- 1Department for Microbial Physiology and Molecular Biology, Institute of Microbiology, University of Greifswald, Greifswald, Germany
- 2Department for Microbial Proteomics, Institute of Microbiology, University of Greifswald, Greifswald, Germany
- 3Braunschweig Integrated Centre of Systems Biology (BRICS), Institute of Microbiology, Technische Universität Braunschweig, Braunschweig, Germany
The anaerobic pathogen Clostridioides difficile is perfectly equipped to survive and persist inside the mammalian intestine. When facing unfavorable conditions C. difficile is able to form highly resistant endospores. Likewise, biofilms are currently discussed as form of persistence. Here a comprehensive proteomics approach was applied to investigate the molecular processes of C. difficile strain 630Δerm underlying biofilm formation. The comparison of the proteome from two different forms of biofilm-like growth, namely aggregate biofilms and colonies on agar plates, revealed major differences in the formation of cell surface proteins, as well as enzymes of its energy and stress metabolism. For instance, while the obtained data suggest that aggregate biofilm cells express both flagella, type IV pili and enzymes required for biosynthesis of cell-surface polysaccharides, the S-layer protein SlpA and most cell wall proteins (CWPs) encoded adjacent to SlpA were detected in significantly lower amounts in aggregate biofilm cells than in colony biofilms. Moreover, the obtained data suggested that aggregate biofilm cells are rather actively growing cells while colony biofilm cells most likely severely suffer from a lack of reductive equivalents what requires induction of the Wood-Ljungdahl pathway and C. difficile’s V-type ATPase to maintain cell homeostasis. In agreement with this, aggregate biofilm cells, in contrast to colony biofilm cells, neither induced toxin nor spore production. Finally, the data revealed that the sigma factor SigL/RpoN and its dependent regulators are noticeably induced in aggregate biofilms suggesting an important role of SigL/RpoN in aggregate biofilm formation.
Introduction
In recent years, the anaerobic gastrointestinal pathogen Clostridioides difficile has established itself as one of the major causative agents of pseudomembranous colitis and toxic megacolon (Wiegand et al., 2012; Nasiri et al., 2018; Martínez-Meléndez et al., 2020; Doll et al., 2021). C. difficile does not only produce enterotoxins that damage the gastrointestinal epithelium (Fletcher et al., 2021) but also forms easily transmittable spores that significantly contribute to C. difficile’s efficient spreading in the environment, hospitals and elderly homes (McLure et al., 2019; Hernandez et al., 2020; Werner et al., 2020; Khader et al., 2021). If conditions are favorable, e.g., after antibiotic-induced dysbiosis when the reduced microbiome fails to convert primary bile acids into secondary bile acids, C. difficile spores are able to germinate to successively colonize the large intestine (Theriot et al., 2014; Pike and Theriot, 2020). Even though antibiosis mostly stops the acute infection, C. difficile spores as well as some vegetative cells survive in the intestine and can subsequently cause a relapse as soon as antibiotic concentrations are sufficiently low (Goulding et al., 2009; Chilton et al., 2018; Castro-Córdova et al., 2020; Feuerstadt et al., 2021; Normington et al., 2021). Initially, sporulation was assumed to be one of the major prerequisites for C. difficile’s persistence under clinical circumstances. However, recent publications failed to correlate sporulation efficiency of a certain strain with the corresponding virulence and persistence potential, i.e., highly infectious strains do not necessarily produce more spores than other strains (Sirard et al., 2011; Oka et al., 2012; Plaza-Garrido et al., 2015; Gómez et al., 2017). In conclusion, persistence does obviously not solely rely on sporulation but also on additional features of C. difficile (Smits, 2013). In this context, biofilm formation was proposed to be a major additional factor (Dawson et al., 2012; Ðapa et al., 2013). Surface-associated biofilms, consisting of multiple microorganisms embedded in a slimy extracellular matrix, represent a form of community lifestyle for many bacteria (Hansen et al., 2007; Oliveira et al., 2015; Ren et al., 2015). Many nosocomial infections caused by Staphylococcus aureus, Streptococci and numerous other pathogens rely on biofilms (Jamal et al., 2018). In this context, biofilm formation has been frequently linked to pro-longed infection and persistence (Burmølle et al., 2010; Jamal et al., 2018). An important feature of biofilms is their extracellular matrix, which mostly consists of extracellular DNA (eDNA), lipids, proteins and polysaccharides. The extracellular matrix provides protection against chemical and mechanical stress and confers resistance to therapeutics and the host immune system (Karygianni et al., 2020). Anaerobic bacteria, including C. difficile have also been found to produce biofilms in vitro, and biofilm-like structures have been observed on the intestinal mucosal surface of C. difficile infected mice (Donelli et al., 2012; Lawley et al., 2012; Soavelomandroso et al., 2017).
Initial biofilm studies using C. difficile revealed mechanisms of formation and involved components comparable to those determined for other bacteria (Dawson et al., 2012; Vuotto et al., 2018). For instance, the involvement of adhesion-mediating cell surface structures, such as flagella, pili and various adhesion molecules, has been reported (Reynolds et al., 2011; Ðapa et al., 2013; Pantaléon et al., 2015). Similarly, c-di-GMP signaling, quorum sensing, and regulators, such as Spo0A and CodY, are involved in coordination of the process of biofilm formation (Ðapa et al., 2013; Purcell et al., 2016; McKee et al., 2018b; Dubois et al., 2019). Knockout mutants of some genes encoding certain proteins commonly linked to biofilm formation, such as adhesion and cell signaling proteins, were found impaired in biofilm formation in vitro and revealed a reduced infection and persistence behavior in rodent models (Barketi-Klai et al., 2011; Dawson et al., 2012; Ðapa et al., 2013; Batah et al., 2017; McKee et al., 2018a; Slater et al., 2019). Corresponding cell-cell aggregates were observed in a CDI murine model and multispecies biofilms have been shown to be a reservoir for C. difficile spores in triple-stage chemostat human gut model (Lawley et al., 2012; Soavelomandroso et al., 2017; Normington et al., 2021). While these data clearly indicate that biofilm formation is indeed an important virulence factor our knowledge on the physiology of C. difficile biofilms is still scarce. Moreover, it remains to be determined whether in vitro biofilm model systems sufficiently resemble in vivo biofilm formation. Currently, in vitro C. difficile biofilms are often studied in plastic microtiter plates, where cells initially attach to the surface, followed by a maturation of the biofilm and a final detachment of cell-cell-aggregates (Dawson et al., 2012; Ðapa et al., 2013; Dubois et al., 2019). Similar biofilms have been observed in continuous-flow micro-fermenters (Poquet et al., 2018). Alternatively, colony biofilms on agar plates and related biofilm models have been used (Crowther et al., 2014; Semenyuk et al., 2014; James et al., 2018). Inconsistent results from in vitro experiments suggest that biofilm physiology strongly depends on the chosen strain, growth conditions and phase as well as the experimental setup (Ðapa et al., 2013; Maldarelli et al., 2016; Mathur et al., 2016; Pantaléon et al., 2018). For example, differences in flagella production and contribution to biofilm formation has been reported for different strains and phases of biofilm formation (Ðapa et al., 2013; Maldarelli et al., 2016). Analogously, a comprehensive RNA-seq approach revealed that the expression levels of some genes which had previously been linked to biofilm formation were significantly different between C. difficile colony biofilms grown on agar plates and biofilms formed on glass beads (Maldarelli et al., 2016).
Considering the strong evidence that C. difficile colonizes the gut in a biofilm-like manner a profound molecular characterization of C. difficile biofilms is crucial, e.g., for the targeted development of therapeutics and vaccines. Since most vaccines are directed against cell-surface exposed structures, the comprehensive characterization of C. difficile’s cell-surface proteins and polysaccharides in biofilms is of particular importance (Bruxelle et al., 2017; Kirk et al., 2017a; Péchiné et al., 2018; Bradshaw et al., 2019). Here, we applied a comparative proteomics approach to investigate the proteome repertoire of C. difficile strain 630Δerm grown either as aggregate or colony biofilm to (i) identify proteins contributing to biofilm formation of one or the other growth condition and (ii) elucidate the underlying regulatory networks.
Materials and Methods
Bacterial Strains and Media
The reference strain C. difficile 630Δerm (DSM28645) (Hussain et al., 2005; van Eijk et al., 2015) was grown at 37°C in an anaerobic workstation (Toepffer Lab Systems, Germany) in Brain Heart Infusion (BHIS; Oxoid (Thermo Fisher Scientific), Waltham, MA) supplemented with L-cysteine (0.1%(wt/vol), Sigma-Aldrich, St. Louis, MO), and yeast extract (5 mg/ml; Carl Roth, Germany) as described earlier (Ðapa et al., 2013). Prior to cultivation, spores were allowed to germinate for 72 h in BHIS medium. Subsequently, the germinated cells were used to inoculate pre-cultures which were grown for 18 h in BHIS medium. For planktonic growth main cultures were inoculated to an optical density of 0.05 at 600 nm.
Growth of Colony and Aggregate Biofilms
The growth of colony biofilms was performed as previously described with some modifications (Semenyuk et al., 2014). Briefly, BHI medium was supplemented with yeast extract and agarose (1.5% v/v) and autoclaved for 15 min. Subsequently, sterile-filtered cysteine in BHI medium was added to the medium, mixed and transferred to petri dishes. Plates were allowed to dry for 24 h in the anaerobic chamber. Prior to cultivation, a filter membrane with a pore size of 0.45 μm (cellulose ester, Sigma-Aldrich, St. Louis, MO) was placed on top of the BHIS agar plate. Per plate 100 μl of C. difficile-containing BHIS medium were placed on top of the filter membrane. The BHIS medium was inoculated by an exponentially grown C. difficile main culture (OD600nm: ∼0.5) in a 100-fold dilution. Plates were wrapped with parafilm in order to protect them from drying. The colony biofilms were grown for three and six days at 37°C in the anaerobic environment.
Aggregated biofilms were cultivated as previously reported with some modifications (Dawson et al., 2012). Briefly, sterile 6-well plates (polystyrene, Corning, NY) were filled with 2 ml C. difficile-containing BHIS medium. The BHIS medium was inoculated by an exponentially grown C. difficile main culture (OD600nm: ∼0.5) in a 100-fold dilution. The 6-well plates were placed in a plastic bag to avoid evaporation of the medium. Cells were grown for three and six days at 37°C in the anaerobic environment.
Cell Harvest and Protein Extraction
Planktonically-grown cells were harvested after 6 and 12 h of cultivation at 6.000 × g for 20 min and pellets were stored at −80°C. Filter membranes with colony biofilms were placed into 15 ml reaction tubes and stored at −80°C. In order to separate aggregated cells from free-living planktonic cells the culture-medium was carefully removed from the 6-well plates and pooled (one 6-well plate = one biological sample). The pooled culture-medium was filtered through a 10 μm filter (IsoporeTM PC Membrane, Merck Millipore, Tullagreen, Ireland), washed with 5 ml of 0.9% NaCl (w/v) and subsequently the filter was stored at −80°C. The filtrate containing the planktonic cells was centrifuged at 6.000 × g and the cell pellet was stored at −80°C.
