- 1CAS Key Laboratory of Special Pathogens and Biosafety, Wuhan Institute of Virology, Chinese Academy of Sciences, Wuhan, China
- 2University of Chinese Academy of Sciences, Beijing, China
- 3National Laboratory of Biomacromolecules, Institute of Biophysics, Chinese Academy of Sciences, Beijing, China
- 4Shanghai Center for Systems Biomedicine, Key Laboratory of Systems Biomedicine (Ministry of Education), Shanghai Jiao Tong University, Shanghai, China
- 5Guangdong Province Key Laboratory of TB Systems Biology and Translational Medicine, Foshan, China
After several decades of use, trimethoprim (TMP) remains one of the key access antimicrobial drugs listed by the World Health Organization. To circumvent the problem of trimethoprim resistance worldwide, a better understanding of drug-resistance mechanisms is required. In this study, we screened the single-gene knockout library of Escherichia coli, and identified mgrB and other several genes involved in trimethoprim resistance. Subsequent comparative transcriptional analysis between ΔmgrB and the wild-type strain showed that expression levels of phoP, phoQ, and folA were significantly upregulated in ΔmgrB. Further deleting phoP or phoQ could partially restore trimethoprim sensitivity to ΔmgrB, and co-overexpression of phoP/Q caused TMP resistance, suggesting the involvement of PhoP/Q in trimethoprim resistance. Correspondingly, MgrB and PhoP were shown to be able to modulated folA expression in vivo. After that, efforts were made to test if PhoP could directly modulate the expression of folA. Though phosphorylated PhoP could bind to the promotor region of folA in vitro, the former only provided a weak protection on the latter as shown by the DNA footprinting assay. In addition, deleting the deduced PhoP box in ΔmgrB could only slightly reverse the TMP resistance phenotype, suggesting that it is less likely for PhoP to directly modulate the transcription of folA. Taken together, our data suggested that, in E. coli, MgrB affects susceptibility to trimethoprim by modulating the expression of folA with the involvement of PhoP/Q. This work broadens our understanding of the regulation of folate metabolism and the mechanisms of TMP resistance in bacteria.
Introduction
Folic acid is essential for the survival of various living organisms. Unlike bacteria, mammals can take folic acid from food without creating folic acid from de novo synthesis. Therefore, the key enzyme in bacterial de novo synthesis of folic acid is an ideal target for antibacterial drug design (Bermingham and Derrick, 2002). To date, thousands of folate antagonists have been designed for bacterial dihydropteroate synthase (DHPS) and dihydrofolate reductase (DHFR) (Estrada et al., 2016), of which sulfamethoxazole (SMX) and trimethoprim (TMP) are most widely used. TMP can specifically inhibit the activity of DHFR and inhibit the growth of bacteria by blocking the synthesis of folate (Hitchings, 1973). TMP was first used clinically in 1962 in the treatment of Proteus septicemia (Noall et al., 1962). Since 1967, Co-trimoxazole, a fixed-dose combination of trimethoprim (TMP) and SMX, has been developed and used as a broad-spectrum antibacterial drug (Bushby and Barnett, 1967; Csonka and Knight, 1967; Akinkugbe et al., 1968; Bushby and Hitchings, 1968; Reeves, 1971). Initially, co-trimoxazole was used to treat urinary tract infections and lower respiratory tract infections (Hughes, 1969; Reeves et al., 1969), but later on, it was found to have good prophylactic activity against Pneumocystis jiroveci pneumonia (Hughes et al., 1974). The use of co-trimoxazole greatly increased with the emergence of the HIV epidemic in the 1980s since it provided good protection against various opportunistic infections during the pre-antiretroviral therapy (ART) era (DeHovitz et al., 1986; Youle et al., 1990; Canessa et al., 1992; Carr et al., 1992; Plourde et al., 1992; Schneider et al., 1992). In recent years, researchers have found that co-trimoxazole is able to prevent the development of tuberculosis in HIV-positive patients, especially in those who have not received prior ART (Hasse et al., 2014).
However, the widespread use of co-trimoxazole has resulted in increasing resistance, just like many other antimicrobial agents (Huovinen et al., 1995). So far, known mechanisms of TMP resistance include: (1) plasmid-mediated drug-insusceptible variants of chromosomal dihydrofolate reductase (DHFR) (Fleming et al., 1972; Amyes and Smith, 1974; Skold and Widh, 1974; Then, 1993), which was considered as the target protein of the TMP, encoded by folA in Escherichia coli; (2) chromosomal location of transposon Tn7 (Lichtenstein and Brenner, 1981); (3) Mutation of the thymidylate synthase encoding gene thyA (Chatterjee et al., 2008); (4) overproduction of DHFR, or alteration of the enzyme, or a combination of both (Sirotnak and McCuen, 1973; Sheldon and Brenner, 1976; Smith and Calvo, 1982; Palmer and Kishony, 2014). Even so, at present, co-trimoxazole is still one of the first-line drugs for the treatment of urinary tract infections, skin and soft tissue infections, and community-acquired methicillin resistant Staphylococcus aureus infections (Gupta et al., 2011). To combat the problem, it is important to understand resistance-mechanism and accordingly develop alternative strategies.
The PhoP/Q pathway is a well-known two-component signaling system found in Enterobacteriaceae, such as E. coli, Salmonella typhimurium, and related bacteria. PhoQ, a transmembrane histidine kinase, is stimulated by exposure to low extracellular Mg2+, certain cationic antimicrobial peptides, and low pH (Groisman, 2001; Bader et al., 2005; Cho et al., 2006). Once activated, PhoQ autophosphorylates and transfers the phosphoryl group to PhoP, which then induces the expression of its target genes (Igo et al., 1989). It has been proposed that phosphorylated PhoP binds to a hexanucleotide direct repeat [(T/G)GTTTA] separated by five nucleotides, the so-called “PhoP box” (Kato et al., 1999). The PhoP box was located at various distances from the −10 region recognized by RNA polymerase in the promoters of PhoP target genes (Kato et al., 1999; Yamamoto et al., 2002; Minagawa et al., 2003; Zwir et al., 2005, 2012; Li et al., 2008; Zhao et al., 2008; Harari et al., 2010). One of the targets of PhoP is MgrB, a broadly conserved small transmembrane protein, which inhibits the kinase activity of PhoQ via a negative feedback loop (Lippa and Goulian, 2009). PhoQ is bifunctional: when its kinase activity is inhibited, it induces the dephosphorylation of PhoP (Yeo et al., 2012). Thus, MgrB drives the inactivation of PhoP (Lippa and Goulian, 2009; Salazar et al., 2016). Previous studies have demonstrated that the PhoP/Q pathway functions as a critical part of the survival stress response under disparate environmental conditions (Eguchi et al., 2011); this pathway is also involved in drug resistance and virulence in many pathogenic bacteria (Gunn and Miller, 1996; Gunn et al., 1998; Mitrophanov et al., 2008; Cheng et al., 2010; Cannatelli et al., 2013, 2014; Hicks et al., 2015; Aires et al., 2016). Recently, it has been shown that MgrB is also involved in the resistiance of Klebsiella pneumoniae to polymyxins (polymyxin B and colistin, also known as polymyxin E) (Cannatelli et al., 2013; Aires et al., 2016).
In this study, many genes involved in TMP resistance, including mgrB, were identified by screening the E. coli single-gene knockout library (Baba et al., 2006). And the mechanism of TMP resistance induced by deletion of mgrB was investigated by subsequent microbiological and biochemical experiments.
Results
Screening of Genes Associated With TMP Susceptibility
To identify genes in the E. coli genome potentially involved in resistance or susceptibility to TMP, we screened 3985 single-gene knockout mutants of E. coli K-12 BW25113 (the Keio collection). Deletion of 31 of these genes led to changes in TMP susceptibility (Supplementary Table 1). To verify these results, all in-frame deletion mutants were reconstructed in E. coli K-12 W3110, along with the corresponding overexpression and complemented gene strains. We tested the susceptibility of these strains to TMP. We found that the deletion of five genes (rfaH, acrB, acrA, phoP, and phoQ) increased sensitivity to TMP, while the deletion of six other genes (mgrB, ymjC, rcsC, tehB, cysB, and torR) decreased sensitivity to TMP (Table 1). Of the deletion mutants tested, two (acrA and acrB) encode drug efflux proteins (Ma et al., 1995), which might affect TMP susceptibility. However, all other tested genes encode proteins which are not directly related to bacterial drug resistance. For example, ymjC encodes an putative oxidoreductase; rcsC encodes a histidine kinase of the RCS regulatory cascade; tehB encodes a tellurite methyltransferase; rfaH encodes a transcription antitermination protein; cysB encodes a transcriptional regulator involved in sulfur utilization; and torR encodes the response regulator of the TorT-TorS-TorR signal transduction system.
The ΔmgrB mutant was shown to be moderately more resistant to TMP (an eight-fold increase in TMP MIC compared with the wild-type strain), while the ΔphoP and ΔphoQ mutants were slightly more sensitive to TMP (a two-fold decrease in TMP MIC compared with the wild-type strain).
Deletion of phoP or phoQ Restores TMP Sensitivity to ΔmgrB
It is known that mgrB encodes MgrB, which feeds back to inhibit the kinase activity of PhoQ, so the MgrB-dependent negative feedback loop plays a crucial role in E. coli PhoQ-PhoP signaling (Lippa and Goulian, 2009; Salazar et al., 2016). We thus hypothesized that the TMP-resistant phenotype resulting from mgrB deletion might be mediated by the PhoP/Q pathway. To prove this hypothesis, we constructed two double-knockout mutants (E. coli W3110 ΔmgrB ΔphoQ and E. coli W3110 ΔmgrB ΔphoP) and tested their susceptibilities to TMP. The results showed that the TMP MICs of both double knockout mutants were identical to that of the wild-type strain, two times that of ΔphoP or ΔphoQ, demonstrating that disruption of the PhoP/Q pathway could partially reversed the effects of mgrB deletion (Table 2).
Deletion of pmrK Does Not Affect Susceptibility of E. coli to TMP
Previous studies have shown that the deletion of mgrB led to the upregulation of the PhoQ/PhoP pathway and the activation of pmrK (part of pmrHFIJKLM operon), eventually causing resistance to polymyxins via modifications of the lipopolysaccharide target in K. pneumoniae (Helander et al., 1996; Cannatelli et al., 2013). To investigate whether pmrK was also involved in the TMP resistance of E. coli ΔmgrB, we both knocked out and overexpressed pmrK in E. coli W3110. Our results showed that pmrK did not affect TMP susceptibility in E. coli (Table 2). In addition, the ΔmgrB mutant was not more resistant to polymyxin B or colistin than was the wild-type strain (Table 3).
