc. Natl. Acad. Sci. U. S. A. 104, 1883–1888. doi: 10.1073/pnas.0604851104
Auernik, K. S., and Kelly, R. M. (2008). Identification of components of electron transport chains in the extremely thermoacidophilic crenarchaeon Metallosphaera sedula through iron and sulfur compound oxidation transcriptomes. Appl. Environ. Microbiol. 74, 7723–7732. doi: 10.1128/aem.01545-08
Auernik, K. S., Maezato, Y., Blum, P. H., and Kelly, R. M. (2008). The genome sequence of the metal-mobilizing, extremely thermoacidophilic archaeon Metallosphaera sedula provides insights into bioleaching-associated metabolism. Appl. Environ. Microbiol. 74, 682–692. doi: 10.1128/aem.02019-07
Baker, B. J., and Banfield, J. F. (2003). Microbial communities in acid mine drainage. FEMS Microbiol. Ecol. 44, 139–152. doi: 10.1016/s0168-6496(03)00028-x
Bathe, S., and Norris, P. R. (2007). Ferrous iron- and sulfur-induced genes in Sulfolobus metallicus. Appl. Environ. Microbiol. 73, 2491–2497. doi: 10.1128/aem.02589-06
Beal, E. J., House, C. H., and Orphan, V. J. (2009). Manganese- and iron-dependent marine methane oxidation. Science 325, 184–187. doi: 10.1126/science.1169984
Bernstein, H. C., Beam, J. P., Kozubal, M. A., Carlson, R. P., and Inskeep, W. P. (2013). In situ analysis of oxygen consumption and diffusive transport in high-temperature acidic iron-oxide microbial mats. Environ. Microbiol. 15, 2360–2370. doi: 10.1111/1462-2920.12109
Bird, L. J., Bonnefoy, V., and Newman, D. K. (2011). Bioenergetic challenges of microbial iron metabolisms. Trends Microbiol. 19, 330–340. doi: 10.1016/j.tim.2011.05.001
Bodegom, P. M., Scholten, J. C., and Stams, A. J. (2004). Direct inhibition of methanogenesis by ferric iron. FEMS Microbiol. Ecol. 49, 261–268. doi: 10.1016/j.femsec.2004.03.017
Boetius, A., Ravenschlag, K., Schubert, C. J., Rickert, D., Widdel, F., Gieseke, A., et al. (2000). A marine microbial consortium apparently mediating anaerobic oxidation of methane. Nature 407, 623–626. doi: 10.1038/35036572
Bond, D. R., and Lovley, D. R. (2002). Reduction of Fe(III) oxide by methanogens in the presence and absence of extracellular quinones. Environ. Microbiol. 4, 115–124. doi: 10.1046/j.1462-2920.2002.00279.x
Bonnefoy, V., and Holmes, D. S. (2012). Genomic insights into microbial iron oxidation and iron uptake strategies in extremely acidic environments. Environ. Microbiol. 14, 1597–1611. doi: 10.1111/j.1462-2920.2011.02626.x
Brierley, C. L., and Brierley, J. A. (1973). A chemoautotrophic and thermophilic microorganism isolated from an acid hot spring. Can. J. Microbiol. 19, 183–188. doi: 10.1139/m73-028
Cai, C., Leu, A. O., Xie, G. J., Guo, J., Feng, Y., Zhao, J. X., et al. (2018). A methanotrophic archaeon couples anaerobic oxidation of methane to Fe(III) reduction. ISME J. 12, 1929–1939. doi: 10.1038/s41396-018-0109-x
Castelle, C. J., Roger, M., Bauzan, M., Brugna, M., Lignon, S., Nimtz, M., et al. (2015). The aerobic respiratory chain of the acidophilic archaeon Ferroplasma acidiphilum: A membrane-bound complex oxidizing ferrous iron. Biochim. Biophys. Acta 1847, 717–728. doi: 10.1016/j.bbabio.2015.04.006
Chen, L. X., Huang, L. N., Mendez-Garcia, C., Kuang, J. L., Hua, Z. S., Liu, J., et al. (2016). Microbial communities, processes and functions in acid mine drainage ecosystems. Curr. Opin. Biotechnol. 38, 150–158. doi: 10.1016/j.copbio.2016.01.013
Cheng, S., Xing, D., Call, D. F., and Logan, B. E. (2009). Direct biological conversion of electrical current into methane by electromethanogenesis. Environ. Sci. Technol. 43, 3953–3958. doi: 10.1021/es803531g
Deppenmeier, U., and Muller, V. (2008). Life close to the thermodynamic limit: how methanogenic archaea conserve energy. Results Probl. Cell Differ. 45, 123–152. doi: 10.1007/400_2006_026
Dopson, M., Baker-Austin, C., Hind, A., Bowman, J. P., and Bond, P. L. (2004). Characterization of Ferroplasma isolates and Ferroplasma acidarmanus sp. nov., extreme acidophiles from acid mine drainage and industrial bioleaching environments. Appl. Environ. Microbiol. 70, 2079–2088. doi: 10.1128/aem.70.4.2079-2088.2004
Edwards, K. J., Bond, P. L., Gihring, T. M., and Banfield, J. F. (2000). An archaeal iron-oxidizing extreme acidophile important in acid mine drainage. Science 287, 1796–1799. doi: 10.1126/science.287.5459.1796
Edwards, M. J., Baiden, N. A., Johs, A., Tomanicek, S. J., Liang, L., Shi, L., et al. (2014). The X-ray crystal structure of Shewanella oneidensis OmcA reveals new insight at the microbe-mineral interface. FEBS Lett. 588, 1886–1890. doi: 10.1016/j.febslet.2014.04.013
Edwards, M. J., White, G. F., Butt, J. N., Richardson, D. J., and Clarke, T. A. (2020). The crystal structure of a biological insulated transmembrane molecular wire. Cell 181, 665–673e610.