The samples (cell pellets of filtrate samples and membrane filters of both biofilm types) were kept in 0.6–1 ml of an urea-containing buffer (7 M urea, 2 M thiourea, 50 mM dichlorodiphenyltrichloroethane (DDT), 4% (w/v) 3-[(3-cholamidopropyl) dimethylammonio]-1-propanesulfonate (CHAPS), 50 mM Tris-HCl). Cell lysis was performed by sonication in six cycles on ice as done previously (Sonoplus, Bandelin, Berlin, Germany, Otto et al., 2016; Berges et al., 2018). Cell debris was removed by centrifugation at 6,000 × g for 20 min at 4°C. 200 μl of the resulting lysates were precipitated by ice-cold acetone (in a 1:7 ratio v/v) for 20 h at −20°C. Subsequently, samples were allowed to warm up at RT and were centrifuged at 22,000 × g for 45 min at RT. The supernatant was discarded and the pellets were dried at RT. The protein pellets were solubilized in 100 μl of an SDS-containing urea-buffer (7 M urea, 2 M thiourea, 1% SDS v/v). In order to estimate the relative protein concentration, 10 μl of each sample was mixed with SDS-loading buffer and separated by SDS-PAGE (Criterion TGX Precast Gels 12%, Biorad, Hercules, CA, Laemmli, 1970). Protein gels were fixed for 1 h at RT (40% EtOH, 10% glacial acidic acid and 50% H2O), washed in H2O and stained by the Flamingo fluorescent dye (Biorad, Hercules, CA) for 1 h at RT. Remaining dye was removed by a washing step in H2O and fluorescence signals of the samples were obtained by Typhoon scanner (GE Healthcare, Little Chalfont, United Kingdom). The fluorescence signals of the gel image were quantified by ImageQuant (Biorad, Hercules, CA). These fluorescent signal intensities were used for normalization to load comparable protein amounts (30 μg of protein per sample) on the final SDS-Gel. For each sample, the entire gel lane was cut into 10 gel blocks and proteins were digested in-gel with trypsin as follows: the excised gel pieces were destained using 50% (v/v) methanol in 100 mM NH4HCO3. Subsequently, gel pieces were dehydrated using 100% ACN and allowed to dry. Modified trypsin (sequencing grade, Promega, Germany) was added to a final ratio of 1:10 (trypsin/sample) in 50 mM Tris/HCl, pH 7.5 and the sample incubated at 37°C overnight. Peptides were iteratively extracted from the gel by a four-step procedure, using ACN, 5% (v/v) formic acid in ddH2O and further two steps of ACN. Peptide-containing supernatants were pooled and completely dried using a Speedvac concentrator (Eppendorf AG). Samples were subsequently resolved in buffer A (5% (v/v) ACN, 0.1% (v/v) formic acid) and desalted using ZipTips (C18, Millipore). Desalted peptides were again vacuum-dried and stored at −20°C. Finally, the samples were solubilised in 10 μL 0,1% acetic acid solution and transferred into vials for MS-analysis.
Mass Spectrometric Measurement and Data Analysis
Samples were subjected to LC-MS/MS measurements using an EASYnLC 1,000 (Thermo Fisher Scientific, Odense, Denmark) with self-packed columns [(Luna 3 μC18(2) 100A, Phenomenex, Germany)] in a one-column setup on-line coupled to an Orbitrap Elite (Thermo Fisher Scientific, Bremen, Germany) setting parameters as previously described (Berges et al., 2018).
Database search and intensity based absolute quantification (iBAQ) was achieved using the MaxQuant proteomics software package (Cox and Mann, 2008; Tyanova et al., 2016a; version: 1.6.10.43). A protein sequence database of C. difficile strain 630Δerm containing 3781 entries was obtained from UniProt. Common laboratory contaminants and reverse sequences were added by the MaxQuant software. Parameters were set as follows: Trypsin cleavage with a maximum of two missed cleavages was assumed and oxidation of methionine was set as variable modification. Default parameters were used for protein identification. For label-free protein quantification unique and razor peptides were considered with a minimum ratio count of 2. Match between runs was enabled with default settings within each sample group. C difficile proteins were considered as identified if they were identified with at least two unique peptides in at least two out of three biological replicates. riBAQ values were calculated as published previously (Shin et al., 2013). Averaged riBAQs were used to calculate log2 fold changes. Proteins significantly altered in their abundance between two conditions were identified by two-way analysis of variance (ANOVA) followed by a Tukey post hoc test using the Perseus software package (Tyanova et al., 2016b; version: 1.6.2.2). Cellular localization of identified proteins was predicted by PSORTb (Yu et al., 2010; version: 3.0).
Voronoi-Regulon Treemaps
Global protein expression patterns were analyzed and visualized using Voronoi treemaps (Bernhardt et al., 2013) which were adapted to illustrate regulons of several well defined global regulators of C. difficile as described in the literature. For this purpose, data extracted from eight transcriptomic studies was used to generate a scaffold for the Voronoi-regulon-treemaps. In these previous studies, regulons of fourteen global regulators were characterized, i.e., SigB (Kint et al., 2017), SigH (Saujet et al., 2011), SigD (El Meouche et al., 2013), SigL/RpoN (Soutourina et al., 2020), CodY (Dineen et al., 2010), CcpA (Antunes et al., 2012), Fur (Ho and Ellermeier, 2015), Hfq (Boudry et al., 2014), c-di-GMP (McKee et al., 2018b), Spo0A (Pettit et al., 2014), SigF, SigE, SigG, and SigK (Fimlaid et al., 2013). The corresponding regulons, comprising negatively as well as positively regulated genes, differ in size in the range of 30 to more than 400 genes (Table 1). In total, these transcriptional regulators modulate the expression of 1252 different C. difficile genes. For the illustration of the defined regulons each regulated gene was assigned to its corresponding regulator(s). Additionally, the regulatory effect (positive or negative) of a regulator on the expression of a specific gene is indicated by a plus or minus symbol. Finally, C. difficile log2 fold changes of biofilm models compared to filtrate samples were mapped onto the regulon maps.
Results and Discussion
A Comparative Proteomics Approach to Investigate the Protein Repertoire of C. difficile Biofilms
In order to identify proteins required for and/or characteristic of C. difficile biofilm formation the protein inventory of biofilm- and planktonically-grown bacteria was comparatively analyzed. Two different types of biofilms were investigated: 1. colony biofilms on agar plates and 2. aggregate biofilms formed in 6-well plates. Although colonies on agar plates do not meet the traditional criteria of a biofilm, the densely packed cells likewise present a form of multicellular growth attached to a biotic surface (Gingichashvili et al., 2017; Kesel et al., 2017) and it cannot be excluded that the growth conditions that bacteria face during this form of multicellular living are relevant during infection. Colony biofilms were directly analyzed after scraping them off the plates. Aggregate biofilms from 6-well plates were harvested by filtration and cells that passed the filter were analyzed in parallel as non-biofilm fraction. Previous studies investigating C. difficile biofilm gene expression by RNA-seq used planktonically-grown cells as reference (Maldarelli et al., 2016; Poquet et al., 2018). Thus, planktonically-grown cells from exponential (6 h) and stationary (12 h) growth phase were also included in this study (Figure 1).
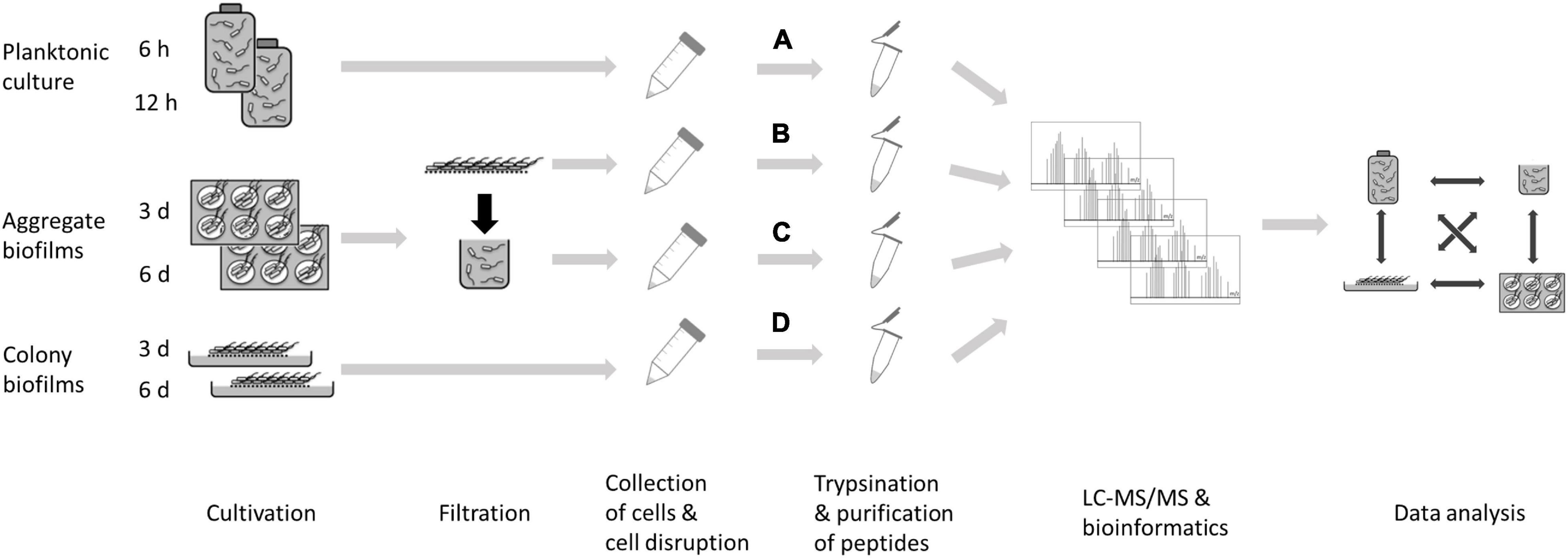
Figure 1. Experimental setup for a comparative proteomics approach to investigate biofilm formation in C. difficile. C. difficile strain 630Δerm was grown as planktonic culture for 6 and 12 h (A), or as aggregate biofilm in six-well plates for 3 or 6 days (B) or as colony biofilm on solid growth medium for 3 or 6 days (D). In addition, cells from the filtrate of aggregate biofilms were analyzed (C). Cells were harvested and their protein repertoire was subsequently analyzed using a LC-MS/MS approach.
Principal component analyses revealed that the various proteome parameters obtained for every tested condition clustered together within the biological replicates but revealed a clear cut separated distribution in dependence of the growth condition and time (Supplementary Figure 1). This was also true for the data obtained for cells from the filtrate (sample C of Supplementary Figure 1) of the aggregate biofilms which clearly separated from the corresponding biofilm cells (sample B of Supplementary Figure 1), although both types of cells experienced the same cultivation time and growth medium along with nutrient limitation and accumulated waste products. We propose physiological differences between these cells to be mainly attributed to cell aggregation/biofilm formation and therefore used the filtrate samples as the reference for non-biofilm conditions rather than the planktonic cells (sample A of Supplementary Figure 1) which were not further considered.
Global hierarchical cluster analyses on all proteins that were found differentially expressed between the six remaining data sets using ANOVA (analysis of variance) yielded four major clusters A–D (Figure 2 and Supplementary Table 2). Cluster A contained proteins with higher abundance in 6-day old filtrate cells and consisted of a high proportion of membrane proteins (54%) while all other clusters contained approximately 75% cytosolic proteins. In line with this, cluster A contained transporters for cations and amino acids. The largest cluster B contained 607 proteins of high abundance in aggregate biofilms which were assigned to the functional categories “cell wall biosynthesis”, “cell surface polysaccharide biosynthesis”, “flagella biosynthesis”, “regulation and cell signaling”, and “amino acid and carbohydrate metabolism”. Cluster C was composed of 99 proteins highly abundant in colony biofilms, and the 267 proteins of cluster D were found in higher abundance in colony biofilms and filtrate cells. Both clusters share a high proportion of cell-wall proteins including the S-layer protein SlpA and 12 of the 28 cell wall proteins (CWPs) encoded in one operon with SlpA. Additionally, “fermentation and energy generation” proteins of cluster C were assigned to the Wood-Ljungdahl pathway and the corresponding glycine cleavage system, and to subunits of the V-type ATPase, but also “sporulation”-related proteins such as CotA, CotB, and SipL were observed.