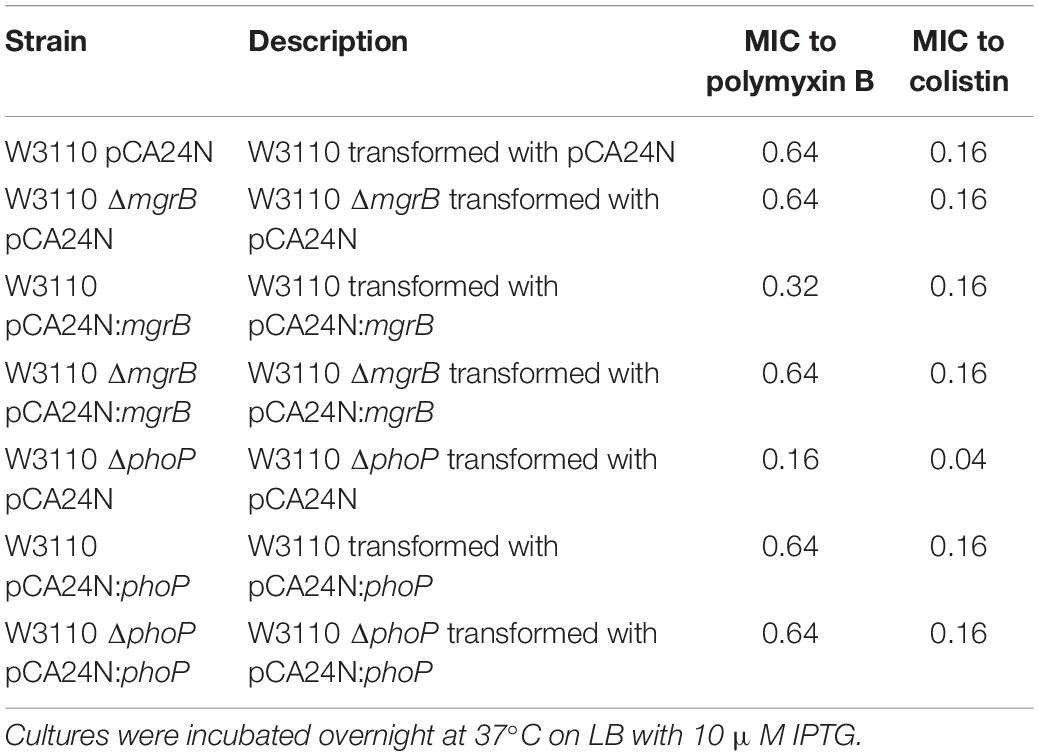
Table 3. MICs (μg ml–1) to polymyxins in mgrB-related and phoP-related strains of E. coli K-12 W3110.
Overexpression of tolC Does Not Affect Susceptibility of E. coli to TMP
TolC, an outer membrane protein, is part of the AcrAB-TolC drug efflux system (Tikhonova and Zgurskaya, 2004). This system plays an important role in both intrinsic and acquired resistance to a wide variety of currently available antimicrobial agents (Du et al., 2014). Previous studies have identified a putative PhoP binding site (PhoP box) in the tolC promoter region (Eguchi et al., 2003). As our results indicated that PhoP/Q was involved in the TMP resistance of the ΔmgrB mutant, we speculated that the PhoP/Q pathway might affect TMP sensitivity by modulating the expression of tolC. To test this theory, we overexpressed tolC in E. coli W3110. We found that the overexpression of tolC did not affect TMP susceptibility (Table 2). In addition, our qRT-PCR results suggested that the deletion of mgrB did not affect tolC expression (Figure 1).
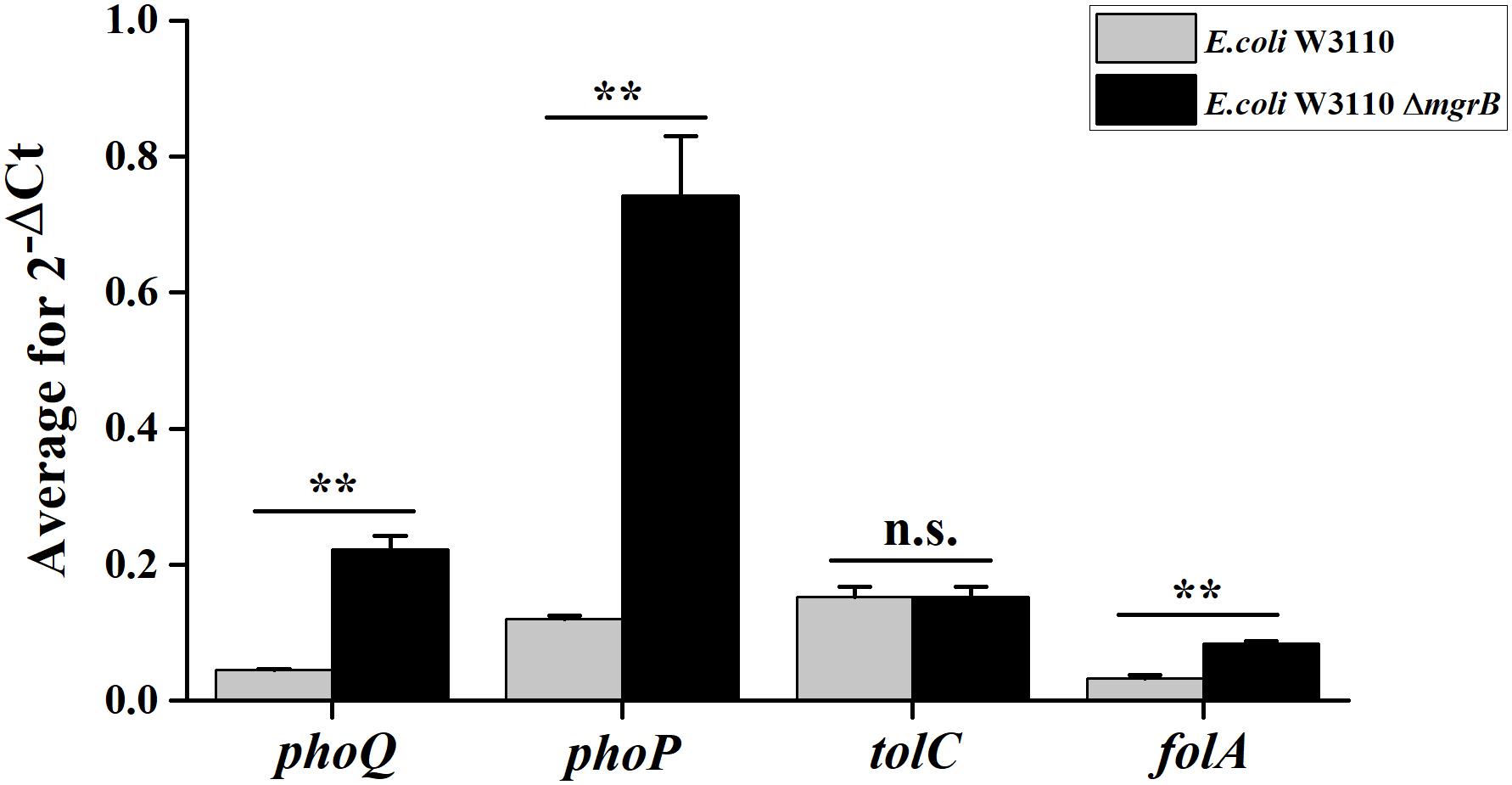
Figure 1. Average transcription levels of the genes phoP, phoQ, folA, and tolC in E. coli W3110 (wild type) and E. coli W3110 ΔmgrB, as measured with qRT-PCR. Expression levels of gapA were normalized as an endogenous control. Data represent mean ± SD from three independent experiments. P values were calculated using t tests (*P < 0.05; **P < 0.01; ***P < 0.001).
Transcriptome Analysis of the ΔmgrB Mutant
To further understand how mgrB deletion led to TMP resistance in E. coli, we compared the global transcription profiles of the E. coli K12 W3110 ΔmgrB mutant and the wild-type E. coli strain using RNA-seq (PRJNA523715). We identified 518 significantly upregulated genes and 433 significantly downregulated genes (951 in total; Supplementary Table 2). As expected, the deletion of mgrB was followed by a significant upregulation of phoQ and phoP. Interestingly, folA also was upregulated. The folA gene encodes the DHFR of E. coli, the target of TMP (Table 4). We verified these results with qRT-PCR (Figure 1).
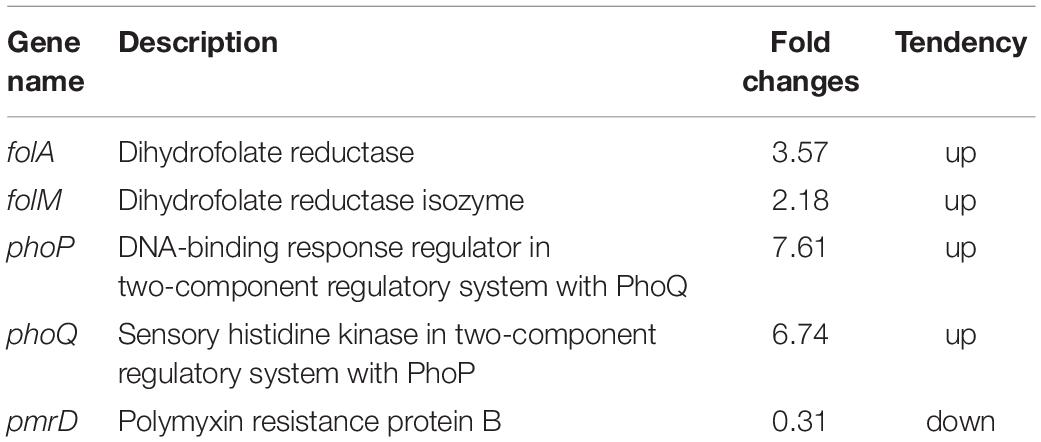
Table 4. Genes differentially regulated in E. coli K-12 W3110 ΔmgrB compared with wild-type E. coli K-12 W3110 by comparative transcriptional analyses.
