- 1School of Biological Sciences, Nanyang Technological University, Singapore, Singapore
- 2Singapore Centre for Environmental Life Sciences Engineering, Nanyang Technological University, Singapore, Singapore
- 3Faculty of Health Sciences, University of Macau, Macau, China
BPI-inducible protein A (BipA), a highly conserved paralog of the well-known translational GTPases LepA and EF-G, has been implicated in bacterial motility, cold shock, stress response, biofilm formation, and virulence. BipA binds to the aminoacyl-(A) site of the bacterial ribosome and establishes contacts with the functionally important regions of both subunits, implying a specific role relevant to the ribosome, such as functioning in ribosome biogenesis and/or conditional protein translation. When cultured at suboptimal temperatures, the Escherichia coli bipA genomic deletion strain (ΔbipA) exhibits defects in growth, swimming motility, and ribosome assembly, which can be complemented by a plasmid-borne bipA supplementation or suppressed by the genomic rluC deletion. Based on the growth curve, soft agar swimming assay, and sucrose gradient sedimentation analysis, mutation of the catalytic residue His78 rendered plasmid-borne bipA unable to complement its deletion phenotypes. Interestingly, truncation of the C-terminal loop of BipA exacerbates the aforementioned phenotypes, demonstrating the involvement of BipA in ribosome assembly or its function. Furthermore, tandem mass tag-mass spectrometry analysis of the ΔbipA strain proteome revealed upregulations of a number of proteins (e.g., DeaD, RNase R, CspA, RpoS, and ObgE) implicated in ribosome biogenesis and RNA metabolism, and these proteins were restored to wild-type levels by plasmid-borne bipA supplementation or the genomic rluC deletion, implying BipA involvement in RNA metabolism and ribosome biogenesis. We have also determined that BipA interacts with ribosome 50S precursor (pre-50S), suggesting its role in 50S maturation and ribosome biogenesis. Taken together, BipA demonstrates the characteristics of a bona fide 50S assembly factor in ribosome biogenesis.
Introduction
The ability of bacteria to respond, adapt, and grow at suboptimal temperature is known as cold shock response, which is activated in Escherichia coli under a condition of a sudden drop in culturing temperature (usually from 37 to 15°C) (Barria et al., 2013). Suboptimal temperature can cause both physiological and morphological changes in the bacteria and even cell death if the temperature shift is beyond bacterial tolerance. The majority of cold-inducible proteins involves RNA metabolism, indicating that regulation of RNA metabolism is crucial for suboptimal temperature adaptation (Barria et al., 2013). The most notable examples include the following: (i) cold shock protein (Csp) family proteins, which are RNA chaperones and able to prevent formation of RNA secondary structures (Phadtare et al., 2003; Barria et al., 2013; Uppal and Jawali, 2015); (ii) DEAD-box RNA helicase (DeaD) that can unwind RNA secondary structure to promote degradation (Charollais et al., 2004; Prud’homme-Généreux et al., 2004; Resch et al., 2010); and (iii) RNase R, the only 3′–5′ exonuclease in E. coli that degrades double-stranded RNA without the help of a helicase (Cairrão et al., 2003; Awano et al., 2010; Barria et al., 2013).
The translational stress response involves a key component of protein-synthesizing machinery, the ribosome. Bacteria utilize a series of protein factors that bind ribosome to modulate translation in order to cope with stress. For example, the RelA/SpoT homolog (RSH) proteins bind ribosome when there is a surge of uncharged tRNAs caused by the shortage of amino acids. Consequently, binding of RSH to ribosome triggers the alarmone synthesis and is followed by the stringent response (Hauryliuk et al., 2015). Notably, there are two proteins that bind to the ribosome to exert their stress response function: ObgE and BPI-inducible protein A (BipA). ObgE is an essential GTPase, and its homologs have been ubiquitously found across all kingdoms of life (Sato et al., 2005; Jiang et al., 2006). An analysis of those immature 50S particles, accumulated in cells with the ObgE depleted, showed that late assembling r-proteins (L33, L34, and L16) were under-represented, indicating that ObgE is an important factor during the late step of 50S biogenesis (Jiang et al., 2006).
BipA has been implicated in various functions of pathogenic bacteria, such as Salmonella typhimurium (S. typhimurium), Pseudomonas aeruginosa (P. aeruginosa), and enteropathogenic E. coli (EPEC). BipA is upregulated by ∼7-fold when S. typhimurium is “attacked” by bactericidal/permeability increasing (BPI) antimicrobial peptide (Qi et al., 1995). BipA is also known as TypA, referring to tyrosine phosphorylated protein A, and this is the case in P. aeruginosa where TypA was found to be involved in virulence, antimicrobial resistance, and biofilm formation (Neidig et al., 2013). In EPEC, BipA has been reported to upregulate the virulence and reduce flagella-mediated motility (Grant et al., 2003). The bipA deletion would result in growth delay of E. coli K12 strain while cultured at low temperature (e.g., 20°C) (Pfennig and Flower, 2001). Furthermore, Choudhury and Flower (2015) reported that the ribosomal particle distribution of the bipA deletion strain changes dramatically, with the accumulation of the 30S and the presence of 50S precursor (pre-50S), indicating a possible role of BipA in ribosome biogenesis (Choudhury and Flower, 2015). Interestingly, a transposon-mediated random insertion mutation into E. coli K12 strain genome revealed that the disruption of the rluC gene can suppress the phenotype of the bipA deletion (Krishnan and Flower, 2008). RluC is a pseudouridine synthase, which converts three uridines (U955, U2504, and U2580) in 23S rRNA to pseudouridines (Ψ955, Ψ2504, and Ψ2580). Pseudouridine is a uridine isomer where the uracil base is linked to the pentose sugar by a carbon-to-carbon bond, resulting in the ability to form an additional hydrogen bond and thereby increasing the stability compared to uridine (Kierzek et al., 2013). In addition, Choudhury and Flower (2015) discovered that the deletion of DeaD exacerbates both the growth and ribosomal particle distribution defects (Choudhury and Flower, 2015). Since DeaD is involved in 50S biogenesis, it is not surprising that bipA and deaD double mutations could cause an evident defect in ribosome biogenesis. Crystal structures of the apo-, as well as the nucleotides [GDP, (p)ppGpp, and GDPCP] bound BipA, showed no significant difference in their overall conformations (Fan et al., 2015; Kumar et al., 2015). However, a drastic conformational change was observed in domains III, V, and the C-terminal domain (CTD), upon GTP form BipA binding to 70S ribosome (Kumar et al., 2015). The conformational change of the CTD was particularly interesting because the CTD loop extends into the ribosomal A site to establish extensive contacts with the tRNA acceptor stem; however, the precise role of such interactions is largely unknown (Kumar et al., 2015). Nevertheless, the structure of BipA with ribosome demonstrated an activated form of BipA in the ribosome, with the catalytic residue, histidine 78 (H78), situated close to the 23S rRNA sarcin–ricin loop (SRL) in a ratcheting ribosome as well as interacting with the bound nucleotide (GDPCP). Collectively, BipA is an authentic ribosome-dependent trGTPase. However, how BipA in association with 70S ribosome is correlated with bacterial stress response as well as how BipA functions in conditional translation for bacterial cells at suboptimal temperature require further studies.
Notably, recent quantitative mass spectrometry data nicely showed the accumulated pre-50S intermediates in bipA mutant cells at suboptimal temperature, with several r-proteins absent, further demonstrating the role of BipA in 50S subunit assembly (Gibbs et al., 2020). Furthermore, a paper published during our manuscript preparation revealed that bacteria can remodel their protein expression relevant to biofilm formation in a temperature-dependent manner by modulating BipA abundance (Del Peso Santos et al., 2021). With the desire to support the ongoing effort in BipA-related studies and to expand our efforts on structural study of ribosome-associated proteins and biofilm formation as well as its relevant pathogenesis and resistance (Tanaka et al., 2008; Selmer et al., 2012; Kumar et al., 2015; Yu et al., 2015; Ero et al., 2016; Kumar et al., 2016; Yang et al., 2017), here we report additional evidence to demonstrate the role of BipA in ribosome biogenesis, specifically in large subunit 50S maturation, and conditional protein translation at suboptimal temperature through combinative approaches. In particular, our findings by tandem mass tag (TMT)-based quantitative proteomic analysis identified proteins relevant to RNA metabolism (e.g., DeaD) with increased expression levels upon BipA deletion, such an effect can be suppressed by a further mutation of RluC or complementation by BipA. The upregulation of these protein possibly indicates that bacterial cells can compensate for the loss of BipA during 50S biogenesis.
Materials and Methods
Bacterial Strains and Culturing
The E. coli strains used in this project are listed in Table 1. Strains were grown in Lysogeny broth (LB) at 37°C for optimal growth and at 25°C for suboptimal growth. The antibiotics chloramphenicol (34 μg/ml), gentamicin (15 μg/ml) and kanamycin (30 μg/ml) were added when required for selection.
Deletion of rluC gene was carried out using the pRed/ET system (Heermann et al., 2008), replacing rluC with gentamicin resistance cassette through sequence-specific homologous recombination. Linear DNA fragments consisting of the homologous arms (50-bp sequences upstream and downstream of rluC), sandwiching the gentamicin antibiotic cassette, were transformed into pRed/ET-harboring E. coli K12 strain (K12WT) using electroporation.
Deletion of genomic bipA and spoT genes was carried out using the pDS132 (Philippe et al., 2004) suicide plasmid. Approximately 500 bp upstream and downstream of bipA and spoT were amplified as the homologous arms. The upstream and downstream arms were combined through restriction site ligation and cloned into pDS132 to obtain pDS132-bipA and pDS132-spoT plasmids. The plasmids were transformed into target cells using electroporation and two rounds of selections. Kanamycin-resistant cells were selected from the first round of selection, and sucrose-sensitive cells were selected from the second round of selection.
Knock-in of lacZ downstream of deaD and obgE was done using pVIK111 (Zheng et al., 2007) suicide vector. In-frame insertion was created by amplifying approximately 500 bp of 3′-end of the target gene, while excluding the stop codon, and cloned into pVIK111 yielding pVIK111-deaD and pVIK111-obgE. The plasmids were then transformed into E. coli K12 strain (K12WT), ΔbipA, ΔbipA (pCA24N-BipA), and ΔrluC/ΔbipA using electroporation. Kanamycin-resistant clones were selected.
Site-Directed Mutagenesis
Modifications of pCA24N-BipA were done using the QuikChange method to produce pCA24N-BipAH78A, pCA24N-BipAH78Q, and pCA24N-BipAT544_D552del. Primers were designed to have equal lengths of nucleotides extending toward 5′- and 3′-ends from the point of modification. Briefly, the first step was PCR amplification of pCA24N-BipA to produce two single-stranded circular DNAs by carrying out the forward and reverse amplification in separate reactions. Secondly, the samples were mixed in the presence of 1 μl DpnI enzyme (NEB®), which digested the template DNA. Next was activation at 37°C for 2.5 h, followed by deactivation at 80°C for 20 min, and then denaturation at 98°C for 15 min. Lastly, samples were incubated at room temperature to allow the denatured products to re-anneal. Chloramphenicol-resistant clones were selected for sequence verification.
Growth Assay
Growth curves were determined using a 96-well microplate. The overnight cultures were diluted in LB to OD600 ≈ 0.02 in a final volume of 100 μl/well. Plates were shaken in a shaker incubator at 200 RPM and temperature of 37°C for optimal growth and 25°C for suboptimal growth. The cell density was measured by a TECAN SparkTM 10M multimode microplate reader without microplate lid every half an hour (37°C) or every hour (25°C) under the settings of 600-nm light absorbance, 25 flashes, and 120-ms wait time between wells. Growth curves were performed in biological triplicates.
Swimming Motility Assay
Overnight cultures were diluted to OD600 ≈ 1.0 in 0.9% (w/v) NaCl. Soft LB agar (0.3% w/v) was inoculated with overnight cultures by using a drawing needle to dip into the diluted overnight cultures and then pierced through the soft agar to the middle. The plates were incubated at room temperature for 24 and 48 h. Results were recorded in the form of images and measurements of swimming diameter.
Sucrose Gradient Sedimentation Analysis
Cell pellets [K12WT, ΔbipA, ΔbipA (pCA24N-BipA), ΔbipA (pCA24N-BipAH78A), ΔbipA (pCA24N-BipAH78Q), ΔbipA (pCA24N-BipAT544_D552del), ΔrluC, and ΔrluC/ΔbipA] were resuspended in RNA lysis buffer [20 mM HEPES pH 7.5, 10.5 mM MgOAc, 100 mM NH4Cl, 0.5 mM EDTA pH 8.0, and 6 mM β-mercaptoethanol (β-ME)] in the presence of 20 U/ml DNase I and 1 mg/ml lysozyme. Cells were lysed by three rounds of freeze and thaw (30 min freezing in −80°C and complete thawing in ice water). Ten A260 units was layered onto 5–45% (w/v) sucrose gradient for polysome profiling. The 5% and 45% (v/v) sucrose solutions were prepared by dissolving sucrose in overlay buffer (10 mM HEPES pH 7.5, 50 mM KCl, 10 mM NH4Cl, 10.25 mM MgOAc, and 0.25 mM EDTA pH 8.0), and 6 mM β-ME was added before use. The gradients were formed using Gradient Master (BioComp, Munich, Germany). The sucrose gradients were centrifuged at 36,000 RPM for 1.5 h (ω2t = 7.6746 × 1010) at 4°C using SW 41 Ti rotor (Beckman Coulter, Brea, CA, United States). A density gradient fractionator system (Brandel, Gaithersburg, MD, United States) was used to analyze the ribosomal particles by continuous monitoring of A260 and to fractionate the samples.