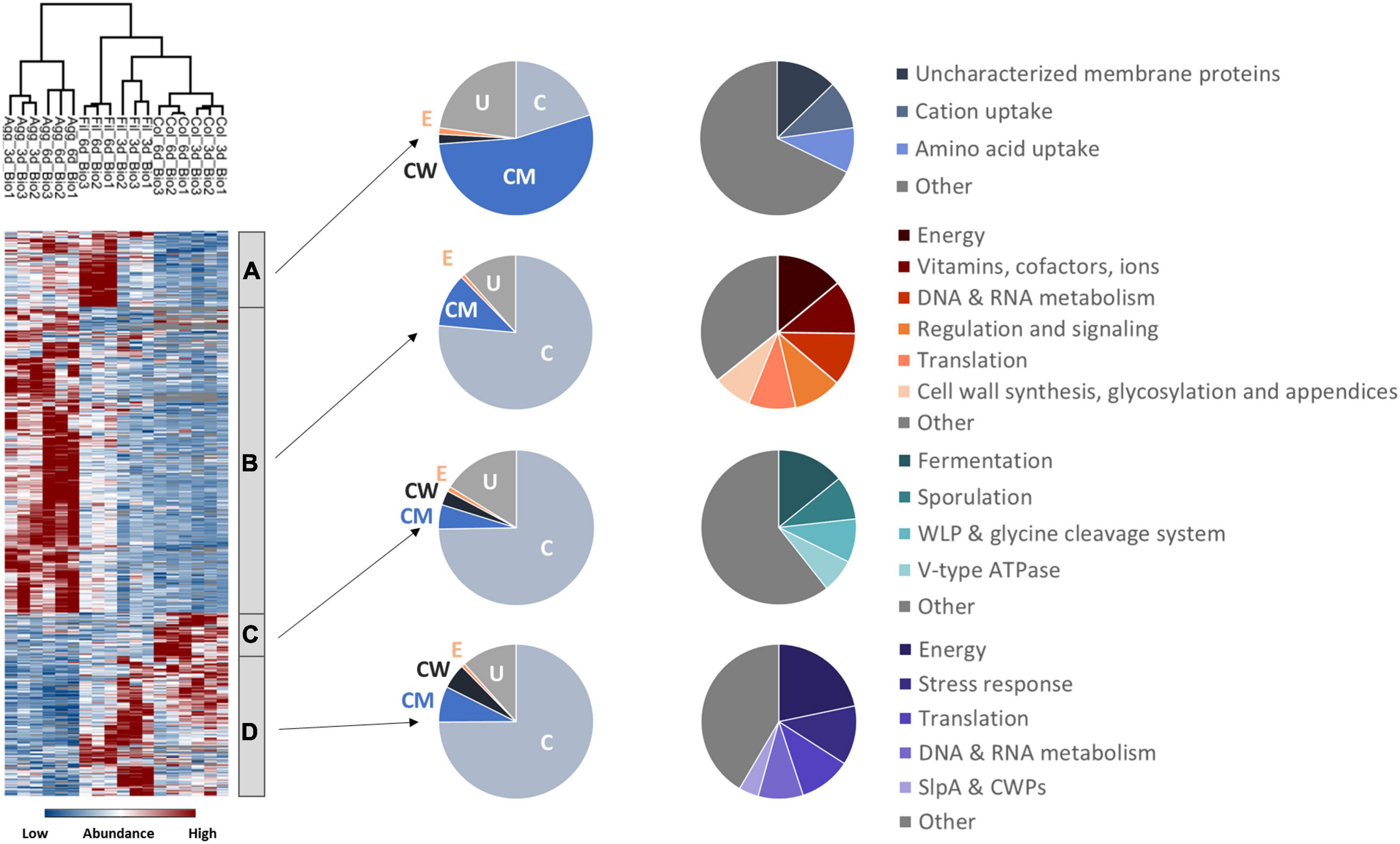
Figure 2. Hierarchical cluster analyses of C. difficile proteome data sets. The heatmap on the left side shows differentially abundant proteins between aggregate biofilms (Agg), filtrate cells (Fil), and colony biofilms (Col) from day three (3d) and day six (6d) for three biological replicates (Bio1-3). Z-transformed abundance values are displayed as a color gradient where blue colors indicate weakest abundance levels and red colors the strongest abundance levels among the growth conditions with maximum z-scores of +/–1.5. Hierarchical clustering revealed four major cluster (A–D) representing 6-day old filtrate cells cluster (A), aggregate biofilms (B), colony biofilms (C) or both, colony biofilms and filtrate samples (D). In the pie charts on the right the various clusters shown on left were further analyzed with regard to the subcellular location (middle: C for cytoplasmic; CM for cytoplasmic membrane; CW for cell wall; E for extracellular; U for unassigned). In addition, proteins within a cluster were grouped according to their function and the most prominent functional groups of each cluster are presented in the pie charts on the right (WLP for Wood-Ljungdahl pathway). A detailed list of all significantly differentially abundant proteins including fold changes can be found in Supplementary Table 2 (Supplementary Material). Agg: Aggregate biofilms; Col: Colony biofilms; Fil: Filtrate samples; 3d: Samples from day three; 6d: Samples from day six; C, cytoplasmic; CM, cytoplasmic membrane; CW, cell wall; E, extracellular; U, unassigned; WLP, Wood-Ljungdahl pathway; CWPs, cell wall proteins.
In agreement with transcriptome data comparing biofilm and non-biofilm cells (Maldarelli et al., 2016; Poquet et al., 2018), we identified genes encoding cell-surface exposed proteins, but also genes from the categories “energy metabolism”, “stress response”, “virulence” and “regulation and cell signaling” as biofilm signature.
Production of Cell Surface-Associated Proteins in C. difficile Biofilms
Adhesion to epithelial cells as well as cell-cell-aggregation is mainly mediated by cell-surface exposed proteins, polysaccharides and cell appendices such as pili and flagella (Ðapa et al., 2013; McKee et al., 2018a; Arato et al., 2019). Notably, proteins from the respective categories were found differently expressed between colony and aggregate biofilms (Figure 3 and Supplementary Table 3). Most flagella proteins (i.e., FliE, FliF, FliG, FliH, FliM, FlgE, FlgG, FhlA, FhlF) and type IV pili proteins (i.e., PilT, PilB2, and PilM2) were detected in higher concentrations in aggregate biofilms than in colony biofilms and filtrates. Similar behavior was observed for proteins involved in cell wall biogenesis like enzymes of the mur operon (i.e., MurB, MurE, MurG), enzymes involved in lipoteichoic acid synthesis (GtaB, GtaB1) and modification (DltA), enzymes of the Cell Wall Glycopolymer (CWG) locus (CD2783 to CD2769), and proteins of membrane-lipid biosynthesis encoded by the fab operon (i.e., FabD, FabH, FabK). In contrast, the S-layer protein SlpA and most CWPs (i.e., Cwp12, Cwp16, Cwp19, Cwp22, Cwp84) were of significantly lower abundance in aggregate biofilms.
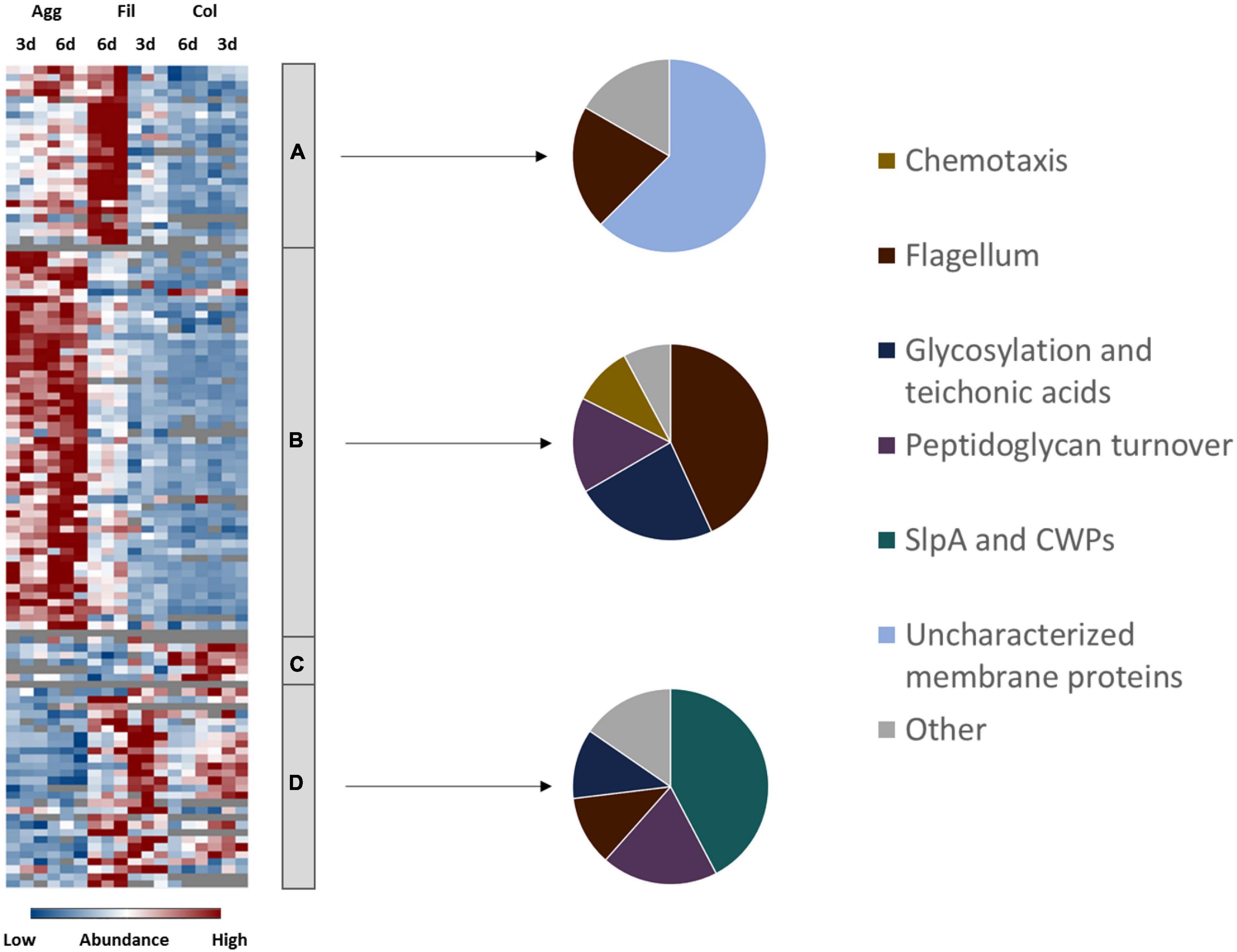
Figure 3. Differential abundance of cell surface-associated proteins. 108 surface-associated proteins were found differentially abundant (blue is down, red is up) between aggregate biofilms (Agg), filtrate samples (Fil) and colony (Col) from day three (3d) and day six (6d). Z-transformed abundance values are displayed as a color gradient where blue colors indicate weakest abundance levels and red colors the strongest abundance levels among the growth conditions with maximum z-scores of +/–1.5. The heatmap on the left site shows that they were found in all 4 clusters, representing the 6-day old filtrate cells cluster (A), aggregate biofilms (B), colony biofilms (C) or both, colony biofilms and filtrate samples (D). Pie charts on the right reveal that each individual cluster comprised mostly proteins from a specific functional group of cell surface-associated proteins. A list of the underlying proteins for this heatmap including their fold changes in abundance can be found in Supplementary Table 3. Agg, Aggregate biofilms; Col, Colony biofilms; Fil, Filtrate samples; 3d, Samples from day three; 6d, Samples from day six; CWPs, cell wall proteins.
Flagella and Type IV Pili
While comparable RNA-seq based expression profiles were observed for cell wall and S-layer biogenesis genes when aggregate biofilms and planktonic cells were compared (Poquet et al., 2018), transcription of flagella genes has been found to be reduced in aggregate and colony biofilms in comparison to planktonic cells of C. difficile (Maldarelli et al., 2016; Poquet et al., 2018). However, both transcriptomic studies were performed with biofilms grown in different setups and to different timepoints. Hence, flagella might be required for aggregate biofilm growth in our rather static set up, but might be obsolete or even obstructive for biofilm formation in continuous flow systems (Poquet et al., 2018) or for biofilms grown on glass beads (Maldarelli et al., 2016). As discussed above, somewhat contradictory observations have been obtained regarding the role of flagella during C. difficile biofilm formation (Ðapa et al., 2013; Jain et al., 2017) that may either reflect the complexity of the regulatory system underlying flagella expression in C. difficile (El Meouche et al., 2013; Soutourina et al., 2013; Anjuwon-Foster and Tamayo, 2017, 2018) and/or the different functions of flagella that depend on their posttranslational modification state (Twine et al., 2009; Faulds-Pain et al., 2014; Bouché et al., 2016; Valiente et al., 2016). Additionally, biofilms are often composed of subpopulations (Vlamakis et al., 2008; Besharova et al., 2016) and flagella in one or the other modification state might be relevant for one subpopulation and obstructive for the other. Alterations of flagella post-translational modifications were shown to impact motility and cell-cell-aggregation properties of C. difficile (Faulds-Pain et al., 2014; Valiente et al., 2016). Significant upregulation of C. difficile’s conserved glycosyltransferase CDIF630erm_00362 in conjunction with the flagella proteins in aggregate biofilms in our study might suggest that flagella in aggregate biofilms were glycosylated. The CDIF630erm_00362 gene is encoded directly downstream of fliC which is essential for flagella formation and the bacterium’s virulence in vivo (Valiente et al., 2016). Obviously, flagella are not always required for biofilm formation in C. difficile but might be beneficial depending on their state of modification in some cases.
