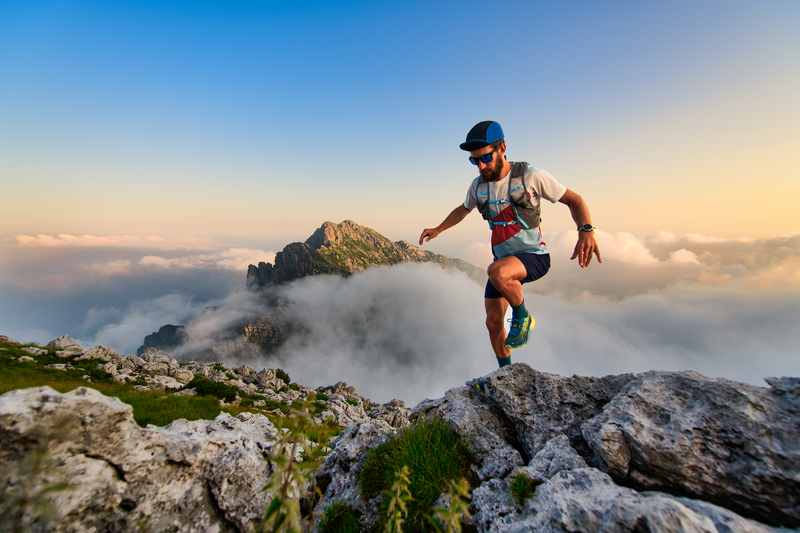
94% of researchers rate our articles as excellent or good
Learn more about the work of our research integrity team to safeguard the quality of each article we publish.
Find out more
ORIGINAL RESEARCH article
Front. Microbiol. , 28 June 2021
Sec. Antimicrobials, Resistance and Chemotherapy
Volume 12 - 2021 | https://doi.org/10.3389/fmicb.2021.690744
Ribosome stalling on ermBL at the tenth codon (Asp) is believed to be a major mechanism of ermB induction by erythromycin (Ery). In this study, we demonstrated that the mechanism of ermB induction by Ery depends not only on ermBL expression but also on previously unreported ermBL2 expression. Introducing premature termination codons in ermBL, we proved that translation of the N-terminal region of ermBL is the key component for ermB induced by Ery, whereas translation of the C-terminal region of ermBL did not affect Ery-induced ermB. Mutation of the tenth codon (Asp10) of ermBL with other amino acids showed that the degree of induction in vivo was not completely consistent with the data from the in vitro toe printing assay. Alanine-scanning mutagenesis of ermBL demonstrated that both N-terminal residues (R7-K11) and the latter part of ermBL (K20-K27) are critical for Ery induction of ermB. The frameshifting reporter plasmid showed that a new leader peptide, ermBL2, exists in the ermB regulatory region. Further, introducing premature termination mutation and alanine-scanning mutagenesis of ermBL2 demonstrated that the N-terminus of ermBL2 is essential for induction by Ery. Therefore, the detailed function of ermBL2 requires further study.
The mechanism of bacterial resistance is most likely due to the expression of antibiotic-resistance genes. Macrolide antibiotics are used to treat infections caused by gram-positive and gram-negative bacteria (Dinos, 2017). These antibiotics hinder bacterial growth by inhibiting protein synthesis through binding to the nascent peptide exit tunnel (NPET) (Kannan et al., 2014; Patel and Hashmi, 2020). In some cases, macrolide antibiotics act not only as inhibitors of translation, but also as activators of several resistance genes (Vazquez-Laslop et al., 2008; Arenz et al., 2014; Sothiselvam et al., 2014; Almutairi et al., 2015). For example, macrolide antibiotics bind to the ribosome, promote ribosome stalling on the regulatory leader peptide ermCL or ermBL, and then activate the expression of the antibiotic resistance genes ermC or ermB (Vazquez-Laslop et al., 2008; Arenz et al., 2014).
The ermB gene encodes the ribosomal methylase that dimethylates a single adenine in 23S rRNA, which causes high-level macrolide resistance and cell survival (Chassy and Thompson, 1983; Boerlin et al., 2001; Leclercq and Courvalin, 2002). ErmB is often found in macrolide-resistant isolates of several gram-positive bacteria such as staphylococci, streptococci, enterococci, and clostridia, but is increasingly found in gram-negative bacteria (Schroeder and Stephens, 2016). The expression of ermB can be either constitutive (M19270) (Brisson-Noel et al., 1988) or inducible (M11180) (Shaw and Clewell, 1985) depending on the regulatory region located upstream of the ermB gene.
In the Enterococcus faecalis strain DS16 transposon Tn917 (M11180) (Smith and Pappano, 1985), ermB is preceded by a 258 nt regulatory region, which contains a regulatory open reading frame ermBL encoding a 27 amino acid-long leader peptide. A hypothesis of translational arrest on ermBL as a mechanism of ermB induction by erythromycin (Ery) has been proved many times using the in vitro toe-printing assay. Specifically, the regulatory region of ermB includes a short leader peptide called ermBL with its ribosome binding site (RBS1), non-translational loop-stem structure, and several coding sequences of ermB, including its ribosome binding site (RBS2) (Figure 1A and Supplementary Figure 1). Without Ery, expression of ermBL is normal, the translation initiation site (RBS2 and AUG) of ermB is sequestered in the secondary structure formed by elements ➂ and ➃, and ermB expression is translationally attenuated (Supplementary Figure 1). In the presence of Ery, the ribosome stalls when the tenth (Asp) codon of the ermBL ORF enters the ribosomal P site. The stalled ribosome destabilizes helix ➀–➁ and, as a result, promotes the formation of an alternative structure of the switch region in which the translation initiation site of ermB is liberated and ermB can be translated (Min et al., 2008; Arenz et al., 2014, 2016; Dzyubak and Yap, 2016; Gupta et al., 2016; Supplementary Figure 1). However, the detailed mechanism has not yet been demonstrated in vivo. In addition, there are many nucleotides between elements ➁ and ➂, and their functions have not been studied.