Tandem Mass Tag-Mass Spectrometry-Based Quantitative Proteomics
Tandem mass tag-mass spectrometry (TMT-MS) was carried out as described in a previous work (Park et al., 2019), with slight modifications. K12WT, ΔbipA, ΔbipA (pCA24N-BipA), ΔrluC, and ΔrluC/ΔbipA were cultured in 200 ml LB at 25°C in a shaking incubator at 200 RPM to OD600 ≈ 1.0. The cultures were immediately cooled on ice before centrifugation at 4,500 RPM for 8 min at 4°C. The resulting cell pellets were resuspended in 1 ml TMT lysis buffer [100 mM tetraethylammonium bromide (TEAB) pH 8.5, 1 mM PMSF, 1% Triton X-100, and EDTA-free protease inhibitor cocktail]. Lysis was done using sonication for 5 min at 40% amplitude with pulses set at 5 s on followed by 5 s off. Lysates were incubated at 4°C on a rotator for 1 h and then clarified by centrifugation at 15,000 RPM for 45 min at 4°C. The clarified lysates were filtered through a 0.22-μm spin filter (Corning® Costar® Spin-X®) and sent to the Proteomic Core Facility of the Biological Research Center of Nanyang Technological University (Singapore) for TMT-MS services. The TMT-MS was done with technical triplicates.
β-Galactosidase Assay
The β-galactosidase assay was performed using 96-well microplates by referring to a reported work (Schaefer et al., 2016). The obgE:: pVIK111 and deaD:: pVIK111 strains were cultured in 10 ml LB at 25°C with shaking at 200 RPM. When the OD600 reached the value of 0.2, 0.5, and 1.0, 20 μl of the culture was collected and mixed with 80 μl permeabilization solution (100 mM Na2HPO4, 20 mM KCl, 2 mM MgSO4, 0.04% sodium deoxycholate, 5 mM β-mercaptoethanol, and 1 mg/ml lysozyme) in a 96-well microplate (NuncTM MicroWellTM, Thermo Scientific, Waltham, MA, United States). Permeabilizing samples were stored at 4°C until all the samples were prepared, and then, 25 μl of permeabilized samples were mixed with 150 μl of substrate solution (60 mM Na2HPO4, 40 mM NaH2PO4, 1 mg/ml ONPG, and 5 mM β-mercaptoethanol) in a 96-well microplate (NuncTM MicroWellTM, Thermo Scientific, Waltham, MA, United States) and mixed well before loading the plate without its lid into the TECAN SparkTM 10M multimode microplate reader. The OD420 absorbance was measured every 5 min for a total of 80 min incubation at 37°C. The settings for the OD420 absorbance measurement were 25 flashes and 120-ms wait time between wells. Biological triplicates were analyzed for all strains.
Purification of Pre-50S Particles
E. coli ΔbipA strain was cultured in large scale (six flasks of 800 ml LB) at 25°C with shaking at 200 RPM, until the OD600 ≈ 0.4–0.5. The cultures were immediately cooled down on ice before centrifugation at 4,000 RPM for 15 min using JLA-8.1000 rotor (Beckman Coulter, Brea, CA, United States). The resulting pellets were pooled and resuspended in 30 ml lysis buffer (20 mM HEPES pH 7.5, 10.5 mM MgOAc, 100 mM NH4Cl, 0.5 mM EDTA pH 8.0, 6 mM β-ME, 20 U/ml DNase I, and 1 mg/ml lysozyme) and aliquoted into Eppendorf tubes to go through three rounds of freeze and thaw for cell lysis. The lysates were centrifuged at 14,500 RPM for 30 min at 4°C, and the supernatants were pooled. The supernatant was diluted using lysis buffer to a concentration of 80 A260 units in a final volume of 800 μl. Then, 10–25% (w/w) sucrose gradients were formed by mixing sucrose solution with overlay buffer (10 mM HEPES pH 7.5, 50 mM KCl, 10 mM NH4Cl, 10.2 mM MgOAc, and 0.25 mM EDTA pH 8.0). Onto the sucrose gradients, 800 μl of samples were layered in 38.5-ml polyallomer open-top tubes (Beckman Coulter, Brea, CA, United States). The gradients were centrifuged at 19,000 RPM for 17.5 h (ω2t = 2.5 × 1011 at 4°C) in SW 28 Ti rotor (Beckman Coulter, Brea, CA, United States). Ribosomal particles were analyzed by a density gradient fractionator system (Brandel, Gaithersburg, MD, United States) by continuous monitoring of A260 and to fractionate the samples. Fractions that corresponded to the peak between the 30S and 50S subunit peaks were collected and pooled. Pooled samples were split into 26-ml polycarbonate bottles with cap assembly (Beckman Coulter, Brea, CA, United States) and centrifuged at 43,000 RPM for a minimum of 19 h at 4°C in Ti70 rotors. The supernatant was discarded, and the resulting pellets were washed with 70S buffer (5 mM HEPES pH 7.5, 50 mM KCl, 10 mM NH4Cl, and 10 mM MgOAc) twice to remove the sucrose. The pellets were resuspended in 1 ml of 70S buffer and stored at −80°C.
Purification of BipA
BipA was overexpressed from the plasmid pNIC28-Bsa-bipA in E. coli BL21 (DE3) and purified through three consecutive chromatography steps. Cells transformed with the plasmid were cultured large scale at 37°C until the OD600 ≈ 0.8 and then overexpression was induced by adding isopropyl β-D-thiogalactoside (IPTG) to a final concentration of 125 μM. Culture temperature was reduced to 16°C upon IPTG induction for overnight incubation. Cells were harvested by centrifugation at 4,000 RPM for 15 min at 4°C. The resulting cell pellet was resuspended in 100 ml of lysis buffer [50 mM NaH2PO4 pH 8.0, 300 mM NaCl, 10% (v/v) glycerol, and 5 mM β-ME] and lysed using LM20 Microfluidizer® (MicrofluidicsTM, Newton, MA, United States) with 20,000 PSI pressure. The lysate was clarified by centrifugation at 20,000 RPM for 1 h at 4°C using a JA-25.50 rotor, filtered through a 0.45-μm filter, and kept on ice before loading onto HisTrap® HP 5 ml Ni2+ column for affinity chromatography using ÄKTA Purifier (GE Healthcare Life Sciences, Marlborough, MA, United States). The column was then washed with two column volumes (CV) of lysis buffer. The elution was performed by gradually increasing the concentration of His elution buffer [50 mM NaH2PO4 pH 8.0, 300 mM NaCl, 10% (v/v) glycerol, 5 mM β-ME, and 500 mM imidazole). The fractions containing BipA as determined by sodium dodecyl sulfate–polyacrylamide gel electrophoresis (SDS-PAGE) were pooled and diluted with 25 mM Tris pH 8.0 buffer to a final concentration of 50 mM NaCl before loading onto HiTrap® Q Fastflow 5-ml column for anion exchange chromatography using ÄKTA Purifier. The column was then washed with two CVs of Q buffer A (25 mM Tris pH 8.0, 50 mM NaCl, and 5 mM β-ME). The elution was carried out by gradually increasing the concentration of Q buffer B (25 mM Tris pH 8.0, 1 M NaCl, and 5 mM β-ME). The fractions containing BipA as determined by SDS-PAGE were pooled and concentrated to a volume of 5 ml using Amicon® Ultra-15 Centrifugal Filter Units with 30-kDa cut-off membrane (MERCK, Darmstadt, Germany). A concentrated sample was loaded onto HiLoad® 16/60 Superdex® 200-pg column equilibrated with GF buffer (25 mM Tris pH 8.0, 100 mM NaCl, and 5 mM β-ME) for size exclusion chromatography using ÄKTA Explorer. The fractions containing BipA were pooled and concentrated to 13 mg/ml using Amicon® Ultra-15 Centrifugal Filter Units with 10-kDa cut-off membrane (MERCK, Darmstadt, Germany). The concentrated proteins were snap frozen using liquid nitrogen and stored at −80°C.
Ribosome Binding Assay
For at least 30 min on ice, 50 μl of BipA (13 mg/ml) in GF buffer was incubated with 100 μM GDPCP. For western blotting of polysome profiling fractions, 10 A260 units of ΔbipA cell lysate was prepared, and BipA pre-incubated with GDPCP was added five times in excess (10 A260 = 23.9 nM; BipA concentration = 1.195 μM) and incubated on ice for 1.5 h. The sample was layered onto 5%–45% sucrose gradient in overlay buffer (10 mM HEPES pH 7.5, 50 mM KCl, 10 mM NH4Cl, 10.25 mM MgOAc, and 0.25 mM EDTA pH 8) in 13.2-ml thin-wall polypropylene tubes (Beckman Coulter, Brea, CA, United States) and centrifuged at 36,000 RPM for 1.5 h (ω2t = 7.6746 × 1010) at 4°C using an SW 41 Ti rotor (Beckman Coulter, Brea, CA, United States). The gradients were fractionated by 10 drops per 1.5-ml Eppendorf tubes. Fractions were precipitated by adding 2.5 times sample volume of ice-cold 100% ethanol and one-tenth sample volume of 3 M NaOAc and incubation at −20°C overnight. The precipitated ribosomal samples were recovered by centrifuging the samples at 14,500 RPM for 30 min at 4°C, then air-dried before resuspending in 10 μl of RNase-free water. Recovered ribosomal samples were then loaded onto 10% polyacrylamide gel followed by semi-dry transfer onto nitrocellulose membrane. Recombinant BipA was detected by western blotting using 1:2,000 of HRP-conjugated anti-His6 antibody (Santa Cruz Biotechnology, Dallas, TX, United States), and then, Clarity ECL western blotting substrates (Bio-Rad, Hercules, CA, United States) was applied before visualization using ChemiDocTM (Bio-Rad, Hercules, CA, United States).
BipA binding was also analyzed by co-pelleting through sucrose cushion. Two separate samples were prepared on ice. Then, 50 μl of BipA (13 mg/ml) in GF buffer was incubated with 100 μM GDPCP for at least 30 min on ice. The sample with BipA pre-incubated with GDPCP only was prepared by mixing 1X Buffer G (5 mM HEPES pH 7.5, 50 mM KCl, 10 mM NH4Cl, 10 mM MgOAc, and 6 mM β-ME) and 144 μM of BipA pre-incubated with GDPCP and topped up to 50 μl with RNase-free water. The BipA pre-incubated with GDPCP complex with pre-50S particles was prepared by mixing 1X Buffer G with 144 μM of BipA pre-incubated with GDPCP and 24 OD260 units (0.576 μM) of pre-50S particles and then topped up to 50 μl with RNase-free water. Then, samples were layered onto 1.1 M sucrose in 1X Buffer G and centrifuged at 45,000 RPM in a TLA-100 rotor for 16 h at 4°C. Post-centrifugation, 1 μl of supernatant was aliquoted from each sample. The remaining supernatant was discarded, and the pellet was washed with 1X Buffer G three times to remove the sucrose. The pellets were then resuspended in 20 μl of 1X Buffer G. The RNA concentration of sample with BipA pre-incubated with GDPCP complex with pre-50S particles was measured using a NanoDrop 2000c spectrophotometer (Thermo Fisher Scientific, Waltham, MA, United States) and adjusted to 24 OD260 units using 1X Buffer G if dilution was required. Samples for PAGE were prepared by mixing 1 μl of the sample, 2.5 μl of 4X loading buffer, 1 μl of β-mercaptoethanol, and 5.5 μl of RNase-free water and incubated at 70°C for 10 min. Then, samples were loaded onto 4–12% NuPAGE Bis-Tris Gel (Invitrogen, Waltham, MA, United States) and run in 1X MES buffer at 200 V.
Results
Deletion of Genomic bipA Gene Affects E. coli Growth at Suboptimal Temperature
While the growth defects of E. coli ΔbipA knock-out strains at suboptimal culturing temperature (mostly at 20°C) have been reported by several groups (Pfennig and Flower, 2001; Krishnan and Flower, 2008; Choudhury and Flower, 2015; Choi and Hwang, 2018), the phenotype is not well consistent and understood. For a better understanding of the importance of BipA in cold stress conditions, we first determined the growth curves of E. coli K12 strain (K12WT) and its corresponding bipA knock-out strain (ΔbipA), both harboring the empty pCA24N vector, under optimal (37°C, Figure 1A) and suboptimal (25°C, Figure 1B) temperatures. While the two strains demonstrated a similar growth rate at 37°C (Figure 1A), the ΔbipA strain revealed a notable growth retardation at 25°C, resulting from a significantly longer lag phase (Figure 1B). Further corroborating the role of BipA in cold stress is the finding that BipA expression from plasmid pCA24N-BipA could restore the growth of ΔbipA strain, albeit not entirely (Figure 1B). In line with a previous report (Choudhury and Flower, 2015), RluC deficiency (ΔrluC) can complement the growth defect of ΔbipA at 25°C (Figure 1B).