Co-overexpression of phoP and phoQ Causes TMP Resistance
To further test the involvement of PhoP/Q in the TMP resistant phenotype of ΔmgrB, we constructed three strains of E. coli W3110, overexpressing phoP, phoQ, or phoPQ, and tested their sensitivity to TMP. Our results showed that while overexpressing phoP or phoQ did not affect TMP sensitivity, co-overexpression of phoP and phoQ caused TMP resistance (Table 2).
PhoP Recognizes the folA Promoter in vitro
As the deletion of mgrB was followed by the increased expression of folA, phoP, and phoQ, we hypothesized that MgrB might modulate the expression of folA via the PhoP/Q pathway. To verify this hypothesis, we used electrophoretic mobility shift assay (EMSA) assay to test whether PhoP recognized and bound the promoter regions of folA directly, by using the promoter region of mgrB, a known PhoP substrate (Kato et al., 1999), as the positive control. Phosphorylated PhoP with a poly-histidine tag was prepared, and the biotin-labeled DNA probe containing the promoter region of folA (−231/+26) were amplified by PCR using 3′end biotin-labeled primers. After combined incubation and subsequent electrophoresis, it was clearly that PhoP hindered the mobility of the probe, and the hindering effect increased correlating to the quantities of PhoP, suggesting that PhoP specifically recognize and bind the promoter region of folA (Figure 2).
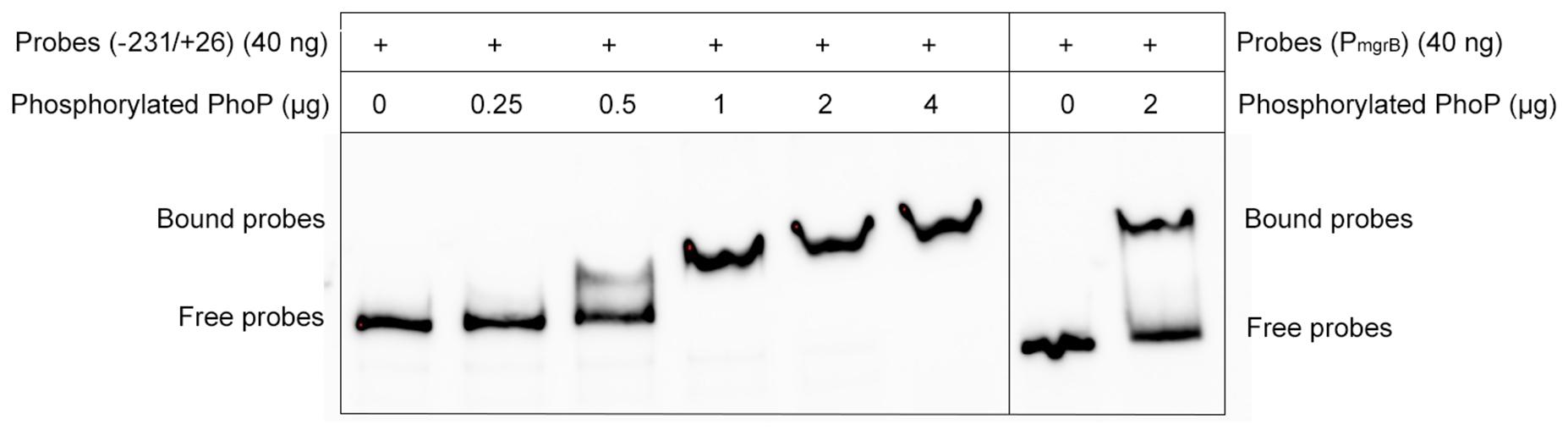
Figure 2. Electrophoretic mobility shift assays (EMSAs) of PhoP to the folA promoter region. A varying concentration of the PhoP (0, 0.25, 0.5, 1, 2, and 4 μg) was incubated with PCR-amplified biotin-labeled probes of the promoter regions of folA, and the binding of PhoP (2 μg) to the promoter regions of mgrB was used as the positive control, showing that PhoP recognized and bound to the promoter of folA. The experiment was repeated at least three times and a representative result was presented.
MgrB and PhoP Regulate folA Gene Expression in vivo
To confirm that MgrB and PhoP regulated the expression of folA, the β-galactosidase activities of strains (the wild type, the ΔphoP mutant, the ΔmgrB mutant, and the ΔmgrB ΔphoP mutant) harboring a reporter plasmid pZT102 (Li et al., 2014) (Supplementary Figure 1) carrying a lacZ gene fusion with the folA promoter region (−231/+26) were determined during the exponential growth phase. Our results showed that deletion of mgrB caused a ∼2-fold increase in the β-galactosidase activity, and deletion of phoP led to about 40% decrease of the β-galactosidase activity (Figure 3). Further deletion of phoP gene in the ΔmgrB mutant decreased the β-galactosidase activity back to the level of the wild type strain, but not to that of the ΔphoP mutant.
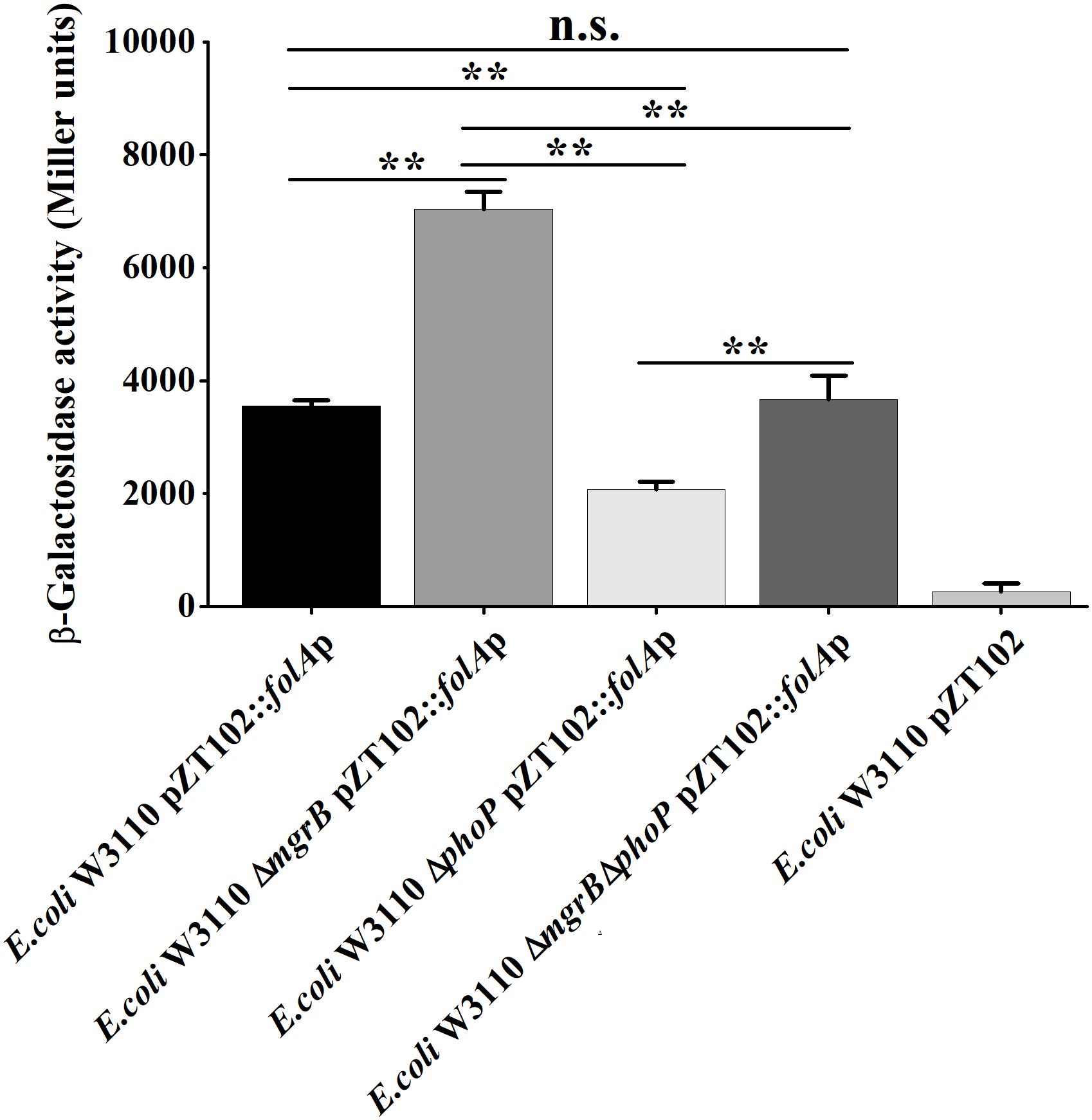
Figure 3. A comparison of promoter activity of folA in various strains of E. coli using lacZ-fusion analysis. β-Galactosidase activities were measured from exponential phase aerobic cultures in LB of several E. coli W3110 strains: wild-type (WT), null mutants (ΔphoP and ΔmgrB), and a double mutant (ΔmgrB ΔphoP), all of them carrying a reporter plasmid pZT102:folAp-257 containing the promoter region of folA (–231/+26). CK is empty pZT102 plasmid control in E. coli W3110. Means and standard deviations are represented. Data represent mean ± SD from three independent experiments. P values were calculated using t tests (*P < 0.05; **P < 0.01; ***P < 0.001).
Determination of the PhoP-Binding Sites in the Promoter of folA
To determine the PhoP-binding site in the folA promoter, DNase I footprinting assay was employed. The non-coding strand of the folA gene (−216 to −12) was labeled with FAMs and utilized as substrate for the assay. The mixture of phosphorylated PhoP and the substrate was co-incubated with DNase I, and then protected regions were analyzed. The results showed that the fluorescence signal faded out obviously from position −144 to the end of the strand, but not completely disappeared (Figure 4).