Figure 1. N-terminal, not C-terminal, region of ermBL is necessary for ermB induction by erythromycin. (A) The structure of the ermBL-ermB-based pGEX reporter plasmid. (B) β-Galactosidase activity of the ermBL-ermB-lacZα reporter gene during erythromycin (Ery) titration. Data are mean ± SEM from three independent experiments (unpaired two-tailed Student’s t-test). (C) Agar diffusion assays of cells transformed with the reporter plasmid containing ermBL grown on plates with IPTG and X-gal; each filter disc was spotted with different concentration of Ery and DMSO. (D) Flow cytometry of GFP intensity is shown. Data are mean ± SEM from three independent experiments (unpaired two-tailed Student’s t-test). (E) β-Galactosidase activity and agar diffusion assays of different premature terminations of ermBL. Plate were wetted with 1 μL of a solution of Ery (128 mg/mL).
Here, we used a well-studied ermBL-ermB operon (M11180) as a model to investigate the detailed induction mechanism of Ery in vivo (Shaw and Clewell, 1985). We constructed a pGEX-ermBL-ermB’-reporter plasmid. ErmB’ is the N-terminal region of the ermB gene and does not have any ribosomal methylase activity, but is essential for structural transition. We found that the N-terminus, not the C-terminus, of ermBL was necessary for ermB induction by Ery. Synonymous mutation of V9-K11 of ermBL indicated that amino acids, rather than nucleotide sequences, are critical for induction by Ery. Mutation of D10 with other amino acids revealed that the degree of induction in vivo was not completely consistent with the efficiency of ribosome stalling by in vitro toe printing assay. Alanine-scanning mutagenesis of ermBL demonstrated that both N-terminal residues (R7-K11) and the latter part of ermBL (K20-K27) are critical for Ery induction of ermB. Frameshifting reporter translational fusion showed that there is a new leader peptide, ermBL2, in the ermB regulatory region. Introducing premature termination mutation and alanine-scanning mutagenesis of ermBL2 demonstrated that the N-terminus of ermBL2 is essential for induction by Ery. The detailed mechanism requires further research, and this study can further enhance the understanding of the mechanism of ermB induction by erythromycin.
Antibiotics (Erythromycin, Ery) were obtained from Sigma-Aldrich. Isopropyl-β-D-thiogalactopyranoside (IPTG) and 5-bromo-4-chloro-3-indolyl-D-galactopyranoside (X-Gal) were purchased from Sigma-Aldrich. Luria-Bertani (LB) broth components and agar were purchased from Sigma-Aldrich. The enzymes used for DNA cloning were obtained from Fermentas. All oligonucleotide primers were synthesized using Invitrogen DNA Technologies. E. coli strains were grown in LB broth at 37°C. Plasmid pGEX-4T-3(GE Healthcare) was used as the vector to generate the pGEX-ermBL-ermB’-lacZα or GFP reporter plasmid. pGEX-ermBL-ermB’-lacZα included the IPTG-inducible tac promoter (Ptac), followed by unique KpnI and AflII restriction sites and the transcription terminator, lacIq gene, ampicillin resistance (Ampr) marker, and pBR322 origin of replication. All the cloning procedures and most experiments with the engineered constructs were carried out with E. coli strain JM109 (Promega) [Genotype:endA1, recA1, gyrA96, thi, hsdR17 (rk–, mk+), relA1, supE44, Δ (lac-proAB), [F’ traD36, proAB, laqIqZΔ M15].
The pGEX vector was digested with BspMI, TthIII, and a new multiple cloning site (SmaI-KpnI-XbaI-AflII-XhoI-TthIIII). The ermBL-ermB’ cassette (Enterococcus faecalis strain DS16 transposon Tn917), including the tac promoter, leader ORF, and a part of the ermB coding sequence (ermB’), was directly synthesized from Invitrogen DNA Technologies. KpnI and AflII restriction sites were introduced at the ends of the amplified fragments using PCR primers. The ermB-ermB’ cassette was cloned between the KpnI and AflII sites of the pGEX vector to produce the translational fusion plasmid pGEX-Ptac-ermBL-ermB’. ErmB’ was the N-terminal region of the ermB gene. ErmB’ does not have any ribosomal methylase activity, but is essential for conformational change in the proposed Ery-induced model of ermB (Figure 1A and Supplementary Figure 1). The reporter gene lacZα or GFP (without its start codon) was cloned into the vector following ermB by AflII and XhoI (Supplementary Figure 2).
The disc diffusion assay protocol for testing the inducibility of the pGEX reporter was carried out as described previously (Bailey et al., 2008). Briefly, the culture of cells transformed with the pGEX lacZα reporter plasmid was incubated overnight in LB broth containing 100 μg/mL ampicillin. The culture was diluted 1:100 in fresh LB broth containing ampicillin (100 μg/mL) and IPTG (0.5 mM) and incubated for 1.5–2 h until the OD600 approached 0.2. Subsequently, 1 × 108 CFU cells were added into 5 mL of 0.6% LB agar at 50°C. After brief mixing, the cell suspension was poured on top of a 1.5% LB agar plate containing 100 μg/mL ampicillin, 0.5 mM IPTG, and 160 μg/mL X-Gal. Once the soft agar had solidified, the 3 MM paper discs were placed on agar and wetted with erythromycin (128 mg/mL, 1 μL). The plates were incubated for 18–24 h at 37°C.
E. coli strains carrying the pGEX-ermBL-ermB’- lacZα plasmid were grown in LB until OD600 ≈ 0.2. Cultures were split and treated with a series of antibiotic concentrations. After treatment at 37°C for 3 h, 0.2 mL cell cultures were harvested in triplicate, and β-galactosidase activity was measured in these samples using standard protocols. Miller units were calculated by normalizing to cell density (OD600). At least three independent biological replicates were analyzed.
E. coli strains carrying the pGEX-ermBL-ermB’- GFP plasmid were grown in LB until OD600 ≈ 0.2. Cultures were split and treated with a series of antibiotic concentrations. After treatment at 37°C for 3 h, 0.2 mL cell cultures were washed with PBS and centrifuged at 500 g for 2 min. The supernatant was discarded, and the cells were resuspended in PBS. GFP levels were analyzed using a BD Aria II flow cytometer (BD Biosciences) with a 70 μm nozzle. The cell populations were detected using forward and side scatter (FSC and SSC) parameters, and fluorescence was analyzed with an emitting laser of 488 nm and a bandpass filter of 525/15 nm. Graphs were generated using FlowJo (Tree Star software), and the mean fluorescence intensity (MFI) is presented in relative fluorescence units (RFUs).
pGEX-ermBL-ermB’-lacZα or GFP mutants were generated using the QuickChange site-directed mutagenesis kit (Stratagene). Mutagenic primers containing the desired mutations were amplified using Pfu Ultra DNA polymerase. The parental DNA was digested with DpnI enzyme (Fermentas). The pure mutated DNA was transformed into competent cells, and the cell suspension was poured on an LB agar plate containing 100 μg/mL ampicillin. Mutated fragments of the monoclonal bacteria were confirmed by sequencing. All the mutagenic primers sequence were showed in Supplementary Table 2.