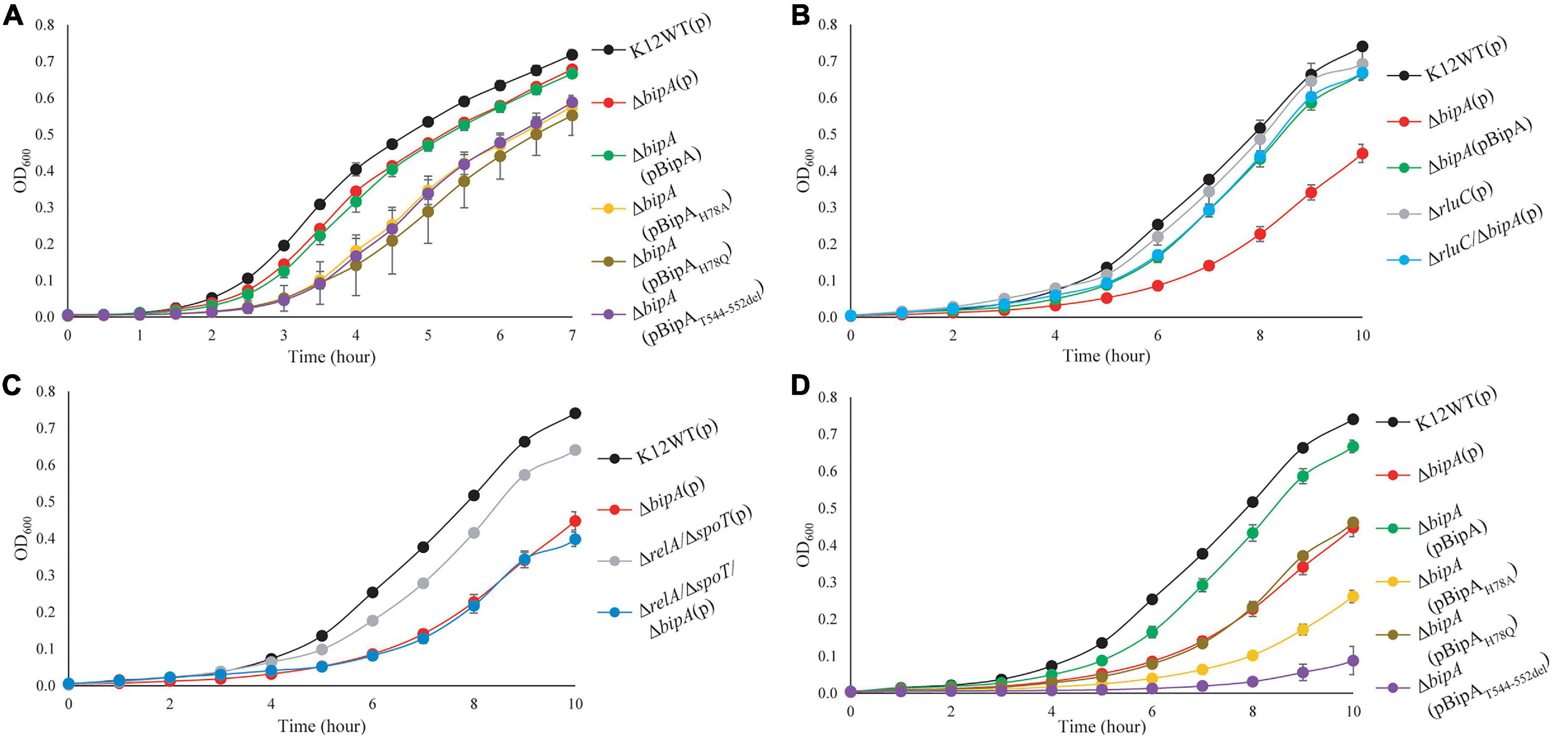
Figure 1. Growth curves of E. coli wild-type, bipA-deficient, and complementation strains under optimal (37°C) and suboptimal (25°C) growth temperatures. (A) Growth curves at 37°C of E. coli with various BipA mutations. Under optimal condition, the strain ΔbipA did not present a significant growth defect, but the strains ΔbipA transformed with pCA24N-BipA variants presented a significant delay in growth. (B) Growth curves at 25°C of E. coli with deletions for bipA and/or rluC. Loss of bipA (ΔbipA) led to growth defect at 25°C, which can be complemented by the presence of BipA expression (pCA24N-BipA) or suppressed by genomic deletion of rluC. The complementation was judged by the growth curve progression of the diverse strains (ΔbipA, ΔbipA + pCA24N-BipA, ΔrluC, and ΔbipA + ΔrluC) in comparison to the wild-type strain (K12WT). (C) Growth curves at 25°C of E. coli with deletions for bipA, relA, and/or spoT. Deletion of both relA and spoT caused a slight growth delay but did not exacerbate the growth delay of ΔbipA in the triple knock-out strains. (D) Growth curves at 25°C of E. coli with various BipA mutations. The ΔbipA strain expressing pCA24N-BipA variants presented different growth curves at 25°C. The cells with BipAH78Q demonstrated the same growth rate as the strain ΔbipA (pCA24N), while preceding with BipAH78A that preceded with BipAT544_D552del. Note that biological triplicates were analyzed, and pCA24N without gene of interest was transformed into cells not harboring any plasmid so that all strains have the similar cellular burden of holding a plasmid. The “p” in the bracket alone or preceding “BipA” represents plasmid pCA24N.
Next, we sought to investigate the effect of the loss of genes linked to bacterial stress response. Namely, we examined whether the growth defect is exacerbated if alarmone synthetase genes relA and spoT were deleted in the ΔbipA background. Our results showed that the double mutant strains (ΔrelA/ΔspoT) demonstrated a slight growth defect compared to the wild type, whereas the growth curve was similar to relA/spoT knock-out in the ΔbipA background (triple mutations) (Figure 1C), implying that alarmone level perhaps has little influence on the growth of E. coli at suboptimal temperature.
GTP hydrolysis activity is important for trGTPase turnover on ribosome and in turn its physiological function through conformational change (Kumar et al., 2015; Ero et al., 2016). To study the significance of GTP hydrolysis for BipA functioning under sub-optimal growth temperature, mutations were introduced into the plasmid-borne bipA gene and subsequently transferred to the ΔbipA strain. We mutated the proposed catalytic residue histidine 78 to alanine (H78A) or glutamine (H78Q) to abolish the GTP hydrolysis, respectively, based on structure and sequence comparison of BipA with other trGTPases such as EF-G and EF-Tu (Scarano I, Krab et al., 1995; Gao et al., 2009; Schmeing et al., 2009; Koripella et al., 2015). In addition, the C-terminal loop (CTL) of BipA was truncated (T544_D552del) given that the CTL is believed to be essential for BipA binding with 70S ribosome (deLivron et al., 2009; Kumar et al., 2015). Growth complementation results showed that under an optimal growth condition (37°C), leaky expression of BipA mutants BipAH78A, BipAH78Q, and BipAT544_D552del would have a negative effect on the growth of ΔbipA strain whereas the native BipA expression had no effect on cell growth (Figure 1A). In line with the role of GTP hydrolysis essential for BipA turnover, these findings likely suggest that the aforementioned mutations cause BipA turnover defects, resulting in the “trapped” ribosomes leading to decrease in translation and, ultimately, affecting cell growth. By being “trapped,” the ribosomes would be prevented from carrying out its task due to the bound translational factors being unable to dissociate itself from the ribosome, similar to that in which EF-G was trapped by fusidic acid (Gao et al., 2009).
Interestingly, the growth defects that vary in magnitude were observed when the ΔbipA strains transformed with diverse bipA mutations were grown at suboptimal temperature of 25°C (Figure 1D). Namely, the growth complementation of ΔbipA strain by plasmid-borne BipA was not achieved for BipAH78A, BipAH78Q, and BipAT544_D552del mutants, whereas BipA could completely restore the growth of ΔbipA strain (Figure 1D). In particular, the expression of BipAH78A and BipAT544_D552del mutants resulted in further notable defects in ΔbipA strain at suboptimal conditions, suggesting that ribosome binding and GTP hydrolysis are crucial for the role of BipA in bacterial growth under cold shock stress.
Taken together, our results demonstrated that the elimination of alarmone synthesis by removing RSH proteins has a minute effect on cell growth of at low temperature, whereas the loss of bipA would cause a significant growth defect, which could be complemented or suppressed by pCA24N-BipA supplementation and genomic rluC deletion, respectively.
Loss of bipA Gene Causes Swimming Motility Defect in E. coli at Suboptimal Temperature
It was recently reported that E. coli with bipA deletion demonstrated motility defects while incubated at 20°C (Choi and Hwang, 2018). Hence, we would like to examine the swimming motility of our E. coli strains using agar plate assay and incubation at room temperature, with the agar plate images after 24- and 48-h incubation shown (Figures 2A,B). The results of 24-h incubation demonstrated that the swimming motility was severely diminished for ΔbipA strain, and it could be complemented by plasmid harboring native bipA gene, but not the bipAH78A, bipAH78Q, and bipAT544_D552del mutants (Figure 2A). Interestingly, all ΔbipA strains produced chemotactic rings after 48-h incubation (Figure 2B). Despite that the rings for the BipA mutants are notably smaller than those for the strains expressing BipA, the results clearly showed that swimming motility was not diminished, but rather reduced, for ΔbipA and the bipA mutants. Furthermore, ΔbipA strain expressing BipAH78Q produced significantly larger rings than ΔbipA strain expressing BipAH78A or BipAT544_D552del after 48-h incubations (Figure 2B), which suggests that H78Q substitution retains BipA function to a certain extent, likely the ribosome binding and transition state stabilizing. In line with this hypothesis, ΔbipA (pCA24N-BipAH78A) produced a significantly larger ring than ΔbipA (pCA24N-BipAT544_D552del) after 48-h incubation, indicating that the truncation of BipA CTL causes more severe motility defects for E. coli (Figure 2B), and note that BipA CTL is required for BipA binding to ribosome (deLivron et al., 2009; Kumar et al., 2015). On the other hand, while RluC-deficient (ΔrluC) strain behaved similarly to E. coli wild-type strain (K12WT), the ΔrluC/ΔbipA strain demonstrated significant suppression on the swimming defect of ΔbipA (Figures 2A,B); this revealed a functional link between BipA and RluC as previously reported (deLivron et al., 2009).
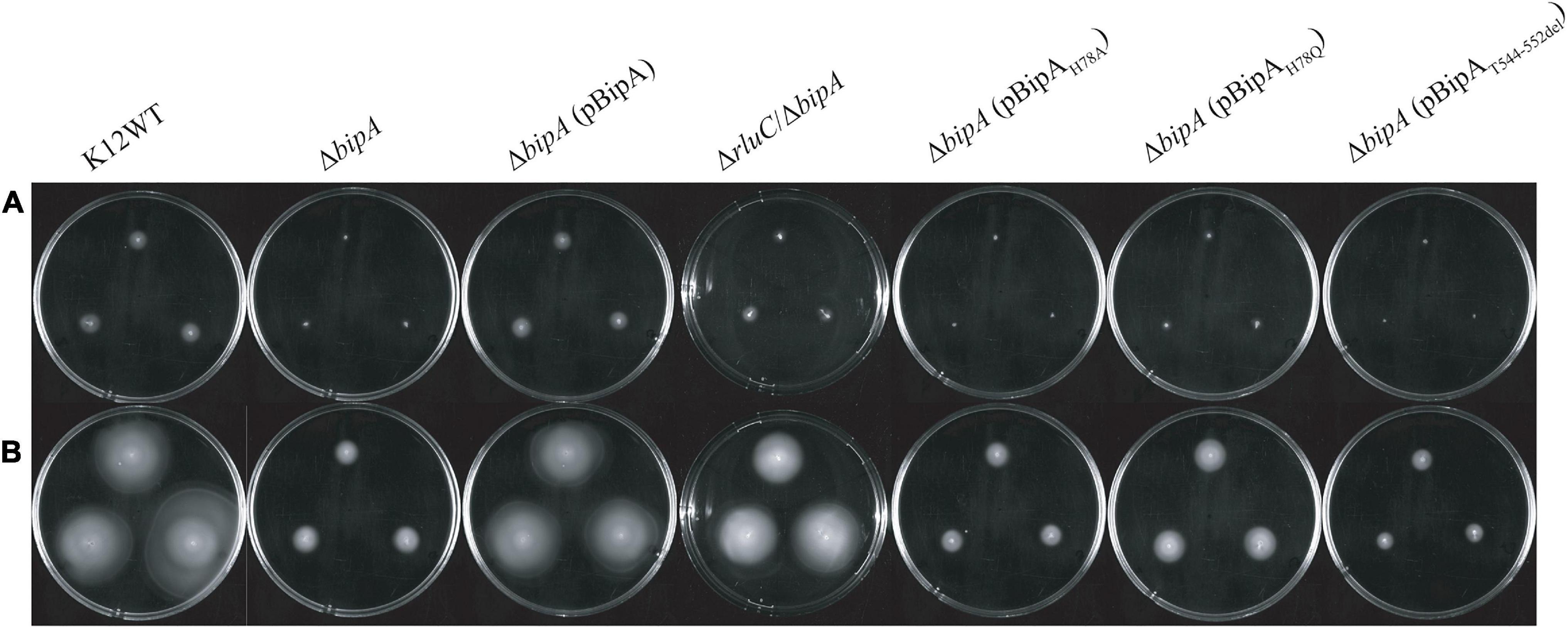
Figure 2. The effect of BipA on swimming motilities of various strains of E. coli K12 BW25113 after (A) 24-h incubation and (B) 48-h incubation. The agar plate-based assay was employed, and it was assessed by the development of chemotactic ring. (A) After 24-h incubation at room temperature (suboptimal temperature), cells with bipA deletion showed no notable chemotactic rings except for cells with double mutations (ΔrluC/ΔbipA). Cells with the plasmid-borne BipA were compensated for the loss of genomic bipA and yielded chemotactic ring with similar size to the strain K12WT. The ΔbipA strains expressing BipA mutants also demonstrated the lack of chemotactic ring except for the strain ΔbipA (pCA24N-BipAH78Q), which showed slight development of chemotactic ring as compared to the ΔbipA strain. (B) After 48-h incubation, all the strains developed chemotactic rings despite variations in size. The ΔbipA strain expressing BipA mutants presented significant increase in chemotactic ring size, where the strain ΔbipA (pCA24N-BipAH78Q) yielded chemotactic ring larger than the strains ΔbipA (pCA24N-BipAH78A and pCA24N-BipAT544_D552del). The “p” in the bracket alone or preceding “BipA” represents plasmid pCA24N.