Edwards, M. J., White, G. F., Norman, M., Tome-Fernandez, A., Ainsworth, E., Shi, L., et al. (2015). Redox linked flavin sites in extracellular decaheme proteins involved in microbe-mineral electron transfer. Sci. Rep. 5:11677.
Emerson, D., Fleming, E. J., and Mcbeth, J. M. (2010). Iron-oxidizing bacteria: an environmental and genomic perspective. Annu. Rev. Microbiol. 64, 561–583. doi: 10.1146/annurev.micro.112408.134208
Ettwig, K. F., Zhu, B., Speth, D., Keltjens, J. T., Jetten, M. S. M., and Kartal, B. (2016). Archaea catalyze iron-dependent anaerobic oxidation of methane. Proc. Natl. Acad. Sci. U. S. A. 113, 12792–12796. doi: 10.1073/pnas.1609534113
Feinberg, L. F., and Holden, J. F. (2006). Characterization of dissimilatory Fe(III) versus NO3- reduction in the hyperthermophilic archaeon Pyrobaculum aerophilum. J. Bacteriol. 188, 525–531. doi: 10.1128/jb.188.2.525-531.2006
Feinberg, L. F., Srikanth, R., Vachet, R. W., and Holden, J. F. (2008). Constraints on anaerobic respiration in the hyperthermophilic archaea Pyrobaculum islandicum and Pyrobaculum aerophilum. Appl. Environ. Microbiol. 74, 396–402. doi: 10.1128/aem.02033-07
Filman, D. J., Marino, S. F., Ward, J. E., Yang, L., Mester, Z., Bullitt, E., et al. (2019). Cryo-EM reveals the structural basis of long-range electron transport in a cytochrome-based bacterial nanowire. Commun. Biol. 2:219.
Golyshina, O. V., Ferrer, M., and Golyshin, P. N. (2016). “Diversity and physiologies of acidophilic archaea,” in Acidophiles: Life in Extremely Acidic Environments, eds R. Quatrini and D. B. Johnson (Norfolk, UK: Caister Academic Press), 93–106. doi: 10.21775/9781910190333.06
Golyshina, O. V., Pivovarova, T. A., Karavaiko, G. I., Kondrateva, T. F., Moore, E. R., Abraham, W. R., et al. (2000). Ferroplasma acidiphilum gen. nov., sp. nov., an acidophilic, autotrophic, ferrous-iron-oxidizing, cell-wall-lacking, mesophilic member of the Ferroplasmaceae fam. nov., comprising a distinct lineage of the Archaea. Int. J. Syst. Evol. Microbiol. 50, 997–1006. doi: 10.1099/00207713-50-3-997
Golyshina, O. V., Tran, H., Reva, O. N., Lemak, S., Yakunin, A. F., Goesmann, A., et al. (2017). Metabolic and evolutionary patterns in the extremely acidophilic archaeon Ferroplasma acidiphilum Y(T). Sci. Rep. 7:3682.
