- 1Quantitative and Systems Biology, University of California, Merced, Merced, CA, United States
- 2Sierra Nevada Research Institute, University of California, Merced, Merced, CA, United States
- 3Evolutionary Systems Biology of Symbionts, Institute for Integrative Systems Biology (I2SysBio), University of Valencia and Spanish Research Council (CSIC), Valencia, Spain
- 4Sequencing and Bioinformatics Service of the Foundation for the Promotion of Health and Biomedical Research of the Valencia Region (FISABIO), Valencia, Spain
- 5Department of Life and Environmental Sciences, University of California, Merced, Merced, CA, United States
The rapidly increasing global population and anthropogenic climate change have created intense pressure on agricultural systems to produce increasingly more food under steadily challenging environmental conditions. Simultaneously, industrial agriculture is negatively affecting natural and agricultural ecosystems because of intensive irrigation and fertilization to fully utilize the potential of high-yielding cultivars. Growth-promoting microbes that increase stress tolerance and crop yield could be a useful tool for helping mitigate these problems. We investigated if commercially grown almonds might be a resource for plant colonizing bacteria with growth promotional traits that could be used to foster more productive and sustainable agricultural ecosystems. We isolated an endophytic bacterium from almond leaves that promotes growth of the model plant Arabidopsis thaliana. Genome sequencing revealed a novel Erwinia gerundensis strain (A4) that exhibits the ability to increase access to plant nutrients and to produce the stress-mitigating polyamine spermidine. Because E. gerundensis is known to be able to colonize diverse plant species including cereals and fruit trees, A4 may have the potential to be applied to a wide variety of crop systems.
Introduction
Climate change and the growing world population create increasing pressure for agricultural systems to produce sufficient food (United Nations, 2019). To compensate for this demand, modern agriculture uses high-yielding cultivars and applies abundant irrigation water, fertilizers, and pesticides (Martinho, 2020). Irrigation water is not only a limited resource, but its excessive use can also negatively impact yield and the environment. For example, intensive irrigation can lead to loss of fertile soils and increased soil salinity (Dale and Polasky, 2007). Additionally, these surface runoffs can contaminate surface water as well as groundwater (Dale and Polasky, 2007; Martinho, 2020).
Almonds are an example of a high demanding agricultural product (Zhang et al., 2019). They are important for diverse culinary cultures around the world, and their consumption can improve cholesterol and blood sugar levels (Li et al., 2011; Ortiz et al., 2012; Choudhury et al., 2014; Berryman et al., 2015). These positive attributes among others have led to a high market demand that requires large fertilizer inputs and ample amounts of water usage to fully utilize the yield potential of almond trees (Zhang et al., 2019). For example, almond tree irrigation requirements are high, with estimates of 12 L of water to produce a single almond kernel (Fulton et al., 2019). To mitigate the direct and indirect negative impacts of modern agriculture on natural and agroecosystems, more sustainable strategies are required. Utilizing bacteria could be a possible approach to counter the negative impacts of modern agriculture (Compant et al., 2010). Diverse bacteria living in association with plants are able to increase the stress tolerance and nutrient availability of their host and, therefore, could reduce the need for irrigation and fertilizer inputs (Sturz et al., 2000; Lodewyckx et al., 2002; Ryu et al., 2004; Chen et al., 2010; Yandigeri et al., 2012; Timmusk et al., 2014; Soares et al., 2016; Verma et al., 2018).
In many soil types, negatively charged forms of phosphorus (P) are attached to cations, such as iron, aluminum, and calcium (Hinsinger, 2001). Microbes are crucial for making this P availability for plants. For example, bacterial enzymes can increase in the proton concentration of the soil via the production of organic acids, and this pH change solubilizes inorganic P (Kafle et al., 2019). One example is the bacterial enzyme glucose dehydrogenase that is encoded by the gcd gene and which uses glucose for the synthesis of the organic acid gluconic acid (Goldstein, 1995; Liang et al., 2020). This enzyme requires the bacterial redox active cofactor pyrroloquinoline quinone (PQQ), which is produced by six Pqq proteins (Anthony, 2001; Shen et al., 2012). The presence of gcd and the pqq cluster indicates the bacterial strains’ ability to lower the pH of alkaline soils in order to increase availability of phosphorous and iron. Furthermore, bacteria are able to produce siderophores, low-molecular-mass molecules with a high affinity for iron (Fe; Richardson et al., 1999). For example, enterobactin has an exceptionally high affinity for Fe3+ (Ka = 1052) and is synthesized by several enzymes encoded by ent genes (Avdeef et al., 1978; Raymond et al., 2003; McRose et al., 2018). Several studies have shown that plants are able to access Fe by the uptake of microbial siderophores (Bar-Ness et al., 1992; Vansuyt et al., 2007; Jin et al., 2010; Shirley et al., 2011).
Plants synthesize low-molecular-mass linear polyamines, like spermidine, that are essential for plant growth (Kusano et al., 2008). Supplemental spermidine, provided either by overexpression of spermidine synthetase or exogenous application, enhances plant defense responses and increases tolerance to diverse abiotic stresses, like salinity and drought (Yoda et al., 2003; Kasukabe et al., 2004; Moschou et al., 2009). Overall, these findings suggest that increasing spermidine could improve plant tolerance to diverse environmental stresses. Bacteria are a potential source for providing supplemental spermidine to plants (Xie et al., 2014). They can synthesize spermidine from the two amino acids, methionine and arginine, catalyzed by enzymes encoded by the metK and spe genes (Tabor and Tabor, 1985; Shah and Swiatlo, 2008; Guerra et al., 2018).
To identify potential resources for a more sustainable agriculture, the primary goal of this study was to isolate and characterize a growth-promoting bacterial endophyte from almond leaves that carries genes to improve nutrient availability and plant stress tolerance. We successfully isolated a growth-promoting bacterium, and whole genome sequencing analysis identified this bacterium as novel strain of Erwinia gerundensis. This bacterial species has been found in diverse agricultural ecosystems around the globe. This suggests that the bacterial strain described here has a potential use not only in almonds but also for a wide variety of other crops.
Materials and Methods
Sample Collection and Leaf Tissue Sterilization
Leaves of visually healthy almond trees growing in an orchard located in Modesto, California (37°42′21.8″N, 120°56′55.1″W) were collected aseptically in July 2019. Leaves were placed in sterile bags and immediately stored at 4°C in a portable cooler and then transported to the laboratory at the University of California, Merced. Within 3 h after collection, leaves were surface sterilized using 8.25% sodium hypochlorite and afterward rinsed twice with sterile water. Removal of epiphytic microbes was confirmed using scanning electron microscopy (SEM; Figure 1). Surface sterilization and SEM procedures were previously described for cottonwood leaves (Saldierna Guzmán et al., 2020).
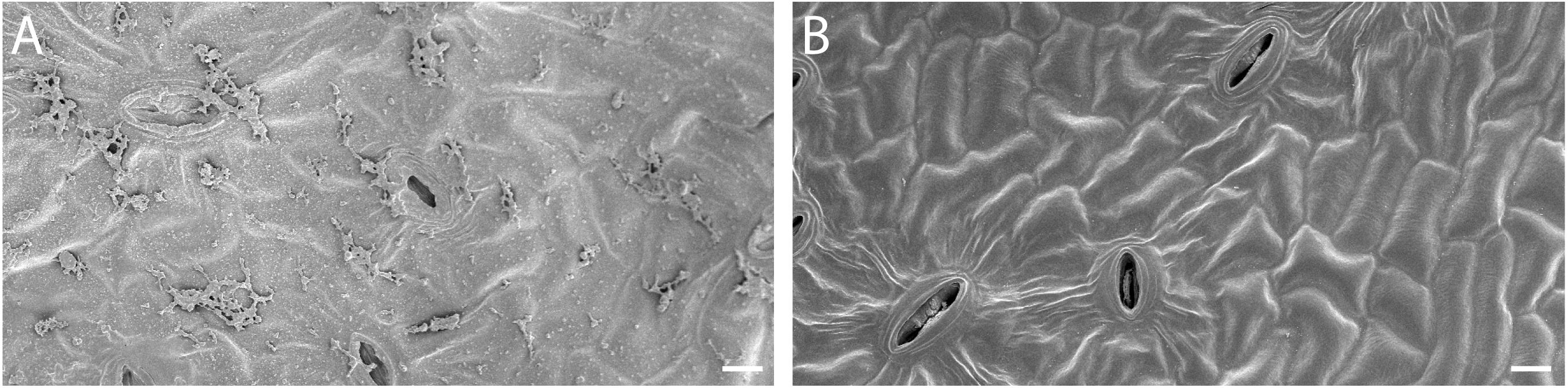
Figure 1. Removal of surface microbes from almond leaves (A) Before and (B) after surface sterilization. Scale bar = 10 μm.