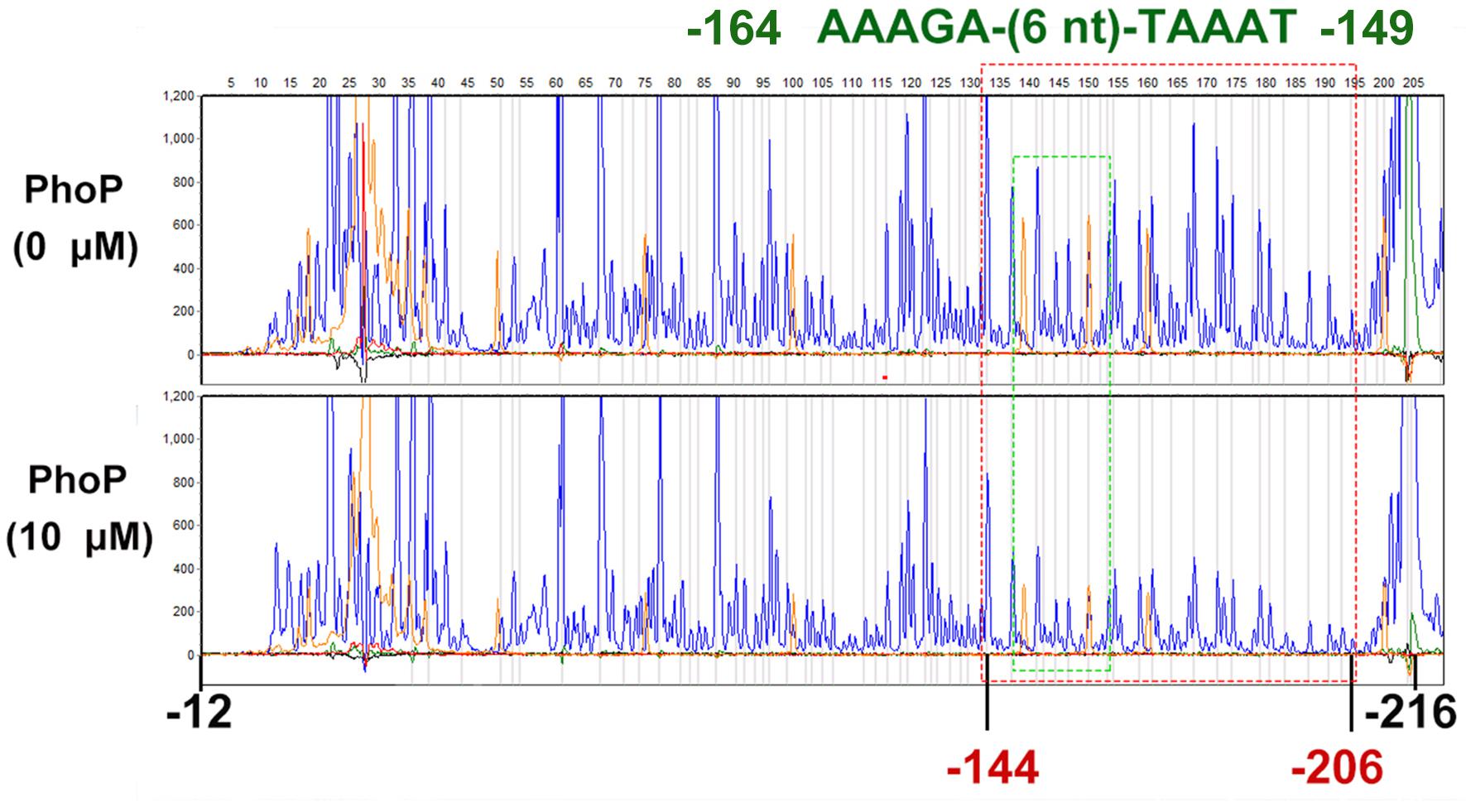
Figure 4. DNase I footprinting assays for mapping the binding sequence recognized by PhoP. FAM-labeled primer-based DNase footprinting experiments. Protection of the folA promoter DNA against DNase digestion by phosphorylated PhoP (10 μM) was evaluated. The sequences of the protected regions on the non-coding strand are framed in the figures. The fluorescence signal faded out obviously from position −144 to the end of the strand, but not completely disappeared (Dotted red box). Dotted green box contains a tandem repeat sequence AAAGA-(6 nt)-TAAAT (−164 to −149). The experiment was repeated at least three times and a representative result was presented.
Further EMSA assays were performed to identify the precise PhoP binding sites. Another two sets of gradually truncated folA promoter fragments in the direct and reverse orientations were prepared (Figure 5A). The results showed that more phosphorylated PhoP bound to the fragments containing segment 2 (−165 to −136) than those without segment 2, suggesting that segment 2 plays an important role on PhoP binding to the promoter region of folA (Figure 5B).
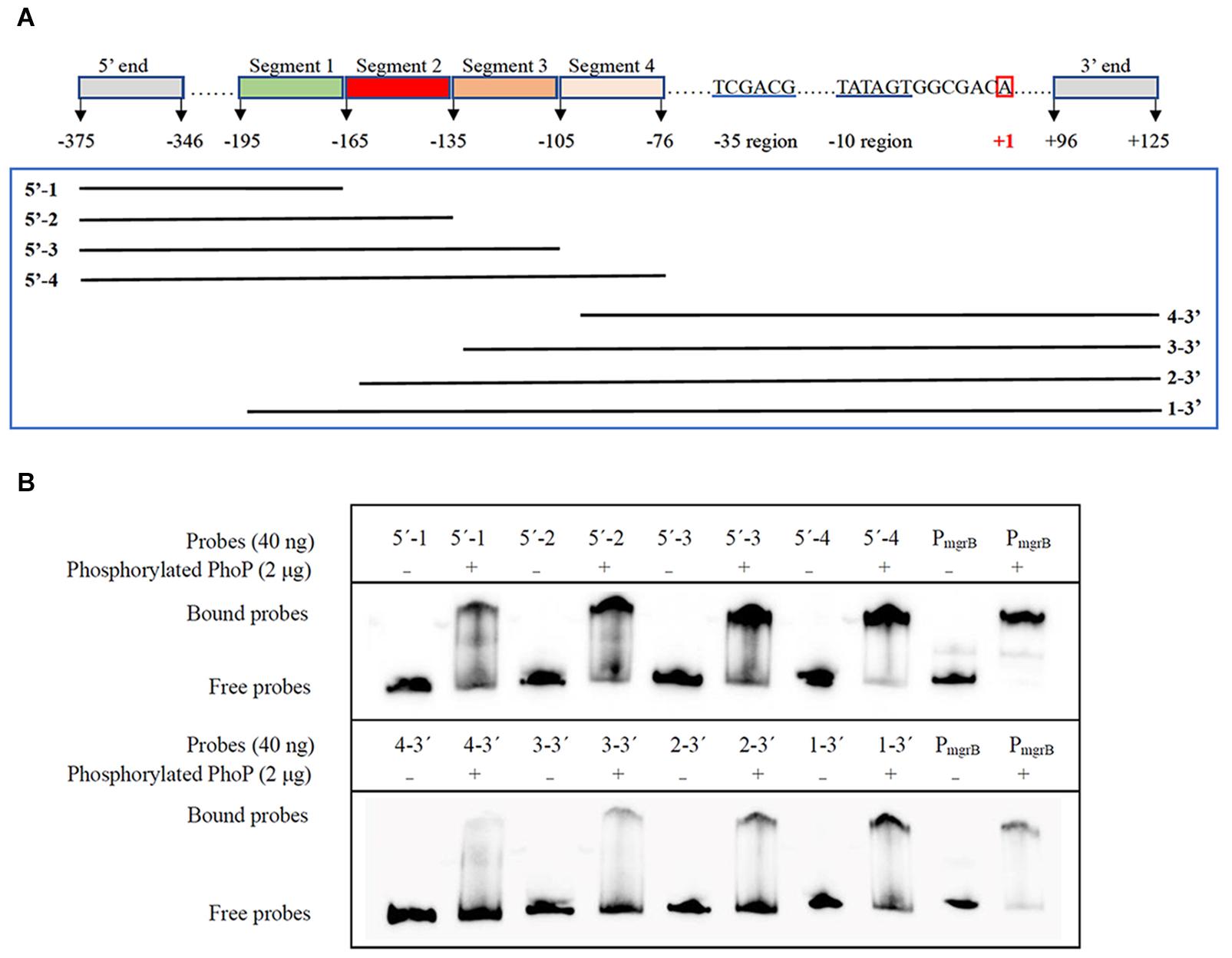
Figure 5. Determination of the PhoP-binding fragment in the promoter of folA using EMSA assay. (A) Nucleotide sequence of the folA promoter regulatory region. The numbering of the sequence is relative to the start site of transcription indicated with red box. The –35 and–10 regions of the folA promoter are underlined in blue. Segment 1 (–195/–166), segment 2 (–165/–136), segment 3 (–135/–106), and segment 4 (–105/–76) are indicated with highlights or underlines. The two sets of probes of gradually shortened folA promoter fragments are framed in green. (B) Binding of PhoP to the probes showed in (A), and the binding of PhoP to the promoter regions of mgrB was used as the positive control. The experiment was repeated at least three times and a representative result was presented.
To further determine the PhoP binding sites in segment 2, sequences alignment of the known “reverse PhoP boxes” (Supplementary Figure 2) was performed (Zwir et al., 2005, 2012). The results of the footprinting experiment also showed that fluorescence signals attenuated more significantly from position −165 to −155 (Supplementary Table 3). Thus, a tandem repeat sequence AAAGA-(6 nt)-TAAAT (−164 to −149) was deduced to be a putative “reverse PhoP box” in the promotor region of folA.
Furthermore, mutational analysis was carried out to test the above deduced “reverse PhoP box.” A deletion mutant (−166 to −147 deleted, abbreviated to “dm”) of the folA promotor were designed and ligated to pZT102 (Figure 6). The results of subsequent β-galactosidase reporter assay showed that, deletion of the predicted “reverse PhoP box” led to decreased transcriptional activity of the folA promotor (Figure 6). The transcriptional activity of the folA promotor with deletion of the predicted “reverse PhoP box” tested in W3110 was shown to be slightly but significantly higher to that of the intact folA promotor tested in the phoP deletion mutant. On the other hand, deletion of the predicted “reverse PhoP box” led to an about 30% decrease of the transcriptional activity of the folA promotor when tested in W3110, but no difference could be observed when tested in the phoP deletion mutant.