Minimal inhibitory concentration (MIC) determinations were performed as previously described (Xiong et al., 2005). Briefly, cells were grown overnight in LB medium containing 100 μg/mL ampicillin. On the second day, the cultures were diluted 100-fold into fresh LB medium containing ampicillin and grown for 2 h. Exponential-phase cultures were then diluted to an A600 (OD600 of 0.002 and placed into fresh LB medium with 3 mL/tube. After the addition of antibiotics (0, 2, 4, 8, 16, 32, 64, 128, 256, 512, 1,024, 2,048, and 4,096 μg/mL), the tubes were incubated for 15 h at 37°C without shaking and 3 h with shaking. The MIC was recorded as the lowest concentration of the drug in the tube, with no obvious turbidity.
The expression of ermB is controlled by a leader ORF ermBL that encodes a 27-amino acid long peptide and is translationally attenuated in the absence of Ery (Min et al., 2008; Arenz et al., 2014, 2016; Dzyubak and Yap, 2016; Gupta et al., 2016). We first constructed a new pGEX reporter with the tac promoter (Ptac) following the regulatory region of the ermBL-ermB’ operon and translational fusion to lacZα or GFP (Figure 1A and Supplementary Figure 2). The regulatory region includes ermBL with its ribosome binding site (RBS1), non-translational loop-stem structure, and several coding sequences of ermB with its ribosome binding site (RBS2) (Figure 1A and Supplementary Figure 2). ErmB’ is the N-terminal of the ermB gene and has no ribosomal methylase activity, but ermB’ is essential for structural changes in the proposed Ery-induced ermB model. This system allows for easy monitoring of induction by antibiotics, either by measuring β-galactosidase enzyme activity (Figures 1B,C) or GFP intensity (Figure 1D). GFP intensity is showed by histograms which Y-axis represents the number of cells and the X-axis represents the relative GFP fluorescence intensity of each cell. We used mean fluorescence intensity (MFI) to present in relative fluorescence units (RFUs).
Translational arrest of ermBL is believed to be a mechanism of ermB induction by Ery (Min et al., 2008; Arenz et al., 2014, 2016; Dzyubak and Yap, 2016; Gupta et al., 2016). In a previous study, ermB was strongly induced by the subinhibitory concentration (approximately 10–25% MIC) of Ery (Min et al., 2008; Gupta et al., 2016). We first determined the minimal inhibitory concentration (MIC) of antibiotics for E. coli carrying the pGEX-ermBL-ermB’-reporter plasmid (Supplementary Table 1). We next used our plasmid to qualitatively and quantitatively investigate the induction by Ery at a subinhibitory concentration. Erythromycin induced ermBL-ermB’ lacZα at a broad range of concentrations (Figures 1B,C). As expected, the antibiotic solvent DMSO did not induce ermB expression (Figure 1C). When we changed the reporter gene from lacZα to GFP, Ery also induced the expression of ermBL-ermB’ GFP. These results showed that Ery specificity induces ermB expression, regardless of the reporter gene.
To prove that ermBL expression is critical for ermB induction by Ery in our reporter system. We constructed some mutations impaired the expression of complete ermBL. The leader ORF ermBL translated 27 long amino acids. Therefore, we deleted the RBS1 sequence (GGAGG) or changed the first codon ATG to a stop codon (ATG1:TAA) to stop expression of ermBL, as expect, ermB induction by Ery was impaired (Figure 1E) which means ermBL expression is critical for ermB induction by Ery. When we changed the 20th codon of ermBL to a stop codon (AAA20:TGA), the C-terminus of ermBL is premature termination. ermB could also be induced by Ery, which means that the N-terminus of ermBL is necessary for ermB induction by Ery while the C-terminus (K20-K27) of ermBL is not important (Figure 1E).
Ribosome stalling is believed to depend on amino acid sequences but not nucleotide sequences (Min et al., 2008; Vazquez-Laslop et al., 2008; Dinos, 2017). Previous study showed that the antibiotic does not interact directly with ermBL, but rather redirects the path of the peptide within the tunnel (Arenz et al., 2014) which remind us ribosome stalling on ermBL may be amino acid-dependent. To check whether ribosome stalling on ermBL is amino acid-dependent, we changed the V9-K11 codon to synonymous mutations. We changed the ninth codon of ermBL to synonymous mutations (GTA9:GTT, GTA9:GTC, GTA9:GTG), tenth codon GAT to GAC, 11th codon AAA to AAG separately. β-galactosidase assay and disc diffusion assay showed reporter genes were induced by Ery with all synonymous mutations (Figures 2A,B), indicating that ribosome stalling on ermBL was amino acid-dependent.
Figure 2. Ribosome stalling efficiency in vitro is not consistent with the degree of induction in vivo. (A,B) β-Galactosidase activity and agar diffusion assays of various ermBL synonymous mutations following Ery exposure. Data are mean ± SEM from three independent experiments (unpaired two-tailed Student’s t-test). (C,D) β-Galactosidase activity and agar diffusion assays of the degree of Ery induction in vivo for the Asp10 mutation of ermBL. Data are mean ± SEM from three independent experiments (unpaired two-tailed Student’s t-test).
The tenth codon Asp of ermBL is a key amino acid for ribosome stalling, and a previous in vitro study showed that ribosome stalling efficiency could be altered by mutation of the tenth codon with other amino acids (Gupta et al., 2016). Therefore, we investigated whether changes in Asp10 that affect ribosome stalling in vitro would result in similar changes in -sensitive gene expression in vivo. Gupta et al. showed that the stalling efficiency is Asp10 > Glu10 > Gly10 > …… > Tyr10 > Ala10 > Gln10 > Arg10 > Val10 > Cys10 by in vitro toeprinting assay (Gupta et al., 2016). The stalling efficiency of most amino acids in vitro is consistent with sensitivity to Ery in vivo. The Asp10, Glu10, Gly10 mutations showed residual stalling with Ery in vitro also showed high induction with Ery in vivo, whereas Arg10, Cys10 mutations greatly diminished ribosome stalling in response to Ery in vitro also showed low induction with Ery in vivo (Figures 2C,D). β-gal activity showed that Val10 and Gln10 were also sensitive to Ery in vivo (Figures 2C,D), while Val10 and Gln10 prevented sufficiently strong stalling in vitro in previous study (Gupta et al., 2016). These results indicate that there may be other mechanisms that are independent of ribosome stalling on ErmBL for Ery-induced ermB.