Enrichment and Isolation of Endophytic Bacterial Isolates
Immediately after removing epiphytic microbes, 20 g of surface sterilized leaves were blended using bacterial cell extraction buffer (50 mM Tris–HCl [pH7.5], 1% Triton X-100, 2 mM β-mercaptoethanol) for 3 min to enrich for endophytic bacterial cells (Ikeda et al., 2009). We found that filtering through Miracloth and centrifugation at 500 × g was sufficient to remove plant cell debris. Therefore, we excluded the Nycodenz density gradient centrifugation described in Ikeda et al. (2009). The enriched endophytic bacterial cells were resuspended using 1x phosphate-buffered saline (PBS). Ten microliters of this suspension was plated on Lysogeny Broth (LB) agar media (Bertani, 1951) or on Norris Glucose Nitrogen Free Media (HIMEDIA, Mumbai, Maharashtra, India), and then incubated 5–7 days at 28°C. Subsequently, the obtained colonies were repeatedly streaked and incubated on LB agar media (Bertani, 1951) to obtain pure isolates. A total of 100 bacterial isolates were obtained from surface-sterilized almond leaves. Of these bacteria, 22 isolates were randomly selected and tested for their plant growth-promoting abilities (see below under “Plant inoculation”). Only one of these 22 isolates, named A4, was chosen for its plant growth-promoting ability.
In order to identify the isolates, 16S rRNA gene amplification of each sample was conducted using universal primers 27f and 1492r (Lane, 1991). The 50-μl PCR contained 1x complete reaction buffer, 200 μM dNTPs, 0.5 μM forward primer, 0.5 μM reverse primer, 10 ng of template DNA, and 1.25 U DFS-Taq DNA polymerase (BIORON GmbH, Römerberg, Rheinland-Pfalz, Germany). Cycling conditions were 95°C for 2 min, followed by 25 cycles of 95°C for 30 s, 55°C for 30 s, 72°C for 2 min, with a final extension of 72°C for 10 min. All samples were purified from 1.5% agarose gels using the Zymoclean Gel DNA Recovery Kit (Zymo research, Irvine, CA, United States) following the recommended procedure of the manufacturer. Subsequently, amplicons were sent for Sanger sequencing at Eton Bioscience (San Diego, CA, United States). The Sanger sequencing results have been uploaded to the NCBI database and can be found under the GenBank accession numbers MZ227353–MZ227374.
Competent Cells and Transformation of Endophytic A4 Bacterial Strain
In order to transform the strain A4 with a plasmid that carries maker genes for visualization of A4 in plant tissues, competent cells were generated. For this purpose, A4 cells were grown overnight at 28°C until they reached an optical density (OD600) of 0.5. They were cooled in an ice bath and washed with sterile, cold water four times after centrifugation steps at 4,000 × g. Afterward, cells were resuspended in 10% glycerol, aliquoted, and snap frozen in liquid nitrogen. Electroporation was used for transformation (Cadoret et al., 2014) of A4 with the pRU1156 plasmid, Addgene catalog 14473 (Karunakaran et al., 2005).
Western Blotting
Transformed and untransformed A4 strains were grown on LB liquid media overnight at 28°C. Bacterial suspensions were normalized by measuring OD600 of 0.1, and equal amounts of cells were boiled in sample loading buffer (Sigma-Aldrich, Darmstadt, Germany) and separated in SDS-PAGEs. For immunoblotting, anti-GFP (1:5,000, Roche, 11814460001, Darmstadt, Germany) was used to detect free GFP (Bürger et al., 2017).
Plant Inoculation
Arabidopsis thaliana (ecotype Columbia-0) seeds were surface sterilized by hydrochloric fumigation for 3 h and stratified for 3–5 days at 4°C (Lindsey et al., 2017). Subsequently, the sterile seeds were grown in vitro on half-strength Linsmaier and Skoog (LS) medium at pH 5.7 with 7% plant agar. Seedlings were grown on vertically oriented plates in a growth chamber (Percival, Iowa, United States) with continuous white light (100 μmol m– 2 s– 1) at 21°C (Qiu et al., 2019). Prior to inoculation, the 22 selected endophytic isolates were grown for 24 h in LB media with 50 μg tetracycline ml– 1 and 100 μg ampicillin ml– 1 at 28°C. The bacterial cells were centrifuged at 3,500 × g, resuspended in infiltration buffer (10 mM MgSO4, 10 mM MES-KOH pH 5.5), and adjusted to an OD600 of 0.1 in the same buffer. Subsequently, the roots of 7-days old seedlings were inoculated with 2–5 μl of bacterial inoculum per root, being careful not to contaminate shoot tissues, following the protocol for Salmonella enterica and Escherichia coli O157:H7 (Cooley et al., 2003). Infiltration buffer without bacteria served as the negative control (mock treatment).
Plant Growth Promotion Assay
The effect of the 22 bacterial isolates on Arabidopsis growth was evaluated 2 weeks postinoculation. Three independent experiments were performed with 38–41 seedlings per treatment in each experiment. Fresh mass of seedlings was measured and compared to mock (infiltration buffer)-treated plants, which served as negative control. Statistical analysis was performed by one-way ANOVA with Tukey’s test using GraphPad Prism 7 (GraphPad Software, San Diego California, United States). A difference was considered statistically significant using an a priori determined α level of 0.05.
GUS and Green Fluorescent Protein (GFP)
In order to test colonization of the endophytic strain A4, plant tissues were histochemically stained with X-Gluc (GUS) 7 days after inoculation of A. thaliana seedlings and observed with a stereomicroscope (Leica MZ16, Leica, Germany; Willige et al., 2011). Moreover, seedlings were screened for GFP expression using a Zeiss LSM 710 fluorescence microscope (Carl Zeiss, Germany).
Genome Sequencing
The bacterial strain A4 was sent to Novogene Biotech (Beijing, China) for DNA extraction, library preparation, and whole genome sequencing and assembly using PacBio Single Molecule, Real-Time (SMRT) Sequencing (Eid et al., 2009). Falcon software (Chin et al., 2016) was used for genome assembly, and BUSCO for the assessment of the genome assembly, gene set, and transcriptome completeness (Simão et al., 2015). Prokka software was used for genome annotation (Seemann, 2014), and functional annotation was done by aligning the sequence with sequences previously deposited in diverse protein databases, including the National Center for Biotechnology Information (NCBI) non-redundant protein (Nr) database, UniProt/Swiss-Prot, Kyoto Encyclopedia of Genes and Genomes (KEGG), and Cluster of Orthologous Groups of proteins (COG).
Bioinformatics
The phylogenetic tree was constructed with SpeciesTreeBuilder v 0.1.0 from the Kbase platform (Arkin et al., 2018). The Artemis Comparison Tool (ACT; Carver et al., 2005) was used to compare our bacterium genome with an already sequenced genome. To display circular comparisons between both genomes and plasmids, the Blast Ring Image Generator (BRIGS) was used (Alikhan et al., 2011). Finally, a nucleotide identity analysis between strain A4 and a publicly available, closely related bacterial genome was performed with the ANI calculator from EZbiocloud (Yoon et al., 2017).
Phosphate Solubilization
Strain A4 was tested for its ability to solubilize phosphate using NBRIP medium (Nautiyal, 1999). Prior to inoculation, strain A4 was grown at 28°C until they reached an OD600 of 0.5. An aliquot of 10 μl was spotted on plates containing National Botanical Research Institute’s Phosphate growth medium (NBRIP; Nautiyal, 1999) and incubated at 28°C for 7 days.
Siderophore
Siderophore production was determined by chrome azurol S (CAS) agar plates. The medium was prepared according to the method described by Schwyn and Neilands (1987). Strain A4 was grown overnight in LB liquid medium at 28°C. A4 bacterial suspension was normalized by adjusting to an OD600 of 0.5 with LB medium. Subsequently, 10 μl of the suspension was spotted on CAS agar plates and incubated for 7 days at 28°C.
Results
Isolation, Characterization, and Effect of A4 Endophyte on Plant Growth
Twenty-two strains were isolated from surface-sterilized almond leaves (Figure 1). They were tested for their plant growth-promoting ability, and only the strain A4 had a positive effect on plant growth. Therefore, A4 was selected for further characterization. Sanger sequencing of the 16S rRNA gene revealed its phylogenetic relationship to the genera Pantoea and Erwinia. Three independent experiments revealed that endophyte-treated plants had about 30% higher fresh mass than control plants (p ≤ 0.001; Figures 2A,B). Additionally, the A4 treatment resulted in increased root hair length and abundance (Figures 2C,D). These results suggest that inoculation with an almond-derived endophyte could promote growth of Arabidopsis seedlings.