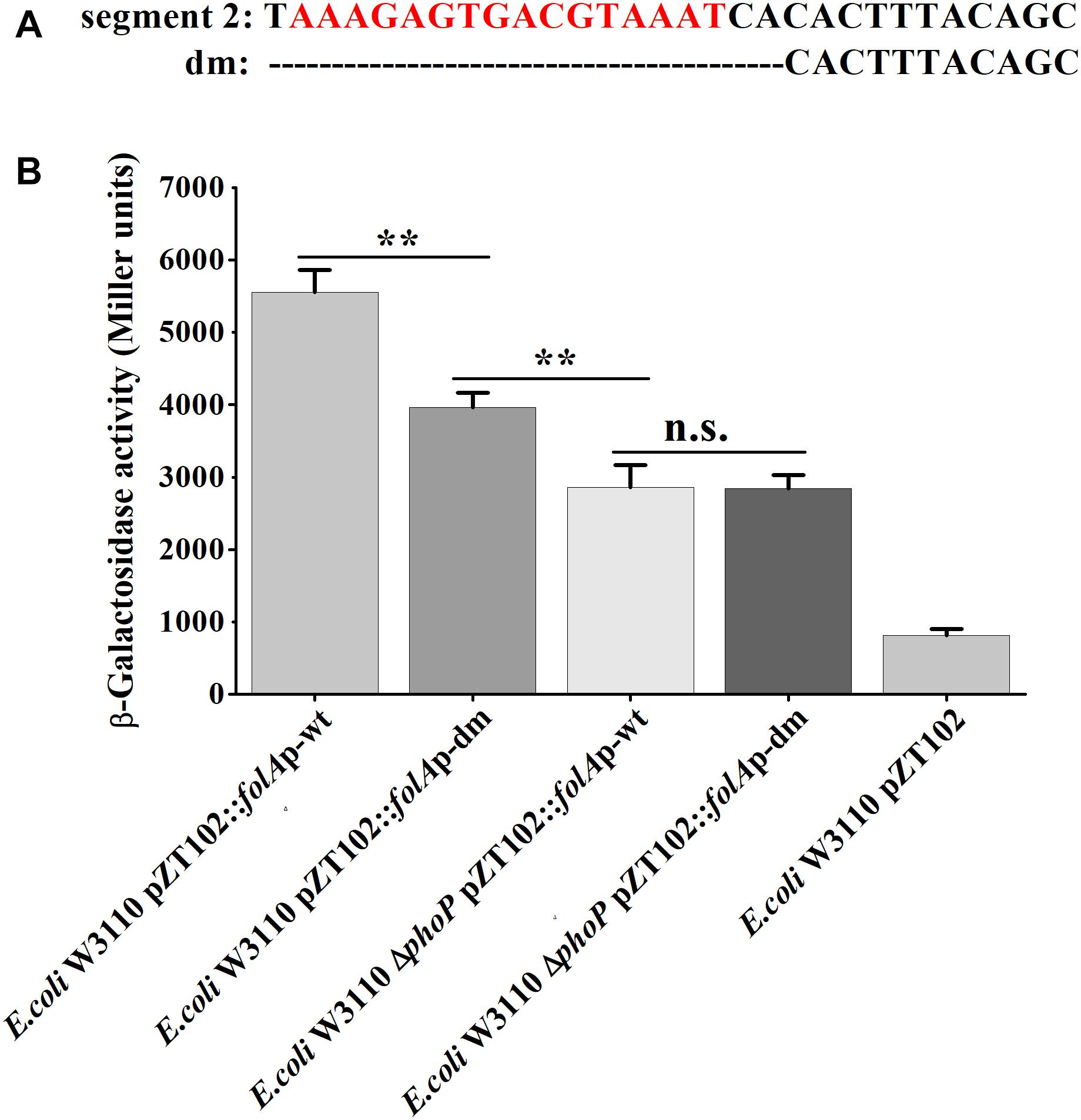
Figure 6. Determination of the PhoP-binding sites in the promoter of folA. (A) The deletion mutant was designed in segment 2 of the promotor region of folA, designated as dm. The predicted putative “reverse PhoP box” is indicated with red letters. (B) β-Galactosidase activities were measured from exponential phase aerated cultures in LB of the wild-type E. coli W3110 strain and ΔphoP mutant strain. They either carry the pZT102 reporter plasmids containing the promotor region of folA (–165/+26) or its mutants (dm). Data represent mean ± SD from three independent experiments. P values were calculated using t tests (*P < 0.05; **P < 0.01; ***P < 0.001).
Meanwhile, we also replaced the promoter region of folA in the chromosome of E. coli W3110 ΔmgrB with the promoter fragments having dm mutation, or the wild type promoter fragment, and measured TMP susceptibilities of the three recombinant strains. Our results showed that deletion of the predicted “reverse PhoP box” in the folA promotor led to slightly increased sensitivity to TMP (Table 5).
Discussion
Antifolates have been extensively used as anti-infective drugs for decades, but the mechanisms of antifolates resistance are not yet fully understood. In a recent screening of a library of transposon mutants, about 50 chromosomal loci were shown to be involved in the intrinsic antifolate resistance of Mycobacterium smegmatis (Guzzo et al., 2016). Here, we screened the E. coli Keio (single-gene, knockout mutants) library, and several new determinants of TMP resistance were also identified, including MgrB, a negative regulator of the PhoP/Q pathway.
As expected, deleting mgrB caused up-regulation of the PhoP/Q regulon, since MgrB is known to inhibit the enzymatic activity of PhoQ. Further deleting phoP or phoQ could partially reverse the TMP resistance phenotype of ΔmgrB mutant, and co-overexpression of phoP/Q caused TMP resistance, suggesting the involvement of the PhoP/Q system in mediating the TMP resistance phenotype of ΔmgrB mutant. However, the downstream effector needs to be identified. From the comparative transcriptome analysis, we found that deleting mgrB caused increased expression of folA, which was verified by subsequent RT-PCR and β-gal assays. Meanwhile, deleting phoP led to decreased expression of folA. The folA gene encodes the DHFR in E. coli, which is the target of TMP. Thus, over-expression of folA is the direct cause of TMP resistance in ΔmgrB. These data indicates the connection of the PhoP/Q system and folate metabolism in E. coli. To further probe the potential clinical relevance of this work, the mgrB gene was also deleted in O157, but that did not affect the susceptibility to TMP (Supplementary Table 4.). Subsequent RT-PCR analysis showed that, deleting mgrB did not affect the expression levels of phoP/Q (Supplementary Figure 3), suggesting the defect of feedback inhibition of PhoP/Q by MgrB in O157.
Though it is not clear why the PhoP/Q system is connected with folate metabolism in E. coli, clues could be found in previous works. Lippa and Goulian (2012) found that, except for low extracellular Mg2+, cationic antimicrobial peptides, and low pH, perturbation of the oxidizing environment of the periplasm can also stimulate the PhoP/Q system in E. coli, which is mediated by MgrB. Later, Cardenal-Munoz and Ramos-Morales (2013) showed that in Salmonella enterica, cellular redox status contributes to the regulation of genes regulated by the PhoQ/PhoP system. On the other hand, Waller et al. (2010) discovered that, tetrahydrofolate plays a role on the metabolism of iron-sulfur clusters in all domains of life and hence connects with oxidative stress response. So, the PhoP/Q system may possibly be linked with folate metabolism by sensing the cellular redox status of E. coli.
As mentioned above, though PhoP/Q was shown to be involved in modulating the transcription of folA by MgrB, further deleting phoP could only partially reverse the TMP resistance phenotype of ΔmgrB. This suggests that though MgrB can modulate the transcripton of folA through PhoP/Q, it can also do that through another way, which merits further investigation. Previously, we found that deleting mgrB caused acid resistance in E. coli, and further deleting of phoP could partially reverse the phenotype of ΔmgrB (Xu et al., 2019). Recently, we found that, MgrB can also affect acid stress response of E. coli through TyrR (will be published in another manuscript), a known transcriptional factor of folA (Yang et al., 2007). So it is possible that MgrB might also affect the transcription of folA through TyrR, which merits further investigation.
Next, we tried to see if PhoP could directly modulate the transcription of folA in E. coli. Though the data of EMSA experiments showed that phosphorylated PhoP could bind to the promotor region of folA in vitro, the former could only provide weak protection to the latter in the subsequent DNA footprinting experiments. Since it was not possible to locate the precise PhoP binding sites from the data of DNA footprinting analysis, we chose to combine our data with the data of sequences alignments of published “PhoP box” (Zwir et al., 2005, 2012; Harari et al., 2010), and then deduced a putative “reverse PhoP box” (AAAGA-(6 nt)-TAAAT, −164 to −149). However, deleting the deduced “reverse PhoP box” in the promotor region of folA could only slightly reverse the TMP resistance phenotype. It is difficult to determine whether this is really caused by disrupting the binding of PhoP to the promotor region of folA. From these data, we think it is less likely that PhoP can directly modulate the transcription of folA in E. coli though the possibility cannot be completely ruled out. More works are required to elucidate how PhoP modulates the transcription of folA.
Though folA has been shown to be a member of the TyrR regulon, the role of TyrR in folate metabolism remains unclear. Except for TyrR, no other transcriptional regulator of folA has ever been identified so far. Here, we show that PhoP can modulate the transcription of folA, though the fine mechanism remains to be uncovered.
Taken together, in this manuscript, we show that MgrB can modulate the transcription of folA in E. coli, thus affect the susceptibility to TMP, an important broad-spectrum antimicrobial drugs extensively used in clinic. Though MgrB can modulate the expression of folA through PhoP/Q, it may also achieve this through other pathway. From the data obtained, it is more likely that PhoP modulates the transcription of folA from an indirect way, which merits further investigation. Previously, MgrB has been shown to be involved in polymyxin resistance in K. pneumoniae. Here we show that MgrB is an important determinant of TMP resistance in E. coli. These results broaden our understanding of the mechanisms of the regulation of folate metabolism, as well as the mechanisms of TMP resistance in bacteria. Because E. coli is a widespread pathogenic bacterium, it is vital to further explore the role of mgrB mutations on TMP resistance in clinical isolates of pathogenic E. coli strains.
Materials and Methods
Bacterial Strains, Plasmids, and Culture Conditions
All plasmids and strains used in this study are listed in Supplementary Table 5. Strains of E. coli were cultured in Luria-Bertani (LB) medium (Difco) at 37°C. Plasmids pKD4, pKD46, and pCP20 were used for the construction of gene deletion mutants; plasmid pCA24N (Supplementary Figure 4) was used for gene expression and purification; and plasmid pZT102 was a reporter plasmid used for lacZ-fusion. The details for relevent strains and plasmids are listed in Supplementary Table 5. The gene-specific primers used for the construction of the recombinant plasmids are listed in Supplementary Table 6. Where appropriate, the E. coli culture medium was supplemented with 50 μg ml–1 kanamycin, 50 μg ml–1 chloramphenicol, and 100 μg ml–1 ampicillin.