In a previous study, the seventh to the eleventh codons of ermBL (R7-K11) were found to be key amino acids for translation arrest by Ery in vitro (Arenz et al., 2014). To determine which amino acids are necessary for ermB induction by Ery in vivo, we performed an alanine-scanning mutagenesis assay in vivo (Figure 3A). As expected, R7-K11 mutated to alanine impaired ermB induction by Ery which means R7-K11 are critical for ermB induction by Ery (Figures 3B,C and Supplementary Figure 7A). Unexpectively, the last codons of ermBL (Ser22, Asp23, Tyr24, Lys27) mutated to alanine severely impaired ermB induction by Ery showed these last codons of ermBL were also determined to be critical for ermB induction (Figures 3B,C and Supplementary Figure 7A). When we changed the 20th codon to a stop codon (AAA20:TGA), ermB could be induced by Ery, suggesting that the last codons of ermBL (S22-K27) are not important (Figure 1E). However, alanine-scanning mutagenesis of ermBL showed that the last codons of ermBL (S22-K27) are very important for Ery-induced ermB (Figures 3B,C). These contradictory results indicate that there may be another mechanism for Ery-induced ermB.
Figure 3. Alanine-scanning mutagenesis of ermBL confirms key amino acids for ribosome stalling. (A) Amino acid sequences of ermBL peptide (WT) and its alanine-scanning mutations. (B,C) β-Galactosidase activity and agar diffusion assays of the degree of induction by Ery in vivo following Ala mutation of ermBL amino acid sequences. Data are mean ± SEM from three independent experiments (unpaired two-tailed Student’s t-test).
Because the last codons of ermBL are critical for ermB induction, we examined other mechanisms to control the ermB expression by Ery. Regulation of ermC gene expression by ketolides is controlled by ribosomal frameshifting (Gupta et al., 2013). In this mechanism, the last codons of ermCL are critical for ketolide-induced ermC induction (Gupta et al., 2013). Therefore, we constructed an ermBL-GFP frameshift mutation reporter (Figure 4A and Supplementary Figure 3). In brief, the Ptac ermBL(0) frameshift GFP fusion included the tac promoter, RBS1 sequence, ermBL leader peptide with TAA codon, and GFP reporter gene (GFP does not have its own start codon) (Figures 4A,D). Without any (0) frameshift induced by Ery, GFP could not be expressed with ermBL expression. Ptac ermBL(−1) frameshift GFP fusion contained the same sequence as Ptac ermBL(0) frameshift GFP fusion, except with inclusion of two nucleotides (AT) behind the stop codon TAA; therefore, without any (−1) frameshifting induced by Ery, GFP could not be expressed with ermBL expression (Figures 4A,C). Further, the Ptac ermBL(+1) frameshift GFP fusion had the same sequence as Ptac ermBL(0) frameshift GFP fusion, except for one nucleotide (A) behind the stop codon TAA; therefore, without any (+1) frameshifting induced by Ery, GFP could not be expressed with ermBL expression (Figures 4A,B). All these reporter fusions were repressed by the lacI gene. Without IPTG, ermBL transcription was repressed.
Figure 4. ermBL GFP frameshift mutation reveals new leader peptide. (A) The structure of the ermBL reporter for testing erythromycin-dependent frameshifting. (B) Structure of the ermBL (+1) frameshift reporter, ermBL with TAA codon following two nucleotides (AT) fused to GFP. GFP does not have its own start codon. Flow cytometry of GFP intensity is shown. (C) Structure of the ermBL(0) frameshift reporter, ermBL with a TAA codon directly fused to GFP. GFP does not have its own start codon. Flow cytometry of GFP intensity is shown. (D) The structure of the ermBL(−1) frameshift reporter, ermBL with TAA codon following one nucleotide (A) fused to GFP. GFP does not have its own start codon. Flow cytometry of GFP intensity is shown. Data are mean ± SEM from three independent experiments (unpaired two-tailed Student’s t-test).
Flow cytometry showed that (0) frameshifting, (−1) frameshifting and (+1) frameshifting mutation reporters did not induce GFP expression by Ery with IPTG, indicating that Ery cannot induce these types of frameshifting (Figures 4B–D). At the same time, GFP expression was almost at the same level with IPTG compared to that without IPTG in (0) frameshifting and (−1) frameshifting reporters, while GFP expression was higher with IPTG than without IPTG in the (+1) frameshifting construct (Figures 4B–D), which indicated that there was another leader peptide that (+1) frameshift with known leader peptide ermBL.
In the above study, we identified a new peptide in the ermB regulatory region. According to the genetic code triplet principle, start codon (ATG, TTG, or GTG), stop codon (TAA, TAG, or TGA), and (+1) frameshift with known leader peptide ermBL, we predicted the sequence information of a new leader peptide named ermBL2 (Figure 5A and Supplementary Figure 4). Based on our predicted sequences, we constructed four clones to verify the complete sequence of the new leader peptide. PGEX M1 included the RBS1 sequence, a part of ermBL, and translational fusion to GFP without the start codon of predicted ermBL2. ermBL and GFP contained (+1)frameshift mutations; therefore, GFP could not be expressed with ermBL expression (Figure 5B). PGEX M2 included the predicted start codon (GTG) of ermBL2 and translational fusion to GFP (Figure 5C). PGEX M3 did not have a stop codon of predicted ermBL2, and ermBL2 is translational fusion to GFP (Figure 5D). PGEX M4 had a stop codon of ermBL2 following translational fusion to GFP (Figure 5E). All the mutations were controlled by tac promoter, Without IPTG, GFP couldn’t be expressed. At the same time, all the fusions were (+1) frameshift with ermBL, therefore, GFP could not be expressed with ermBL expression. Without IPTG, mean fluorescence intensity (MFI) of all four clones was very low (Figures 5B–E). With IPTG, PGEX M2 and PGEX M4 had higher GFP expression than those without IPTG (Figures 5C,D), indicating that the new leader peptide named ermBL2 is from GTG to TAA (MITQINKYVILIPTSD) (Figure 5F).