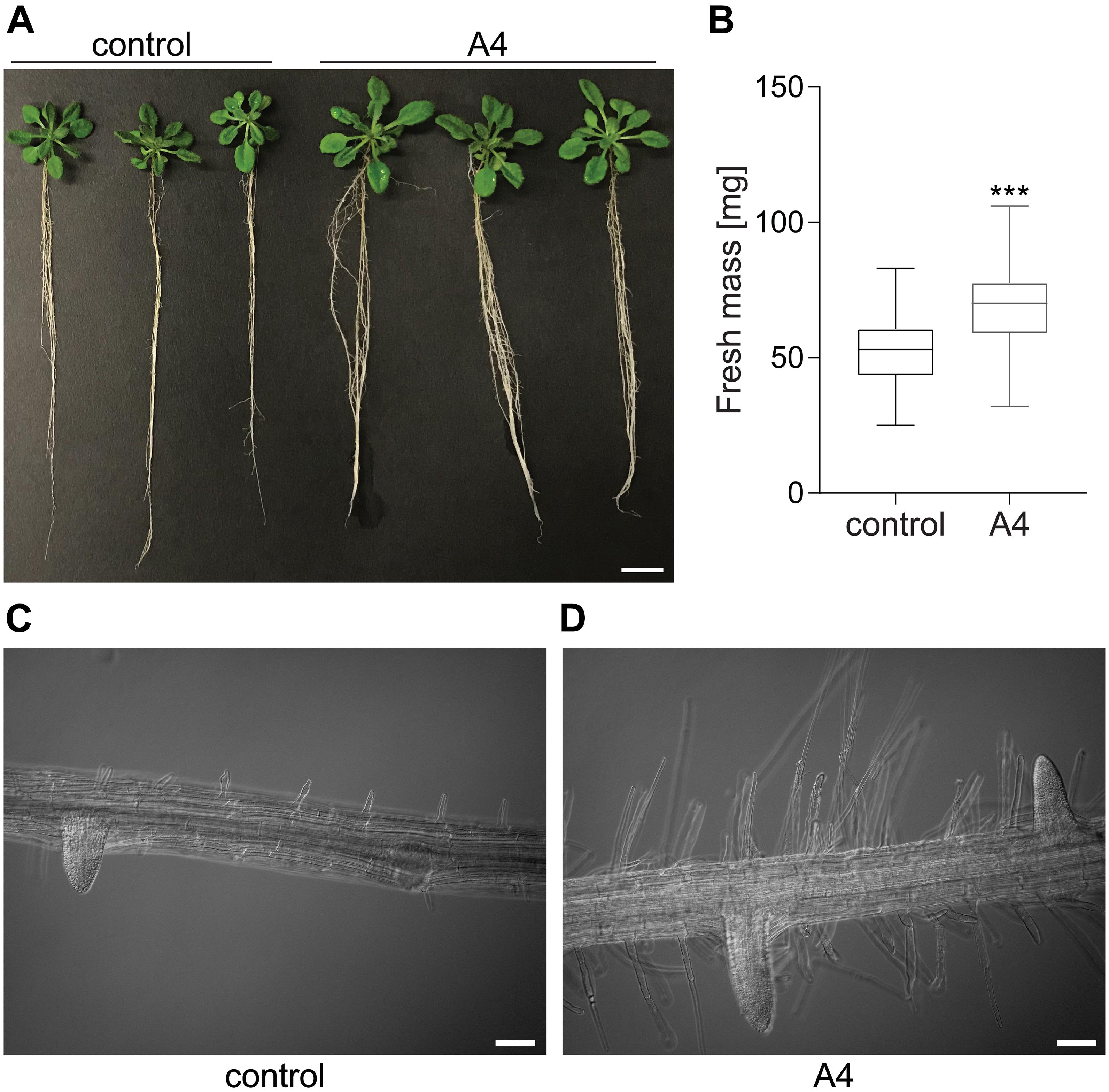
Figure 2. Effect of endophytic strain A4 on Arabidopsis thaliana (A) Photo taken after 2 weeks postinoculation in comparison to the control. Scale bar = 1 cm. (B) Fresh mass of seedlings compared to the control (one-way ANOVA, Tukey’s multiple comparisons test, *** p ≤ 0.001), n = 41. (C,D) Differences in the root after 2 weeks of inoculation with A4 in contrast to the control. Scale bar = 0.2 mm. Control for all experiments: mock (infiltration buffer)-treated plants.
Transformation of Strain A4
To test plant colonization by A4, we transformed A4 to express the marker genes GFP and gusA in order to track the colonization of A4 within internal plant tissues. Successful transformation of A4 was confirmed by Western blotting to visualize expression of GFP encoded in the transformed plasmid (Figure 3A).
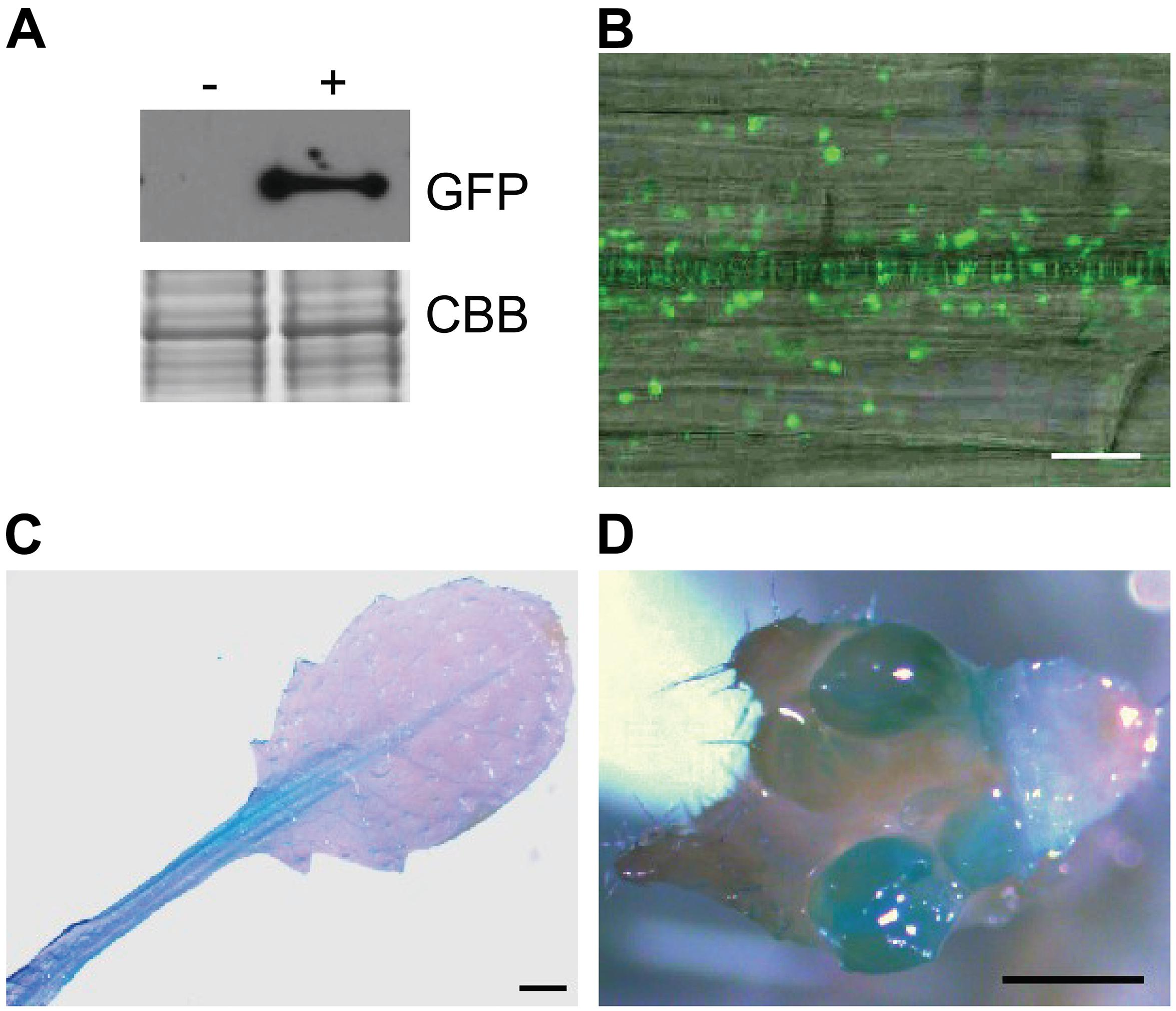
Figure 3. Colonization of the endophytic strain A4 (A) Western blot analysis of A4 transformant expressing GFP. Coomassie stain (CBB) shows equivalent protein loading. An antibody against GFP was used for visualization, and non-transformant A4 strain as a negative control. Seven days after inoculation of Arabidopsis thaliana seedlings, (B) GFP-expressing A4 inside the primary root. Scale bar = 20 μm. Histochemical GUS activity of A4 strain in (C) leaf tissue and (D) inflorescence. Scale bar = 1 mm.
Arabidopsis roots were inoculated with endophytic bacteria A4 labeled with GFP to determine whether intracellular colonization was occurring by live-cell imaging. Confocal observation revealed GFP-expressing A4 colonizing internal root tissues 7 days after inoculation. Many of these bacterial cells were observed within the root vasculature (Figure 3B). Furthermore, analyses 14 days postinoculation showed that A4 had colonized shoot tissues such as leaves and flowers, as visualized by GUS staining (Figures 3C,D). These observations indicated that the root-inoculated bacteria were able to spread inside the plant and colonize above ground tissues. The mock-inoculated plants did not show any blue staining or fluorescent bacteria.
Genomic Features of A4
The whole-genome sequence analysis of A4 was conducted in order to obtain reliable taxonomic classification and identify genes or pathways that could potentially contribute to the plant growth-promoting effects. The A4 genome consisted of a single circular chromosome of 3,858,052 base pairs (bp), with an average GC content of 55%. The A4 strain had one plasmid we named pA401, with a size of approximately 576,382 bp, and also had an average GC content of 55% like the chromosome. The chromosome contained 3,552 genes, including genes for 78 tRNAs, for 22 rRNAs, and 3,451 protein-coding sequences (CDS). Among these CDSs, 2,929 genes were classified into clusters of orthologous group (COG) families composed of 23 categories. KEGG pathway annotation resulted in the functional annotation of 3,414 genes (96%). Of these annotated genes, most were grouped into the two categories: “metabolism” and “environmental information processing” (Table 1). The A4 plasmid pA401 had 521 protein-coding sequences and 1 contig.