Golyshina, O. V., Yakimov, M. M., Lunsdorf, H., Ferrer, M., Nimtz, M., Timmis, K. N., et al. (2009). Acidiplasma aeolicum gen. nov., sp. nov., a euryarchaeon of the family Ferroplasmaceae isolated from a hydrothermal pool, and transfer of Ferroplasma cupricumulans to Acidiplasma cupricumulans comb. nov. Int. J. Syst. Evol. Microbiol. 59, 2815–2823. doi: 10.1099/ijs.0.009639-0
Gralnick, J. A., and Newman, D. K. (2007). Extracellular respiration. Mol. Microbiol. 65, 1–11. doi: 10.1111/j.1365-2958.2007.05778.x
Hafenbradl, D., Keller, M., Dirmeier, R., Rachel, R., Rossnagel, P., Burggraf, S., et al. (1996). Ferroglobus placidus gen. nov., sp. nov., A novel hyperthermophilic archaeum that oxidizes Fe2 + at neutral pH under anoxic conditions. Arch. Microbiol. 166, 308–314. doi: 10.1007/s002030050388
Haroon, M. F., Hu, S., Shi, Y., Imelfort, M., Keller, J., Hugenholtz, P., et al. (2013). Anaerobic oxidation of methane coupled to nitrate reduction in a novel archaeal lineage. Nature 500, 567–570. doi: 10.1038/nature12375
Hawkes, R. B., Franzmann, P. D., O’hara, G., and Plumb, J. J. (2006). Ferroplasma cupricumulans sp. nov., a novel moderately thermophilic, acidophilic archaeon isolated from an industrial-scale chalcocite bioleach heap. Extremophiles 10, 525–530. doi: 10.1007/s00792-006-0527-y
Huang, L. N., Kuang, J. L., and Shu, W. S. (2016). Microbial ecology and evolution in the acid mine drainage model system. Trends Microbiol. 24, 581–593. doi: 10.1016/j.tim.2016.03.004
Huber, G., Spinnler, C., Gambacorta, A., and Stetter, K. O. (1989). Metallospaera sedula gen. and sp. nov. resprents a new genus of aerobic, metal-mobilizing, thermoacidophilic archaebacteria. System. Appl. Microbial. 12, 38–47. doi: 10.1016/s0723-2020(89)80038-4
Huber, G., and Stetter, K. O. (1991). Sulfolobus metallicus, sp. nov., a novela strictly chemolithoautotrophic thermophilic archaeal species of metal-mobilizers. System. Appl. Microbial. 14, 372–378. doi: 10.1016/s0723-2020(11)80312-7
Ilbert, M., and Bonnefoy, V. (2013). Insight into the evolution of the iron oxidation pathways. Biochim. Biophys. Acta 1827, 161–175. doi: 10.1016/j.bbabio.2012.10.001
Jay, Z. J., Beam, J. P., Dlakic, M., Rusch, D. B., Kozubal, M. A., and Inskeep, W. P. (2018). Marsarchaeota are an aerobic archaeal lineage abundant in geothermal iron oxide microbial mats. Nat. Microbiol. 3, 732–740. doi: 10.1038/s41564-018-0163-1
Jiang, Y., Shi, M., and Shi, L. (2019). Molecular underpinnings for microbial extracellular electron transfer during biogeochemical cycling of earth elements. Sci. China Life Sci. 62, 1275–1286. doi: 10.1007/s11427-018-9464-3
Johnson, D. B., Kanao, T., and Hedrich, S. (2012). Redox transformations of iron at extremely low pH: fundamental and applied aspects. Front. Microbiol. 3:96. doi: 10.3389/fmicb.2012.00096
Johs, A., Shi, L., Droubay, T., Ankner, J. F., and Liang, L. (2010). Characterization of the decaheme c-type cytochrome OmcA in solution and on hematite surfaces by small angle x-ray scattering and neutron reflectometry. Biophys. J. 98, 3035–3043. doi: 10.1016/j.bpj.2010.03.049
Kashefi, K., and Lovley, D. R. (2000). Reduction of Fe(III), Mn(IV), and toxic metals at 100 degrees C by Pyrobaculum islandicum. Appl. Environ. Microbiol. 66, 1050–1056. doi: 10.1128/aem.66.3.1050-1056.2000
Kashefi, K., Moskowitz, B. M., and Lovley, D. R. (2008). Characterization of extracellular minerals produced during dissimilatory Fe(III) and U(VI) reduction at 100 degrees C by Pyrobaculum islandicum. Geobiology 6, 147–154. doi: 10.1111/j.1472-4669.2007.00142.x
Kashefi, K., Tor, J. M., Holmes, D. E., Gaw Van Praagh, C. V., Reysenbach, A. L., et al. (2002). Geoglobus ahangari gen. nov., sp. nov., a novel hyperthermophilic archaeon capable of oxidizing organic acids and growing autotrophically on hydrogen with Fe(III) serving as the sole electron acceptor. Int. J. Syst. Evol. Microbiol. 52, 719–728. doi: 10.1099/00207713-52-3-719
Kashyap, S., and Holden, J. F. (2021). Microbe-mineral interaction and novel proteins for iron oxide mineral reduction in the hyperthermophilic crenarchaeon Pyrodictium delaneyi. Appl. Environ. Microbiol. 87:e2330.