Figure 5. Complete sequence information of new leader peptide named ermBL2. (A) The detailed sequence of ermBL and predicted new leader peptide. (B) The structure of PGEX M1. The fusion contains the predicted RBS3, but does not have the predicted start codon. GFP (without its own start codon) is not expressed with ermBL, as ermBL and GFP have (+1) frameshifts. Flow cytometry of GFP intensity is shown. (C) The structure of PGEX M2. The fusion contains both the predicted RBS3 and predicted start codon. (D) The structure of PGEX M3. The fusion contains the predicted RBS3, predicted start codon, and full length of predicted ermBL2 except the TAA codon. (E) The structure of PGEX M4. The fusion contains the predicted RBS3, predicted start codon, and full length of predicted ermBL2 including the TAA codon. (F) Model of the ermB regulatory region, including two leader peptides. Data are mean ± SEM from three independent experiments (unpaired two-tailed Student’s t-test).
Ribosome stalling on ermBL is a major mechanism of Ery-induced ermB induction. The subinhibitory concentration (25% of the minimal inhibitory concentration, MIC) of Ery induced ermB expression (Min et al., 2008). Macrolides selectively inhibit translation of a subset of cellular proteins rather than global inhibitors of protein synthesis and that their action most depends on the nascent protein sequence and on the antibiotic structure (Kannan et al., 2012; Almutairi et al., 2017). On the other hand, Ery does not inhibit ermBL expression, which is a prerequisite for ermB induction by Ery. We wanted to determine the effect of Ery on the expression of the leader peptide. Thus, we constructed three translational fusions (ermBL1 GFP, ermBL1-ermBL2 GFP, and ermBL2 GFP) (Figures 6A–D and Supplementary Figure 5). All three fusions were IPTG-dependent. Without IPTG, MFI of three fusions is very low (Figures 6B–D). We found that the subinhibitory concentration of Ery did not have any effect on the expression of the leader peptides as MFI of fusions is almost same with Ery compared to DMSO (Figures 6B–D). These results showed that ribosome stalling on the leader peptide may not be a stationary state, as ribosome stalling on ermBL does not affect ermBL expression.
Figure 6. Erythromycin has no effect on leader peptide expression. (A) The detailed sequence of ermBL and the new leader peptide named ermBL2. (B) The structure of the ermBL-GFP translational fusion. The fusion contains the RBS1 sequence and full length of ermBL without the TAA stop codon. Flow cytometry of GFP intensity is shown. (C) The structure of the ermBL-ermBL2-GFP translational fusion. The fusion contains the full length of ermBL and ermBL2 without the stop codon of ermBL2. Flow cytometry of GFP intensity is shown. (D) The structure of the ermBL2-GFP translational fusion. The fusion contains the RBS3 sequence and full length of ermBL2 without the TAA stop codon. Flow cytometry of GFP intensity is shown. Data are mean ± SEM from three independent experiments (unpaired two-tailed Student’s t-test).Figure 7 | N-terminal region of ermBL2 is necessary for ermB induction by erythromycin. (A) Amino acid sequences of ermBL2 peptide (WT) and its alanine-scanning mutations. (B) β-Galactosidase activity and agar diffusion assays of the degree of Ery induction in vivo following Ala mutation of ermBL2 amino acid sequences. (C) β-Galactosidase activity and agar diffusion assays of the degree of Ery induction in vivo following introduction of premature termination codons in ermBL2. Data are mean ± SEM from three independent experiments (unpaired two-tailed Student’s t-test).
In this study, we identified a new peptide, ermBL2, and wanted to determine whether its expression was critical for ermB induction by Ery. Alanine-scanning mutagenesis of ermBL2 showed that the N-terminus of ermBL2 was necessary for ermB induction by Ery as several amino acid of ermBL2 mutated to alanine severely impaired ermB induction by Ery (Figures 7A,B and Supplementary Figure 7B). Especially, N-terminal of ermBL2 is necessary for ermB induction by erythromycin (Figure 7B and Supplementary Figure 7B). Introducing premature termination codons in ermBL2 also led to the same conclusion which N-terminal of ermBL2 is necessary for ermB induction by erythromycin (Figure 7C). Taken together, these results may explain the contradictory phenomenon found in the above study: introducing a termination codon showed that the last codons of ermBL are not important for ermB induction by Ery (Figure 1E), whereas alanine-scanning mutagenesis of ermBL showed that the last codons of ermBL are very important (Figure 3B). Alanine mutagenesis of the last codon of ermBL not only changed the amino acids of ermBL but also changed the N-terminal amino acids of ermBL2 (Supplementary Figure 6), suggesting that the N-terminus of ermBL and ermBL2 are synergetic and indispensable for Ery induction of ermB.
Figure 7. (A) Amino acid sequences of ermBL2 peptide (WT) and its alanine-scanning mutations. 720 (B) β-Galactosidase activity and agar diffusion assays of the degree of Ery induction in 721 vivo following Ala mutation of ermBL2 amino acid sequences. (C) β-Galactosidase 722 activity and agar diffusion assays of the degree of Ery induction in vivo following 723 introduction of premature termination codons in ermBL2. Data are mean ± SEM from 724 three independent experiments. (unpaired two-tailed Student t test).