Taxonomic Affiliation of Strain A4
We used Tree builder to search for closely related genomes and to assess the phylogenetic relationship between A4 and publicly available genomes (Figure 4 and Supplementary Table 1). This analysis revealed that A4 grouped separately from strains of Pantoea, but together with Erwinia strains and in particular very next to E. gerundensis strain EM595 (Rezzonico et al., 2016). Therefore, we compared chromosomes and plasmids of A4 and EM595 (Figure 5). While the chromosomes of both strains share about 90% of their genes (3175), A4 had 224 unique genes (Supplementary Table 2). Furthermore, EM595 carried two plasmids (pEM01 and pEM02), and pEM01 shared the greatest overlap with pA401 (71%, 463 genes); 20 of the common genes were also present on pEM02. Additionally, the A4 plasmid had 48 unique genes that were not present in both plasmids of the previously sequenced Erwinia strain EM595 (Supplementary Table 3). Taken together, these results suggest A4 represents a novel E. gerundensis strain.
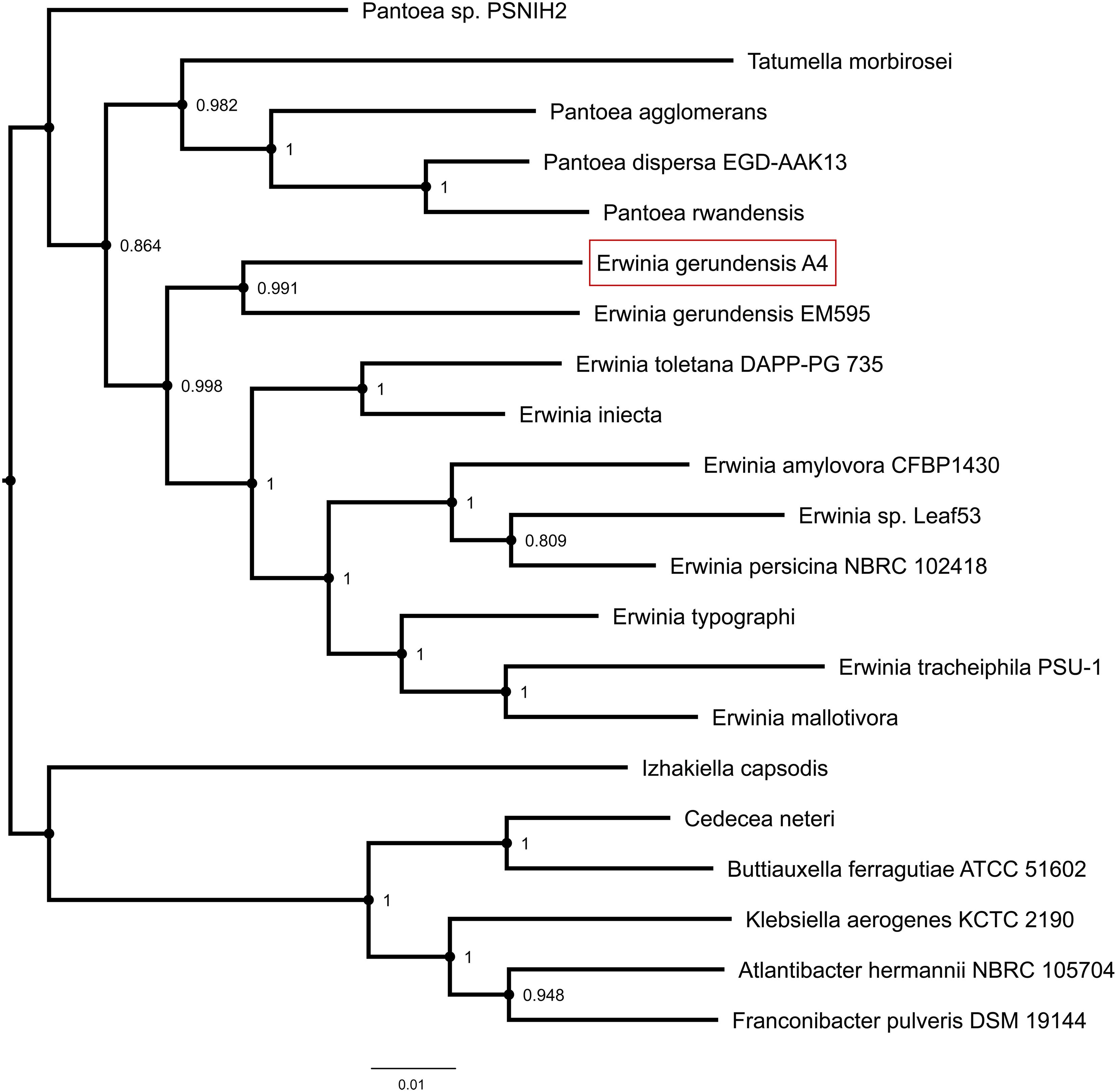
Figure 4. Phylogenetic tree highlighting the position of E. gerundensis strain A4 in comparison to publicly available genomes. Node labels represent confidence levels based on 1,000 bootstrap replicates.
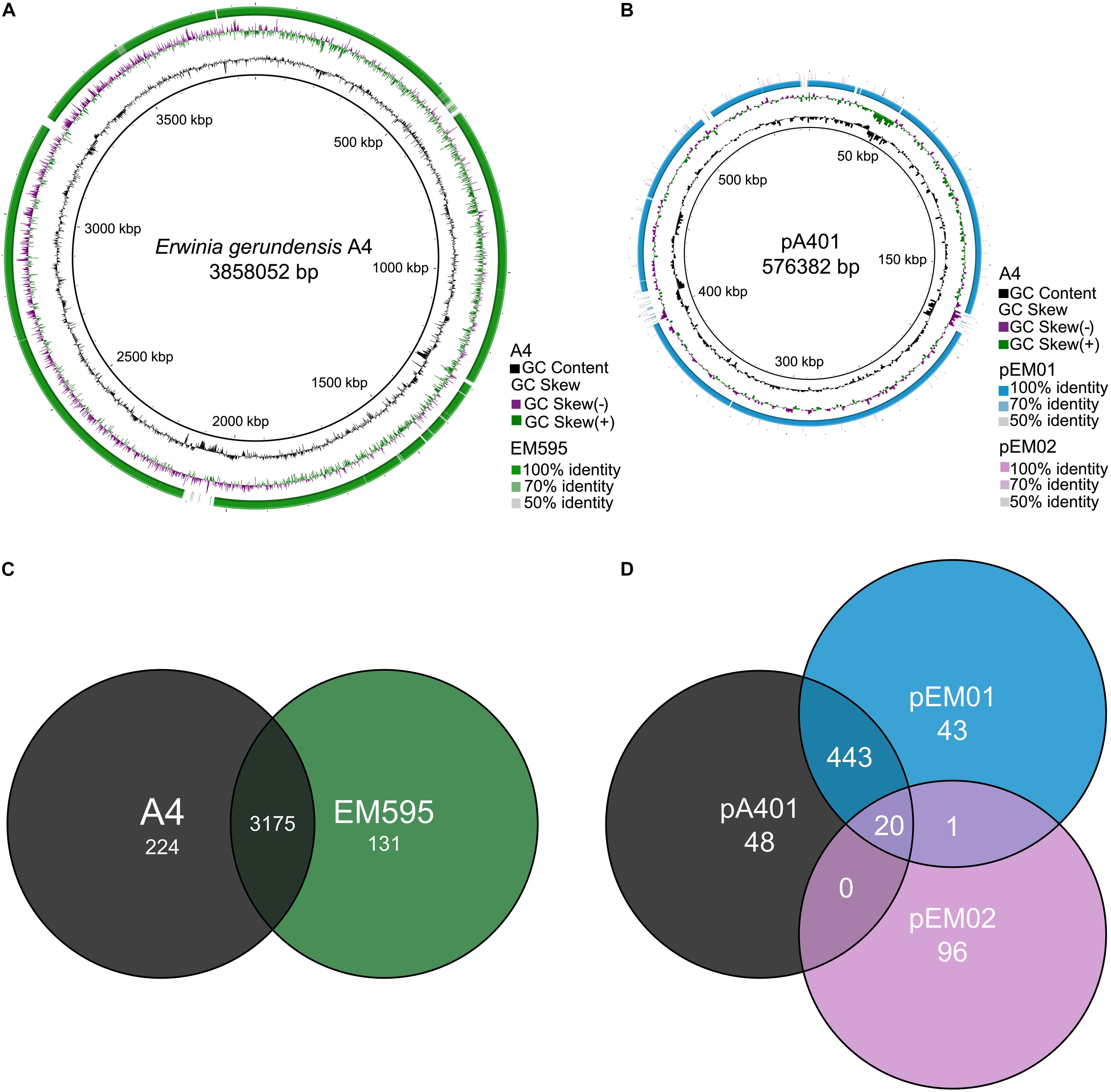
Figure 5. Comparison between E. gerundensis strain A4 and EM595. (A,B) Circular comparisons between both genomes and plasmids. The inner black rings depict the coordinates in scale and total size of chromosome (A) and plasmid (B) of A4. Black histograms represent GC content, while green-purple histograms show GC deviations. Orthologous sequences are displayed with the percentage of similarity. (C,D) Venn diagrams of chromosomes and plasmids of both strains.