In contrast, there is growing consensus that type IV pili are dispensable for early biofilm development, but required in mature biofilms of C. difficile (Maldarelli et al., 2016; Purcell et al., 2016; McKee et al., 2018a). We found type IV pili-associated proteins like PilT, PilB2 and PilM2 in aggregate biofilms and in lower amounts in filtrates but not in colony biofilms. Therefore, our data suggest that aggregate biofilms in contrast to colony biofilms obviously utilized glycosylated flagella and certain pili components during their formation.
Cell Surface Glyco-Polymers and Teichoic Acids
Proteins from the PSII locus for the synthesis of the teichoic-acid-like cell-surface polysaccharide II (e.g., CDIF630erm_03033, CDIF630erm_03035, CDIF630erm_03041), GtaB and GtaB1 providing UDP-glucose for teichoic acid synthesis and DltA involved in D-alanylation of teichoic acids were detected in aggregate biofilms and in lower amounts in the filtrates, and even lower or not in colony biofilms. Polysaccharide II is one of three of C. difficile’s cell surface polysaccharides (Ganeshapillai et al., 2008; Reid et al., 2012; Willing et al., 2015). The ubiquitously found polysaccharide, attached to the cell surface, represents either an important antigen or is masking surface antigens with higher inflammatory potential. Its presence in C. difficile biofilms was reported before (Ðapa et al., 2013; Chu et al., 2016). Likewise, lipoteichoic acids revealed a significant immunogenic potential. They have been found to fulfill numerous functions in antibiotic resistance, cell wall homeostasis, cell division and metabolism (McBride and Sonenshein, 2011; Schade and Weidenmaier, 2016).
The S-Layer
The S-layer covers the cell surface of C. difficile and consists of the main S-layer protein SlpA which is encoded in one operon with 28 CWPs. C. difficile’s S-layer protein SlpA and the 28 CWPs have been shown to be immunogenic (Péchiné et al., 2011; Bruxelle et al., 2016; Mizrahi et al., 2018). Deletion of the S-layer encoding genes renders a pathogenic C. difficile strain apathogenic (La Riva et al., 2011; Kirk et al., 2017b; Vaz et al., 2019). Furthermore, the S-layer, was found to play a role in cell-cell-aggregation and attachment to epithelial cells (Waligora et al., 2001; Merrigan et al., 2013; Bradshaw et al., 2017; Kirk et al., 2017b; Richards et al., 2018). Interestingly, the proteome data revealed that SlpA and 23 of its adjacent CWPs could be identified in colony biofilms and filtrate samples but were almost not detected in aggregate biofilms. This is in line with observations made by Janoir et al. (2013) who reported the downregulation of SlpA in the late stage of a mouse colonization model. The cysteine protease Cwp084, which was among the lower abundant CWPs, is responsible for the proteolytic processing of S-layer proteins (Kirby et al., 2009; Gooyit and Janda, 2016). Interestingly, a cwp84 mutant produces more biofilm mass compared to the wildtype (Pantaléon et al., 2015). Since the mutant was unable to cleave the SlpA precursor protein, a different more hydrophobic surface and different matrix proteome composition was observed, which might lead to an enhanced surface attachment of the cells, potentially explaining the increased biofilm production (La Riva et al., 2011; Pantaléon et al., 2015). Pantaléon et al. (2015) further detected most CWPs in the biofilm matrix (Cwp5, Cwp6, Cwp 9, Cwp14, Cwp21) and supernatant (CwpV, Cwp2, Cwp11, Cwp12, Cwp13, Cwp16, Cwp18, Cwp19, Cwp22, Cwp25, Cwp66) rather than in the surface proteome by comparative MALDI TOF analyses.
The Potential Role of PrkC Kinase During Biofilm Formation of C. difficile
Cwp7 was one of the few CWPs that revealed a differential expression pattern between the analyzed growth conditions and was significantly lower abundant in colony biofilms than in filtrates although not as low in aggregate biofilms as most other CWPs. Interestingly, a previous investigation showed that deletion of the membrane-associated serine/threonine kinase PrkC which is involved in cell wall homeostasis and antimicrobial resistance led to the downregulation of almost all CWPs but to an upregulation of Cwp7. Additionally, the ΔprkC mutant showed an increased release of polysaccharide II into the supernatant, was less motile and produced more biofilm (Cuenot et al., 2019). Although PrkC was found to be significantly higher abundant in the 3-day old aggregate biofilms and significantly lower abundant in 6-day old colony biofilms, the similar expression pattern of aggregate biofilms and the ΔprkC mutant suggest a possible role of PrkC in the regulation of biofilm formation in C. difficile.
In summary, aggregate biofilms analyzed here were characterized by higher abundance of potentially glycosylated flagella, type IV pili proteins, proteins required for cell surface polysaccharide synthesis and proteins involved in cell wall and membrane turnover compared to colony biofilms while SlpA and CWPs were lower abundant in aggregate biofilms compared to colony biofilms.
Energy Metabolism
The unique energy metabolism of C. difficile preferentially utilizes amino acids through a process called Stickland fermentation which firstly produces ATP via substrate level phosphorylation (Stickland, 1934; Neumann-Schaal et al., 2015). Secondly, various carbohydrates are the basis for a complex mixed acid fermentation (Riedel et al., 2017; Hofmann et al., 2021). Some of the involved processes are coupled via the membrane associated Rnf complex to the formation of an ion gradient which in turn drives ATP formation via a classical FOF1-ATPase (Müller et al., 2008). Alternative carbon sources like ethanolamine are used additionally (Nawrocki et al., 2018; Hofmann et al., 2021). When preferred amino acids and glucose are depleted from the medium, C. difficile is able to generate energy from lactate fermentation and to fix CO2 via the Wood-Ljungdahl pathway (Köpke et al., 2013; Hofmann et al., 2021).
Although all biofilm and filtrate cells were obviously subject to nutrient limitations due to extended incubation time, proteomic profiles for proteins involved in various pathways of energy generation indicated an aggregate- and colony biofilm specific energy metabolism (Figure 4 and Supplementary Table 4).
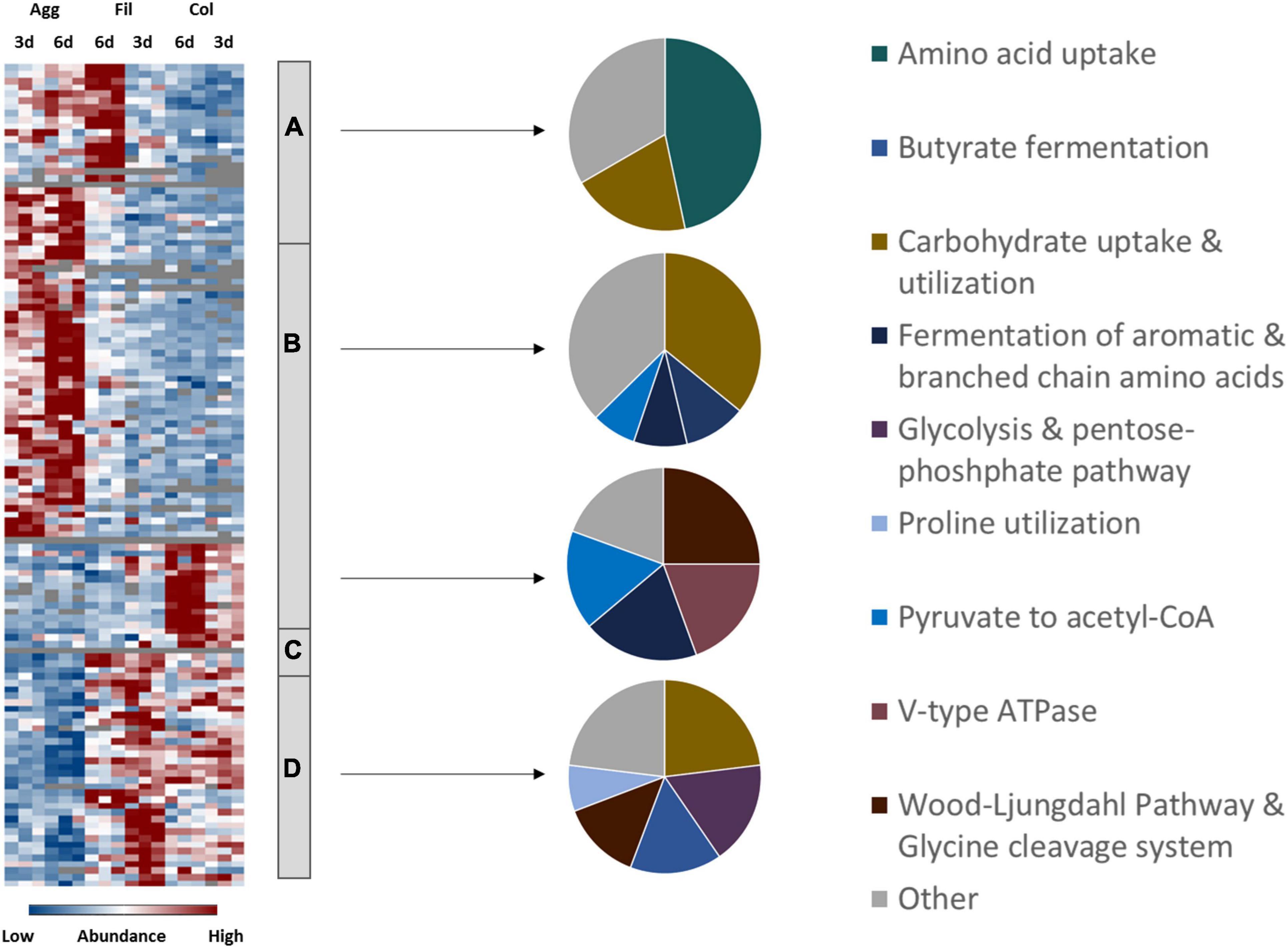
Figure 4. Differential abundance of energy metabolism-associated proteins. 185 metabolism-related proteins were found differentially abundant between aggregate biofilms (Agg), filtrate samples (Fil) and colony (Col) from day three (3d) and day six (6d). Z-transformed abundance values are displayed as a color gradient where blue colors indicate weakest abundance levels and red colors the strongest abundance levels among the growth conditions with maximum z-scores of +/–1.5. The heatmap on the left site shows that they were found in all 4 clusters, representing the 6-day old filtrate cells cluster (A), aggregate biofilms (B), colony biofilms (C) or both, colony biofilms and filtrate samples (D). Pie charts on the right reveal that each individual cluster comprised mostly proteins from a specific functional metabolic pathway. A list of proteins from this heatmap including their fold changes in abundance can be found in Supplementary Table 4. Agg, Aggregate biofilms; Col, Colony biofilms; Fil, Filtrate samples; 3d, Samples from day three; 6d, Samples from day six.