The alanine scanning experiments require at least one nucleotide changes. The Alanine-scanning mutagenesis C-terminus of ermBL or N-terminus of ermBL2 could affect the interaction between region ➁ with region ➂, which forms the stem and loop structure that releases the RBS2 and start codon of ermB (Supplementary Figure 1 induced). If alanine scanning experiments of ermBL2 breaks the ➂–➃ stem structure, therefore the ➁–➂ stem structure maintains even in the presence of erythromycin which will keep low expression of their reporter genes. Under this possibility, ermB could not be induced by Ery just because of lost of structure change but not function of ermBL2. In order to exclude this possibility, we carried out a single nucleotide mutation in the start codon of ermBL2(GTG) to ATG, TTG, CTG, GAG, GGG, GCG, GTA, GTT, or GTC. The single base mutation has the least damage to the interaction between region ➁ with region ➂. ErmB could be induced by Ery with ATG, TTG or CTG mutations while other single base mutation impaired ErmB induction by Ery (Supplementary Figure 8B). Except GTG could be as start codon, ATG, TTG, or CTG also could be as start codon. These single nucleotide mutations showed expression of ermBL2 is major reason for ErmB induction by Ery. We also changed the start codon of ermBL2(GTG) to alanine codon (GCT,GCC,GCA) (Supplementary Figure 8C) or stop codon (TAA,TAG,TGA) (Supplementary Figure 8D) and found ErmB induction by Ery is impaired which further proves the importance of ermBL2 expression for ErmB induction by Ery. The fourth codon of ermBL2(CAG) is in the loop structure as the model of Supplementary Figure 1 induced (Supplementary Figure 8A). Theoretically, the mutation of this amino acid does not affect the interaction between region ➁ and region ➂. We changed the fourth codon of ermBL2 (CAG) to stop codon (TGA), alanine (GCC), arginine (CGG), proline (CCG), or leucine (CTG) (Supplementary Figure 8E). We found the fourth codon mutated to stop codon or alanine impaired the ErmB induction by Ery while mutated to arginine, proline and leucine maintained ErmB induction by Ery (Supplementary Figure 8E). These data showed the expression of ermBL2 is critical for ErmB induction by Ery and is amino-acid dependent.
Antibiotic resistance is a growing public health concern (Bader et al., 2017). The abuse of antibiotics and the lack of new antibiotics aggravate the severity of antibiotic resistance (Ventola, 2015). The mechanism of bacterial resistance is mostly due to the expression of antibiotic-resistance genes (Pontes et al., 2018). Resistance genes can be expressed in either constitutive or inducible forms. Therefore, additional studies on the expression mechanism of inducible resistance genes may address the challenge of antibiotic resistance and delay the urgent need for new antibiotics.
Macrolide antibiotics are used to treat infections caused by gram-positive and gram-negative bacteria (Patel and Hashmi, 2020). They kill bacteria by inhibiting bacterial protein synthesis and impeding the passage of newly synthesized polypeptides through the nascent peptide exit tunnel of the bacterial ribosome. Recent data have challenged this view by showing that macrolide antibiotics can differentially affect the synthesis of individual proteins in a sequence-specific manner (Kannan et al., 2014).
Ribosome stalling on ermBL has been proven several times in vitro (Arenz et al., 2014, 2016). In this study, introduction of a premature termination codon and alanine-scanning mutagenesis of ermBL showed a contradictory phenomenon. Specifically, introducing a termination codon suggested that the last codon of ermBL is not important, while alanine-scanning mutagenesis of ermBL highlighted the critical importance of the last codons (Figures 1E, 3B,C). To explain this phenomenon, we constructed three frameshifting fusions (Figure 4A). We did not find any frameshifting induced by Ery (Figure 4). However, we found a new peptide, ermBL2, expressed in the ermB regulatory region (Figure 4). We then introduced translational fusion constructs to determine the start and stop codons of ermBL2 (Figure 5). Introducing premature termination mutation and alanine-scanning mutagenesis of ermBL2 demonstrated that the N-terminus of ermBL2 is essential for Ery induction of ermB (Figure 7). Further, while the C-terminus of ermBL and the N-terminus of ermBL2 share the nucleotide sequence but not the amino acid sequence. Alanine-scanning mutagenesis of the C-terminal region of ermBL also simultaneously altered amino acids at the N-terminus of ermBL2 (Supplementary Figure 6), which may explain the impaired ermB induction by Ery with mutation of the C-terminal region of ermBL.
Inducible Erm-type methyltransferase genes are usually preceded by a regulatory leader peptide (ORF). Currently, only a handful of macrolide resistance genes (ermA, ermB, ermC, ermD, and ermS) have been investigated in detail (Kamimiya and Weisblum, 1988; Vazquez-Laslop et al., 2008; Ramu et al., 2011; Arenz et al., 2014; Sothiselvam et al., 2014). ErmA and ermD are believed to contain two leader peptides in the regulatory region. Even ribosome stalling takes place both on ermAL1 and ermAL2, yet the interplay of the two regulatory ORFs in the control of ermA induction is not clearly understood. Two models have interpreted the role of ermAL1 and ermAL2: (1) ribosome stalling at ermAL1 activates translation of ermAL2, whereas ribosome stalling at ermAL2 directly leads to the translation of ermA. (2) Alternatively, ribosome stalling at ermAL1 may control mRNA stability, whereas formation of the stalled complex at ermAL2 may regulate the translation of ermA (Murphy, 1985; Clarebout et al., 2001; Ramu et al., 2011). ErmDL and ermDL2 are present in the ermD regulatory region. Ribosome stalling at ermDL is critical for Ery induction, but the role of ermDL2 has not been explored (Gryczan et al., 1984; Kwak et al., 1991; Hue and Bechhofer, 1992). Before our study, only one leader peptide was believed to exist in the ermB regulatory region. Our study showed that there is another leader peptide, ermBL2, which is also critical for ermB induction (Figure 5). Therefore, the classic model of the predicted conformational switch of ermB induced by Ery should be adjusted (Supplementary Figure 1). To date, we do not have any biochemical data (in vitro toe-printing assay) or bioinformatics evidence to examine whether ribosome stalling takes place on ermBL2. However, it is known that ermBL1 and ermBL2 are synergistic and indispensable for ermB induction by Ery.
In addition, we found that ermBL2 expression was repressed in the ermBL-ermBL2 sequence, as ermBL2-GFP expression was higher than ermBL-ermBL2-GFP expression (Figure 6). However, ermBL-ermBL2-GFP expression could not be induced by Ery, which means that ribosome stalling on ermBL could not induce ermBL2 expression. As such, the mechanism of Ery-induced ermB and the necessity of two leader peptides warrants further study.