Agar plate assay alone does not provide sufficient evidence to conclude whether the chemotactic ring represents the swimming motility of the bacteria, given that the possible influence of cell growth on the ring formation cannot be completely ruled out. The rings observed on semi-solid agar might represent bacterial growth instead of swimming motility because all the strains except K12WT and ΔbipA (pCA24N-BipA) did not present the typical chemotactic rings where highly motile populations form an outer layer of the ring (Koster et al., 2012; Cremer et al., 2019; Liu et al., 2019). Furthermore, the cells might be defective in swimming and were tumbling, or the observations were restricted to semi-solid environment (Kinosita et al., 2020). Thus, inverted microscopy was employed to detect the diluted overnight cultures of K12WT, ΔbipA, ΔbipA (pCA24N-BipA), and ΔrluC/ΔbipA cells (Supplementary Movies 1–4), and these animated movies show that all four strains demonstrated swimming motility in liquid media. Taken together, strains with bipA yielded larger chemotactic rings at room temperature, and deletion of bipA caused a significant delay in the appearance of chemotactic rings, which could be complemented by introducing functional BipA or suppressed by genomic deletion of rluC.
The Effect of BipA Mutations on the Defect of Ribosome Assembly
The loss of bipA had been found to cause ribosome assembly defect with the accumulation of pre-50S, which can be alleviated by expressing BipA from a plasmid or genomic deletion of rluC (Krishnan and Flower, 2008). Very recently, BipA has been implicated in ribosome (specifically large subunit) assembly at low temperature growth (Choi and Hwang, 2018; Gibbs et al., 2020). Here, we examine whether the bipAH78A, bipAH78Q, and bipAT544_D552del mutants affect the complementation of ribosome assembly defect in the ΔbipA cells through sucrose gradient sedimentation analysis. As shown in Figure 3A, compared to the wild-type strain K12WT, bipA deficiency resulted in significantly reduced 70S ribosome and 50S subunit populations (Figure 3A), and simultaneously, a minor peak between the 30S and 50S peaks was observed, which is likely representing a population of pre-50S particles and is consistent with the previous results (Choi and Hwang, 2018; Gibbs et al., 2020). Similar to that presented by Gibbs et al. (2020), the pre-50S peak was not observed in the ribosomal particle distribution of ΔbipA expressing exogenous BipA (pCA24N-BipA), suggesting that BipA is involved in ribosome assembly, particularly in the maturation of the 50S subunit (Figure 3A). On the other hand, ΔbipA strain with rluC genomic deletion (ΔrluC/ΔbipA) yielded ribosomal particle distribution without a notable pre-50S peak, which differs from the previous study (Choudhury and Flower, 2015), and concurrent rluC deletion failed to fully compensate the ribosome assembly defect of bipA deficiency (Figure 3A). Note that all the strains demonstrated a similar profile of polysome level.
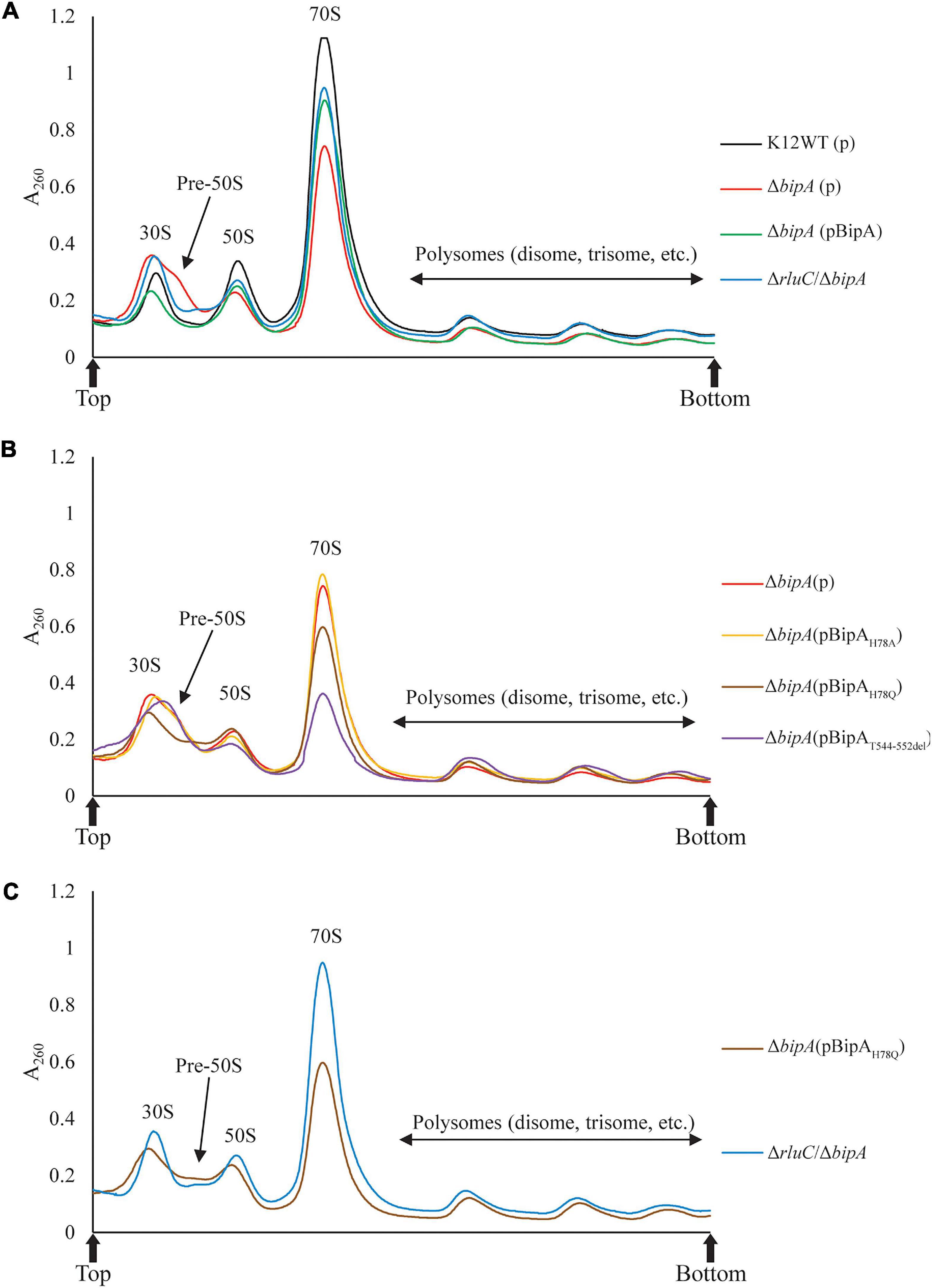
Figure 3. Ribosomal particle distribution showing ribosome assembly defects caused by bipA deletion and BipA mutants. Peaks corresponding to polysomes, 70S ribosome, and free subunits are indicated. (A) Using K12WT (black) as a reference, ΔbipA (red) presented accumulation of ribosomal subunits and reduction of 50S and 70S ribosomal particles, deduced from the higher 30S peak and lower 50S and 70S peaks, respectively. The pre-50S peak appeared as a minor peak between 30S and 50S peaks in ΔbipA ribosomal particle distribution. Although with a lower 70S peak, the profile of ΔbipA (pCA24N-BipA) (green) was similar to K12WT. The ΔrluC/ΔbipA (blue) yielded similar 50S and 70S peaks as K12WT, but similar 30S peak as ΔbipA. Notably, the region between the peak of 30S and 50S subunits was slightly elevated indicating maturing pre-50S. (B) The ΔbipA (pCA24N-BipAH78A) (yellow) yielded almost identical ribosomal particle distribution as ΔbipA. While ΔbipA (pCA24N-BipAH78Q) (brown) had lesser 70S ribosome, the pre-50S peak was not visible. The ΔbipA (pCA24N-BipAT544_D552del) (purple) produced the least 70S and 50S particles with a skewed 30S peak, which may include a large population of pre-50S particles. (C) A comparison between sucrose gradient profiles of the strains ΔrluC/ΔbipA and ΔbipA (pCA24N-BipAH78Q). The data showed similarity in terms of reduced pre-50S with elevated area under the peak between 30S and 50S peaks, likely representing a population of pre-50S that had mature further than what was seen in ΔbipA. The “p” in the bracket alone or preceding “BipA” represents plasmid pCA24N. Peaks corresponding to subunits (30S, pre-50S, and 50S), monosomes (70S), and polysomes are indicated. Top and bottom of each gradient are marked with arrows.
Comparisons among the ΔbipA strains expressing pCA24N-BipA mutants revealed very interesting results on the ribosomal particle distribution (Figure 3B). The expression of BipAH78A in the ΔbipA strain resulted in a very similar profile to that of the control (ΔbipA strain), suggesting that the H78A mutation might have rendered BipA non-functional (Figure 3B). In contrast, the ribosomal particle distribution of ΔbipA strain with the expression of BipAT544_D552del showed the lowest 70S and 50S peaks of all strains as well as an abnormal 30S peak, demonstrating the importance of intact CTL (Figure 3B). The relatively skewed 30S peak might be an accumulation of heterogeneous population of ribosomal subunits and likely includes the pre-50S particles; therefore, the lower 50S peak perhaps resulted from fewer 50S subunits matured in the strain ΔbipA (pCA24N-BipAT544_D552del). The expression of BipAH78Q in the ΔbipA strain generated a medium level of compensation for BipA, demonstrated by the absence of pre-50S peak. In addition, the ribosomal particle distribution was significantly different from that of the ΔbipA (pCA24N-BipAH78A) strain, but similar to that of the ΔrluC/ΔbipA strain, implying that H78Q substitution of BipA might have retained the function of BipA to a certain degree (Figures 3B,C). As rluC deletion is known to compensate the loss of BipA, the similarity in complementation would therefore support the notion that glutamine is able to partially substitute histidine as the catalytic residue of BipA GTPase activity (Koripella et al., 2015).
Taken together, ribosome assembly defect caused by the loss of endogenous BipA could be partially complemented by introducing BipA and the mutant BipAH78Q or suppressed by genomic rluC deletion, but not the BipAH78A and BipAT544_D552del, demonstrating varied and complicated effects of GTP hydrolysis and ribosome binding (e.g., CTL) of BipA in ribosome assembly at low temperature.
Loss of bipA Resulted in Upregulation of Proteins Involved in RNA Metabolism
TMT is an isobaric mass tag-based multiplexed quantitative proteomics method by mass spectrometry (Thompson et al., 2003). Tryptic peptides from different samples are labeled with different isobaric tags for accurate relative quantitation of protein expression across the samples. Using tandem mass spectrometry, proteins can be identified by the fragment ions of peptides, and their expression levels quantitated with reported ion intensities. Next, we sought to further investigate the effect of BipA on protein expression and the rationale behind the suppression of ΔbipA phenotypes by genomic deletion of rluC for cells under suboptimal temperature with TMT approach. Volcano plots of the TMT proteomic datasets were used to determine significant changes in protein expression between different conditions. The cut-off for fold change (FC) with statistical significance (p < 0.05) was determined to be 1.5 (log2 abundance ratio = 0.585). Differentially expressed proteins were shortlisted for further data analysis and interpretation (Supplementary Tables 2–7). The data was reliable as validated by the protein expression level of the deleted genes.
As compared to wild-type strain K12WT, several proteins demonstrated higher expression level in the ΔbipA strain in response to cold stress (Figure 4A). Particular attention was drawn to two proteins DeaD and ObgE given that both have been implicated in 50S subunit biogenesis (Charollais et al., 2004; Sato et al., 2005). Other proteins, with significantly higher expression level in ΔbipA but not implicated in ribosome assembly, include CspA, RNase R, and RpoS. In contrast, by introducing BipA (pCA24N-BipA) to the ΔbipA strain, the expression levels of DeaD, ObgE, CspA, RNase R, and RpoS become similar to those in the strain K12WT (Figure 4B). Furthermore, a comparison of expression levels of these five proteins in the ΔbipA (pCA24N-BipA) and ΔbipA strains showed lower levels for the former (Figure 4C). In addition, the proteins relevant to cell motility were found significantly upregulated while the ΔbipA strains express exogenous BipA (pCA24N-BipA) (Figures 4B,C and Supplementary Tables 3, 4), suggesting that BipA has a direct or indirect influence on bacterial motility. Notably, upregulation of these proteins also rationalized our motility assay for the role of BipA, as observed in Figure 2.