Plant Growth-Promoting Traits in A4
In order to identify genes responsible for growth promotion, we searched the genome and plasmid of A4 for genes involved in nitrogen fixation, and organic acid, siderophore, and spermidine synthesis. Even though A4 was isolated on bacterial medium that is denoted as nitrogen free, we could not detect any genes encoding for nitrogenase subunits. However, we identified a pqq gene cluster (pqqABCDEF) as well as for a gcd gene, indicating that A4 carries all necessary enzymatic components to solubilize phosphorus by organic acid synthesis. Moreover, we found entABCDEF and two entS genes in A4, suggesting that this strain is able to produce and export the siderophore enterobactin. Furthermore, the strain carries all necessary genes (i.e., metK and speABDE) to produce the polyamine spermidine. In addition, we identified several genes involved in spermidine and putrescine transport (i.e., potA, potB, potD, potG, potH, and potI; Figure 6).
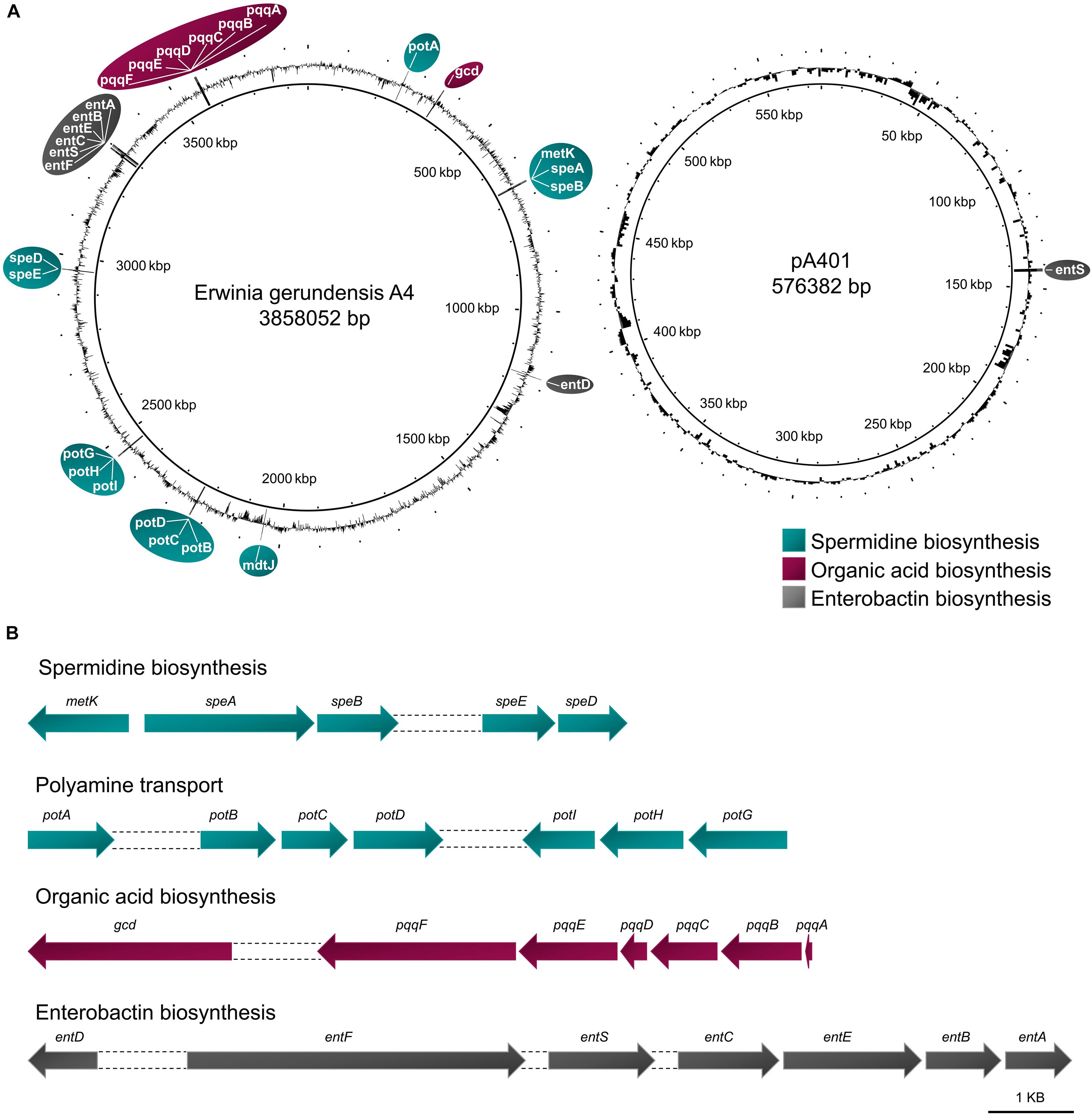
Figure 6. Genes encoding for plant-promoting traits in E. gerundensis strain A4. (A) Location and (B) cluster of genes encoding production and transport of spermidine, the phosphate-solubilizing gluconic acid, and the siderophore enterobactin.
Nutrient Acquisition by A4 Strain
Because the genome of A4 encodes for proteins that are involved in the production of organic acids and siderophores, we tested if A4 is able to release insoluble phosphate and synthesize iron chelators. A4 was able to produce a clear zone around the colony, suggesting phosphate solubilization activity by the production of organic acids (Figure 7A). Additionally, production and secretion of siderophores were visualized by a color change of Chrome Azurol S (CAS). In association with ferric ions, CAS appears blue, while the removal of the ferric ions by siderophores changes the color of the media from blue to yellow. Consistent with the presence of genes encoding for siderophore-synthesizing enzymes, A4 was able to cause the color change of the CAS media (Figure 7B). In contrast, a non-growth-promoting Frigoribacterium strain that we isolated from almond leaves was not able to solubilize phosphate or induce a yellow color of the CAS media (Supplementary Figure 1). This indicates that A4 could supply its plant host with essential nutrients such as phosphate and iron to promote growth.
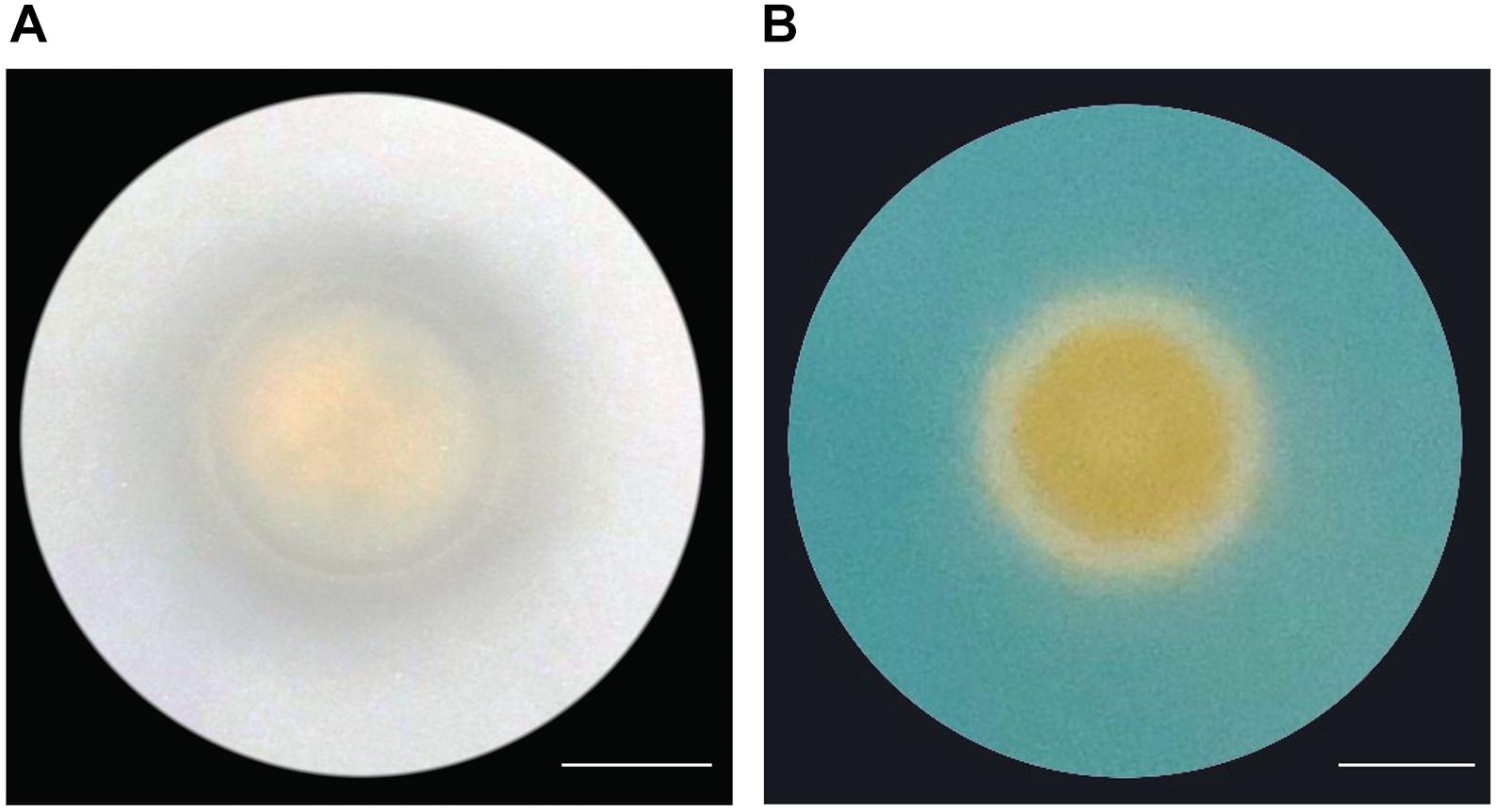
Figure 7. Nutrient acquisition abilities by strain A4 (A) Phosphate solubilization by A4. Clear zone surrounding the bacterial colony represents solubilization of Ca3(PO4)2. (B) Siderophore production in CAS medium. The color change from blue to yellow caused by siderophore production can be observed surrounding the bacterial colony. Scale bars = 10 mm.