Kozubal, M., Macur, R. E., Korf, S., Taylor, W. P., Ackerman, G. G., Nagy, A., et al. (2008). Isolation and distribution of a novel iron-oxidizing crenarchaeon from acidic geothermal springs in Yellowstone National Park. Appl. Environ. Microbiol. 74, 942–949. doi: 10.1128/aem.01200-07
Kozubal, M. A., Dlakic, M., Macur, R. E., and Inskeep, W. P. (2011). Terminal oxidase diversity and function in “Metallosphaera yellowstonensis”: gene expression and protein modeling suggest mechanisms of Fe(II) oxidation in the sulfolobales. Appl. Environ. Microbiol. 77, 1844–1853. doi: 10.1128/aem.01646-10
Kozubal, M. A., Macur, R. E., Jay, Z. J., Beam, J. P., Malfatti, S. A., Tringe, S. G., et al. (2012). Microbial iron cycling in acidic geothermal springs of yellowstone national park: integrating molecular surveys, geochemical processes, and isolation of novel Fe-active microorganisms. Front. Microbiol. 3:109. doi: 10.3389/fmicb.2012.00109
Kozubal, M. A., Romine, M., Jennings, R., Jay, Z. J., Tringe, S. G., Rusch, D. B., et al. (2013). Geoarchaeota: a new candidate phylum in the Archaea from high-temperature acidic iron mats in Yellowstone National Park. ISME J. 7, 622–634. doi: 10.1038/ismej.2012.132
Leu, A. O., Cai, C., Mcilroy, S. J., Southam, G., Orphan, V. J., Yuan, Z., et al. (2020a). Anaerobic methane oxidation coupled to manganese reduction by members of the Methanoperedenaceae. ISME J. 14, 1030–1041. doi: 10.1038/s41396-020-0590-x
Leu, A. O., Mcilroy, S. J., Ye, J., Parks, D. H., Orphan, V. J., and Tyson, G. W. (2020b). Lateral gene transfer drives metabolic flexibility in the anaerobic methane-oxidizing archaeal family Methanoperedenaceae. mBio 11, e1325–e1320.
Liang, L., Wang, Y., Sivan, O., and Wang, F. (2019). Metal-dependent anaerobic methane oxidation in marine sediment: Insights from marine settings and other systems. Sci. China Life Sci. 62, 1287–1295. doi: 10.1007/s11427-018-9554-5
Light, S. H., Meheust, R., Ferrell, J. L., Cho, J., Deng, D., Agostoni, M., et al. (2019). Extracellular electron transfer powers flavinylated extracellular reductases in Gram-positive bacteria. Proc. Natl. Acad. Sci. U. S. A. 116, 26892–26899. doi: 10.1073/pnas.1915678116
Light, S. H., Su, L., Rivera-Lugo, R., Cornejo, J. A., Louie, A., Iavarone, A. T., et al. (2018). A flavin-based extracellular electron transfer mechanism in diverse Gram-positive bacteria. Nature 562, 140–144. doi: 10.1038/s41586-018-0498-z
Liu, D., Dong, H., Bishop, M. E., Wang, H., Agrawal, A., Tritschler, S., et al. (2011a). Reduction of structural Fe(III) in nontronite bt methanogen Methanosarcina barkeri. Geochim. Cosmochim. Acta 75, 1057–1071. doi: 10.1016/j.gca.2010.11.009
Liu, L. J., You, X. Y., Guo, X., Liu, S. J., and Jiang, C. Y. (2011b). Metallosphaera cuprina sp. nov., an acidothermophilic, metal-mobilizing archaeon. Int. J. Syst. Evol. Microbiol. 61, 2395–2400. doi: 10.1099/ijs.0.026591-0
Liu, L. J., You, X. Y., Zheng, H., Wang, S., Jiang, C. Y., and Liu, S. J. (2011c). Complete genome sequence of Metallosphaera cuprina, a metal sulfide-oxidizing archaeon from a hot spring. J. Bacteriol. 193, 3387–3388. doi: 10.1128/jb.05038-11
Liu, S. V., Zhou, J., Zhang, C., Cole, D. R., Gajdarziska-Josifovska, M., and Phelps, T. J. (1997). Thermophilic Fe(III)-reducing bacteria from the deep subsurface: the evolution implication. Science 277, 1106–1109. doi: 10.1126/science.277.5329.1106
Lovley, D. R. (1991). Dissimilatory Fe(III) and Mn(IV) reduction. Microbiol. Rev. 55, 259–287. doi: 10.1128/mmbr.55.2.259-287.1991
Lovley, D. R. (2017a). Happy together: microbial communities that hook up to swap electrons. ISME J. 11, 327–336. doi: 10.1038/ismej.2016.136
Lovley, D. R. (2017b). Syntrophy goes electric: direct interspecies electron transfer. Annu. Rev. Microbiol. 71, 643–664. doi: 10.1146/annurev-micro-030117-020420
Lovley, D. R., and Walker, D. J. F. (2019). Geobacter protein nanowires. Front. Microbiol. 10:2078. doi: 10.3389/fmicb.2019.02078
Lower, B. H., Lins, R. D., Oestreicher, Z., Straatsma, T. P., Hochella, M. F. Jr., Shi, L., et al. (2008). In vitro evolution of a peptide with a hematite binding motif that may constitute a natural metal-oxide binding archetype. Environ. Sci. Technol. 42, 3821–3827. doi: 10.1021/es702688c
Manzella, M. P., Holmes, D. E., Rocheleau, J. M., Chung, A., Reguera, G., and Kashefi, K. (2015). The complete genome sequence and emendation of the hyperthermophilic, obligate iron-reducing archaeon “Geoglobus ahangari” strain 234(T). Stand. Genomic Sci. 10:77.