Amino Acid Utilization
Several systems of the oxidative and reductive branches of the Stickland amino acid fermentation were found differentially produced under the different biofilm conditions investigated in comparison to the filtrate samples. To start with, proteins from the CDIF630erm_00522-…-etfA1 operon (AcdB, EtfA1, EtfB1) for the reductive fermentation of leucine and phenylalanine to 3-phenylproprionate/isocoproate and the activator protein of the 2-hydroxyisocaproyl-CoA dehydratase, HadI, were higher abundant in aggregate biofilms compared to other culture conditions. The reductive fermentation of leucine and phenylalanine is indirectly coupled to the Rnf complex via ferredoxin (Kim et al., 2006; Schiffels and Selmer, 2019). In agreement, proteins from the Rnf complex (RnfB, RnfC, RnfD, RnfG) were higher abundant in filtrate and aggregate biofilms than in colony biofilms. In contrast, the proline reductase (PrdA, PrdB) for reductive degradation of proline to 5-aminovalerate, which is directly coupled to the Rnf complex (Jackson et al., 2006), showed the highest abundance levels in filtrate samples. Similarly, the subunits of the glycine reductase (GrdB, GrdC, GrdD) and associated proteins TrxA2 and TrxB3 for degradation of glycine to acetate were higher abundant in filtrate cells than in biofilm cells. Enzymes of the oxidative branch for fermentation of branched chain (VorA1, VorB1, VorC1, Ptb1) and aromatic amino acids (CDIF630erm_02622, IorA, IorB, Ptb1) were higher abundant in colony biofilms. To react to the reduced availability of nutrients, both filtrate samples and aggregate biofilms were found to have transporters for the uptake of amino acids such as those encoded by the app operon for oligo-peptide transport and MetNQ required for methionine uptake. In contrast, colony biofilms showed an overall lower abundance of any kind of transporters which might reflect the even more impaired diffusion of nutrients inside the dense biofilm matrix. It cannot be excluded that membrane protein extraction was less efficient for colony biofilms and reinforced this observation. However, overall only a very minor bias toward cytoplasmic and against membrane proteins was observed and significant changes in protein formation between the different analyzed conditions caused by regulatory processes were clearly visible.
Carbohydrate Utilization
C. difficile degrades carbohydrates via glycolysis and the pentose-phosphate pathway yielding pyruvate (Hofmann et al., 2018). Proteome analysis showed that enzymes of both pathways, such as PfkA, Pgi, Tpi, and Eno from the glycolysis and Tal, Tal1, RpiB1, RpiB2, Rpe1, and Tkt’ from the pentose-phosphate pathway, were higher abundant in filtrate samples. This is in contradiction to results of Poquet et al. (2018) who reported induction of glycolysis and pentose-phosphate pathway in aggregate biofilms compared to planktonically-grown cells. However, both data sets agree on active carbohydrate utilization within aggregate biofilms. As observed for amino acid uptake systems, both filtrate samples and aggregate biofilms revealed the presence of PTS systems for the uptake of carbohydrates. Moreover, aggregate biofilms revealed an extensive amount of enzymes for the utilization of carbohydrates which underlines the importance of carbohydrates for C. difficile under infection-relevant conditions (Theriot et al., 2014; Jenior et al., 2017, 2018; Fletcher et al., 2018). Also, Dubois et al. (2019) reported that induction of biofilm formation by deoxycholate was enhanced in the presence of fermentable carbohydrates.
Ethanolamine Utilization
An abundant nutrient source in the gut is the membrane lipid phosphatidylethanolamine. The derived amino alcohol ethanolamine can serve as carbon and nitrogen source. Availability of ethanolamine has been shown to reduce virulence in C. difficile and to delay the onset of CDI in a hamster model (Nawrocki et al., 2018). Interestingly, proteins involved in ethanolamine utilization were more or less exclusively identified in filtrate cells. However, e.g., in Entercoccus faecalis ethanolamine utilization is dependent on the presence of cobalamin (Del Papa and Perego, 2008). Assuming similar regulation in C. difficile, induction of cobalamin biosynthesis pathways in the aggregate biofilms (Supplementary Table 1) suggests that these biofilms were limited in cobalamin what might explain the inhibition of ethanolamine utilization gene expression under these growth conditions.
Utilization of the Intermediate Products Pyruvate and Acetyl-CoA
The intermediate product of the glycolysis as well as alanine oxidation, pyruvate, is further metabolized to either acetyl-CoA and formate via the pyruvate formate-lyase PflDE or to lactate by the lactate dehydrogenase Ldh (Dannheim et al., 2017; Hofmann et al., 2018). Acetyl-CoA is in turn degraded to acetate by Ptb1 and AckA or to butyrate via the butyrate fermentation pathway encoded in the bcd2-…-thlA and 4hbD-…-CDIFerm_02583 operons (Hofmann et al., 2018). Formate can be further metabolized to hydrogen by the formate hydrogenases FdhD and FhdF and the hydrogenases HydN1, HydN2, and HydA (Berges et al., 2018). According to our proteome data, several proteins for butyrate fermentation (ThlA1, Hbd, Crt2, EtfB3, Ptb, AbfD) and the hydrogenase HydN2 were found in higher amounts in colony biofilms and in lower amounts in aggregate biofilms. In contrast, the electron bifurcating lactate dehydrogenase encoded by CDIF630erm_01319-01321 was detected in higher amounts in aggregate biofilms. Pyruvate formate-lyase PflDE was found induced in biofilms compared to filtrate cells.
Expression of FOF1-Type and V-Type ATPase
Rarely observed among bacteria, C. difficile encodes for two ATPases. In addition to its FOF1-ATPase, which uses the membrane potential to generate ATP, C. difficile encodes a Na +–or H + -transporting V-type ATPase which uses ATP to maintain the ion gradient across the membrane and which has been linked to the Wood-Ljungdahl pathway and glycine cleavage system before (Saujet et al., 2011; Poquet et al., 2018). While the subunits of the FOF1-ATPase were overall lower abundant in both biofilm types compared to filtrate cells, the V-type ATPase subunits were significantly higher abundant in colony biofilms but less abundant in aggregate biofilms compared to filtrate cells. This suggests that the V-type ATPase is of importance for the colony biofilms.
Role of the Wood-Ljungdahl Pathway
In line with this, several proteins from the Wood-Ljungdahl pathway (WLP) were higher abundant in colony biofilms while repressed in aggregate biofilms in comparison to filtrate cells. With the exception of AcsABE that were found higher abundant in aggregate biofilms, almost all enzymes of the WLP, such as the carbon monoxide dehydrogenase (CooS, CDIFerm_00297, CDIFerm_00298), Fhs, FchA, FoldD, MetV and MetF, and the glycine cleavage system proteins (GcvTPA, GcvPB), which provide 5,10-methylene-tetrahydrofolate for the WLP, were significantly higher abundant in colony biofilms but lower abundant in aggregate biofilms compared to filtrate cells. The WLP, also known as the reductive Acetyl-CoA pathway, is able to generate acetyl-CoA from CO2 (Stupperich et al., 1983; Köpke et al., 2013). Moreover, the WLP has recently been suggested as an electron sink to maintain cell homeostasis in the absence of Stickland acceptors (Gencic and Grahame, 2020). Taken together, the higher levels of proteins from the oxidative branch of Stickland fermentation, of butyrate fermentation and of the V-type ATPase discussed above as well of WLP proteins in colony biofilms strongly support the hypothesis that the WLP plays an important role in maintaining cell growth in environments depleted in reductive equivalents and potentially maintains the membrane potential in concert with the coupled V-type ATPase (Gencic and Grahame, 2020). Of note, Poquet et al. (2018) reported a downregulation of WLP, glycine cleavage system and V-type ATPase genes in aggregate biofilms compared to planktonic cells which is in line with results of this proteome analysis.
In conclusion, higher production of proteins for less favorable energy pathways such as the Wood-Ljungdahl pathway and the lactate dehydrogenase and lower production of proteins for more favorable energy pathways and enzymes such as the proline and glycine reductase as well as glycolysis in biofilm cells compared to filtrate cells suggest that biofilms were more affected by the nutrient limitation than filtrate cells. However, both biofilms obviously responded differently. Presence of several PTS and enzymes for carbohydrate uptake and degradation and the induction of cofactor biosynthesis proteins in aggregate biofilms suggest that aggregate biofilms are (1) rather active cells that are able to invest ATP to take up nutrients from the environments and (2) permeable enough to allow nutrients to reach cells inside the biofilm. In contrast, the observed uniform induction of WLP, V-type ATPase, butyrate fermentation and oxidative branch of the Stickland fermentation in colony biofilms compared to aggregate biofilm and filtrate cells likely points at severe limitation of reductive equivalents in colony biofilms that rather supports survival than reproduction.
Stress Response and Virulence
In general, biofilm cells are embedded inside an extracellular matrix that protects cells from antibiotics and disinfectants but at the same time also impairs diffusion of nutrients and waste products (Anwar et al., 1992; Karygianni et al., 2020). Consequently, cells from the inner biofilm have to cope with nutrient limitation and accumulation of waste products. To survive such stressful conditions C. difficile’s genome encodes for various stress response systems as a first line of defense (Sebaihia et al., 2006). If conditions remain unfavorable, C. difficile initiates toxin synthesis or, as a last resort, sporulation (Onderdonk et al., 1979; Lawley et al., 2009; Underwood et al., 2009). Analysis of C. difficile’s stress reponse systems revealed that both biofilm types and filtrate cells revealed different expression of stress response and virulence-associated pathways similar to the results for the energy metabolism (Figure 5 and Supplementary Table 5).
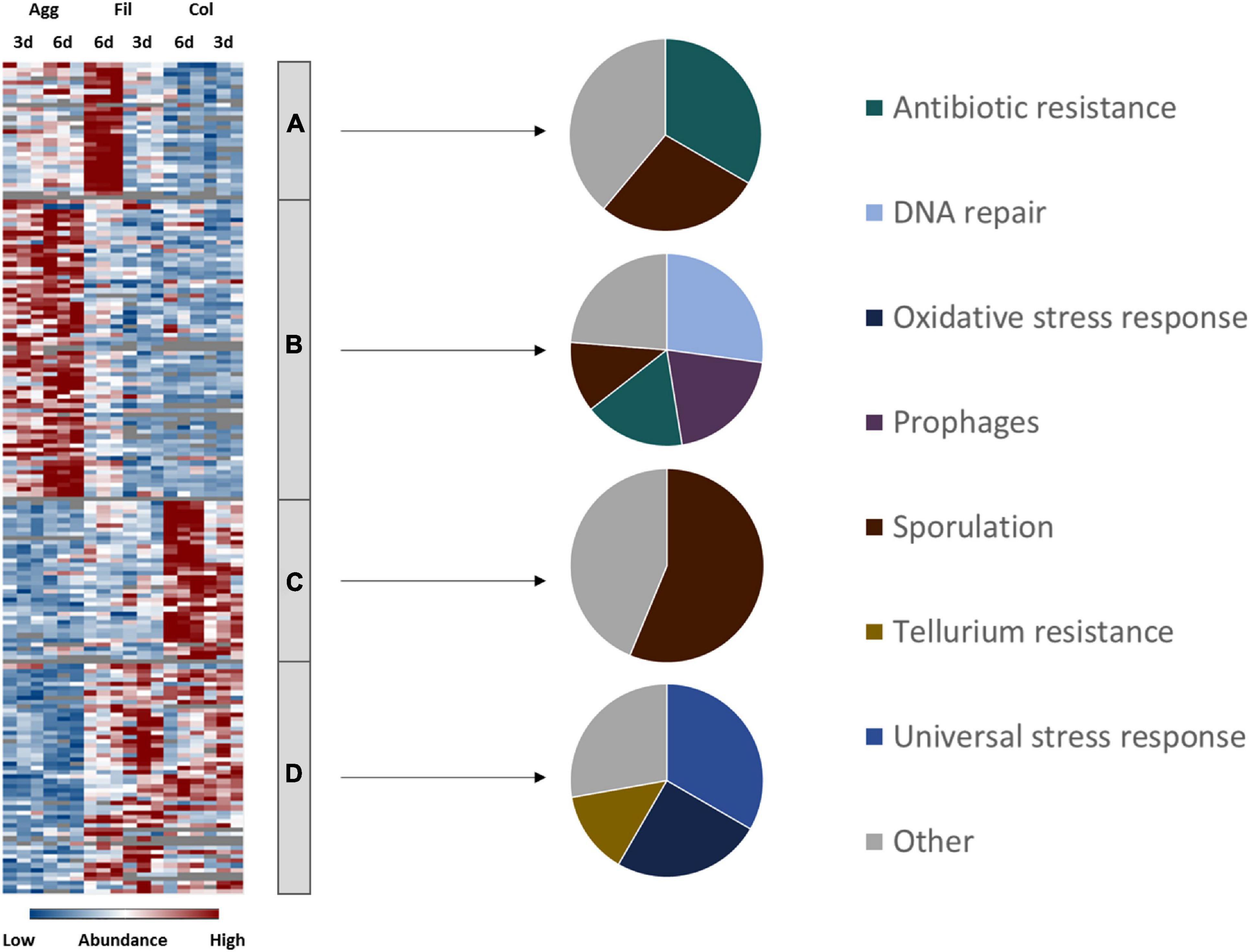
Figure 5. Differential abundance of virulence, antibiotic resistance and stress response-associated proteins. 129 proteins assigned to virulence, antibiotic resistance and stress-response related pathways were found differentially abundant (blue is down, red is up) between aggregate biofilms (Agg), filtrate samples (Fil) and colony (Col) from day three (3d) and day six (6d). Z-transformed abundance values are displayed as a color gradient where blue colors indicate weakest abundance levels and red colors the strongest abundance levels among the growth conditions with maximum z-scores of +/–1.5. The heatmap on the left site shows that they were found in all 4 clusters, representing the 6-day old filtrate cells cluster (A), aggregate biofilms (B), colony biofilms (C) or both, colony biofilms and filtrate samples (D). Pie charts on the right reveal that each individual cluster comprised proteins from specific stress response and resistance systems. A list of proteins from this heatmap including their fold changes in abundance can be found in Supplementary Table 5 (Supplementary Material). Agg, Aggregate biofilms; Col, Colony biofilms; Fil, Filtrate samples; 3d, Samples from day three; 6d, Samples from day six.