Discussion
Bacteria living in association with plants can either be harmful, neutral, or beneficial. Once a beneficial bacterium has been established on or in a plant, it can influence the physiology of the host via a variety of mechanisms. For example, bacteria can produce growth-promoting metabolites, easing the effects of biotic and abiotic stresses and providing nutrients (Bulgarelli et al., 2013). Here, we described the isolation, characterization, and sequencing of the growth-promoting E. gerundensis strain A4. This strain was isolated from inner leaf tissues from almonds growing in a commercial orchard. So far, only one other E. gerundensis strain has been sequenced, which was isolated from leaf surfaces of a pear tree (Rezzonico et al., 2016). Our sequence analysis identified A4 as a novel E. gerundensis strain. Furthermore, whole genome sequencing revealed that A4 exhibits the ability to produce polyamines, gluconic acid, and enterobactin.
Polyamines, like spermidine, are organic compounds produced by prokaryotes and eukaryotes that are essential for their growth and development (Kusano et al., 2008). In plants, they play a role in a variety of process like embryogenesis, root growth, flowering, fruit maturation, and retardation of senescence (Evans and Malmberg, 1989; Pandey et al., 2000). For example, in Arabidopsis thaliana, loss of spermidine synthase activity leads to embryo lethality (Imai et al., 2004). Other than participating in plant growth, polyamines have a protective role against several environmental stresses. Kasukabe et al. (2004) showed that overexpression of plant-derived spermidine synthase confers tolerance to freezing, salinity, drought, and osmotic stresses in Arabidopsis thaliana. Additionally, several studies have shown that polyamines are involved in defense responses against pathogens. The upregulated expression of polyamine synthesis genes in response to pathogenic attacks has been observed in several plant species (Fu et al., 2011; Moselhy et al., 2016). Furthermore, overexpression of spermidine synthase or exogenous application of spermidine enhances plant defense responses to viruses and bacteria (Yoda et al., 2003; Moschou et al., 2009). Altogether, these studies indicate that increasing polyamine levels minimizes the detrimental effects caused by biotic and abiotic stresses. A4 could provide spermidine and ease the damaging effects of various environmental stresses, because it carries the genes not only for spermidine synthesis but also for the export of the polyamines spermidine and putrescine.
Besides spermidine production, we identified several traits that may support nutrient acquisition of plants. For example, at a morphological level, A4 increases root growth and the elongation and density of root hairs in Arabidopsis. These plant growth responses increase root surface area that can enhance the access to water and the uptake of nutrients of the plant (Sukumar et al., 2013). Furthermore, the genome of A4 encodes for two enzymatic pathways involved in nutrient acquisition. Microbes developed diverse metabolic capacities to improve the bioaccessibility of recalcitrant P by the production of different organic acids (Richardson and Simpson, 2011). Bacterial PQQ-dependent glucose dehydrogenase, encoded by the gcd gene, catalyzes the oxidation of glucose to gluconic acid (Goldstein, 1995; Liang et al., 2020). Glucose dehydrogenase is characterized as a key enzyme necessary for phosphate solubilization in microbes. In fact, detection of the gcd gene in soil samples was found to be a major determinant of bioavailable P (Liang et al., 2020). Our genomic analysis of A4 identifies genes encoding for the entire enzymatic pathway for synthesis of the redox cofactor PQQ and for gluconic acid production. We found that A4 is able to solubilize Ca3(PO4)2, suggesting that gluconic acid is excreted by A4 to lower the pH of alkaline environments. In contrast, low soil pH leads to P fixation by iron. In acidic soils, bacteria can increase the phosphate accessibility for plants by producing siderophores that form complexes with ferric iron (Kafle et al., 2019). The genome and plasmid of A4 carry all necessary genetic information to produce and export the siderophore enterobactin. Our in vitro assay suggested that ent genes of A4 are all functional. Several studies have demonstrated that plants are able to access Fe by the uptake of microbial siderophores (Bar-Ness et al., 1992; Vansuyt et al., 2007; Jin et al., 2010; Shirley et al., 2011). This indicates that siderophores produced by growth-promoting bacteria have a dual function for the nutrient availability of the plants in acidic soils by providing phosphate as well as iron. Therefore, A4 could exhibit plant growth promotion traits not only as a leaf endophyte where it could provide spermidine to foster stress tolerance, but also as a member of the plant rhizospheres to ease access to nutrients. Furthermore, we observed floral colonization by A4. This opens the possibility that A4 might colonize reproductive tissues and may suggest that A4 might be vertically transmitted from one generation to the next.
We isolated E. gerundensis strain A4 from inner tissues of almond leaves and showed that A4 is able to colonize below and above ground tissues of Arabidopsis thaliana. Other E. gerundensis strains were found in diverse agroecosystems around the globe: Two strains were isolated from leaf surfaces of pome fruit trees in Spain, and two other strains were isolated from wheat roots in Australia (Rezzonico et al., 2009, 2016). Taken together, these results suggest that E. gerundensis can colonize different tissues of various plant species, including both monocots and dicots, in diverse agricultural environments. This promiscuous colonization behavior and the growth promotion traits of E. gerundensis A4 suggest that this strain might have the potential to improve production not only of almonds but also of a variety of other crop species around the globe.
Data Availability Statement
The sequencing data was deposited to GenBank database under the BioProject PRJNA717909.
Author Contributions
JSG and SH designed the research and wrote the manuscript. JSG performed the experiments. JSG and MR-P analyzed the data. All authors revised the manuscript and approved the final version.
Funding
This work was supported by UC MEXUS-CONACYT Doctoral Fellowship (to JSG), Yara North America Almond Fellowship (to JSG), USDA HSI Education Training program 2017-38422-27227 (to JSG), and NASA MIRO grant no. NNX15AQ01A (to the Imaging and Microscopy Facility, UC Merced).
Conflict of Interest
The authors declare that the research was conducted in the absence of any commercial or financial relationships that could be construed as a potential conflict of interest.
Publisher’s Note
All claims expressed in this article are solely those of the authors and do not necessarily represent those of their affiliated organizations, or those of the publisher, the editors and the reviewers. Any product that may be evaluated in this article, or claim that may be made by its manufacturer, is not guaranteed or endorsed by the publisher.
Acknowledgments
We would like to thank Björn C. Willige for his assistance with sample collection and bacterial isolation. Roger A. Duncan for giving us access to the field site, Robert Longstreth for allowing us to work in his orchard, Rudy M. Ortiz, Miriam Barlow, and Clarissa J. Nobile for use of laboratory equipment, and the Duarte Nursery and Alma Cruz for providing us with almond seedlings to establish our protocols.
Supplementary Material
The Supplementary Material for this article can be found online at: https://www.frontiersin.org/articles/10.3389/fmicb.2021.687971/full#supplementary-material
Supplementary Figure 1 | Non-growth-promoting strain Frigoribacterium strain isolated from almond leaves was not able to solubilize phosphate (A) or induce a yellow color of the CAS media (B). Scale bars = 10 mm.