Manzella, M. P., Reguera, G., and Kashefi, K. (2013). Extracellular electron transfer to Fe(III) oxides by the hyperthermophilic archaeon Geoglobus ahangari via a direct contact mechanism. Appl. Environ. Microbiol. 79, 4694–4700. doi: 10.1128/aem.01566-13
Mardanov, A. V., Slododkina, G. B., Slobodkin, A. I., Beletsky, A. V., Gavrilov, S. N., Kublanov, I. V., et al. (2015). The Geoglobus acetivorans genome: Fe(III) reduction, acetate utilization, autotrophic growth, and degradation of aromatic compounds in a hyperthermophilic archaeon. Appl. Environ. Microbiol. 81, 1003–1012. doi: 10.1128/aem.02705-14
Marritt, S. J., Lowe, T. G., Bye, J., Mcmillan, D. G., Shi, L., Fredrickson, J., et al. (2012a). A functional description of CymA, an electron-transfer hub supporting anaerobic respiratory flexibility in Shewanella. Biochem. J. 444, 465–474. doi: 10.1042/bj20120197
Marritt, S. J., Mcmillan, D. G., Shi, L., Fredrickson, J. K., Zachara, J. M., Richardson, D. J., et al. (2012b). The roles of CymA in support of the respiratory flexibility of Shewanella oneidensis MR-1. Biochem. Soc. Trans. 40, 1217–1221. doi: 10.1042/bst20120150
Marsili, E., Baron, D. B., Shikhare, I. D., Coursolle, D., Gralnick, J. A., and Bond, D. R. (2008). Shewanella secretes flavins that mediate extracellular electron transfer. Proc. Natl. Acad. Sci. U. S. A. 105, 3968–3973. doi: 10.1073/pnas.0710525105
McGlynn, S. E., Chadwick, G. L., Kempes, C. P., and Orphan, V. J. (2015). Single cell activity reveals direct electron transfer in methanotrophic consortia. Nature 526, 531–535. doi: 10.1038/nature15512
Mcmillan, D. G., Marritt, S. J., Butt, J. N., and Jeuken, L. J. (2012). Menaquinone-7 is specific cofactor in tetraheme quinol dehydrogenase CymA. J. Biol. Chem. 287, 14215–14225. doi: 10.1074/jbc.m112.348813
Mcmillan, D. G., Marritt, S. J., Firer-Sherwood, M. A., Shi, L., Richardson, D. J., Evans, S. D., et al. (2013). Protein-protein interaction regulates the direction of catalysis and electron transfer in a redox enzyme complex. J. Am. Chem. Soc. 135, 10550–10556. doi: 10.1021/ja405072z
Meitl, L. A., Eggleston, C. M., Colberg, P. J. S., Khare, N., Reardon, C. L., and Shi, L. (2009). Electrochemical interaction of Shewanella oneidensis MR-1 and its outer membrane cytochromes OmcA and MtrC with hematite electrodes. Geochim. Cosmochim. Acta 2009, 5292–5307. doi: 10.1016/j.gca.2009.06.021
Morgan, J. W., and Anders, E. (1980). Chemical composition of Earth, Venus, and Mercury. Proc. Natl. Acad. Sci. U. S. A. 77, 6973–6977. doi: 10.1073/pnas.77.12.6973
Nitschke, W., and Bonnefoy, V. (2016). “Energy accuisition in low-pH environments,” in Acidophiles: Life in Extremely Acidic Environments, eds R. Quatrini and D. B. Johnson (Norfolk, UK: Caister Academic Press), 19–48. doi: 10.21775/9781910190333.02
Pace, N. R. (1997). A molecular view of microbial diversity and the biosphere. Science 276, 734–740. doi: 10.1126/science.276.5313.734
Prakash, D., Chauhan, S. S., and Ferry, J. G. (2019). Life on the thermodynamic edge: Respiratory growth of an acetotrophic methanogen. Sci. Adv. 5:eaaw9059. doi: 10.1126/sciadv.aaw9059
Quatrini, R., and Johnson, D. B. (2018). Microbiomes in extremely acidic environments: functionalities and interactions that allow survival and growth of prokaryotes at low pH. Curr. Opin. Microbiol. 43, 139–147. doi: 10.1016/j.mib.2018.01.011