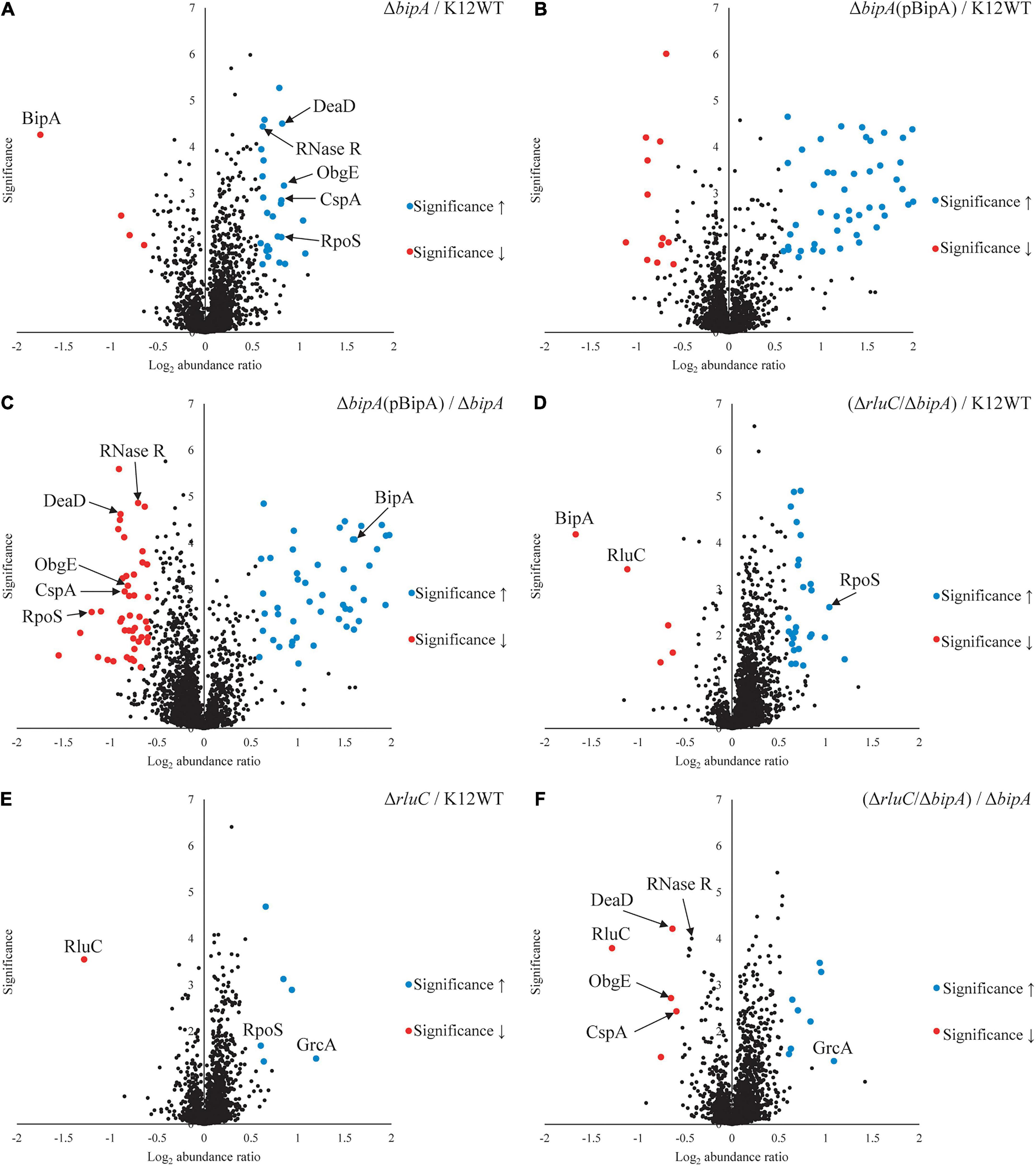
Figure 4. Tandem mass tag-mass spectrometry (TMT-MS) analysis of various strains of E. coli K12 BW25113. A TMT-based quantitative proteomic method was used to determine differential protein expression under suboptimal cell culture condition between wild-type, ΔbipA (pCA24N-BipA), and ΔrluC/ΔbipA strains. (A) A volcano plot showing protein expression level of the strain ΔbipA against the strain K12WT. The bipA was indeed deleted based on significantly low log2 FC. The five proteins DeaD, ObgE, RpoS, CspA, and RNase R yielded significantly higher reads in the strain ΔbipA than the strain K12WT. (B) The expression levels of DeaD, ObgE, RpoS, CspA, and RNase R proteins were not changed between K12WT and ΔbipA (pCA24N-BipA) strain. (C) Comparison of ΔbipA (pCA24N-BipA) against ΔbipA. We put DeaD, ObgE, RpoS, CspA, and RNase R in the negative log2 abundance ratio side of the plot, meaning peptide reads of the proteins were lesser in the presence of pCA24N-BipA. The blue dot labeled as BipA was an indication that BipA was indeed expressed. (D) The rluC deletion in ΔbipA background produced a volcano plot similar to (A), but the DeaD, ObgE, CspA, and RNase R were close to K12WT as differential expressions against K12WT were not detected. As in (A,D), higher readout of RpoS was detected in ΔrluC/ΔbipA. Red dots labeled with BipA and RluC showed that these bipA and rluC were indeed deleted. (E) Genomic deletion of rluC only yielded six differentially expressed proteins relative to K12WT, and out of which, two interesting changes were RpoS and GrcA upregulations. The significant negative log2 abundance ratio of RluC indicates that the gene was indeed removed. (F) Comparisons between ΔrluC/ΔbipA and ΔbipA showed a reduced expression level of the DeaD, ObgE, and CspA to wild-type level. The RNase R readout was found to be reduced by the loss of rluC, close to the cut-off for FC. The pBipA refers to pCA24N-BipA.
Furthermore, the expression levels of these proteins (except for RpoS) in the ΔrluC/ΔbipA double mutant strain were similar to those in the strain K12WT, within cut-off value for different expression level (Figure 4D). In the case of RpoS, it was significantly upregulated in the ΔrluC strain as compared to the strain K12WT (Figure 4E), implying that rluC genomic deletion would affect RpoS expression and thereby leading to an additional effect in the ΔrluC/ΔbipA strain. A comparison of protein expression level of the ΔrluC/ΔbipA strain with that of the ΔbipA strain also revealed significantly lower levels of DeaD, ObgE, and CspA (Figure 4F). Notably, the expression level of RNase R in the ΔrluC/ΔbipA strain was about 0.7 times lower than that in the ΔbipA strain with high statistical significance (log2 abundance ratio = 0.432), indicating that RNase R was indeed downregulated in ΔrluC/ΔbipA, but did not meet the FC cut-off. These observations together demonstrated that the upregulation of the aforementioned proteins likely was ascribed to compensation of the bipA loss in E. coli when cultured at suboptimal temperature.
Interestingly, the upregulation of GrcA, a stress-induced alternate pyruvate formate-lyase subunit, was observed in ΔrluC strains. The mRNA of GrcA can be cleaved by MazF, leading to leaderless mRNA with anti-Shine–Dalgarno sequence removed; therefore, the resultant mRNA is favorably translated by a ribosome (Vesper et al., 2011). The significance of GrcA in bacterial stress and cold shock response remains poorly understood, but it is of interest for further study.
Ribosome Maturation Factor DeaD Is Upregulated in ΔbipA Strain at Suboptimal Growth Temperature
To further validate the upregulation of 50S biogenesis factors detected by TMT-MS, β-galactosidase reporter assay was employed. The lacZ gene was inserted downstream of the target gene in the genome, and its activity (β-galactosidase activity) could be easily measured. Therefore, a fusion design (target gene–lacZ) with the stop codon of the target gene excluded can be used to assess the expression level of this target protein based on the output of the β-galactosidase (LacZ) activity. In our case, the β-galactosidase activity was used to reflect the expression level of the fused DeaD (DeaD–LacZ) protein, which would be consequently used to validate our observation by TMT-MS.
Upon the bipA deletion (ΔbipA), the β-galactosidase activity was dramatically increased for all tests at OD600 ∼0.2, ∼0.5, and ∼1.0, demonstrating DeaD was highly expressed (Figures 5A–C). However, with further deletion of the gene rluC (ΔrluC/ΔbipA), the elevated β-galactosidase activity was reduced, almost to the level of the wild-type strain K12WT. Similarly, exogenous expression of BipA in its deletion strain (ΔbipA + pCA24N-BipA) was also able to complement the effect of bipA deletion on DeaD expression. Collectively, DeaD–LacZ activities for the three strains (K12WT, ΔbipA + pCA24N-BipA, and ΔrluC/ΔbipA) show similar time course, but lower than that for the bipA deletion strain (ΔbipA), suggesting that upregulation of DeaD was triggered by bipA deletion, which can be complemented or suppressed by overexpressing BipA or rluC genomic deletion, respectively. These data are in line with the expression changes in DeaD detected by TMT-MS and are in support of upregulated RNA helicase DeaD, which likely plays an important role in ribosome assembly at low temperature upon the loss of bipA. However, the precise and detailed mechanism remains unknown.
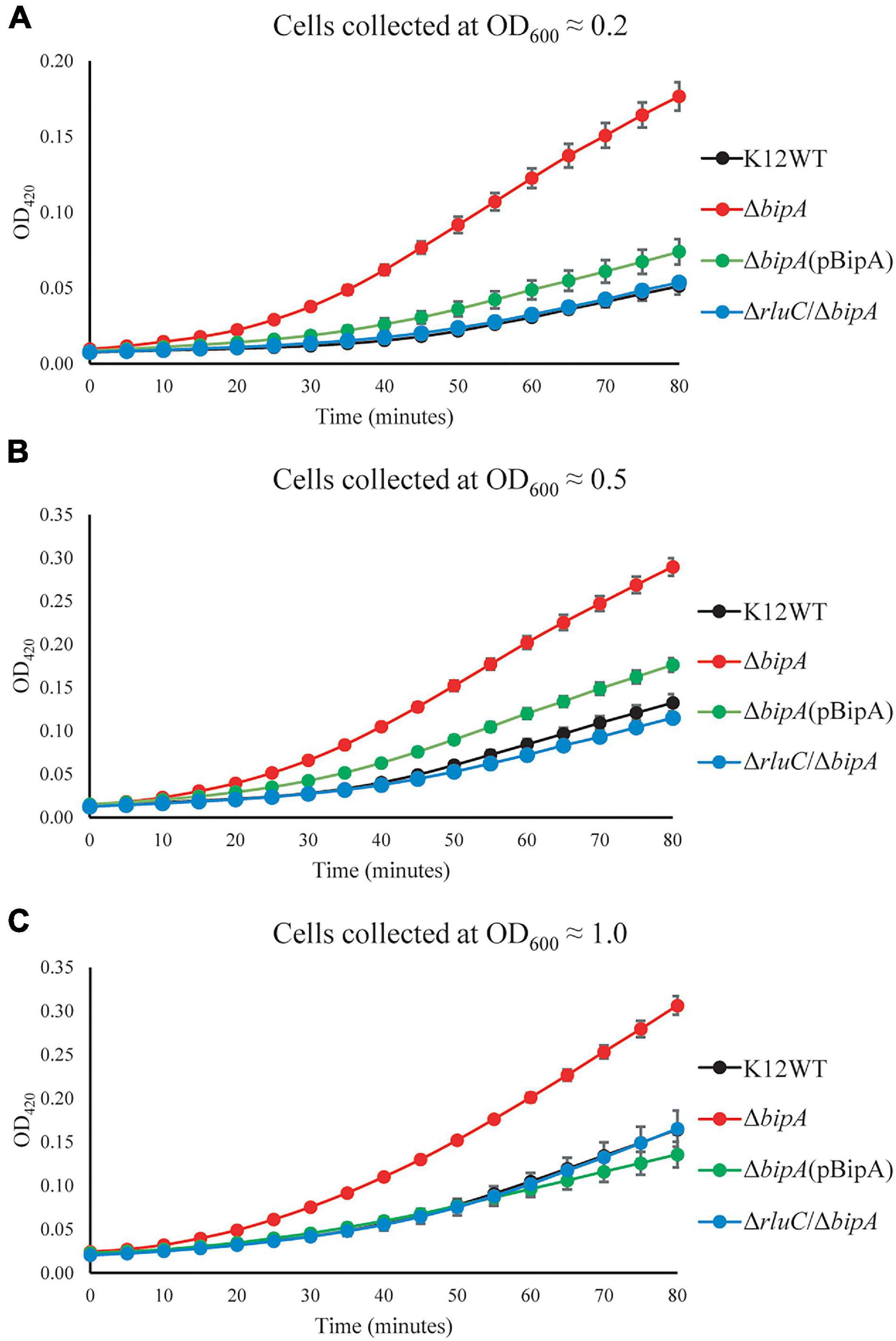
Figure 5. β-galactosidase activity assays demonstrated the upregulation of DeaD in the strain ΔbipA. The cells were harvested at OD600 ≈ 0.2 (A), 0.5 (B), and 1.0 (C), respectively, and the activity was assessed by measuring the breakdown of ONPG through recording OD420. ΔbipA cells (red) harvested at all three OD600 presented the highest β-galactosidase activity, indicated by the significantly stronger OD420 absorbance over time as compared to K12WT (black), ΔbipA (pCA24N-BipA) (green), and ΔrluC/ΔbipA (blue). The pBipA refers to pCA24N-BipA.
BipA Binds Pre-50S Ribosomal Subunit and 70S Ribosome in vitro
We next tested whether BipA can bind pre-50S particle for its proposed function as 50S maturation factor through in vitro reconstitution. First, we mixed the purified BipA, which was pre-incubated with GDPCP with clarified lysate of the ΔbipA cells followed by sucrose gradient centrifugation and ribosome fractionation, and subsequently, western blot was employed to detect BipA. Our data clearly showed that BipA is able to co-sediment with both large and small ribosomal units, as well as the pre-50S ribosomal unit (Figure 6A). BipA could bind to even small ribosomal unit 30S, perhaps through its β-barrel domain II, implying a yet unknown function in bacterial translational machinery ribosome (Figure 6A). Second, we combined BipA pre-incubated with GDPCP and purified pre-50S population of ΔbipA strain, layered on top of 1.1 M sucrose cushion and performed high-speed centrifuge to find out if BipA would co-sediment with pre-50S. As shown in Figure 6B, the results clearly demonstrated that BipA was indeed co-sedimented with pre-50S, corroborating that BipA is capable of binding to the pre-50S ribosomal particle.