References
Alikhan, N.-F., Petty, N. K., Ben Zakour, N. L., and Beatson, S. A. (2011). BLAST ring image generator (BRIG): simple prokaryote genome comparisons. BMC Genomics 12:402. doi: 10.1186/1471-2164-12-402
Anthony, C. (2001). Pyrroloquinoline quinone (PQQ) and quinoprotein enzymes. Antioxid. Redox Signal. 3, 757–774. doi: 10.1089/15230860152664966
Arkin, A. P., Cottingham, R. W., Henry, C. S., Harris, N. L., Stevens, R. L., Maslov, S., et al. (2018). KBase: the United States department of energy systems biology knowledgebase. Nat. Biotechnol. 36, 566–569. doi: 10.1038/nbt.4163
Avdeef, A., Sofen, S. R., Bregante, T. L., and Raymond, K. N. (1978). Coordination chemistry of microbial iron transport compounds. 9. Stability constants for catechol models of enterobactin. J. Am. Chem. Soc. 100, 5362–5370. doi: 10.1021/ja00485a018
Bar-Ness, E., Hadar, Y., Chen, Y., Shanzer, A., and Libman, J. (1992). Iron uptake by plants from microbial siderophores. Plant Physiol. 99, 1329. doi: 10.1104/pp.99.4.1329
Berryman, C. E., West, S. G., Fleming, J. A., Bordi, P. L., and Kris-Etherton, P. M. (2015). Effects of daily almond consumption on cardiometabolic risk and abdominal adiposity in healthy adults with elevated LDL-cholesterol: a randomized controlled trial. J. Am. Heart Assoc. 4:e000993. doi: 10.1161/JAHA.114.000993
Bertani, G. (1951). Studies on lysogenesis. I. The mode of phage liberation by lysogenic Escherichia coli. J. Bacteriol. 62, 293–300. doi: 10.1128/JB.62.3.293-300.1951
Bulgarelli, D., Schlaeppi, K., Spaepen, S., van Themaat, E. V. L., and Schulze-Lefert, P. (2013). Structure and functions of the bacterial microbiota of plants. Annu. Rev. Plant Biol. 64, 807–838. doi: 10.1146/annurev-arplant-050312-120106
Bürger, M., Willige, B. C., and Chory, J. (2017). A hydrophobic anchor mechanism defines a deacetylase family that suppresses host response against YopJ effectors. Nat. Commun. 8:2201. doi: 10.1038/s41467-017-02347-w
Cadoret, F., Soscia, C., and Voulhoux, R. (2014). “Gene transfer: transformation/electroporation,” in Pseudomonas Methods and Protocols, eds A. Filloux and J.-L. Ramos (New York, NY: Springer New York), 11–15. doi: 10.1007/978-1-4939-0473-0_2
Carver, T. J., Rutherford, K. M., Berriman, M., Rajandream, M.-A., Barrell, B. G., and Parkhill, J. (2005). ACT: the Artemis comparison tool. Bioinformatics 21, 3422–3423. doi: 10.1093/bioinformatics/bti553
Chen, L., Luo, S., Xiao, X., Guo, H., Chen, J., Wan, Y., et al. (2010). Application of plant growth-promoting endophytes (PGPE) isolated from Solanum nigrum L. for phytoextraction of Cd-polluted soils. Appl. Soil Ecol. 46, 383–389. doi: 10.1016/j.apsoil.2010.10.003
Chin, C.-S., Peluso, P., Sedlazeck, F. J., Nattestad, M., Concepcion, G. T., Clum, A., et al. (2016). Phased diploid genome assembly with single-molecule real-time sequencing. Nat. Methods 13, 1050–1054. doi: 10.1038/nmeth.4035
Choudhury, K., Clark, J., and Griffiths, H. R. (2014). An almond-enriched diet increases plasma α-tocopherol and improves vascular function but does not affect oxidative stress markers or lipid levels. Free Radic. Res. 48, 599–606. doi: 10.3109/10715762.2014.896458
Compant, S., Clément, C., and Sessitsch, A. (2010). Plant growth-promoting bacteria in the rhizo- and endosphere of plants: their role, colonization, mechanisms involved and prospects for utilization. Soil Biol. Biochem. 42, 669–678. doi: 10.1016/j.soilbio.2009.11.024
Cooley, M. B., Miller, W. G., and Mandrell, R. E. (2003). Colonization of Arabidopsis thaliana with Salmonella enterica and enterohemorrhagic Escherichia coli O157:H7 and competition by Enterobacter asburiae. Appl. Environ. Microbiol. 69:4915. doi: 10.1128/AEM.69.8.4915-4926.2003
Dale, V. H., and Polasky, S. (2007). Measures of the effects of agricultural practices on ecosystem services. Ecol. Econ. 64, 286–296. doi: 10.1016/j.ecolecon.2007.05.009
Eid, J., Fehr, A., Gray, J., Luong, K., Lyle, J., Otto, G., et al. (2009). Real-Time DNA sequencing from single polymerase molecules. Science 323:133. doi: 10.1126/science.1162986
Evans, P. T., and Malmberg, R. L. (1989). Do polyamines have roles in plant development? Annu. Rev. Plant Physiol. Plant Mol. Biol. 40, 235–269. doi: 10.1146/annurev.pp.40.060189.001315
Fu, X.-Z., Chen, C.-W., Wang, Y., Liu, J.-H., and Moriguchi, T. (2011). Ectopic expression of MdSPDS1 in sweet orange (Citrus sinensis Osbeck) reduces canker susceptibility: involvement of H2O2 production and transcriptional alteration. BMC Plant Biol. 11:55. doi: 10.1186/1471-2229-11-55
Fulton, J., Norton, M., and Shilling, F. (2019). Water-indexed benefits and impacts of California almonds. Ecol. Indic. 96, 711–717. doi: 10.1016/j.ecolind.2017.12.063
Goldstein, A. H. (1995). Recent progress in understanding the molecular genetics and biochemistry of calcium phosphate solubilization by gram negative bacteria. Biol. Agric. Hortic. 12, 185–193. doi: 10.1080/01448765.1995.9754736
Guerra, P. R., Herrero-Fresno, A., Ladero, V., Redruello, B., dos Santos, T. P., Spiegelhauer, M. R., et al. (2018). Putrescine biosynthesis and export genes are essential for normal growth of avian pathogenic Escherichia coli. BMC Microbiol. 18:226. doi: 10.1186/s12866-018-1355-9
Hinsinger, P. (2001). Bioavailability of soil inorganic P in the rhizosphere as affected by root-induced chemical changes: a review. Plant Soil 237, 173–195. doi: 10.1023/A:1013351617532
Ikeda, S., Kaneko, T., Okubo, T., Rallos, L. E. E., Eda, S., Mitsui, H., et al. (2009). Development of a bacterial cell enrichment method and its application to the community analysis in soybean stems. Microbial. Ecol. 58, 703–714. doi: 10.1007/s00248-009-9566-0
Imai, A., Matsuyama, T., Hanzawa, Y., Akiyama, T., Tamaoki, M., Saji, H., et al. (2004). Spermidine synthase genes are essential for survival of Arabidopsis. Plant Physiol. 135, 1565. doi: 10.1104/pp.104.041699
Jin, C. W., Li, G. X., Yu, X. H., and Zheng, S. J. (2010). Plant Fe status affects the composition of siderophore-secreting microbes in the rhizosphere. Ann. Bot. 105, 835–841. doi: 10.1093/aob/mcq071
Kafle, A., Cope, K. R., Raths, R., Krishna Yakha, J., Subramanian, S., Bücking, H., et al. (2019). Harnessing soil microbes to improve plant phosphate efficiency in cropping systems. Agronomy 9:127. doi: 10.3390/agronomy9030127
Karunakaran, R., Mauchline, T. H., Hosie, A. H. F., and Poole, P. S. (2005). A family of promoter probe vectors incorporating autofluorescent and chromogenic reporter proteins for studying gene expression in Gram-negative bacteria. Microbiology (Reading) 151, 3249–3256. doi: 10.1099/mic.0.28311-0
Kasukabe, Y., He, L., Nada, K., Misawa, S., Ihara, I., and Tachibana, S. (2004). Overexpression of spermidine synthase enhances tolerance to multiple environmental stresses and up-regulates the expression of various stress-regulated genes in transgenic Arabidopsis thaliana. Plant Cell Physiol. 45, 712–722. doi: 10.1093/pcp/pch083
Kusano, T., Berberich, T., Tateda, C., and Takahashi, Y. (2008). Polyamines: essential factors for growth and survival. Planta 228, 367–381. doi: 10.1007/s00425-008-0772-7
Lane, D. J. (1991). “16S/23S rRNA sequencing,” in Nucleic Acid Techniques in Bacterial Systematics, eds E. Stackebrandt and M. Goodfellow (New York, NY: John Wiley and Sons), 115–175.
Li, S.-C., Liu, Y.-H., Liu, J.-F., Chang, W.-H., Chen, C.-M., and Chen, C.-Y. O. (2011). Almond consumption improved glycemic control and lipid profiles in patients with type 2 diabetes mellitus. Metabolism 60, 474–479. doi: 10.1016/j.metabol.2010.04.009
Liang, J.-L., Liu, J., Jia, P., Yang, T., Zeng, Q., Zhang, S., et al. (2020). Novel phosphate-solubilizing bacteria enhance soil phosphorus cycling following ecological restoration of land degraded by mining. ISME J. 14, 1600–1613. doi: 10.1038/s41396-020-0632-4
Lindsey, B. E. III, Rivero, L., Calhoun, C. S., Grotewold, E., and Brkljacic, J. (2017). Standardized method for high-throughput sterilization of Arabidopsis seeds. J. Vis. Exp. 2017:56587. doi: 10.3791/56587
Lodewyckx, C., Vangronsveld, J., Porteous, F., Moore, E. R. B., Taghavi, S., Mezgeay, M., et al. (2002). Endophytic bacteria and their potential applications. Crit. Rev. Plant Sci. 21, 583–606. doi: 10.1080/0735-260291044377
Martinho, V. J. P. D. (2020). Exploring the topics of soil pollution and agricultural economics: highlighting good practices. Agriculture 24:10. doi: 10.3390/agriculture10010024
McRose, D. L., Seyedsayamdost, M. R., and Morel, F. M. M. (2018). Multiple siderophores: bug or feature? JBIC J. Biol. Inorg. Chem. 23, 983–993. doi: 10.1007/s00775-018-1617-x
Moschou, P. N., Sarris, P. F., Skandalis, N., Andriopoulou, A. H., Paschalidis, K. A., Panopoulos, N. J., et al. (2009). Engineered polyamine catabolism preinduces tolerance of tobacco to bacteria and oomycetes. Plant Physiol. 149, 1970–1981. doi: 10.1104/pp.108.134932
Moselhy, S. S., Asami, T., Abualnaja, K. O., Al-Malki, A. L., Yamano, H., Akiyama, T., et al. (2016). Spermidine, a polyamine, confers resistance to rice blast. J. Pestic. Sci. 41, 79–82. doi: 10.1584/jpestics.D16-008
Nautiyal, C. S. (1999). An efficient microbiological growth medium for screening phosphate solubilizing microorganisms. FEMS Microbiol. Lett. 170, 265–270. doi: 10.1111/j.1574-6968.1999.tb13383.x
Ortiz, R. M., Garcia, S., and Kim, A. D. (2012). Is almond consumption more effective than reduced dietary saturated fat at decreasing plasma total cholesterol and LDL-c levels? A theoretical approach. J. Nutr. Metab. 2012:265712. doi: 10.1155/2012/265712
Pandey, S., Ranade, S. A., Nagar, P. K., and Kumar, N. (2000). Role of polyamines and ethylene as modulators of plant senescence. J. Biosci. 25, 291–299. doi: 10.1007/BF02703938
Qiu, Y., Li, M., Kim, R. J.-A., Moore, C. M., and Chen, M. (2019). Daytime temperature is sensed by phytochrome B in Arabidopsis through a transcriptional activator HEMERA. Nat. Commun. 10:140. doi: 10.1038/s41467-018-08059-z
Raymond, K. N., Dertz, E. A., and Kim, S. S. (2003). Enterobactin: an archetype for microbial iron transport. Proc. Natl. Acad. Sci. U.S.A. 100:3584. doi: 10.1073/pnas.0630018100
Rezzonico, F., Smits, T. H. M., Born, Y., Blom, J., Frey, J. E., Goesmann, A., et al. (2016). Erwinia gerundensis sp. nov., a cosmopolitan epiphyte originally isolated from pome fruit trees. Int. J. Syst. Evol. Microbiol. 66, 1583–1592. doi: 10.1099/ijsem.0.000920
Rezzonico, F., Smits, T. H., Montesinos, E., Frey, J. E., and Duffy, B. (2009). Genotypic comparison of Pantoea agglomerans plant and clinical strains. BMC Microbiol. 9:204. doi: 10.1186/1471-2180-9-204
Richardson, A. E., and Simpson, R. J. (2011). Soil microorganisms mediating phosphorus availability update on microbial phosphorus. Plant Physiol. 156:989.