Construction of Gene Deletion Mutants, Complemented Strains, and Gene Overexpression Strains
We constructed the E. coli W3110 gene knockout mutants with the λ Red recombination system and verified mutants with junction PCR as previously described (Datsenko and Wanner, 2000). Double-knockout mutants were generated with the same procedure. We amplified the genes mgrB, phoP, phoQ, phoPQ, pmrK, and tolC genes from E. coli W3110 genomic DNA using gene specific primers (see Supplementary Table 6), and then cloned the genes into plasmids pCA24N. The recombinant plasmids were then transduced into either the deletion mutants of E. coli W3110 (to form the complemented strain) or the E. coli wild types (to form the gene overexpression strain). Plasmid pCA24N:phoP was transduced into E. coli BL21(DE3) for the overexpression and purification of the protein PhoP. The details for relevent strains and plasmids are listed in Supplementary Table 5.
Screening for Genes Associated With TMP Resistance or Susceptibility
We cultured the E. coli Keio Knockout Collection (Baba et al., 2006) in 96 Deepwell Multiwell plates in Luria-Bertani (LB) medium (Difco) at 37°C until the OD600 was 0.6. We then diluted each well to 107 colony-forming units (cfu) ml–1 (about OD600 of 0.01) with fresh LB containing either 0.1 μg ml–1 or 1 μg ml–1 TMP. Plates were statically incubated overnight (12 h) at 37°C. We measured the absorbance of each well at 600 nm with a Microplate Reader (BioTek, Winooski, VT, United States) to monitor the growth of all mutants.
Drug Susceptibility Tests
Bacterial cells were grown to the mid-log phase (OD600 of ∼0.6), then diluted to 105 cfu ml–1 in fresh LB. Next, ten-fold serial dilutions were plated onto LB agar solid plates containing different concentrations of TMP (0, 0.01, 0.02, 0.04, 0.08, 0.16, 0.32, 0.64, 1.28, and 2.56 μg ml–1). 10 μM IPTG was contained when the complementation and/or over-expression strains were tested. Cultures were incubated overnight at 37°C. The MIC (minimum inhibitory concentration) was defined as the lowest concentration of the TMP required to inhibit 99% of the colony-forming bacterial units. Each strain tested two times with at least three different clones.
RNA-Seq
Total RNA was isolated with a RNeasy mini kit (Qiagen, Hilden, Germany). Library constructions were prepared using a TruSeq Stranded Total RNA Sample Preparation kit (Illumina, San Diego, CA, United States), and RNA was sequenced with an Illumina HiSeq 2500 at the Shanghai Biotechnology Corporation (Shanghai, China). The insert size of the purified libraries was confirmed with an Agilent 2100 bioanalyzer (Agilent Technologies, Santa Clara, CA, United States). Bowtie2 v2-2.0.5 was used to map the cleaned reads to the genome of E. coli str. K-12 substr. W3110 (downloaded from the National Center for Biotechnology Information)1. To estimate fold changes, HTSeq v2.1.1 was used with a reference annotation to generate values for fragments per kilobase of exon model per million mapped reads. Three biological replicates were used for RNA-seq, and p- and q-values were calculated. Differentially expressed genes were selected only if the false discovery rate q-value ≤ 0.05 and fold-change ≥ 2.
Quantitative Real-Time PCR Assays
Total RNA was extracted and cDNA was synthesized with a ReverTra Ace quantitative polymerase chain reaction (qPCR) kit (TOYOBO, Osaka, Japan), following the manufacturer’s instructions. We used real-time PCR (RT-PCR) to quantify gene expression levels using a 7900 HT Sequence Detection System with ABI Power SYBR Green PCR Master Mix (ABI, Hudson, NH, United States). Primers specific to phoQ, phoP, and tolC were designed and synthesized by Sangon Biotech Co., Ltd. (Shanghai, China). Expression levels of gapA mRNA were normalized as an endogenous control. All gene-specific primers are listed in Supplementary Table 6.
Protein Purification and Phosphorylation
Cultures of E. coli BL21 (DE3)/pCA24N:phoP were incubated at 37°C in LB broth medium supplied with corresponding antibiotics until the exponential phase. IPTG (isopropyl β-D-thiogalactoside) was added to produce a final concentration of 0.1 mM, and cultures were then incubated overnight with shaking at 16°C. Bacterial cells were harvested and then disrupted by sonication. The cell lysate was centrifuged at 10,000 × g for 30 min and the protein was isolated from the supernatant by nickel affinity chromatography. The purified His6-PhoP protein was dialyzed against 50 mM Tris–HCl buffer (pH 7.5). For EMSA assays, the His6-PhoP was phosphorylated as previously described (Tang et al., 2012) with modifications. Briefly, His6-PhoP was incubated in phosphorylation buffer (50 mM Tris–HCl [pH 7.5], 50 mM KCl, 10 mM MgCl2) containing 10 mM acetyl phosphate (Sigma-Aldrich) for 1 h at 37°C.
Electrophoretic Mobility Shift Assay (EMSA)
The biotin-labeled probes containing the promoter regions of the gene folA were amplified using biotin-labeled primers (Supplementary Table 6). EMSA/Gel-Shift Kit (Beyotime, Shanghai, China) was used for the binding of probes and phosphorylated PhoP proteins. We resolved band shifts on 6% non-denaturing TBE (Tris-Boric acid-EDTA)-polyacrylamide gels and subjected the gels to electrophoresis at 80 V for 1 h at 4°C. DNA was transferred to a nylon membrane at 380 mA for 70 min, and ultraviolet cross-linked at 302 nm for 15 min. We subsequently developed membranes using the Chemiluminescent Biotin-labeled Nucleic Acid Detection Kit (Beyotime, Shanghai, China), following the manufacturer’s protocol. We detected signals using a Typhoon 9410 imager (GE Healthcare, Maple Grove, MN, United States).
lacZ-Fusion Construction and β-Galactosidase Assays
The DNA fragments containing promoter regions (including original sequence and sequence with mutation sites) of the folA operon were amplified with BamHI- and HindIII-included primers (see Supplementary Table 6). Amplified fragments were ligated with pZT102 reporter plasmids containing the lacZ operon (obtained from Prof. Shiyun Chen, Wuhan Institute of Virology, Chinese Academy of Sciences, China) to generate a series of lacZ-fused plasmids (Supplementary Table 5). The plasmids were then electroporated into E. coli strains (E. coli W3110, E. coli W3110 ΔphoP, E. coli W3110 ΔmgrB, and E. coli W3110 ΔmgrB ΔphoP). We cultured the recombinant strains at 37°C overnight, then diluted the cultures to 1% with fresh LB broth, grew them again at 37°C, and finally harvested them at an OD600 of ∼0.7. We washed cell pellets with PBS and assayed β-galactosidase activity as described by Miller (1992).
DNase I Footprinting Assay
The promoter region of folA (−216 to −12) was amplified using FAM-labeled reverse primer (Supplementary Table 6). The purified DNA fragment (800 ng) was added to the reaction mixture (containing phosphorylated PhoP proteins) at 37°C for 30 min as described in EMSA. All of the mixtures were treated with DNase (1 unit, Fermentas China Co., Ltd., Shenzhen, China) at 37°C for 45 s. The results were analyzed with Applied Biosystems 37030XL DNA analyzer (manufactured by Tsingke Company, Wuhan, China).
Gene Replacement
The promoter region of folA in the chromosome of E. coli W3110 ΔmgrB was replaced with the wild type promoter fragment, the deletion mutated promoter fragment (−166 to −147 deleted) respectively with linked Kanamycin resistance gene as a selection marker. The gene replacement mutants were constructed with the λ Red recombination system as previously described (Datsenko and Wanner, 2000). The mutants were verified by junction PCR and subsequent sequencing using primers that anneal to the genomic region outside the recombination locus.
Data Availability Statement
The datasets presented in this study can be found in online repositories. The names of the repository/repositories and accession number(s) can be found below: The RNA sequencing reads was deposited in the Sequence Read Archive (SRA) repositories, and the accession number was PRJNA523715 [FEMS Microbiol Lett. 2019, 366(11):fnz123]. The primers used in qRT-PCR have been added into Supplementary Table 5.
Author Contributions
HS, TL, JX, and JY have made major contributions to acquisition and analysis of data. SY, X-EZ, and ST have made contributions to interpretation of data. JG and J-YD have made substantial contributions to (i) conception and design, (ii) acquisition, analysis, and interpretation of data, and (iii) writing of the manuscript. All authors contributed to the article and approved the submitted version.
Funding
This work was supported by the Strategic Priority Research Program of the Chinese Academy of Sciences (Grant No. XDB29020000).
Conflict of Interest
The authors declare that the research was conducted in the absence of any commercial or financial relationships that could be construed as a potential conflict of interest.
Publisher’s Note
All claims expressed in this article are solely those of the authors and do not necessarily represent those of their affiliated organizations, or those of the publisher, the editors and the reviewers. Any product that may be evaluated in this article, or claim that may be made by its manufacturer, is not guaranteed or endorsed by the publisher.
Acknowledgments
We thank LetPub (www.letpub.com) for its linguistic assistance during the preparation of this manuscript.