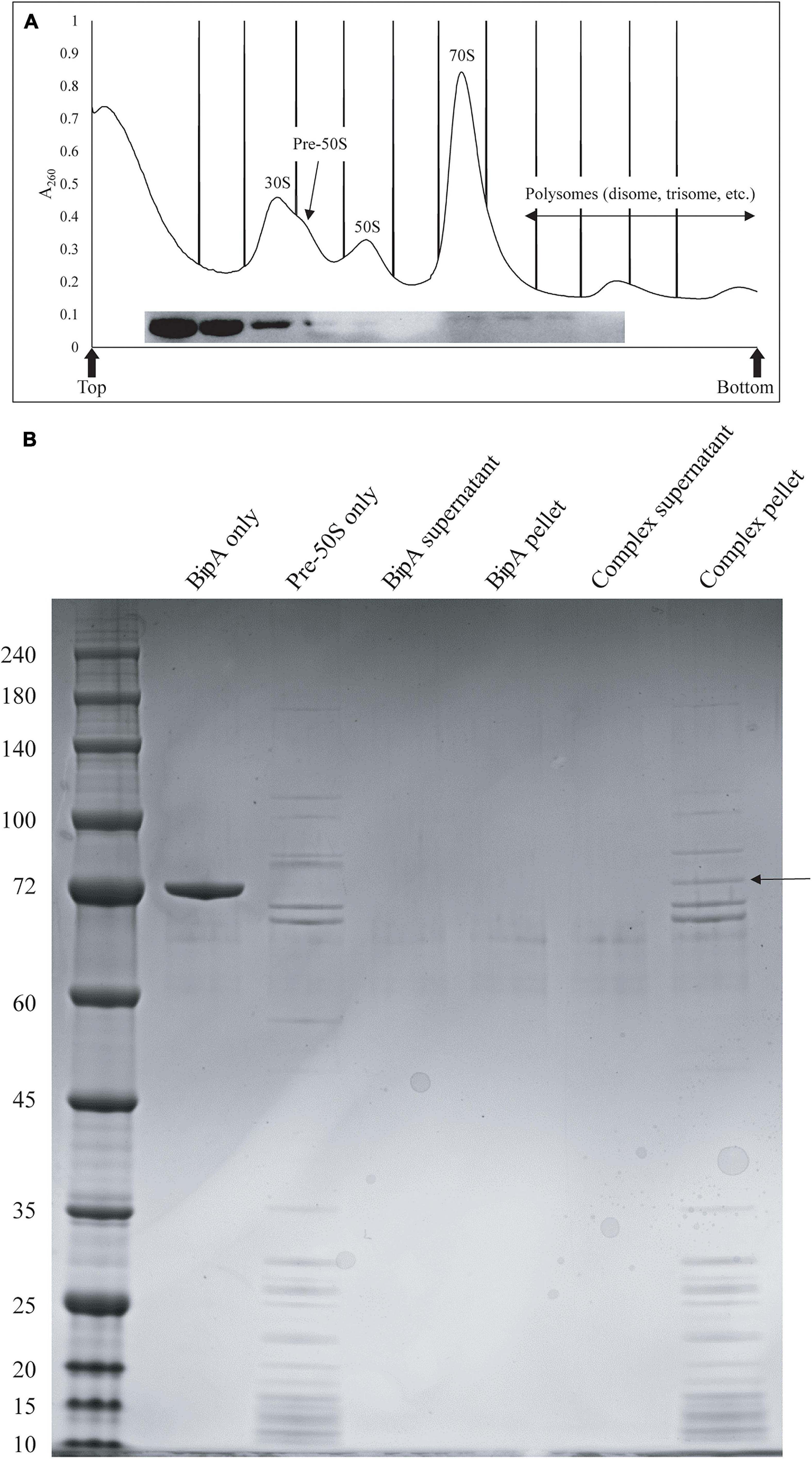
Figure 6. In vitro binding assays showed that BipA pre-incubated with 5X excess GDPCP bound to various ribosomal particles including pre-50S particles. (A) Ribosomal particle distribution of in vitro reconstitution of BipA-GDPCP with ribosomal particles. By analyzing the fractions from ribosomal particle distribution using western blot, most of the BipA pre-incubated with GDPCP were detected in the junk fractions before 30S peak, and the band intensity decreased from 30S toward polysomes. Peaks corresponding to subunits (30S, pre-50S, and 50S), monosomes (70S), and polysomes are indicated. Top and bottom of each gradient are marked with arrows. (B) SDS-PAGE check of ribosome fractions from (A). BipA interaction with pre-50S was observed based on the presence of co-pelleting through sucrose cushion using ultracentrifugation. The band representing co-pelleted BipA is indicated by the black arrow in the “Complex pellet” lane, which consists of pelleted BipA pre-incubated with GDPCP-bound ribosome complex, suggesting that binding occurs.
Discussion
GTP Hydrolysis and CTL of BipA Are Crucial for 50S Biogenesis at Low Temperature
The highly conserved bipA gene has been shown to be significant for bacterial growth and ribosome assembly at suboptimal temperature (Choudhury and Flower, 2015; Ero et al., 2016; Choi and Hwang, 2018; Gibbs et al., 2020). Here, we report that bipA deletion and mutagenesis significantly affect E. coli swimming motility (Figure 2). The experiment was carried out at room temperature (suboptimal for E. coli growth) and revealed that the swimming defect of the ΔbipA strain can be complemented or suppressed by the expression of plasmid-borne BipA (pCA24N-BipA) or the concurrent deletion of rluC gene (ΔrluC/ΔbipA), respectively (Figure 2). The possible reason for this phenotype might be the decelerated global translation due to retardation of ribosome biogenesis, which subsequently shuts down energy-costly pathways, one of which involves the cell motility (Ottemann and Miller, 1997; Martínez-García et al., 2014). The observations of agar plate assay for these mutations correlate with the change of growth trend that can be seen in growth curves (Figure 1) as well as with ribosomal particle distribution observed in Figure 3, suggesting that the loss of bipA leads to a condition affecting growth, motility, ribosome assembly, and protein translation. Indeed, the ribosome assembly defect likely slows down protein translation as the concentrations of 70S ribosomes and polysomes are decreased (Peil et al., 2008; Gibbs et al., 2020). This retardation in protein translation then negatively affects the downstream processes including bacterial growth and motility (Rudra and Warner, 2004).
In this work, we showed that the phenotypes presented by the bipA deletion (ΔbipA) could be complemented by pCA24N-BipA or suppressed by rluC genomic deletion, suggesting that BipA is important for E. coli to thrive at suboptimal temperature, and pseudouridylation on the correct nucleotides (such as RluC involving U955, U2504, and U2580 of 23S rRNA) can be helpful for ribosome biogenesis in E. coli and therefore growth as well as motility. While there is no reported relation to bacterial motility, Choudhury and Flower (2015) proposed that suboptimal temperature caused the ribosome to be dependent on BipA for efficient assembly, and loss of 23S rRNA modification by RluC actually led to an alternative folding pathway that is BipA independent. This further suggests that the improvement of ΔbipA swimming is perhaps not motility regulation related. The unexpected appearance of the chemotactic rings for the strains ΔbipA and ΔbipA with plasmid-expressing BipA mutants after 48-h incubation suggests that the loss of bipA did not diminish swimming motility. Instead, the swimming was delayed probably due to the decreased rate of protein translation and cell growth caused by ribosome assembly defect. This is supported by the microscopy visualization of the bacteria cells grown overnight in liquid culture demonstrating their swimming motility (Supplementary Movies 1–4).
In this study, the plasmid-borne BipA, with H78A or H78Q mutation (catalytic residue H78) or CTL truncation (T544_D552del), was transformed into the ΔbipA strain to examine its effect on complementation for BipA, respectively. The rationale behind the mutations was based on previous mutagenesis studies on catalytic histidine of EF-Tu and EF-G (H84 and H91, respectively) and structural study where the CTL interacts directly with the A-loop of 50S subunit (Supplementary Figure 11). The H84Q substitution in EF-Tu showed a reduction of GTP hydrolysis by 35%, and H84A abolished the GTPase activity (Scarano I, Krab et al., 1995), while the activity of ribosome-associated GTP hydrolysis of EF-G with H91Q substitution was comparable to native protein, and it was found to be defective in organic phosphate release (Koripella et al., 2015). The finding that the expression of BipAH78A did not restore the ribosomal particle distribution as did the wild-type BipA was also observed in a recently published study where the expression of BipAH78A led to slow growth for both WT and ΔbipA strains (Gibbs et al., 2020). On the other hand, Gibbs et al. (2020) expressed suspicion that BipAH78A is able to bind 70S ribosome but is unable to facilitate GTP hydrolysis as well as the subsequent factor release, which ultimately led to halt in translation. The BipAH78Q presented interesting outcomes that could be summarized in three points: (1) similar growth curve as ΔbipA at suboptimal temperature despite longer lag phase at optimum condition (Figures 1C,D); (2) shorter lag phase than ΔbipA (pCA24N-BipAH78A) and ΔbipA (pCA24N-BipAT544_D552del) at suboptimal temperature (Figure 1D); (3) similar ribosomal particle distribution as ΔrluC/ΔbipA, albeit with significantly fewer 70S ribosomal particles (Figure 3C). Based on the aforementioned examples of H84Q and H91Q, glutamine substitution might have rendered BipA less efficient in GTP hydrolysis and/or organic phosphate release (Scarano I, Krab et al., 1995; Koripella et al., 2015), leading to the retention on 70S ribosome for longer period of time and negatively impacting the assembly efficiency. Interestingly, BipAT544_D552del seemed to have an inhibitory effect on E. coli growth and ribosome assembly at suboptimal temperature as deduced from the exacerbated growth defect as compared with the strain ΔbipA (Figure 1D) and lesser 50S and 70S observed in ribosomal particle distribution (Figure 3B). Kumar et al. (2015) reported that the region L543-E553 of CTL projects deep into the peptidyl transferase center (PTC), while the region N536-K542 is critical for ribosome binding. Therefore, the truncation of T544-D552 could potentially play a role in BipA association and function by establishing direct contact with the A-loop of 23S rRNA (Supplementary Figure 11). This corroborates with the study by deLivron et al. (2009) revealing that alanine substitutions of amino acids within the CTL hinder the binding of BipA to the 70S ribosome.
Collectively, growth curves, ribosomal particle distribution, and swimming motility assays demonstrated a correlation whereby the defect in ribosome assembly at suboptimal temperature leads to a delay in growth and swimming motility (Pfennig and Flower, 2001; Choudhury and Flower, 2015; Choi and Hwang, 2018). Perhaps, BipA is a ribosome biogenesis factor that is crucial for ribosome biogenesis at suboptimal temperature, as a previously reported role for BipA is incorporating the ribosomal protein L6 into the 50S ribosome (Choi and Hwang, 2018). In the recent study by Gibbs et al. (2020), structural block 3 of 50S ribosomal subunit demonstrated a growth condition-dependent assembly, including suboptimal temperature. While it was not evident that BipA has a direct role in delaying block 3 folding, the loss of bipA led to the accumulation of pre-50S without ribosomal protein L17, an r-protein associated with block 3 (Gibbs et al., 2020).
Loss of bipA Leads to Upregulation of RNA Metabolism
TMT-MS revealed a significant upregulation of a number of proteins involved in RNA metabolism and chaperoning in the ΔbipA strain, but with expression comparable to the wild-type level in the strains ΔbipA (pCA24N-BipA) and ΔrluC/ΔbipA. Contrary to pCA24N-BipA complementation, the ΔrluC/ΔbipA strain presented an expression of majority of the proteins at above wild-type level while it suppressed ΔbipA phenotypes. Furthermore, the findings from TMT-MS seem to correlate with growth curve, ribosomal particle distribution, and swimming motility assays in a sense that significant changes observed in ΔbipA were found to be reversed in complementation strains. Furthermore, the proteins with significant changes in expression in the ΔbipA strains were involved in ribosome assembly, stress response, and growth. In particular, the expression of DeaD, ObgE, CspA, and RNase R was increased in the ΔbipA strain, but decreased in the complementation strains, indicating their involvement in the phenotypes of the ΔbipA strain. On the other hand, the expression of RpoS was higher in the absence of BipA expression as compared to K12WT, including the ΔrluC/ΔbipA strain. This observation can be explained as the influence of rluC deletion given that the RpoS in the ΔrluC strain was presented higher than the wild-type level, and thus, an additive effect of RpoS reads would be observed in the strain ΔrluC/ΔbipA. To date, no relationship between RluC and RpoS has been reported according to our knowledge, and it is an intriguing topic for further studies. Note that an increased sensitivity to several antibiotics caused by the inactivation of rluC has been reported (Murakami et al., 2005; Toh and Mankin, 2008). This is not surprising as the pseudouridines synthesized by RluC are situated in PTC (U955, U2504, and U2580) where a number of antibiotics bind; hence, the rluC deletion increased the antibiotic susceptibility, and rpoS could be upregulated as a stress response in the ΔrluC strain (Conrad et al., 1998).