Macrolides affect protein synthesis in a sequence-specific manner. In a study by Kannan et al. (2014) they used the ribosome profiling technique to analyze macrolide-induced redistribution of ribosomes on cellular mRNAs and found that genes could be grouped into three major modes by Ery treatment: inhibition of translation at early stages, translation arrest at the internal codons of the gene, and translation through the entire length of the gene. To determine whether Ery affects the expression of leader peptides, we constructed three translational fusions (ermBL1 GFP, ermBL1-ermBL2 GFP, and ermBL2 GFP) (Figures 6A–D and Supplementary Figure 5). Interestingly, Ery did not have any effect on the expression of the leader peptides, even when ribosomes stalling took place on ermBL, ermBL expression is also not change with Ery (Figure 6). It is an interesting phenomenon and there are two possibilities about expression of leader peptide-GFP fusion don’t change with Ery. On the one hand, Macrolides selectively inhibit translation of a subset of cellular proteins rather than global inhibitors of protein synthesis and that their action most depends on the nascent protein sequence and on the antibiotic structure. Macrolides maybe selectively don’t inhibit translation of leader peptide. On the other hand, expression of leader peptide-GFP in the presence of sublethal concentrations of Ery could be the product of free-erythromycin ribosomes moving along their open reading frames. Because it is difficult to calculate the proportion of ribosomes arrested in both ermBL genes with Ery, we are not sure whether the expression of leader peptide with Ery is due to free-erythromycin ribosomes moving along their open reading frames or selectively don’t inhibit translation by Ery. Therefore, knowing how erythromycin work may contribute to understand its action on leader peptide. Its mechanism needs further study.
The raw data supporting the conclusions of this article will be made available by the authors, without undue reservation.
SW, KJ, ZH, and WH contributed to conception and design of the study. SW, XD, and YL organized the database. KJ and LL performed the statistical analysis. WH wrote the first draft of the manuscript and sections of the manuscript. All authors contributed to manuscript revision, read, and approved the submitted version.
This work was supported by the China Postdoctoral Science Foundation 2018M642075 to WH and by the National Natural Science Foundation of China 82002945 to WH and 81772954 to ZH. This work was also funded by Major Program of National Key Research and Development Project (2020YFA0112600 and 2019YFA0801502), Shanghai Zhangjiang National Innovtaion Demonstration Zone (ZJ2018-ZD-004), the Project of Shanghai science and technology commission (19140902900), the Top-Level Clinical Discipline Project of Shanghai Pudong (PWYgf2018-04), Program of Shanghai Academic/Technology Research Leader (20XD1434000), and Peak Disciplines (Type IV) of Institutions of Higher Learning in Shanghai to ZH. This work was also funded by Shanghai Pujiang Program 2020PJD050 and Academic medicine leader’s training Program in health systems of Pudong New Area, PWRd2020-06 to LL.
The authors declare that the research was conducted in the absence of any commercial or financial relationships that could be construed as a potential conflict of interest.
We thank all participants in the study.
The Supplementary Material for this article can be found online at: https://www.frontiersin.org/articles/10.3389/fmicb.2021.690744/full#supplementary-material
Almutairi, M. M., Park, S. R., Rose, S., Hansen, D. A., Vazquez-Laslop, N., Douthwaite, S., et al. (2015). Resistance to ketolide antibiotics by coordinated expression of rRNA methyltransferases in a bacterial producer of natural ketolides. Proc. Natl. Acad. Sci. U. S. A. 112, 12956–12961. doi: 10.1073/pnas.1512090112
Almutairi, M. M., Svetlov, M. S., Hansen, D. A., Khabibullina, N. F., Klepacki, D., Kang, H. Y., et al. (2017). Co-produced natural ketolides methymycin and pikromycin inhibit bacterial growth by preventing synthesis of a limited number of proteins. Nucleic Acids Res. 45, 9573–9582. doi: 10.1093/nar/gkx673
Arenz, S., Bock, L. V., Graf, M., Innis, C. A., Beckmann, R., Grubmuller, H., et al. (2016). A combined cryo-EM and molecular dynamics approach reveals the mechanism of ErmBL-mediated translation arrest. Nat. Commun. 7:12026.
Arenz, S., Ramu, H., Gupta, P., Berninghausen, O., Beckmann, R., Vazquez-Laslop, N., et al. (2014). Molecular basis for erythromycin-dependent ribosome stalling during translation of the ErmBL leader peptide. Nat. Commun. 5: 3501.
Bader, M. S., Loeb, M., and Brooks, A. A. (2017). An update on the management of urinary tract infections in the era of antimicrobial resistance. Postgrad. Med. 129, 242–258. doi: 10.1080/00325481.2017.1246055
Bailey, M., Chettiath, T., and Mankin, A. S. (2008). Induction of erm(C) expression by noninducing antibiotics. Antimicrob. Agents Chemother. 52, 866–874. doi: 10.1128/aac.01266-07
Boerlin, P., Burnens, A. P., Frey, J., Kuhnert, P., and Nicolet, J. (2001). Molecular epidemiology and genetic linkage of macrolide and aminoglycoside resistance in Staphylococcus intermedius of canine origin. Vet. Microbiol. 79, 155–169. doi: 10.1016/s0378-1135(00)00347-3
Brisson-Noel, A., Arthur, M., and Courvalin, P. (1988). Evidence for natural gene transfer from gram-positive cocci to Escherichia coli. J. Bacteriol. 170, 1739–1745. doi: 10.1128/jb.170.4.1739-1745.1988
Chassy, B. M., and Thompson, J. (1983). Regulation of lactose-phosphoenolpyruvate-dependent phosphotransferase system and beta-D-phosphogalactoside galactohydrolase activities in Lactobacillus casei. J. Bacteriol. 154, 1195–1203. doi: 10.1128/jb.154.3.1195-1203.1983
Clarebout, G., Nativelle, E., and Leclercq, R. (2001). Unusual inducible cross resistance to macrolides, lincosamides, and streptogramins B by methylase production in clinical isolates of Staphylococcus aureus. Microb. Drug Resist. 7, 317–322. doi: 10.1089/10766290152773329