Reguera, G., and Kashefi, K. (2019). The electrifying physiology of Geobacter bacteria, 30 years on. Adv. Microb. Physiol. 74, 1–96. doi: 10.1016/bs.ampbs.2019.02.007
Roden, E. E., and Lovley, D. R. (1993). Dissimilatory Fe(III) reduction by the marine microorganism Desulfuromonas acetoxidans. Appl. Environ. Microbiol. 59, 734–742. doi: 10.1128/aem.59.3.734-742.1993
Scheller, S., Yu, H., Chadwick, G. L., Mcglynn, S. E., and Orphan, V. J. (2016). Artificial electron acceptors decouple archaeal methane oxidation from sulfate reduction. Science 351, 703–707. doi: 10.1126/science.aad7154
Segerer, A., Neuner, A., Kristjansson, J. K., and Stetter, K. O. (1986). Acidianus infernus gen.nov., sp. nov., and Acidianus brierleyi comb. nov.: facultatively aerobic, extremely acidophilic thermophilic surfur-metabolizing archaebacteria. Int. J. Syst. Evol. Microbiol. 36, 559–564. doi: 10.1099/00207713-36-4-559
Shi, L., Dong, H., Reguera, G., Beyenal, H., Lu, A., Liu, J., et al. (2016). Extracellular electron transfer mechanisms between microorganisms and minerals. Nat. Rev. Microbiol. 14, 651–662. doi: 10.1038/nrmicro.2016.93
Shi, L., Richardson, D. J., Wang, Z., Kerisit, S. N., Rosso, K. M., Zachara, J. M., et al. (2009). The roles of outer membrane cytochromes of Shewanella and Geobacter in extracellular electron transfer. Environ. Microbiol. Rep. 1, 220–227.
Shi, L., Rosso, K. M., Zachara, J. M., and Fredrickson, J. K. (2012). Mtr extracellular electron-transfer pathways in Fe(III)-reducing or Fe(II)-oxidizing bacteria: a genomic perspective. Biochem. Soc. Trans. 40, 1261–1267. doi: 10.1042/bst20120098
Shi, L., Squier, T. C., Zachara, J. M., and Fredrickson, J. K. (2007). Respiration of metal (hydr)oxides by Shewanella and Geobacter: a key role for multihaem c-type cytochromes. Mol. Microbiol. 65, 12–20. doi: 10.1111/j.1365-2958.2007.05783.x
Sivan, O., Shusta, S. S., and Valentine, D. L. (2016). Methanogens rapidly transition from methane production to iron reduction. Geobiology 14, 190–203. doi: 10.1111/gbi.12172
Skennerton, C. T., Chourey, K., Iyer, R., Hettich, R. L., Tyson, G. W., and Orphan, V. J. (2017). Methane-fueled syntrophy through extracellular electron transfer: uncovering the genomic traits conserved within diverse bacterial partners of anaerobic methanotrophic archaea. mBio 8, e530–e517.
Slobodkin, A. I., Jeanthon, C., L’haridon, S., Nazina, T., Miroshnichenko, M., and Bonch-Osmolovskaya, E. (1999). Dissimilatory reduction of Fe(III) by thermophilic bacteria and archaea in deep subsurface petroleum reservoirs of western siberia. Curr. Microbiol. 39, 99–102. doi: 10.1007/s002849900426
Slobodkina, G. B., Kolganova, T. V., Querellou, J., Bonch-Osmolovskaya, E. A., and Slobodkin, A. I. (2009). Geoglobus acetivorans sp. nov., an iron(III)-reducing archaeon from a deep-sea hydrothermal vent. Int. J. Syst. Evol. Microbiol. 59, 2880–2883. doi: 10.1099/ijs.0.011080-0
Smith, J. A., Aklujkar, M., Risso, C., Leang, C., Giloteaux, L., and Holmes, D. E. (2015). Mechanisms involved in Fe(III) respiration by the hyperthermophilic archaeon Ferroglobus placidus. Appl. Environ. Microbiol. 81, 2735–2744. doi: 10.1128/aem.04038-14
Soo, V. W., Mcanulty, M. J., Tripathi, A., Zhu, F., Zhang, L., Hatzakis, E., et al. (2016). Reversing methanogenesis to capture methane for liquid biofuel precursors. Microb. Cell Fact. 15:11.