Out of the five aforementioned proteins, DeaD and ObgE have been implicated in the biogenesis of ribosome large subunit, suggesting their upregulations may have a functional relationship with BipA in vivo. Similar to BipA, DeaD has been found to be associated with pre-50S particles (Charollais et al., 2004). DeaD has a role in rRNA structural rearrangement using its helicase activity, which aids in 50S biogenesis at low temperature (Charollais et al., 2004). Charollais et al. (2004) also demonstrated that the overexpression of DeaD was able to complement the growth defect of ΔsrmB at low temperature. The finding that the DEAD-box RNA helicase SrmB facilitates 50S biogenesis during early maturation phase (Charollais et al., 2003) suggests an overlap of functions between DeaD and SrmB (Charollais et al., 2004). Such overlapping functions could be possible with BipA and DeaD as well, since both strains ΔdeaD and ΔbipA showed accumulation of pre-50S, and ΔdeaD/ΔbipA double knock-out produced an additive effect (Choudhury and Flower, 2015). From the study by Kitahara and Suzuki (2009), DeaD was shown to be likely to contribute to efficient assembly of circularly permuted rRNAs, implying that the cell would lose the ability to assemble rRNAs with scrambled domains in the absence of DeaD. This finding could be linked to a recent hypothesis on “limited parallel processing” of rRNA and serve as an explanation for the upregulation of DeaD upon the bipA deletion (ΔbipA), which is likely to be a strategy for E. coli to cope with the loss of bipA at suboptimal temperature (Davis et al., 2016). Davis et al. (2016) found that ribosome assembly is dynamic after observing pre-50S from L17-deficient cells mature into the 50S albeit at a slower rate, which the author considered a “limited parallel processing” (Davis et al., 2016). Such behavior confers the bacteria the flexibility in ribosome assembly should there be any factors that are undesirable for ribosome assembly. In addition, Davis et al. (2016) also found that 23S rRNA matures in the form of cooperative folding blocks, whereby different regions of 23S rRNA mature independently in parallel and come into contact with each other to form the tertiary structure. Collectively, their results indicate that ribosome maturation and assembly can occur in multiple pathways, demonstrating the flexibility of bacterial ribosome assembly when there is a bottleneck caused by the shortage of assembly factors or r-proteins.
The importance of RNA secondary structure destabilization at low temperature was demonstrated by Awano et al. (2007), where they presented the growth defect of ΔdeaD at 15°C being complemented by the overexpression of CspA. The CspA is an RNA chaperone that is able to destabilize RNA secondary structure at low temperature with low substrate specificity (Phadtare, 2011). In addition, RNase R, the only 3′–5′ exonuclease in E. coli, is also able to complement the cold sensitivity of ΔdeaD, indicating their tight relationship during cold shock (Awano et al., 2010). The RNase R consists of a helicase and an RNase domain that function independently, and its mutant with only helicase activity was able to complement the cold sensitivity of ΔdeaD, revealing that the helicase activity was the key during cold shock (Awano et al., 2007). Notably, upregulation of RNase R by at least sevenfold is observed during cold shock in E. coli (Cairrão et al., 2003; Guan et al., 2005). Hence, the upregulation of DeaD, CspA, and RNase R in ΔbipA is probably an attempt by the cell to compensate the loss of BipA during 50S biogenesis in which BipA plays a yet unknown role.
In addition to the evidence presented in our work for BipA, the loss of various ribosome assembly factors results in the development of the common phenotype of cold sensitivity coupled with ribosome assembly defect. As summarized in Table 2, 10 out of 24 proteins have previously been reported to play a role in cold sensitivity when deleted or mutated (bold), and several others demonstrated involvement in cold shock. As shown in this study, the deletion of RluC suppresses the cold sensitivity of ΔbipA; the overexpression and deletion of RhlE complement the cold sensitivity of ΔdeaD and ΔsrmB, respectively (Jain, 2008); and LepA is released from the membrane during stress, including cold shock (Pech et al., 2011). Other than these, a recent report revealed that r-protein L6, RplF, an essential protein involved in ribosome biogenesis at low temperature, was missing from the pre-50S fraction of E. coli in the absence of bipA (Bosl and Böck, 1981; Shigeno et al., 2016; Choi and Hwang, 2018). These observations strengthen the connections among cold sensitivity, RNA metabolic process, ribosome biogenesis, and ribosome assembly factors and together support BipA as a bona fide ribosome assembly factor. In addition to the structure of BipA bound to 70S ribosome, it would be of interest to characterize the structure of BipA in complex with the intermediate state of ribosome (like pre-50S in Figure 3) during its biogenesis, which could offer atomic insights into how BipA facilitates ribosome assembly.
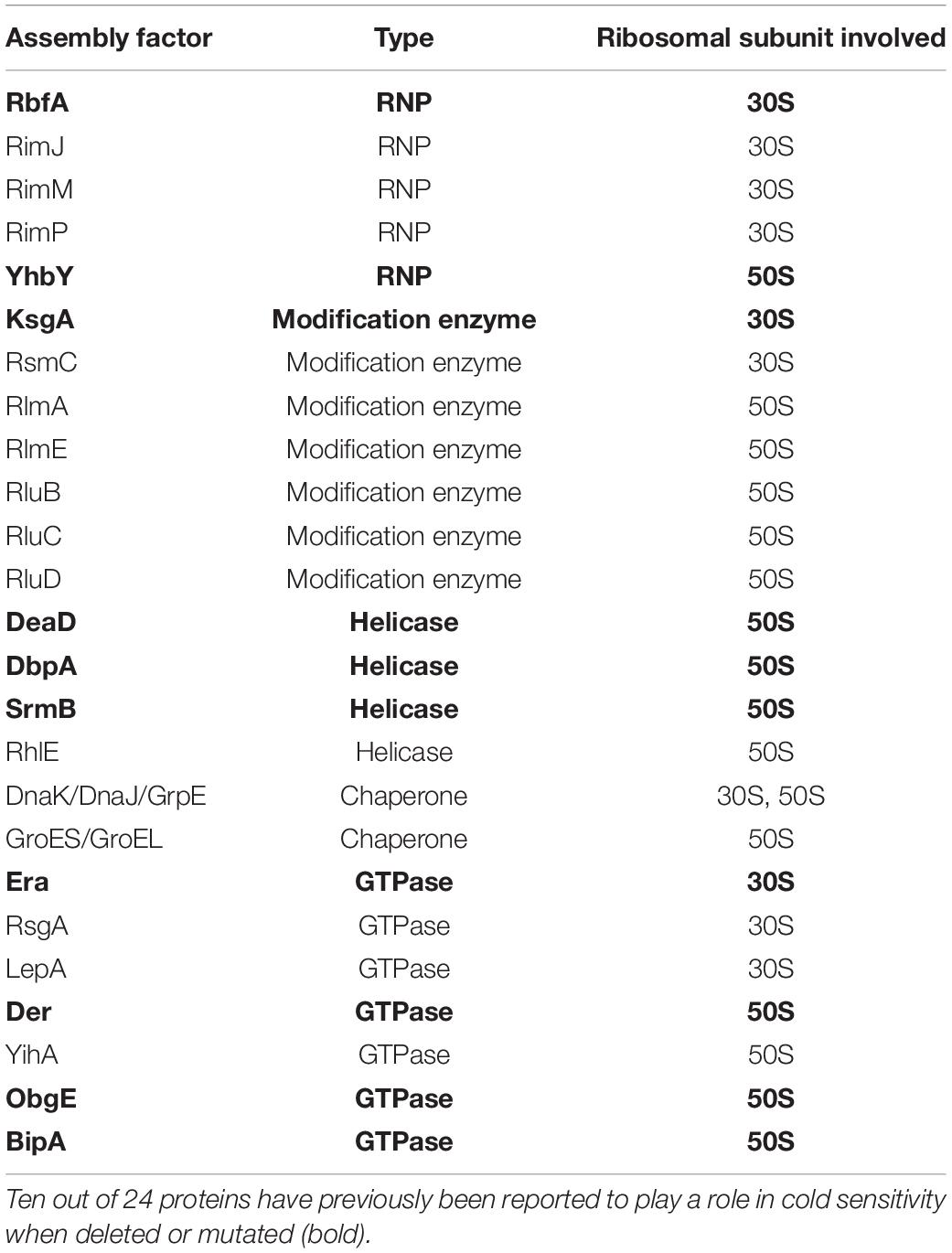
Table 2. Tabulated data adapted from Gibbs and Fredrick (2018), referring to proteins involved in ribosome assembly in E. coli; ObgE is included.
Data Availability Statement
The raw data supporting the conclusions of this article will be made available by the authors, without undue reservation.
Author Contributions
Y-GG directed the project. KJG performed most of the experiments. RE, J-EP, and BK participated in some of the experiments. KJG, X-FY, JZ, SKS, and Y-GG analyzed the data. KJG, RE, X-FY, and Y-GG wrote the manuscript with some input from all other co-authors.
Funding
This work was supported by a Tier I grant RG108/20 (to Y-GG) from the Ministry of Education (MOE) of Singapore.
Conflict of Interest
The authors declare that the research was conducted in the absence of any commercial or financial relationships that could be construed as a potential conflict of interest.
Supplementary Material
The Supplementary Material for this article can be found online at: https://www.frontiersin.org/articles/10.3389/fmicb.2021.686049/full#supplementary-material
References
Awano, N., Rajagopal, V., Arbing, M., Patel, S., Hunt, J., Inouye, M., et al. (2010). Escherichia coli RNase R has dual activities, helicase and RNase. J. Bacteriol. 192, 1344–1352. doi: 10.1128/jb.01368-09
Awano, N., Xu, C., Ke, H., Inoue, K., Inouye, M., and Phadtare, S. (2007). Complementation analysis of the cold-sensitive phenotype of the Escherichia coli csdA deletion strain. J. Bacteriol. 189, 5808–5815. doi: 10.1128/jb.00655-07
Baba, T., Ara, T., Hasegawa, M., Takai, Y., Okumura, Y., Baba, M., et al. (2006). Construction of Escherichia coli K-12 in-frame, single-gene knockout mutants: the Keio collection. Mol. Syst. Biol. 2:2006.0008.
Barria, C., Malecki, M., and Arraiano, C. (2013). Bacterial adaptation to cold. Microbiology 159, 2437–2443. doi: 10.1099/mic.0.052209-0
Bosl, A., and Böck, A. (1981). Ribosomal mutation in Escherichia coli affecting membrane stability. Mol. Gen. Genet. 182, 358–360. doi: 10.1007/bf00269684
Cairrão, F., Cruz, A., Mori, H., and Arraiano, C. M. (2003). Cold shock induction of RNase R and its role in the maturation of the quality control mediator SsrA/tmRNA. Mol. Microbiol. 50, 1349–1360. doi: 10.1046/j.1365-2958.2003.03766.x
Charollais, J., Dreyfus, M., and Iost, I. (2004). CsdA, a cold-shock RNA helicase from Escherichia coli, is involved in the biogenesis of 50S ribosomal subunit. Nucleic Acids Res. 32, 2751–2759. doi: 10.1093/nar/gkh603
Charollais, J., Pflieger, D., Vinh, J., Dreyfus, M., and Iost, I. (2003). The DEAD-box RNA helicase SrmB is involved in the assembly of 50S ribosomal subunits in Escherichia coli. Mol. Microbiol. 48, 1253–1265. doi: 10.1046/j.1365-2958.2003.03513.x
Choi, E., and Hwang, J. (2018). The GTPase BipA expressed at low temperature in Escherichia coli assists ribosome assembly and has chaperone-like activity. J. Biol. Chem. 293, 18404–18419. doi: 10.1074/jbc.ra118.002295
Choudhury, P., and Flower, A. M. (2015). Efficient assembly of ribosomes is inhibited by deletion of bipA in Escherichia coli. J. Bacteriol. 197, 1819–1827. doi: 10.1128/jb.00023-15
Conrad, J., Sun, D., Englund, N., and Ofengand, J. (1998). The rluC gene of Escherichia coli codes for a pseudouridine synthase that is solely responsible for synthesis of pseudouridine at positions 955, 2504, and 2580 in 23 S ribosomal RNA. J. Biol. Chem. 273, 18562–18566. doi: 10.1074/jbc.273.29.18562
Cremer, J., Honda, T., Tang, Y., Wong-Ng, J., Vergassola, M., and Hwa, T. (2019). Chemotaxis as a navigation strategy to boost range expansion. Nature 575, 658–663. doi: 10.1038/s41586-019-1733-y
Davis, J. H., Tan, Y. Z., Carragher, B., Potter, C. S., Lyumkis, D., and Williamson, J. R. (2016). Modular assembly of the bacterial large ribosomal subunit. Cell 167, 1610–1622.e5.