Supplementary Material
The Supplementary Material for this article can be found online at: https://www.frontiersin.org/articles/10.3389/fmicb.2021.682205/full#supplementary-material
Footnotes
References
Aires, C. A., Pereira, P. S., Asensi, M. D., and Carvalho-Assef, A. P. (2016). mgrB Mutations mediating polymyxin B resistance in Klebsiella pneumoniae isolates from rectal surveillance swabs in Brazil. Antimicrob. Agents Chemother. 60, 6969–6972. doi: 10.1128/AAC.01456-16
Akinkugbe, O. O., Lewis, E. A., Montefiore, D., and Okubadejo, O. A. (1968). Trimethoprim and sulphamethoxazole in typhoid. Br. Med. J. 3, 721–722. doi: 10.1136/bmj.3.5620.721
Amyes, S., and Smith, J. (1974). R-factor trimethoprim resistance mechanism: an insusceptible target site. Biochem. Biophys. Res. Commun. 58, 412–418. doi: 10.1016/0006-291x(74)90380-5
Baba, T., Ara, T., Hasegawa, M., Takai, Y., Okumura, Y., Baba, M., et al. (2006). Construction of Escherichia coli K-12 in-frame, single-gene knockout mutants: the Keio collection. Mol. Syst. Biol. 2:2006.0008. doi: 10.1038/msb4100050
Bader, M. W., Sanowar, S., Daley, M. E., Schneider, A. R., Cho, U., Xu, W., et al. (2005). Recognition of antimicrobial peptides by a bacterial sensor kinase. Cell 122, 461–472. doi: 10.1016/j.cell.2005.05.030
Bermingham, A., and Derrick, J. P. (2002). The folic acid biosynthesis pathway in bacteria: evaluation of potential for antibacterial drug discovery. Bioessays 24, 637–648. doi: 10.1002/bies.10114
Bushby, S. R., and Hitchings, G. H. (1968). Trimethoprim, a sulphonamide potentiator. Br. J. Pharmacol. Chemother. 33, 72–90. doi: 10.1111/j.1476-5381.1968.tb00475.x
Bushby, S., and Barnett, M. (1967). “Trimethoprim-sulphonamides-in vitro sensitivity of 384 strains of bacteria,” in Proceedings of the 5th Int Congr Chemother. Abstracta, Part. 1, (Wien: Verlag der Wiener Medizinischen Akademie).
Canessa, A., Del Bono, V., De Leo, P., Piersantelli, N., and Terragna, A. (1992). Cotrimoxazole therapy of Toxoplasma gondii encephalitis in AIDS patients. Eur. J. Clin. Microbiol. Infect. Dis. 11, 125–130. doi: 10.1007/BF01967063
Cannatelli, A., D’andrea, M. M., Giani, T., Di Pilato, V., Arena, F., Ambretti, S., et al. (2013). In vivo emergence of colistin resistance in Klebsiella pneumoniae producing KPC-type carbapenemases mediated by insertional inactivation of the PhoQ/PhoP mgrB regulator. Antimicrob. Agents Chemother. 57, 5521–5526. doi: 10.1128/AAC.01480-13
Cannatelli, A., Di Pilato, V., Giani, T., Arena, F., Ambretti, S., Gaibani, P., et al. (2014). In vivo evolution to colistin resistance by PmrB sensor kinase mutation in KPC-producing Klebsiella pneumoniae is associated with low-dosage colistin treatment. Antimicrob. Agents Chemother. 58, 4399–4403. doi: 10.1128/AAC.02555-14
Cardenal-Munoz, E., and Ramos-Morales, F. (2013). DsbA and MgrB regulate steA expression through the two-component system PhoQ/PhoP in Salmonella enterica. J. Bacteriol. 195, 2368–2378. doi: 10.1128/JB.00110-13
Carr, A., Tindall, B., Brew, B. J., Marriott, D. J., Harkness, J. L., Penny, R., et al. (1992). Low-dose trimethoprim-sulfamethoxazole prophylaxis for toxoplasmic encephalitis in patients with AIDS. Ann. Int. Med. 117, 106–111. doi: 10.7326/0003-4819-117-2-106
Chatterjee, I., Kriegeskorte, A., Fischer, A., Deiwick, S., Theimann, N., Proctor, R. A., et al. (2008). In vivo mutations of thymidylate synthase (encoded by thyA) are responsible for thymidine dependency in clinical small-colony variants of Staphylococcus aureus. J. Bacteriol. 190, 834–842. doi: 10.1128/JB.00912-07
Cheng, H. Y., Chen, Y. F., and Peng, H. L. (2010). Molecular characterization of the PhoPQ-PmrD-PmrAB mediated pathway regulating polymyxin B resistance in Klebsiella pneumoniae CG43. J. Biomed. Sci. 17:60. doi: 10.1186/1423-0127-17-60
Cho, U. S., Bader, M. W., Amaya, M. F., Daley, M. E., Klevit, R. E., Miller, S. I., et al. (2006). Metal bridges between the PhoQ sensor domain and the membrane regulate transmembrane signaling. J. Mol. Biol. 356, 1193–1206. doi: 10.1016/j.jmb.2005.12.032
Csonka, G. W., and Knight, G. J. (1967). Therapeutic trial of trimethoprim as a potentiator of sulphonamides in gonorrhoea. Br. J. Vener Dis. 43, 161–165. doi: 10.1136/sti.43.3.161
Datsenko, K. A., and Wanner, B. L. (2000). One-step inactivation of chromosomal genes in Escherichia coli K-12 using PCR products. Proc. Natl. Acad. Sci. U.S.A. 97, 6640–6645. doi: 10.1073/pnas.120163297
DeHovitz, J. A., Pape, J. W., Boncy, M., and Johnson, W. D. Jr. (1986). Clinical manifestations and therapy of Isospora belli infection in patients with the acquired immunodeficiency syndrome. N. Engl. J. Med. 315, 87–90. doi: 10.1056/NEJM198607103150203
Du, D., Wang, Z., James, N. R., Voss, J. E., Klimont, E., Ohene-Agyei, T., et al. (2014). Structure of the AcrAB-TolC multidrug efflux pump. Nature 509, 512–515. doi: 10.1038/nature13205
Eguchi, Y., Ishii, E., Hata, K., and Utsumi, R. (2011). Regulation of acid resistance by connectors of two-component signal transduction systems in Escherichia coli. J. Bacteriol. 193, 1222–1228. doi: 10.1128/JB.01124-10
Eguchi, Y., Oshima, T., Mori, H., Aono, R., Yamamoto, K., Ishihama, A., et al. (2003). Transcriptional regulation of drug efflux genes by EvgAS, a two-component system in Escherichia coli. Microbiology 149, 2819–2828. doi: 10.1099/mic.0.26460-0
Estrada, A., Wright, D. L., and Anderson, A. C. (2016). Antibacterial antifolates: from development through resistance to the next generation. Cold Spring Harb. Perspect. Med. 6:a028324. doi: 10.1101/cshperspect.a028324
Fleming, M., Datta, N., and Grüneberg, R. (1972). Trimethoprim resistance determined by R factors. Br. Med. J. 1:26.
Groisman, E. A. (2001). The pleiotropic two-component regulatory system PhoP-PhoQ. J. Bacteriol. 183, 1835–1842. doi: 10.1128/JB.183.6.1835-1842.2001
Gunn, J. S., and Miller, S. I. (1996). PhoP-PhoQ activates transcription of pmrAB, encoding a two-component regulatory system involved in Salmonella typhimurium antimicrobial peptide resistance. J. Bacteriol. 178, 6857–6864. doi: 10.1128/jb.178.23.6857-6864.1996
Gunn, J. S., Lim, K. B., Krueger, J., Kim, K., Guo, L., Hackett, M., et al. (1998). PmrA-PmrB-regulated genes necessary for 4-aminoarabinose lipid A modification and polymyxin resistance. Mol. Microbiol. 27, 1171–1182. doi: 10.1046/j.1365-2958.1998.00757.x
Gupta, K., Hooton, T. M., Naber, K. G., Wullt, B., Colgan, R., Miller, L. G., et al. (2011). International clinical practice guidelines for the treatment of acute uncomplicated cystitis and pyelonephritis in women: a 2010 update by the infectious diseases society of America and the European society for microbiology and infectious diseases. Clin. Infect. Dis. 52, e103–e120. doi: 10.1093/cid/ciq257
Guzzo, M. B., Nguyen, H. T., Pham, T. H., Wyszczelska-Rokiel, M., Jakubowski, H., Wolff, K. A., et al. (2016). Methylfolate trap promotes bacterial thymineless death by sulfa drugs. PLoS Pathog. 12:e1005949. doi: 10.1371/journal.ppat.1005949
Harari, O., Park, S. Y., Huang, H., Groisman, E. A., and Zwir, I. (2010). Defining the plasticity of transcription factor binding sites by deconstructing DNA consensus sequences: the PhoP-binding sites among gamma/enterobacteria. PLoS Comput. Biol. 6:e1000862. doi: 10.1371/journal.pcbi.1000862
Hasse, B., Walker, A. S., Fehr, J., Furrer, H., Hoffmann, M., Battegay, M., et al. (2014). Co-trimoxazole prophylaxis is associated with reduced risk of incident tuberculosis in participants in the swiss HIV cohort study. Antimicrob. Agents Chemother. 58, 2363–2368. doi: 10.1128/AAC.01868-13
Helander, I. M., Kato, Y., Kilpelainen, I., Kostiainen, R., Lindner, B., Nummila, K., et al. (1996). Characterization of lipopolysaccharides of polymyxin-resistant and polymyxin-sensitive Klebsiella pneumoniae O3. Eur. J. Biochem. 237, 272–278. doi: 10.1111/j.1432-1033.1996.0272n.x
Hicks, K. G., Delbecq, S. P., Sancho-Vaello, E., Blanc, M. P., Dove, K. K., Prost, L. R., et al. (2015). Acidic pH and divalent cation sensing by PhoQ are dispensable for systemic salmonellae virulence. Elife 4:e06792. doi: 10.7554/eLife.06792
Hitchings, G. H. (1973). Mechanism of action of trimethoprim-sulfamethoxazole—I. J. Infect. Dis. 128, S433–S436.