Suzuki, T., Iwasaki, T., Uzawa, T., Hara, K., Nemoto, N., Kon, T., et al. (2002). Sulfolobus tokodaii sp. nov. (f. Sulfolobus sp. strain 7), a new member of the genus Sulfolobus isolated from Beppu Hot Springs, Japan. Extremophiles 6, 39–44. doi: 10.1007/s007920100221
Thauer, R. K., Kaster, A. K., Seedorf, H., Buckel, W., and Hedderich, R. (2008). Methanogenic archaea: ecologically relevant differences in energy conservation. Nat. Rev. Microbiol. 6, 579–591. doi: 10.1038/nrmicro1931
Timmers, P. H., Welte, C. U., Koehorst, J. J., Plugge, C. M., Jetten, M. S., and Stams, A. J. (2017). Reverse methanogenesis and respiration in methanotrophic archaea. Archaea 2017:1654237.
Tor, J. M., and Lovley, D. R. (2001). Anaerobic degradation of aromatic compounds coupled to Fe(III) reduction by Ferroglobus placidus. Environ. Microbiol. 3, 281–287. doi: 10.1046/j.1462-2920.2001.00192.x
Tyson, G. W., Chapman, J., Hugenholtz, P., Allen, E. E., Ram, R. J., Richardson, P. M., et al. (2004). Community structure and metabolism through reconstruction of microbial genomes from the environment. Nature 428, 37–43. doi: 10.1038/nature02340
Urbieta, M. S., Rascovan, N., Vazquez, M. P., and Donati, E. (2017). Genome analysis of the thermoacidophilic archaeon Acidianus copahuensis focusing on the metabolisms associated to biomining activities. BMC Genom. 18:445. doi: 10.1186/s12864-017-3828-x
Valdes, J., Pedroso, I., Quatrini, R., Dodson, R. J., Tettelin, H., Blake, R. II, et al. (2008). Acidithiobacillus ferrooxidans metabolism: from genome sequence to industrial applications. BMC Genom. 9:597. doi: 10.1186/1471-2164-9-597
Vargas, M., Kashefi, K., Blunt-Harris, E. L., and Lovley, D. R. (1998). Microbiological evidence for Fe(III) reduction on early Earth. Nature 395, 65–67. doi: 10.1038/25720
von Canstein, H., Ogawa, J., Shimizu, S., and Lloyd, J. R. (2008). Secretion of flavins by Shewanella species and their role in extracellular electron transfer. Appl. Environ. Microbiol. 74, 615–623. doi: 10.1128/aem.01387-07
Vorholt, J. A., Hafenbradl, D., Stetter, K. O., and Thauer, R. K. (1997). Pathways of autotrophic CO2 fixation and of dissimilatory nitrate reduction to N2O in Ferroglobus placidus. Arch. Microbiol. 167, 19–23. doi: 10.1007/s002030050411
Walker, J. C. (1984). Suboxic diagenesis in banded iron formations. Nature 309, 340–342. doi: 10.1038/309340a0
Walker, J. C. (1987). Was the Archaean biosphere upside down? Nature 329, 710–712. doi: 10.1038/329710a0
Wang, F., Gu, Y., O’brien, J. P., Yi, S. M., Yalcin, S. E., Srikanth, V., et al. (2019). Structure of microbial nanowires reveals stacked hemes that transport electrons over micrometers. Cell 177, 361–369 e310.
Weber, H. S., Habicht, K. S., and Thamdrup, B. (2017). Anaerobic methanotrophic archaea of the ANME-2d cluster are active in a low-sulfate, iron-rich freshwater sediment. Front. Microbiol. 8:619. doi: 10.3389/fmicb.2017.00619
Wegener, G., Krukenberg, V., Riedel, D., Tegetmeyer, H. E., and Boetius, A. (2015). Intercellular wiring enables electron transfer between methanotrophic archaea and bacteria. Nature 526, 587–590. doi: 10.1038/nature15733
Wheaton, G. H., Vitko, N. P., Counts, J. A., Dulkis, J. A., Podolsky, I., Mukherjee, A., et al. (2019). Extremely thermoacidophilic Metallosphaera species mediate mobilization and oxidation of vanadium and molybdenum oxides. Appl. Environ. Microbiol. 85:e02805–18.