Dinos, G. P. (2017). The macrolide antibiotic renaissance. Br. J. Pharmacol. 174, 2967–2983. doi: 10.1111/bph.13936
Dzyubak, E., and Yap, M. N. (2016). The Expression of Antibiotic Resistance Methyltransferase Correlates with mRNA Stability Independently of Ribosome Stalling. Antimicrob. Agents Chemother. 60, 7178–7188. doi: 10.1128/aac.01806-16
Gryczan, T., Israeli-Reches, M., Del Bue, M., and Dubnau, D. (1984). DNA sequence and regulation of ermD, a macrolide-lincosamide-streptogramin B resistance element from Bacillus licheniformis. Mol. Gen. Genet. 194, 349–356. doi: 10.1007/bf00425543
Gupta, P., Kannan, K., Mankin, A. S., and Vazquez-Laslop, N. (2013). Regulation of gene expression by macrolide-induced ribosomal frameshifting. Mol. Cell 52, 629–642. doi: 10.1016/j.molcel.2013.10.013
Gupta, P., Liu, B., Klepacki, D., Gupta, V., Schulten, K., Mankin, A. S., et al. (2016). Nascent peptide assists the ribosome in recognizing chemically distinct small molecules. Nat. Chem. Biol. 12, 153–158. doi: 10.1038/nchembio.1998
Hue, K. K., and Bechhofer, D. H. (1992). Regulation of the macrolide-lincosamide-streptogramin B resistance gene ermD. J. Bacteriol. 174, 5860–5868. doi: 10.1128/jb.174.18.5860-5868.1992
Kamimiya, S., and Weisblum, B. (1988). Translational attenuation control of ermSF, an inducible resistance determinant encoding rRNA N-methyltransferase from Streptomyces fradiae. J. Bacteriol. 170, 1800–1811. doi: 10.1128/jb.170.4.1800-1811.1988
Kannan, K., Kanabar, P., Schryer, D., Florin, T., Oh, E., Bahroos, N., et al. (2014). The general mode of translation inhibition by macrolide antibiotics. Proc. Natl. Acad. Sci. U. S. A. 111, 15958–15963. doi: 10.1073/pnas.1417334111
Kannan, K., Vazquez-Laslop, N., and Mankin, A. S. (2012). Selective protein synthesis by ribosomes with a drug-obstructed exit tunnel. Cell 151, 508–520. doi: 10.1016/j.cell.2012.09.018
Kwak, J. H., Choi, E. C., and Weisblum, B. (1991). Transcriptional attenuation control of ermK, a macrolide-lincosamide-streptogramin B resistance determinant from Bacillus licheniformis. J. Bacteriol. 173, 4725–4735. doi: 10.1128/jb.173.15.4725-4735.1991
Leclercq, R., and Courvalin, P. (2002). Resistance to macrolides and related antibiotics in Streptococcus pneumoniae. Antimicrob. Agents Chemother. 46, 2727–2734. doi: 10.1128/aac.46.9.2727-2734.2002
Min, Y. H., Kwon, A. R., Yoon, E. J., Shim, M. J., and Choi, E. C. (2008). Translational attenuation and mRNA stabilization as mechanisms of erm(B) induction by erythromycin. Antimicrob. Agents Chemother. 52, 1782–1789. doi: 10.1128/aac.01376-07
Murphy, E. (1985). Nucleotide sequence of ermA, a macrolide-lincosamide-streptogramin B determinant in Staphylococcus aureus. J. Bacteriol. 162, 633–640. doi: 10.1128/jb.162.2.633-640.1985
Pontes, D. S., de Araujo, R. S. A., Dantas, N., Scotti, L., Scotti, M. T., de Moura, R. O., et al. (2018). Genetic Mechanisms of Antibiotic Resistance and the Role of Antibiotic Adjuvants. Curr. Top. Med. Chem. 18, 42–74. doi: 10.2174/1568026618666180206095224
Ramu, H., Vazquez-Laslop, N., Klepacki, D., Dai, Q., Piccirilli, J., Micura, R., et al. (2011). Nascent peptide in the ribosome exit tunnel affects functional properties of the A-site of the peptidyl transferase center. Mol. Cell 41, 321–330. doi: 10.1016/j.molcel.2010.12.031
Schroeder, M. R., and Stephens, D. S. (2016). Macrolide Resistance in Streptococcus pneumoniae. Front. Cell Infect. Microbiol. 6:98. doi: 10.3389/fcimb.2016.00098
Shaw, J. H., and Clewell, D. B. (1985). Complete nucleotide sequence of macrolide-lincosamide-streptogramin B-resistance transposon Tn917 in Streptococcus faecalis. J. Bacteriol. 164, 782–796. doi: 10.1128/jb.164.2.782-796.1985
Smith, C. J., and Pappano, A. J. (1985). A role for adenylate cyclase in the subsensitivity to isoproterenol during ontogenesis of the embryonic chick ventricle. J. Pharmacol. Exp. Ther. 235, 335–343.
Sothiselvam, S., Liu, B., Han, W., Ramu, H., Klepacki, D., Atkinson, G. C., et al. (2014). Macrolide antibiotics allosterically predispose the ribosome for translation arrest. Proc. Natl. Acad. Sci. U. S. A. 111, 9804–9809. doi: 10.1073/pnas.1403586111
Vazquez-Laslop, N., Thum, C., and Mankin, A. S. (2008). Molecular mechanism of drug-dependent ribosome stalling. Mol. Cell 30, 190–202. doi: 10.1016/j.molcel.2008.02.026
Ventola, C. L. (2015). The antibiotic resistance crisis: part 1: causes and threats. P T 40, 277–283.
Keywords: translation arrest, ribosome stalling, translational attenuation, erythromycin, leader peptide
Citation: Wang S, Jiang K, Du X, Lu Y, Liao L, He Z and He W (2021) Translational Attenuation Mechanism of ErmB Induction by Erythromycin Is Dependent on Two Leader Peptides. Front. Microbiol. 12:690744. doi: 10.3389/fmicb.2021.690744
Received: 04 April 2021; Accepted: 03 June 2021;
Published: 28 June 2021.
Edited by:
Santi M. Mandal, Indian Institute of Technology Kharagpur, IndiaReviewed by:
Ganesh Warthi, Augusta University, United StatesCopyright © 2021 Wang, Jiang, Du, Lu, Liao, He and He. This is an open-access article distributed under the terms of the Creative Commons Attribution License (CC BY). The use, distribution or reproduction in other forums is permitted, provided the original author(s) and the copyright owner(s) are credited and that the original publication in this journal is cited, in accordance with accepted academic practice. No use, distribution or reproduction is permitted which does not comply with these terms.
*Correspondence: Weizhi He, aGV3ZWl6aGlAdG9uZ2ppLmVkdS5jbg==; Zhiying He, enloZUB0b25namkuZWR1LmNu
†These authors have contributed equally to this work and share first authorship
Disclaimer: All claims expressed in this article are solely those of the authors and do not necessarily represent those of their affiliated organizations, or those of the publisher, the editors and the reviewers. Any product that may be evaluated in this article or claim that may be made by its manufacturer is not guaranteed or endorsed by the publisher.
Research integrity at Frontiers
Learn more about the work of our research integrity team to safeguard the quality of each article we publish.