Stress Response
As a strictly anaerobic pathogen, C. difficile requires an effective oxidative stress response to be able to react to oxygen and reactive oxygen species (Neumann-Schaal et al., 2018). Interestingly, our proteome data set revealed that some of C. difficile’s oxidative stress response proteins, such as the rubrerythrin Rbr and the reverse rubrerythrins Rbr2 and Rbr3, were drastically lower abundant in aggregate biofilms compared to filtrate samples but slightly higher abundant in colony biofilms than in filtrate samples. Since PerR, a transcriptional regulator which represses oxidative stress response proteins, is inactive in strain Δerm due to a single nucleotide polymorphism in the perR gene (Troitzsch et al., 2021), these effects are possibly a result of post-transcriptional regulation. On the other hand, 6-day old colony biofilms revealed an induction of some oxidative stress response proteins such as NorR and SodA. Since no molecular oxygen was present in any of the tested conditions, the oxidative stress response proteins identified here were either expressed to react to other oxidative species such as reactive nitrogen species or other yet unknown signals not present in aggregate biofilms. Similarly, other stress response-associated proteins such as DnaK, GrpE, GroL, and ClpC, which were previously shown to be induced in response to heat stress, bile acids and antibiotics (Jain et al., 2011; Ternan et al., 2012; Chong et al., 2014; Sievers et al., 2019), were significantly lower abundant in aggregate biofilms compared to filtrate samples while the transcriptional regulators CtsR and HrcA, which repress the above mentioned proteins in other firmicutes species (Schulz and Schumann, 1996; Derré et al., 1999), were higher abundant in aggregate biofilms compared to the filtrate samples. In general, aggregate biofilms seemed to face less stress than colony biofilms and filtrate samples.
Antibiotic Resistance
In contrast, antibiotic resistance-associated proteins such as ClnA and ClnR involved in cationic antimicrobial peptide resistance (Woods et al., 2018), the tetracycline resistance protein TetM (Mullany et al., 1990) and putative multidrug ATP-type transport proteins such as CDIF630erm_00291, CDIF630erm_00940, and CDIF630erm_02245 revealed highest protein levels in aggregate biofilms, but were rarely detected in colony biofilms. Indeed, most studies addressing antibiotic resistance of C. difficile biofilms consistently showed that biofilms are more resistant to various antibiotics such as metronidazole (Semenyuk et al., 2014), vancomycin and linezolide (Tijerina-Rodríguez et al., 2019). In addition to impaired diffusion through the dense extracellular matrix and differential regulation of antibiotic resistance markers (Høiby et al., 2010) increased mutation rates as a result of accumulating metabolic waste products as well as increased horizontal gene transfer that is often observed in biofilms where cell densities are particularly high are assumed to boost antibiotic resistance of biofilms (Molin and Tolker-Nielsen, 2003; Boles and Singh, 2008; Levin and Cornejo, 2009; Ryder et al., 2012). Accordingly, levels of proteins required for homologous recombination and DNA repair (RecN, RuvB, RadA, UvrABC, MutLS, SbcCD, LexA) were significantly higher in aggregate biofilms than in filtrate samples. In line with this, it was shown before, that the induction of the SOS response (RecA, UvrABC) in a lexA deletion mutant resulted in an increased biofilm mass further demonstrating the importance of gene transfer and genetic evolution for efficient biofilm formation (Walter et al., 2015). Again, colony biofilms revealed even lower protein amounts of mentioned proteins than filtrate cells.
Toxin Synthesis
Both forms of biofilms had in common a decreased production of toxin A and B compared to filtrate samples although the effect was more pronounced in aggregate biofilms. In agreement, toxin B mRNA levels were previously determined to be lower in aggregate biofilms than in colony biofilms. Toxin A mRNA levels were found to be decreased in aggregate biofilms vs. planktonic cells (Maldarelli et al., 2016; Poquet et al., 2018). Worth mentioning, toxin expression in C. difficile underlies a sophisticated regulatory network that is tightly coupled to the energy metabolism (Dineen et al., 2007; Antunes et al., 2011; Dubois et al., 2016; Hofmann et al., 2018). In summary, although both biofilms revealed significantly different expression profiles with regard to energy metabolism, toxins were found downregulated in both biofilm models indicating that the biofilm lifestyle rather facilitates persistence than infection.
Sporulation
Although biofilms were initially assumed to be hot spots of sporulation and a potential reservoir for spores during persistence, recent data suggest that this may not be the case and only a few spores can be found in C. difficile biofilms which additionally were determined to be different from other spores with regard to germination efficiency and heat resistance (Ðapa et al., 2013; Semenyuk et al., 2014; Pizarro-Guajardo et al., 2016; Dubois et al., 2019). Moreover, it was reported that sporulation rates in biofilms vary between strains and do not correlate with severity of disease (Semenyuk et al., 2014). In agreement with the previous observations, we determined that sporulation and spore proteins such as spore coat proteins CotA, CotB, SipL, and SpoIVA were less abundant in aggregate biofilms than in filtrate samples which matches the concomitant higher abundance of the negative regulators of sporulation, KipI and Soj, in aggregate biofilms (Ðapa et al., 2013; Poquet et al., 2018; Dubois et al., 2019). In contrast, we found spore proteins significantly higher abundant in colony biofilms. This, however, matches the observation that the carbohydrate utilization- and the amino acid uptake systems App and Opp, whose expression was found negatively correlated with sporulation before (Antunes et al., 2012; Edwards et al., 2014), as well as proteins involved in translation, ribosome maturation and cell division such as RumA, MiaB, BipA, Obg, and InfB showed lower abundance in our colony biofilms than in aggregate biofilms and filtrate samples. Again, these data indicate a higher metabolic activity of C. difficile in aggregate biofilms and in filtrate samples compared to colony biofilms.
Taken together, the lower response levels of aggregate biofilms to unknown metabolic stresses and lower sporulation rates but higher production of antibiotic resistance markers suggest that the extracellular matrix of aggregate biofilms is possibly less dense than in colony biofilms what insufficiently protects cells from antibiotics but prevents accumulation of waste products and allows diffusion of nutrients. While Poquet et al. (2018) who cultivated aggregate biofilms in continuous flow systems argued that the constant renewal of medium is responsible for the observed metabolic activity and low sporulation rates, the data presented here indicate that aggregate biofilms comprise active growing cells regardless of the nutrient supply. Overall, the differential expression of sporulation proteins depending on the choice of biofilm model is an interesting observation and further underlines the urgent need to answer the question of which type of biofilm is produced in the host.
Global Regulatory Circuits and Cell Signaling
Finally, proteins involved in regulation and cell signaling were analyzed to uncover which regulatory circuits possibly underlie the observations discussed in the previous sections. In view of the extensive remodeling of the cell envelope and of metabolic pathways and the tight control of toxin synthesis and sporulation, it was not surprising that multiple central regulatory networks have been affected during biofilm formation in C. difficile (Ðapa et al., 2013; Purcell et al., 2017; Dubois et al., 2019). For example, c-di-GMP signaling and quorum sensing were shown to be required for biofilm formation before (Ðapa et al., 2013; Purcell et al., 2017; Slater et al., 2019). Moreover, several publications have reported that deletion of various regulatory proteins including Spo0A (Dawson et al., 2012; Ðapa et al., 2013), Hfq (Boudry et al., 2014), CcpA (Dubois et al., 2019), CodY (Dubois et al., 2019), and as mentioned above LexA (Bordeleau et al., 20119) impaired biofilm formation in C. difficile. In agreement, proteome data sets presented here suggested that especially aggregate biofilm formation involved complex gene regulatory network restructuring and induction of various transcriptional regulators and two component system proteins (Figure 6 and Supplementary Table 6).
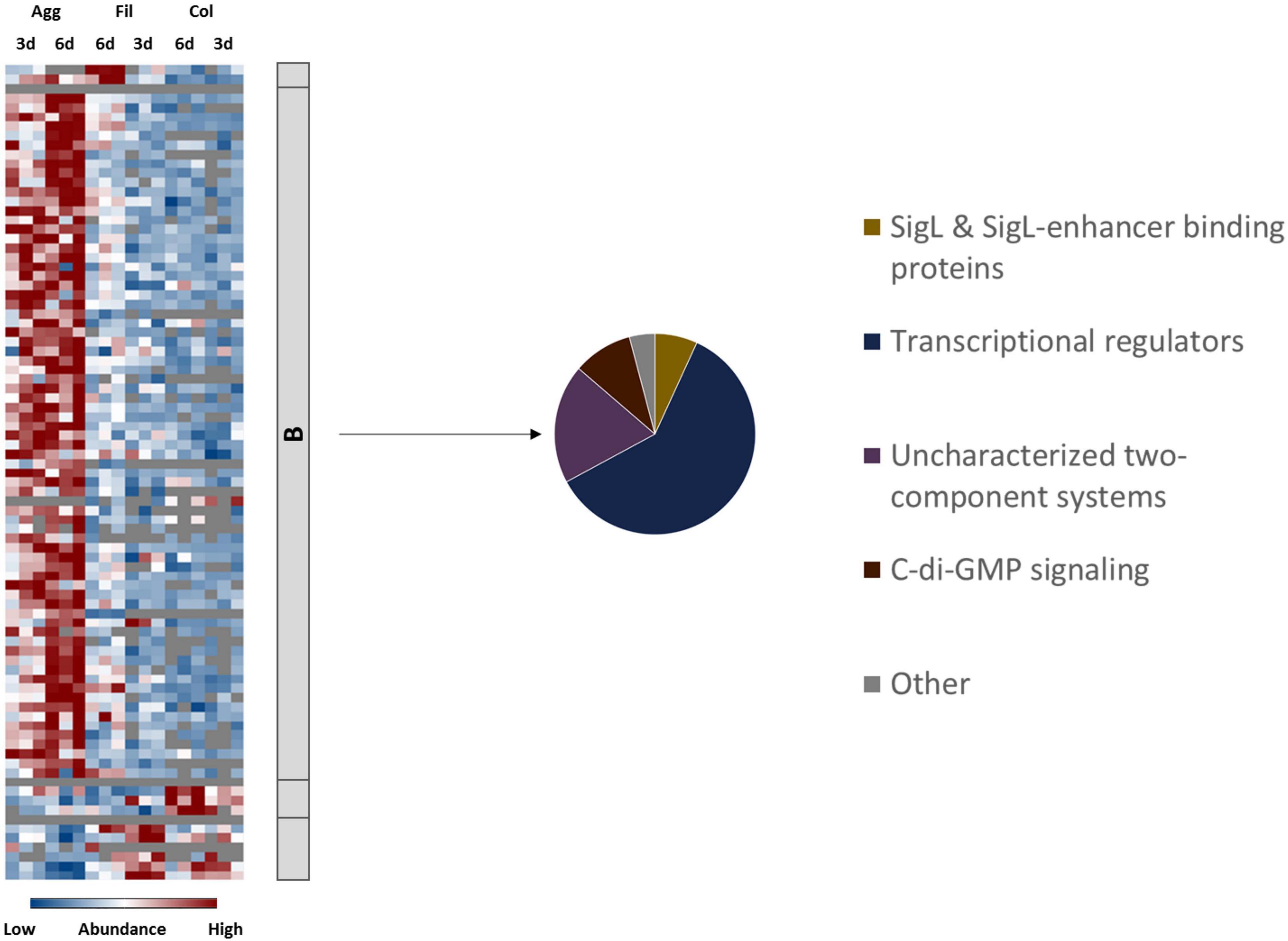
Figure 6. Differential abundance of signaling and regulatory proteins. 84 proteins annotated as regulatory or signaling proteins were differentially abundant (blue is down, red is up) between aggregate biofilms (Agg), filtrate samples (Fil) and colony (Col) from day three (3d) and day six (6d). Z-transformed abundance values are displayed as a color gradient where blue colors indicate weakest abundance levels and red colors the strongest abundance levels among the growth conditions with maximum z-scores of +/–1.5. The heatmap on the left site shows that they were found in all 4 clusters, representing the 6-day old filtrate cells cluster (A), aggregate biofilms (B), colony biofilms (C) or both, colony biofilms and filtrate samples (D). The pie chart on the right reveals that proteins from Cluster B comprised proteins from four major groups of regulatory and signaling proteins. A list of proteins from this heatmap including their fold changes in abundance can be found in Supplementary Table 6 (Supplementary Material). Agg, Aggregate biofilms; Col, Colony biofilms; Fil, Filtrate samples; 3d, Samples from day three; 6d, Samples from day six.