White, G. F., Edwards, M. J., Gomez-Perez, L., Richardson, D. J., Butt, J. N., and Clarke, T. A. (2016). Mechanisms of bacterial extracellular electron exchange. Adv. Microb. Physiol. 68, 87–138. doi: 10.1016/bs.ampbs.2016.02.002
Xiong, Y., Shi, L., Chen, B., Mayer, M. U., Lower, B. H., Londer, Y., et al. (2006). High-affinity binding and direct electron transfer to solid metals by the Shewanella oneidensis MR-1 outer membrane c-type cytochrome OmcA. J. Am. Chem. Soc. 128, 13978–13979. doi: 10.1021/ja063526d
Yalcin, S. E., O’brien, J. P., Gu, Y., Reiss, K., Yi, S. M., Jain, R., et al. (2020). Electric field stimulates production of highly conductive microbial OmcZ nanowires. Nat. Chem. Biol. 16, 1136–1142. doi: 10.1038/s41589-020-0623-9
Yamada, C., Kato, S., Kimura, S., Ishii, M., and Igarashi, Y. (2014). Reduction of Fe(III) oxides by phylogenetically and physiologically diverse thermophilic methanogens. FEMS Microbiol. Ecol. 89, 637–645. doi: 10.1111/1574-6941.12365
Yan, Z., and Ferry, J. G. (2018). Electron bifurcation and confurcation in ethanogenesis and reverse methanogenesis. Front. Microbiol. 9:1322. doi: 10.3389/fmicb.2018.01322
Yan, Z., Joshi, P., Gorski, C. A., and Ferry, J. G. (2018). A biochemical framework for anaerobic oxidation of methane driven by Fe(III)-dependent respiration. Nat. Commun. 9:1642.
Zhang, J., Dong, H., Liu, D., and Agrawal, A. (2013). Microbial reduction of Fe(III) in smectitte minerals by thermiphilic methanogen Methanothermobacter thermautotrophicus. Geochim. Cosmochim. Acta 106, 203–315. doi: 10.1016/j.gca.2012.12.031
Zhang, J., Dong, H., Liu, D., Fischer, T. B., Wang, S., and Huang, L. (2012). Microbial reduction of Fe(III) in illite-smectite minerals by methanogen Methanosarcina mazei. Chem. Geol. 292-293, 35–44. doi: 10.1016/j.chemgeo.2011.11.003
Zhou, H., Zhang, R., Hu, P., Zeng, W., Xie, Y., Wu, C., et al. (2008). Isolation and characterization of Ferroplasma thermophilum sp. nov., a novel extremely acidophilic, moderately thermophilic archaeon and its role in bioleaching of chalcopyrite. J. Appl. Microbiol. 105, 591–601. doi: 10.1111/j.1365-2672.2008.03807.x
Keywords: dissimilatory Fe(III)-reducing archaea, dissimilatory Fe(II)-oxidizing archaea, redox proteins, electron transfer, molecular mechanisms
Citation: Dong Y, Shan Y, Xia K and Shi L (2021) The Proposed Molecular Mechanisms Used by Archaea for Fe(III) Reduction and Fe(II) Oxidation. Front. Microbiol. 12:690918. doi: 10.3389/fmicb.2021.690918
Received: 04 April 2021; Accepted: 02 June 2021;
Published: 01 July 2021.
Edited by:
Kevin Rosso, Pacific Northwest National Laboratory (DOE), United StatesReviewed by:
Abhiney Jain, University of Minnesota Twin Cities, United StatesCopyright © 2021 Dong, Shan, Xia and Shi. This is an open-access article distributed under the terms of the Creative Commons Attribution License (CC BY). The use, distribution or reproduction in other forums is permitted, provided the original author(s) and the copyright owner(s) are credited and that the original publication in this journal is cited, in accordance with accepted academic practice. No use, distribution or reproduction is permitted which does not comply with these terms.
*Correspondence: Liang Shi, bGlhbmcuc2hpQGN1Zy5lZHUuY24=
Disclaimer: All claims expressed in this article are solely those of the authors and do not necessarily represent those of their affiliated organizations, or those of the publisher, the editors and the reviewers. Any product that may be evaluated in this article or claim that may be made by its manufacturer is not guaranteed or endorsed by the publisher.
Research integrity at Frontiers
Learn more about the work of our research integrity team to safeguard the quality of each article we publish.