Del Peso Santos, T., Alvarez, L., Sit, B., Irazoki, O., Blake, J., Warner, B. R., et al. (2021). BipA exerts temperature-dependent translational control of biofilm-associated colony morphology in Vibrio cholerae. Elife 10:e60607.
deLivron, M. A., Makanji, H. S., Lane, M. C., and Robinson, V. L. (2009). A novel domain in translational GTPase BipA mediates interaction with the 70S ribosome and influences GTP hydrolysis. Biochemistry 48, 10533–10541. doi: 10.1021/bi901026z
Ero, R., Kumar, V., Chen, Y., and Gao, Y. G. (2016). Similarity and diversity of translational GTPase factors EF-G, EF4, and BipA: From structure to function. RNA Biol. 13, 1258–1273. doi: 10.1080/15476286.2016.1201627
Fan, H., Hahm, J., Diggs, S., Perry, J. J. P., and Blaha, G. (2015). Structural and functional analysis of BipA, a regulator of virulence in enteropathogenic Escherichia coli. J. Biol. Chem. 290, 20856–20864. doi: 10.1074/jbc.m115.659136
Gao, Y.-G., Selmer, M., Dunham, C. M., Weixlbaumer, A., Kelley, A. C., and Ramakrishnan, V. (2009). The structure of the ribosome with elongation factor G trapped in the posttranslocational state. Science 326, 694–699. doi: 10.1126/science.1179709
Gibbs, M. R., and Fredrick, K. (2018). Roles of elusive translational GTPases come to light and inform on the process of ribosome biogenesis in bacteria. Mol. Microbiol. 107, 445–454. doi: 10.1111/mmi.13895
Gibbs, M. R., Moon, K. M., Warner, B. R., Chen, M., Bundschuh, R., Foster, L. J., et al. (2020). Functional analysis of BipA in E. coli reveals the natural plasticity of 50s subunit assembly. J. Mol. Biol. 432, 5259–5272. doi: 10.1016/j.jmb.2020.07.013
Grant, A. J., Farris, M., Alefounder, P., Williams, P. H., Woodward, M. J., and O’Connor, C. D. (2003). Co-ordination of pathogenicity island expression by the BipA GTPase in enteropathogenic Escherichia coli (EPEC). Mol. Microbiol. 48, 507–521. doi: 10.1046/j.1365-2958.2003.t01-1-03447.x
Guan, Y.-X., Pan, H.-X., Gao, Y.-G., Yao, S.-J., and Cho, M.-G. (2005). Refolding and purification of recombinant human interferon-γ expressed as inclusion bodies in Escherichia coli using size exclusion chromatography. Biotechnol. Bioprocess Eng. 10, 122–127. doi: 10.1007/bf02932581
Hauryliuk, V., Atkinson, G. C., Murakami, K. S., Tenson, T., and Gerdes, K. (2015). Recent functional insights into the role of (p) ppGpp in bacterial physiology. Nat. Rev. Microbiol. 13, 298–309. doi: 10.1038/nrmicro3448
Heermann, R., Zeppenfeld, T., and Jung, K. (2008). Simple generation of site-directed point mutations in the Escherichia coli chromosome using Red®/ET® Recombination. Microb. Cell Factor. 7:14. doi: 10.1186/1475-2859-7-14
Jain, C. (2008). The E. coli RhlE RNA helicase regulates the function of related RNA helicases during ribosome assembly. RNA 14, 381–389. doi: 10.1261/rna.800308
Jiang, M., Datta, K., Walker, A., Strahler, J., Bagamasbad, P., Andrews, P. C., et al. (2006). The Escherichia coli GTPase CgtAE is involved in late steps of large ribosome assembly. J. Bacteriol. 188, 6757–6770. doi: 10.1128/jb.00444-06
Kalogeraki, V. S., and Winans, S. C. (1997). Suicide plasmids containing promoterless reporter genes can simultaneously disrupt and create fusions to target genes of diverse bacteria. Gene 188, 69–75. doi: 10.1016/s0378-1119(96)00778-0
Kierzek, E., Malgowska, M., Lisowiec, J., Turner, D. H., Gdaniec, Z., and Kierzek, R. (2013). The contribution of pseudouridine to stabilities and structure of RNAs. Nucleic acids Res. 42, 3492–3501. doi: 10.1093/nar/gkt1330
Kinosita, Y., Ishida, T., Yoshida, M., Ito, R., Morimoto, Y. V., Goto, K., et al. (2020). Distinct chemotactic behavior in the original Escherichia coli K-12 depending on forward-and-backward swimming, not on run-tumble movements. Sci. Rep. 10:15887.
Kitahara, K., and Suzuki, T. (2009). The ordered transcription of RNA domains is not essential for ribosome biogenesis in Escherichia coli. Mol. Cell 34, 760–766. doi: 10.1016/j.molcel.2009.05.014
Koripella, R. K., Holm, M., Dourado, D., Mandava, C. S., Flores, S., and Sanyal, S. (2015). A conserved histidine in switch-II of EF-G moderates release of inorganic phosphate. Sci. Rep. 5:12970.
Koster, D. A., Mayo, A., Bren, A., and Alon, U. (2012). Surface growth of a motile bacterial population resembles growth in a chemostat. J. Mol. Biol. 424, 180–191. doi: 10.1016/j.jmb.2012.09.005
Krishnan, K., and Flower, A. M. (2008). Suppression of ΔbipA phenotypes in Escherichia coli by abolishment of pseudouridylation at specific sites on the 23S rRNA. J. Bacteriol. 190, 7675–7683. doi: 10.1128/jb.00835-08
Kumar, V., Chen, Y., Ero, R., Ahmed, T., Tan, J., Li, Z., et al. (2015). Structure of BipA in GTP form bound to the ratcheted ribosome. Proc. Natl. Acad. Sci. U.S.A. 112, 10944–10949. doi: 10.1073/pnas.1513216112
Kumar, V., Ero, R., Ahmed, T., Goh, K. J., Zhan, Y., Bhushan, S., et al. (2016). Structure of the GTP Form of Elongation Factor 4 (EF4) Bound to the Ribosome. J. Biol. Chem. 291, 12943–12950. doi: 10.1074/jbc.m116.725945
Liu, W., Cremer, J., Li, D., Hwa, T., and Liu, C. (2019). An evolutionarily stable strategy to colonize spatially extended habitats. Nature 575, 664–668. doi: 10.1038/s41586-019-1734-x
Martínez-García, E., Nikel, P. I., Chavarría, M., and de Lorenzo, V. (2014). The metabolic cost of flagellar motion in Pseudomonas putida KT 2440. Environ. Microbiol. 16, 291–303. doi: 10.1111/1462-2920.12309
Murakami, K., Ono, T., Viducic, D., Kayama, S., Mori, M., Hirota, K., et al. (2005). Role for rpoS gene of Pseudomonas aeruginosa in antibiotic tolerance. FEMS Microbiol. Lett. 242, 161–167.
Neidig, A., Yeung, A. T., Rosay, T., Tettmann, B., Strempel, N., Rueger, M., et al. (2013). TypA is involved in virulence, antimicrobial resistance and biofilm formation in Pseudomonas aeruginosa. BMC Microbiol. 13:77. doi: 10.1186/1471-2180-13-77
Ottemann, K. M., and Miller, J. F. (1997). Roles for motility in bacterial–host interactions. Mol. Microbiol. 24, 1109–1117. doi: 10.1046/j.1365-2958.1997.4281787.x
Park, J. E., Dutta, B., Tse, S. W., Gupta, N., Tan, C. F., Low, J. K., et al. (2019). Hypoxia-induced tumor exosomes promote M2-like macrophage polarization of infiltrating myeloid cells and microRNA-mediated metabolic shift. Oncogene 38, 5158–5173. doi: 10.1038/s41388-019-0782-x
Pech, M., Karim, Z., Yamamoto, H., Kitakawa, M., Qin, Y., and Nierhaus, K. H. (2011). Elongation factor 4 (EF4/LepA) accelerates protein synthesis at increased Mg2+ concentrations. Proc. Natl. Acad. Sci. U.S.A. 108, 3199–3203. doi: 10.1073/pnas.1012994108
Peil, L., Virumäe, K., and Remme, J. (2008). Ribosome assembly in Escherichia coli strains lacking the RNA helicase DeaD/CsdA or DbpA. FEBS J. 275, 3772–3782. doi: 10.1111/j.1742-4658.2008.06523.x
Pfennig, P., and Flower, A. (2001). BipA is required for growth of Escherichia coli K12 at low temperature. Mol. Genet. Genom. 266, 313–317. doi: 10.1007/s004380100559
Phadtare, S. (2011). Unwinding activity of cold shock proteins and RNA metabolism. RNA Biol. 8, 394–397. doi: 10.4161/rna.8.3.14823
Phadtare, S., Hwang, J., Severinov, K., and Inouye, M. (2003). CspB and CspL, thermostable cold-shock proteins from Thermotoga maritima. Genes Cells 8:801. doi: 10.1046/j.1365-2443.2003.00675.x
Philippe, N., Alcaraz, J.-P., Coursange, E., Geiselmann, J., and Schneider, D. (2004). Improvement of pCVD442, a suicide plasmid for gene allele exchange in bacteria. Plasmid 51, 246–255. doi: 10.1016/j.plasmid.2004.02.003
Prud’homme-Généreux, A., Beran, R. K., Iost, I., Ramey, C. S., Mackie, G. A., and Simons, R. W. (2004). Physical and functional interactions among RNase E, polynucleotide phosphorylase and the cold-shock protein, CsdA: evidence for a ‘cold shock degradosome’. Mol. Microbiol. 54, 1409–1421. doi: 10.1111/j.1365-2958.2004.04360.x
Qi, S. Y., Li, Y., Szyroki, A., Giles, I. G., Moir, A., and David O’Connor, C. (1995). Salmonella typhimurium responses to a bactericidal protein from human neutrophils. Mol. Microbiol. 17, 523–531. doi: 10.1111/j.1365-2958.1995.mmi_17030523.x
Resch, A., Većerek, B., Palavra, K., and Bläsi, U. (2010). Requirement of the CsdA DEAD-box helicase for low temperature riboregulation of rpoS mRNA. RNA Biol. 7, 796–802. doi: 10.4161/rna.7.6.13768
Rudra, D., and Warner, J. R. (2004). What better measure than ribosome synthesis? Genes Dev. 18, 2431–2436. doi: 10.1101/gad.1256704
Sato, A., Kobayashi, G., Hayashi, H., Yoshida, H., Wada, A., Maeda, M., et al. (2005). The GTP binding protein Obg homolog ObgE is involved in ribosome maturation. Genes Cells 10, 393–408. doi: 10.1111/j.1365-2443.2005.00851.x
Scarano, G. I., Krab, M., Bocchini, V., and Parmeggiani, A. (1995). Relevance of histidine-84 in the elongation factor Tu GTPase activity and in poly (Phe) synthesis: its substitution by glutamine and alanine. FEBS Lett. 365, 214–218. doi: 10.1016/0014-5793(95)00469-p
Schaefer, J., Jovanovic, G., Kotta-Loizou, I., and Buck, M. (2016). Single-step method for β-galactosidase assays in Escherichia coli using a 96-well microplate reader. Anal. Biochem. 503, 56–57. doi: 10.1016/j.ab.2016.03.017
Schmeing, T. M., Voorhees, R. M., Kelley, A. C., Gao, Y. G., Murphy, F. V. IV, Weir, J. R., et al. (2009). The crystal structure of the ribosome bound to EF-Tu and aminoacyl-tRNA. Science 326, 688–694.
Selmer, M., Gao, Y. G., Weixlbaumer, A., and Ramakrishnan, V. (2012). Ribosome engineering to promote new crystal forms. Acta Crystallogr. D Biol. Crystallogr. 68(Pt 5), 578–583. doi: 10.1107/s0907444912006348
Shigeno, Y., Uchiumi, T., and Nomura, T. (2016). Involvement of ribosomal protein L6 in assembly of functional 50S ribosomal subunit in Escherichia coli cells. Biochem. Biophys. Res. Commun. 473, 237–242. doi: 10.1016/j.bbrc.2016.03.085
Tanaka, Y., Sakamoto, S., Kuroda, M., Goda, S., Gao, Y. G., Tsumoto, K., et al. (2008). A helical string of alternately connected three-helix bundles for the cell wall-associated adhesion protein Ebh from Staphylococcus aureus. Structure 16, 488–496. doi: 10.1016/j.str.2007.12.018
Thompson, A., Schäfer, J., Kuhn, K., Kienle, S., Schwarz, J., Schmidt, G., et al. (2003). Tandem mass tags: a novel quantification strategy for comparative analysis of complex protein mixtures by MS/MS. Anal. Chem. 75, 1895–1904. doi: 10.1021/ac0262560
Toh, S.-M., and Mankin, A. S. (2008). An indigenous posttranscriptional modification in the ribosomal peptidyl transferase center confers resistance to an array of protein synthesis inhibitors. J. Mol. Biol. 380, 593–597. doi: 10.1016/j.jmb.2008.05.027
Uppal, S., and Jawali, N. (2015). Cyclic AMP receptor protein (CRP) regulates the expression of cspA, cspB, cspG and cspI, members of cspA family, in Escherichia coli. Arch. Microbiol. 197, 497–501. doi: 10.1007/s00203-015-1085-4
Vesper, O., Amitai, S., Belitsky, M., Byrgazov, K., Kaberdina, A. C., Engelberg-Kulka, H., et al. (2011). Selective translation of leaderless mRNAs by specialized ribosomes generated by MazF in Escherichia coli. Cell 147, 147–157. doi: 10.1016/j.cell.2011.07.047
Yang, C., Cui, C., Ye, Q., Kan, J., Fu, S., Song, S., et al. (2017). Burkholderia cenocepacia integrates cis-2-dodecenoic acid and cyclic dimeric guanosine monophosphate signals to control virulence. Proc. Natl. Acad. Sci. U.S.A. 114, 13006–13011. doi: 10.1073/pnas.1709048114
Yu, Y., Wu, Y., Cao, B., Gao, Y.-G., and Yan, X. (2015). Adjustable bidirectional extracellular electron transfer between Comamonas testosteroni biofilms and electrode via distinct electron mediators. Electrochem. Commun. 59, 43–47. doi: 10.1016/j.elecom.2015.07.007
Keywords: BipA, ribosome biogenesis, large subunit maturation, conditional protein expression, stress response, suboptimal temperature growth
Citation: Goh KJ, Ero R, Yan X-F, Park J-E, Kundukad B, Zheng J, Sze SK and Gao Y-G (2021) Translational GTPase BipA Is Involved in the Maturation of a Large Subunit of Bacterial Ribosome at Suboptimal Temperature. Front. Microbiol. 12:686049. doi: 10.3389/fmicb.2021.686049
Received: 26 March 2021; Accepted: 09 June 2021;
Published: 13 July 2021.
Edited by:
Amparo Pascual-Ahuir, Universitat Politècnica de València, SpainReviewed by:
Kaoru Nakasone, Kindai University, JapanCinthia Nuñez, Instituto de Biotecnología, Mexico
Copyright © 2021 Goh, Ero, Yan, Park, Kundukad, Zheng, Sze and Gao. This is an open-access article distributed under the terms of the Creative Commons Attribution License (CC BY). The use, distribution or reproduction in other forums is permitted, provided the original author(s) and the copyright owner(s) are credited and that the original publication in this journal is cited, in accordance with accepted academic practice. No use, distribution or reproduction is permitted which does not comply with these terms.
*Correspondence: Yong-Gui Gao, eWdhb0BudHUuZWR1LnNn