Hughes, D. (1969). Single-blind comparative trial of trimethoprim-sulphamethoxazole and ampicillin in the treatment of exacerbations of chronic bronchitis. Br. Med. J. 4, 470–473. doi: 10.1136/bmj.4.5681.470
Hughes, W. T., Mcnabb, P. C., Makres, T. D., and Feldman, S. (1974). Efficacy of trimethoprim and sulfamethoxazole in the prevention and treatment of Pneumocystis carinii pneumonitis. Antimicrob. Agents Chemother. 5, 289–293. doi: 10.1128/aac.5.3.289
Huovinen, P., Sundstrom, L., Swedberg, G., and Skold, O. (1995). Trimethoprim and sulfonamide resistance. Antimicrob. Agents Chemother. 39, 279–289. doi: 10.1128/aac.39.2.279
Igo, M. M., Ninfa, A. J., Stock, J. B., and Silhavy, T. J. (1989). Phosphorylation and dephosphorylation of a bacterial transcriptional activator by a transmembrane receptor. Genes Dev. 3, 1725–1734. doi: 10.1101/gad.3.11.1725
Kato, A., Tanabe, H., and Utsumi, R. (1999). Molecular characterization of the PhoP-PhoQ two-component system in Escherichia coli K-12: identification of extracellular Mg2+-responsive promoters. J. Bacteriol. 181, 5516–5520. doi: 10.1128/jb.181.17.5516-5520.1999
Li, Y., Gao, H., Qin, L., Li, B., Han, Y., Guo, Z., et al. (2008). Identification and characterization of PhoP regulon members in Yersinia pestis biovar Microtus. BMC Genomics 9:143. doi: 10.1186/1471-2164-9-143
Li, Y., Li, L., Huang, L., Francis, M. S., Hu, Y., and Chen, S. (2014). Yersinia Ysc-Yop type III secretion feedback inhibition is relieved through YscV-dependent recognition and secretion of LcrQ. Mol. Microbiol. 91, 494–507. doi: 10.1111/mmi.12474
Lichtenstein, C., and Brenner, S. (1981). Site-specific properties of Tn7 transposition into the E. coli chromosome. Mol. Gen. Genet. 183, 380–387. doi: 10.1007/BF00270644
Lippa, A. M., and Goulian, M. (2009). Feedback inhibition in the PhoQ/PhoP signaling system by a membrane peptide. PLoS Genet. 5:e1000788. doi: 10.1371/journal.pgen.1000788
Lippa, A. M., and Goulian, M. (2012). Perturbation of the oxidizing environment of the periplasm stimulates the PhoQ/PhoP system in Escherichia coli. J. Bacteriol. 194, 1457–1463. doi: 10.1128/JB.06055-11
Ma, D., Cook, D. N., Alberti, M., Pon, N. G., Nikaido, H., and Hearst, J. E. (1995). Genes acrA and acrB encode a stress-induced efflux system of Escherichia coli. Mol. Microbiol. 16, 45–55. doi: 10.1111/j.1365-2958.1995.tb02390.x
Miller, J. H. (1992). RE: A Short Course in Bacterial Genetics: A Laboratory Manual and Handbook for Escherichia coli and Related Bacteria. Cold Spring Harbor, NY: Cold Spring Harbor Laboratory Press.
Minagawa, S., Ogasawara, H., Kato, A., Yamamoto, K., Eguchi, Y., Oshima, T., et al. (2003). Identification and molecular characterization of the Mg2+ stimulon of Escherichia coli. J. Bacteriol. 185, 3696–3702. doi: 10.1128/jb.185.13.3696-3702.2003
Mitrophanov, A. Y., Jewett, M. W., Hadley, T. J., and Groisman, E. A. (2008). Evolution and dynamics of regulatory architectures controlling polymyxin B resistance in enteric bacteria. PLoS Genet. 4:e1000233. doi: 10.1371/journal.pgen.1000233
Noall, E. W., Sewards, H. F., and Waterworth, P. M. (1962). Successful treatment of a case of proteus septicaemia. Br. Med J. 2, 1101–1102. doi: 10.1136/bmj.2.5312.1101
Palmer, A. C., and Kishony, R. (2014). Opposing effects of target overexpression reveal drug mechanisms. Nat. Commun. 5:4296. doi: 10.1038/ncomms5296
Plourde, P. J., D’costa, L. J., Agoki, E., Ombette, J., Ndinya-Achola, J. O., Slaney, L. A., et al. (1992). A randomized, double-blind study of the efficacy of fleroxacin versus trimethoprim-sulfamethoxazole in men with culture-proven chancroid. J. Infect. Dis. 165, 949–952. doi: 10.1093/infdis/165.5.949
Reeves, D. S. (1971). Sulphamethoxazole-trimethoprim: the first two years. J. Clin. Pathol. 24, 430–437. doi: 10.1136/jcp.24.5.430
Reeves, D., Faiers, M., Pursell, R., and Brumfitt, W. (1969). Trimethoprim-sulphamethoxazole: comparative study in urinary infection in hospital. Br. Med. J. 1, 541–544. doi: 10.1136/bmj.1.5643.541
Salazar, M. E., Podgornaia, A. I., and Laub, M. T. (2016). The small membrane protein MgrB regulates PhoQ bifunctionality to control PhoP target gene expression dynamics. Mol. Microbiol. 102, 430–445. doi: 10.1111/mmi.13471
Schneider, M. M., Hoepelman, A. I., Schattenkerk, J. K. M. E., Nielsen, T. L., Van Der Graaf, Y., Frissen, J. P., et al. (1992). A controlled trial of aerosolized pentamidine or trimethoprim–sulfamethoxazole as primary prophylaxis against Pneumocystis carinii pneumonia in patients with human immunodeficiency virus infection. N. Engl. J. Med. 327, 1836–1841.
Sheldon, R., and Brenner, S. (1976). Regulatory mutants of dihydrofolate reductase in Escherichia coli K12. Mol. Gen. Genet. 147, 91–97. doi: 10.1007/BF00337941
Sirotnak, F. M., and McCuen, R. W. (1973). Hyperproduction of dihydrofolate reductase in Diplococcus pneumoniae after mutation in the structural gene. evidence for an effect at the level of transcription. Genetics 74, 543–556. doi: 10.1093/genetics/74.4.543
Skold, O., and Widh, A. (1974). A new dihydrofolate reductase with low trimethoprim sensitivity induced by an R factor mediating high resistance to trimethoprim. J. Biol. Chem. 249, 4324–4325. doi: 10.1016/s0021-9258(19)42520-9
Smith, D. R., and Calvo, J. M. (1982). Nucleotide sequence of dihydrofolate reductase genes from trimethoprim-resistant mutants of Escherichia coli. Mol. Gen. Genet. 187, 72–78. doi: 10.1007/bf00384386
Tang, Y. T., Gao, R., Havranek, J. J., Groisman, E. A., Stock, A. M., and Marshall, G. R. (2012). Inhibition of bacterial virulence: drug-like molecules targeting the Salmonella enterica PhoP response regulator. Chem. Biol. Drug Des. 79, 1007–1017. doi: 10.1111/j.1747-0285.2012.01362.x
Then, R. L. (1993). History and future of antimicrobial diaminopyrimidines. J. Chemother. 5, 361–368. doi: 10.1080/1120009x.1993.11741082
Tikhonova, E. B., and Zgurskaya, H. I. (2004). AcrA, AcrB, and TolC of Escherichia coli form a stable intermembrane multidrug efflux complex. J. Biol. Chem. 279, 32116–32124. doi: 10.1074/jbc.M402230200
Waller, J. C., Alvarez, S., Naponelli, V., Lara-Nunez, A., Blaby, I. K., Da Silva, V., et al. (2010). A role for tetrahydrofolates in the metabolism of iron-sulfur clusters in all domains of life. Proc. Natl. Acad. Sci. U.S.A. 107, 10412–10417. doi: 10.1073/pnas.0911586107
Xu, J., Li, T., Gao, Y., Deng, J., and Gu, J. (2019). MgrB affects the acid stress response of Escherichia coli by modulating the expression of iraM. FEMS Microbiol. Lett. 366:fnz123. doi: 10.1093/femsle/fnz123
Yamamoto, K., Ogasawara, H., Fujita, N., Utsumi, R., and Ishihama, A. (2002). Novel mode of transcription regulation of divergently overlapping promoters by PhoP, the regulator of two-component system sensing external magnesium availability. Mol. Microbiol. 45, 423–438. doi: 10.1046/j.1365-2958.2002.03017.x
Yang, J., Ogawa, Y., Camakaris, H., Shimada, T., Ishihama, A., and Pittard, A. J. (2007). folA, a new member of the TyrR regulon in Escherichia coli K-12. J. Bacteriol. 189, 6080–6084. doi: 10.1128/JB.00482-07
Yeo, W. S., Zwir, I., Huang, H. V., Shin, D., Kato, A., and Groisman, E. A. (2012). Intrinsic negative feedback governs activation surge in two-component regulatory systems. Mol. Cell 45, 409–421. doi: 10.1016/j.molcel.2011.12.027
Youle, M., Chanas, A., and Gazzard, B. (1990). Treatment of acquired immune deficiency syndrome (AIDS)-related pneumonitis with foscarnet: a double-blind placebo controlled study. J. Infect. 20, 41–50. doi: 10.1016/s0163-4453(90)92302-2
Zhao, G., Weatherspoon, N., Kong, W., Curtiss, R. III, and Shi, Y. (2008). A dual-signal regulatory circuit activates transcription of a set of divergent operons in Salmonella typhimurium. Proc. Natl. Acad. Sci. U.S.A. 105, 20924–20929. doi: 10.1073/pnas.0807071106
Zwir, I., Latifi, T., Perez, J. C., Huang, H., and Groisman, E. A. (2012). The promoter architectural landscape of the Salmonella PhoP regulon. Mol. Microbiol. 84, 463–485. doi: 10.1111/j.1365-2958.2012.08036.x
Keywords: Escherichia coli, MgrB, PhoP/Q, folA, gene expression regulation, trimethoprim resistance
Citation: Shi H, Li T, Xu J, Yu J, Yang S, Zhang X-E, Tao S, Gu J and Deng J-Y (2021) MgrB Inactivation Confers Trimethoprim Resistance in Escherichia coli. Front. Microbiol. 12:682205. doi: 10.3389/fmicb.2021.682205
Received: 18 March 2021; Accepted: 30 June 2021;
Published: 28 July 2021.
Edited by:
Prescilla Emy Nagao, Rio de Janeiro State University, BrazilReviewed by:
Kwan Soo Ko, Sungkyunkwan University School of Medicine, South KoreaSrujana Yadavalli, Rutgers, The State University of New Jersey, United States
Copyright © 2021 Shi, Li, Xu, Yu, Yang, Zhang, Tao, Gu and Deng. This is an open-access article distributed under the terms of the Creative Commons Attribution License (CC BY). The use, distribution or reproduction in other forums is permitted, provided the original author(s) and the copyright owner(s) are credited and that the original publication in this journal is cited, in accordance with accepted academic practice. No use, distribution or reproduction is permitted which does not comply with these terms.
*Correspondence: Jing Gu, Z3VqQHdoLmlvdi5jbg==; Jiao-Yu Deng, ZGVuZ2p5QHdoLmlvdi5jbg==
†These authors have contributed equally to this work and share first authorship