In order to visualize and analyze the complex gene regulatory networks that were active in the different biofilm setups and to potentially assign some of the afore-discussed features of each biofilm type to specific regulatory systems, regulon analyses were performed. To do so, available global expression data of C. difficile strains deleted or overexpressed in central regulators were used to assign C. difficile proteins to their respective regulons. Subsequently, our proteome data were mapped onto obtained regulons and the results were visualized in Voronoi treemaps (Figure 7).
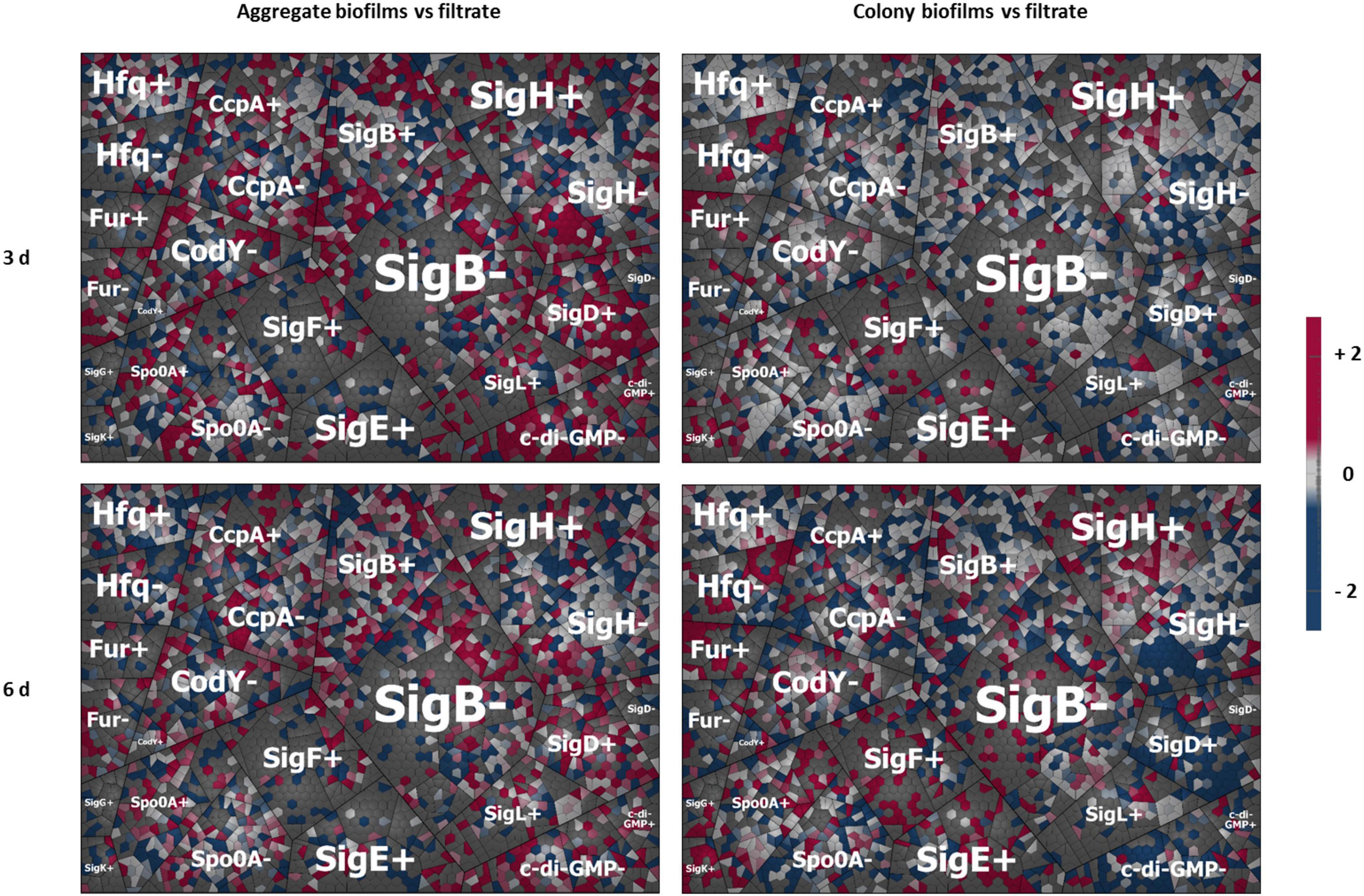
Figure 7. Regulon maps for aggregate and colony biofilms both vs. filtrate samples. Shown are four Voronoi regulon treemap maps. They are summarizing the mapping of our proteome data onto C. difficile’s hitherto characterized regulons created based on available gene expression (transcriptome) data from the literature. All proteins regulated by a respective regulator were depicted as single cells clustered together with all other protein under the control of the same regulator either positively (+) or negatively (–) controlled by the respective regulators. The log2 fold changes of each biofilm sample relative to the corresponding filtrate sample were directly mapped to visualize the impact of a respective regulator on the sample set. Red cells represent proteins induced in biofilm samples relative to filtrate samples while blue cells present proteins downregulated in biofilms relative to filtrate samples. The upper left panel shows the data for aggregate biofilm vs. the filtrate sample after 3 days (3d); lower left panel aggregate biofilms after 6 days (6d); upper right panel colony biofilm after 3 days; lower right panel colony biofilms after 6 days. “+” stands for proteins positively regulated by the respective regulator, “–” for proteins negatively regulated by the respective regulator. A detailed list of all proteins including fold changes and additional information on regulon and operon affiliation can be found in Supplementary Table 6.
Key Regulators of Colony Biofilms
In accordance with the observed induction of sporulation proteins in colony biofilms discussed above, the obtained regulon maps revealed the induction of regulons for the sporulation sigma factors SigE, SigF, SigG, and SigK and the master regulator of sporulation, Spo0A, in the 6-day old colony biofilms (Figure 7). While SigE and SigF were previously shown to be not required for biofilm formation (Dubois et al., 2019), Spo0A was frequently reported to be essential for biofilm formation in C. difficile (Ðapa et al., 2013; Dubois et al., 2019). Moreover, proteins from the SigH and Fur regulon were found upregulated in colony biofilms. Upregulation of Fur proteins might be the result of the impaired diffusion of ions inside the biofilm matrix. The sigma factor SigH is required during transition from exponential growth to stationary phase and was demonstrated to regulate sporulation, toxin production and surface-associated proteins (Saujet et al., 2011). Several of the proteins and operons found to be differentially abundant between colony and aggregate biofilms, such as the flagella operons, the S-layer protein SlpA, the V-type ATPase operon, the oxidative stress response and the glycine reductase complex, were shown to be under control of SigH suggesting that SigH might considerably shape the colony biofilm metabolism (Saujet et al., 2011). In contrast, proteins from the regulon of the motility regulator SigD and the c-di-GMP regulon were rather low abundant in colony biofilms.
Activation of c-di-GMP Signaling in Aggregate Biofilms
c-di-GMP signaling relies on the production of the second messenger c-di-GMP. In contrast to other Gram-positive bacteria, C. difficile’s genome encodes for a large number of functional diguanylate cyclases and phosphodiesterases suggesting special importance of c-di-GMP signaling in C. difficile (Bordeleau et al., 2011). Out of these c-di-GMP associated proteins, the diguanylate cyclases CDIF630erm_01581, CDIF630erm_02043, and CDIF630erm_03665, and the phosphodiesterases PdcA, CDIF630erm_00875 and CDIF630erm_01792 were found significantly upregulated in aggregate biofilms compared to filtrate samples or were even exclusively identified in aggregate biofilms. As shown previously, elevated c-di-GMP levels promote expression of type IV pili that subsequently mediate attachment of the gut bacteria to epithelial cells, cell aggregation and biofilm formation in C. difficile strain R20291 (Purcell et al., 2012, 2016; McKee et al., 2018a). In contrast, flagella proteins that are known to be negatively regulated by c-di-GMP were highly abundant in the aggregate biofilm samples indicating that other regulatory circuits likely interfere with c-di-GMP signaling (Purcell et al., 2012; McKee et al., 2013, 2018b).
The Role of SigL/RpoN in Aggregate Biofilms
Our data suggest that the alternative sigma factor SigL/RpoN, encoded by CD630_31760 and also known as σ54, plays an important role in the gene regulation of aggregate biofilms (Figures 6, 7). The SigL/RpoN regulon has frequently been observed to significantly contribute to biofilm formation in various species by positive regulation of motility (Jagannathan et al., 2001; Saldías et al., 2008; Francke et al., 2011; Iyer and Hancock, 2012), enhancement of extracellular DNA levels inside the extracellular matrix (Iyer and Hancock, 2012) and remodeling of metabolic pathways to adapt to nutrient conditions inside the biofilm (Arous et al., 2004; Francke et al., 2011; Xu et al., 2014; Hayrapetyan et al., 2015). Initially described as sigma regulator induced by nitrogen limitation (Hirschman et al., 1985; Weiss and Magasanik, 1988) SigL/RpoN was later found in most bacterial species to be activated in response to various stressful conditions, such as osmostress and low temperatures (Francke et al., 2011; Nie et al., 2019). The SigL/RpoN regulon in C. difficile and other Clostridiales has recently been characterized leading to the discovery of 30 SigL/RpoN-dependent promoters. Of these 30 SigL/RpoN-dependent promoters, 23 were adjacent to a gene sequence encoding for a SigL/RpoN-dependent regulator, a group of regulators also known as enhancer binding proteins (EBP). Thereby SigL/RpoN was identified as an important central regulator controlling amino acid and carbohydrate catabolism pathways in C. difficile (Nie et al., 2019; Soutourina et al., 2020). Interestingly, 15 of the 23 proposed EBPs for C. difficile were identified in this study. Thirteen EBPs were solely present or detected in higher amounts in aggregate biofilms compared to filtrate samples and colony biofilms suggesting an important role of the SigL/RpoN regulon during aggregate biofilm formation. Indeed, the activation of the SigL/RpoN regulon most likely explains some of the energy-related observations discussed above. For instance, the CDIF630erm_00522-…-etfA1 operon for reductive fermentation of branched chain amino acids, which was higher abundant in aggregate biofilms compared to filtrate samples, is positively controlled by the EBP LeuR which was among the EBPs higher abundant in aggregate biofilms. Similarly, several PTS systems are under control of SigL/RpoN and its EBPs (Soutourina et al., 2020). For instance, the galactitol-specific PTS component CDIF630erm_00104, the mannose-specific PTS components CDIF630erm_00408 and CDIF630_00409 and the ribitol-specific PTS component CDIF630erm_00620 were either found in higher amounts or exclusively in aggregate biofilms. Since toxin synthesis was recently found to be negatively controlled by SigL/RpoN in C. difficile, also the low production of toxins in aggregate biofilms might be a result of SigL/RpoN regulation (Dubois et al., 2016). Overall, our proteome data suggest that SigL/RpoN has an important role in aggregate biofilm formation.