Richardson, N., Davies, J. A., and Radüchel, B. (1999). Iron(III)-based contrast agents for magnetic resonance imaging. Polyhedron 18, 2457–2482. doi: 10.1104/pp.111.175448
Ryu, C.-M., Murphy, J. F., Mysore, K. S., and Kloepper, J. W. (2004). Plant growth-promoting rhizobacteria systemically protect Arabidopsis thaliana against Cucumber mosaic virus by a salicylic acid and NPR1-independent and jasmonic acid-dependent signaling pathway. Plant J. 39, 381–392. doi: 10.1111/j.1365-313X.2004.02142.x
Saldierna Guzmán, J. P., Nguyen, K., and Hart, S. C. (2020). Simple methods to remove microbes from leaf surfaces. J. Basic Microbiol. 60, 730–734. doi: 10.1002/jobm.202000035
Schwyn, B., and Neilands, J. B. (1987). Universal chemical assay for the detection and determination of siderophores. Anal. Biochem. 160, 47–56. doi: 10.1016/0003-2697(87)90612-9
Shah, P., and Swiatlo, E. (2008). A multifaceted role for polyamines in bacterial pathogens. Mol. Microbiol. 68, 4–16. doi: 10.1111/j.1365-2958.2008.06126.x
Shen, Y.-Q., Bonnot, F., Imsand, E. M., RoseFigura, J. M., Sjölander, K., and Klinman, J. P. (2012). Distribution and properties of the genes encoding the biosynthesis of the bacterial cofactor, pyrroloquinoline quinone. Biochemistry 51, 2265–2275. doi: 10.1021/bi201763d
Shirley, M., Avoscan, L., Bernaud, E., Vansuyt, G., and Lemanceau, P. (2011). Comparison of iron acquisition from Fe–pyoverdine by strategy I and strategy II plants. Botany 89, 731–735. doi: 10.1139/b11-054
Simão, F. A., Waterhouse, R. M., Ioannidis, P., Kriventseva, E. V., and Zdobnov, E. M. (2015). BUSCO: assessing genome assembly and annotation completeness with single-copy orthologs. Bioinformatics 31, 3210–3212. doi: 10.1093/bioinformatics/btv351
Soares, M. A., Li, H.-Y., Bergen, M., da Silva, J. M., Kowalski, K. P., and White, J. F. (2016). Functional role of an endophytic Bacillus amyloliquefaciens in enhancing growth and disease protection of invasive English ivy (Hedera helix L.). Plant Soil 405, 107–123. doi: 10.1007/s11104-015-2638-7
Sturz, A. V., Christie, B. R., and Nowak, J. (2000). Bacterial endophytes: potential role in developing sustainable systems of crop production. Crit. Rev. Plant Sci. 19, 1–30. doi: 10.1080/07352680091139169
Sukumar, P., Leguè, V., Vayssières, A., Martin, F., Tuskan, G. A., and Kalluri, U. C. (2013). Involvement of auxin pathways in modulating root architecture during beneficial plant–microorganism interactions. Plant Cell Environ. 36, 909–919. doi: 10.1111/pce.12036
Timmusk, S., Abd El-Daim, I. A., Copolovici, L., Tanilas, T., Kännaste, A., Behers, L., et al. (2014). Drought-tolerance of wheat improved by rhizosphere bacteria from harsh environments: enhanced biomass production and reduced emissions of stress volatiles. PLoS One 9:e96086. doi: 10.1371/journal.pone.0096086
United Nations (2019). World Population Prospects 2019: Highlights. New York, NY: United Nations. eISBN: 978-92-1-004235-2.
Vansuyt, G., Robin, A., Briat, J.-F., Curie, C., and Lemanceau, P. (2007). Iron acquisition from Fe-pyoverdine by Arabidopsis thaliana. Mol. Plant Microbe Interact. 20, 441–447. doi: 10.1094/MPMI-20-4-0441
Verma, S. K., Kingsley, K., Bergen, M., English, C., Elmore, M., Kharwar, R. N., et al. (2018). Bacterial endophytes from rice cut grass (Leersia oryzoides L.) increase growth, promote root gravitropic response, stimulate root hair formation, and protect rice seedlings from disease. Plant Soil 422, 223–238. doi: 10.1007/s11104-017-3339-1
Willige, B. C., Isono, E., Richter, R., Zourelidou, M., and Schwechheimer, C. (2011). Gibberellin Regulates PIN-FORMED abundance and is required for auxin transport–dependent growth and development in Arabidopsis thaliana. Plant Cell 23:2184. doi: 10.1105/tpc.111.086355
Xie, S.-S., Wu, H.-J., Zang, H.-Y., Wu, L.-M., Zhu, Q.-Q., and Gao, X.-W. (2014). Plant growth promotion by spermidine-producing Bacillus subtilis OKB105. Mol. Plant Microbe Interact. 27, 655–663. doi: 10.1094/MPMI-01-14-0010-R
Yandigeri, M. S., Meena, K. K., Singh, D., Malviya, N., Singh, D. P., Solanki, M. K., et al. (2012). Drought-tolerant endophytic actinobacteria promote growth of wheat (Triticum aestivum) under water stress conditions. Plant Growth Regul. 68, 411–420. doi: 10.1007/s10725-012-9730-2
Yoda, H., Yamaguchi, Y., and Sano, H. (2003). Induction of hypersensitive cell death by hydrogen peroxide produced through polyamine degradation in tobacco plants. Plant Physiol. 132, 1973–1981. doi: 10.1104/pp.103.024737
Yoon, S.-H., Ha, S.-M., Kwon, S., Lim, J., Kim, Y., Seo, H., et al. (2017). Introducing EzBioCloud: a taxonomically united database of 16S rRNA gene sequences and whole-genome assemblies. Int. J. Syst. Evol. Microbiol. 67, 1613–1617. doi: 10.1099/ijsem.0.001755
Keywords: Erwinia gerundensis, endophyte, Prunus dulcis, plant growth promotion, spermidine, siderophore, phosphate solubilization
Citation: Saldierna Guzmán JP, Reyes-Prieto M and Hart SC (2021) Characterization of Erwinia gerundensis A4, an Almond-Derived Plant Growth-Promoting Endophyte. Front. Microbiol. 12:687971. doi: 10.3389/fmicb.2021.687971
Received: 30 March 2021; Accepted: 22 July 2021;
Published: 25 August 2021.
Edited by:
Marco Scortichini, Council for Agricultural and Economics Research (CREA), ItalyReviewed by:
Chiaraluce Moretti, University of Perugia, ItalyStefania Loreti, Council for Agricultural and Economics Research (CREA), Italy
Copyright © 2021 Saldierna Guzmán, Reyes-Prieto and Hart. This is an open-access article distributed under the terms of the Creative Commons Attribution License (CC BY). The use, distribution or reproduction in other forums is permitted, provided the original author(s) and the copyright owner(s) are credited and that the original publication in this journal is cited, in accordance with accepted academic practice. No use, distribution or reproduction is permitted which does not comply with these terms.
*Correspondence: J. Paola Saldierna Guzmán, cHNhbGRpZXJuYWd1em1hbkBzYWxrLmVkdQ==
†Present address: J. Paola Saldierna Guzmán, Howard Hughes Medical Institute, Salk Institute for Biological Studies, La Jolla, CA, United States