Other Regulatory Circuits Involved in Aggregate Biofilm Formation
Furthermore, the regulon maps revealed a slightly higher induction of proteins from the SigB, CcpA, and CodY regulons in aggregate biofilms than in colony biofilms which may have contributed to the remodeling of the energy metabolism in aggregate biofilm cells (Dineen et al., 2010; Antunes et al., 2012; Kint et al., 2017). In addition, activation of SigB may have contributed to repression of sporulation proteins (Kint et al., 2017). Minor changes in the Spo0A regulon in aggregate biofilms suggest that Spo0A is possibly required for initial or early biofilm formation or cellular adaptation in general rather than maturation and maintenance of biofilm homeostasis. In contrast, the quorum sensing protein LuxS, which was previously shown to be essential for biofilm formation in C. difficile (Ðapa et al., 2013), was decreased in both aggregate and colony biofilms compared to filtrate cells. LuxS was shown to contribute to biofilm formation via induction of prophage genes that in turn induce cell lysis resulting in higher levels of eDNA in the biofilm matrix of strain R20291 (Slater et al., 2019). Possibly, LuxS is more important in early biofilm formation due to its activation in late exponential phase (Carter et al., 2005) or rather required for adaptation to pre-longed survival in nutrient depleted conditions which is a pre-requisite of biofilm formation. Of note, proteome data of this study still demonstrated induction of prophage genes in aggregate biofilms despite the low abundance of LuxS (Figure 5 and Supplementary Table 5).
To conclude, the prominent synthesis of SigL/RpoN, some of its EBPs and SigL/RpoN-dependent operons such as the operon for branched chain amino acid utilization in aggregate biofilms suggest that SigL is an important regulator of aggregate biofilm formation. In addition, c-di-GMP likely shaped the protein inventory of aggregate biofilms while colony biofilms were most likely shaped by the sporulation sigma factors Spo0A, SigE, SigF, SigG, and SigK as well as sigma factor SigH and the transcriptional regulator Fur. However, it should be stressed that biofilm formation is obviously a dynamic process that involves successive action of various regulatory proteins and pathways. Thus, the data of the presented two sample points only allow a temporal narrow view. Moreover, regulon data were not available for all regulators so that the impact of these regulators might be missed. Furthermore, proteins are often under control of several regulators what makes it difficult to unequivocally link adaptation processes to a certain regulator. Nevertheless, the data provide novel in-depth insight into the complex regulatory network of biofilm formation in C. difficile and likely permit helpful conclusions of the necessity of specific regulators which are less prone to bias than regulator knock-out studies.
Conclusion
The comprehensive proteome data presented here shed light on the protein repertoire of C. difficile strain 630Δerm grown in two different biofilm models. Based on the results, we confirmed that free-floating aggregate biofilms behave drastically different from sessile colony biofilms and could possibly be the more relevant biofilm model. Cells of this biofilm type were characterized by significant metabolic activity, flagellation and activation of various regulatory circuits while they neither produced spores or toxins (Table 2), which is in line with previous transcriptomic data (Maldarelli et al., 2016; Poquet et al., 2018). Three aspects might explain some discrepancies observed between previously reported transcriptome approaches, e.g., by Poquet et al. (2018), and the here presented proteome data set. First, discrepancies could be explained by the initially discussed different experimental set ups used to grow biofilms (6-well plates vs. continuous flow system). Second, the choice of the reference sample sets applied (filtrate cells of same age as biofilms vs. 24 h batch cultures) impedes direct comparison of data sets. Lastly, previous observations demonstrated that transcriptome and proteome data do not necessarily follow the same expression trend (Zapalska-Sozoniuk et al., 2019). Therefore, we would like to emphasize that, although detection of a protein should not be mistaken for the protein’s activity, proteome data probably reveal a more reliable picture of what genes are active within the two biofilm models than transcriptome data could do and might subsequently be more relevant for vaccine and antimicrobial design. On that basis, type IV pili were validated as important cell-surface antigens in C. difficile biofilms while the role of flagella and the S-layer remains unclear (Table 2). Moreover, we did not only confirm the importance of c-di-GMP signaling in biofilm formation but further suggested a central role for SigL/RpoN in C. difficile biofilms and conclude that research on this well-conserved regulator is hitherto underrepresented (Table 2). Of note, Tremblay et al. (2021) recently suggested a role for SigL/RpoN in C. difficile biofilms. Likewise, the data presented here emphasize the importance to characterize various still uncharacterized transcriptional regulators and two-component systems of which several were induced in aggregate biofilms (Table 2). Generally, further research is necessary to completely decipher the process of biofilm formation in C. difficile and to identify the nature of infection-relevant biofilms. Thereby, comprehensive in vivo omics studies including proteomic based studies will be inevitable. Moreover, integration of such in vivo data, which to some extent already exist for animal model studies and analyses of patients’ stool samples, with in vitro data such as those presented in this study will be just as important. For instance, transcriptomic and metabolomic data obtained from mice experiments suggest that C. difficile consumes significant amounts of amino acids but also of several carbohydrates such as mannitol during colonization of the intestine (Theriot et al., 2014; Jenior et al., 2017; Pereira et al., 2020). Consequently, the discussed identification of carbohydrate uptake and utilization pathways in the aggregate biofilms might be highly relevant for infection conditions.
Data Availability Statement
The datasets presented in this study can be found in online repositories. The names of the repository/repositories and accession number(s) can be found below: https://www.ebi.ac.uk/pride/archive/, PXD022830.
Author Contributions
CL, KR, and DJ designed the research. CL and CH performed the research. DB and JH were involved in mass spectrometric analyses. MB and CL analyzed the data. MB, CL, DJ, SS, and KR conceptualized and wrote the manuscript. All authors contributed to the article and approved the submitted version.
Funding
This project was funded by the Federal State of Lower Saxony, Niedersächsisches Vorab (VWZN2889), the Federal State of Mecklenburg-Vorpommern (UG 14 001), and the Federal Ministry of Research and Education (BMBF 0315833B, Urogenomics BMF Cooperative project and InfectControl 2020 project “MOASES”). We acknowledge support for the Article Processing Charge from the DFG (German Research Foundation, 393148499) and the Open Access Publication Fund of the University of Greifswald.
Conflict of Interest
The authors declare that the research was conducted in the absence of any commercial or financial relationships that could be construed as a potential conflict of interest.
Acknowledgments
We thank Silvia Dittmann for technical assistance and Daniela Zühlke and Tjorven Hinzke for helpful support during data analysis.
Supplementary Material
The Supplementary Material for this article can be found online at: https://www.frontiersin.org/articles/10.3389/fmicb.2021.682111/full#supplementary-material
References
Anjuwon-Foster, B. R., and Tamayo, R. (2017). A genetic switch controls the production of flagella and toxins in Clostridium difficile. PLoS Genet. 13:e1006701. doi: 10.1371/journal.pgen.1006701
Anjuwon-Foster, B. R., and Tamayo, R. (2018). Phase variation of Clostridium difficile virulence factors. Gut. Microb. 9, 76–83. doi: 10.1080/19490976.2017.1362526
Antunes, A., Camiade, E., Monot, M., Courtois, E., Barbut, F., Sernova, N. V., et al. (2012). Global transcriptional control by glucose and carbon regulator CcpA in Clostridium difficile. Nucleic Acids Res. 40, 10701–10718. doi: 10.1093/nar/gks864
Antunes, A., Martin-Verstraete, I., and Dupuy, B. (2011). CcpA-mediated repression of Clostridium difficile toxin gene expression. Mol. Microbiol. 79, 882–899. doi: 10.1111/j.1365-2958.2010.07495.x
Anwar, H., Strap, J. L., and Costerton, J. W. (1992). Establishment of aging biofilms: possible mechanism of bacterial resistance to antimicrobial therapy. Antimicrobial. Agents Chemother. 36, 1347–1351. doi: 10.1128/aac.36.7.1347
Arato, V., Gasperini, G., Giusti, F., Ferlenghi, I., Scarselli, M., and Leuzzi, R. (2019). Dual role of the colonization factor CD2831 in Clostridium difficile pathogenesis. Sci. Rep. 9:5554. doi: 10.1038/s41598-019-42000-8
Arous, S., Buchrieser, C., Folio, P., Glaser, P., Namane, A., Hébraud, M., et al. (2004). Global analysis of gene expression in an rpoN mutant of Listeria monocytogenes. Microbiology 150, 1581–1590. doi: 10.1099/mic.0.26860-0
Barketi-Klai, A., Hoys, S., Lambert-Bordes, S., Collignon, A., and Kansau, I. (2011). Role of fibronectin-binding protein A in Clostridium difficile intestinal colonization. J. Med. Microbiol. 60, 1155–1161. doi: 10.1099/jmm.0.029553-0
Batah, J., Kobeissy, H., Bui Pham, P. T., Denève-Larrazet, C., Kuehne, S., Collignon, A., et al. (2017). Clostridium difficile flagella induce a pro-inflammatory response in intestinal epithelium of mice in cooperation with toxins. Sci. Rep. 7:3256. doi: 10.1038/s41598-017-03621-z
Berges, M., Michel, A.-M., Lassek, C., Nuss, A. M., Beckstette, M., Dersch, P., et al. (2018). Iron regulation in Clostridioides difficile. Front. Microbiol. 9:3183. doi: 10.3389/fmicb.2018.03183
Bernhardt, J., Michalik, S., Wollscheid, B., Völker, U., and Schmidt, F. (2013). Proteomics approaches for the analysis of enriched microbial subpopulations and visualization of complex functional information. Curr. Opin. Biotechnol. 24, 112–119. doi: 10.1016/j.copbio.2012.10.009
Besharova, O., Suchanek, V. M., Hartmann, R., Drescher, K., and Sourjik, V. (2016). Diversification of gene expression during formation of static submerged biofilms by Escherichia coli. Front. Microbiol. 7:1568. doi: 10.3389/fmicb.2016.01568
Boles, B. R., and Singh, P. K. (2008). Endogenous oxidative stress produces diversity and adaptability in biofilm communities. Proc. Natl. Acad. Sci. U S A. 105, 12503–12508. doi: 10.1073/pnas.0801499105
Bordeleau, E., Fortier, L.-C., Malouin, F., and Burrus, V. (2011). c-di-GMP turn-over in Clostridium difficile is controlled by a plethora of diguanylate cyclases and phosphodiesterases. PLoS Genet. 7:e1002039. doi: 10.1371/journal.pgen.1002039
Bouché, L., Panico, M., Hitchen, P., Binet, D., Sastre, F., Faulds-Pain, A., et al. (2016). The type B flagellin of hypervirulent Clostridium difficile is modified with novel sulfonated peptidylamido-glycans. J. Biol. Chem. 291, 25439–25449. doi: 10.1074/jbc.M116.749481
Boudry, P., Gracia, C., Monot, M., Caillet, J., Saujet, L., Hajnsdorf, E., et al. (2014). Pleiotropic role of the RNA chaperone protein Hfq in the human pathogen Clostridium difficile. J. Bacteriol. 196, 3234–3248. doi: 10.1128/JB.01923-14
Bradshaw, W. J., Bruxelle, J.-F., Kovacs-Simon, A., Harmer, N. J., Janoir, C., Péchiné, S., et al. (2019). Molecular features of lipoprotein CD0873: A potential vaccine against the human pathogen Clostridioides difficile. J. Biol. Chem. 294, 15850–15861. doi: 10.1074/jbc.RA119.010120
Bradshaw, W. J., Kirby, J. M., Roberts, A. K., Shone, C. C., and Acharya, K. R. (2017). Cwp2 from Clostridium difficile exhibits an extended three domain fold and cell adhesion in vitro. FEBS J. 284, 2886–2898. doi: 10.1111/febs.14157
Bruxelle, J. F., Mizrahi, A., Hoys, S., Collignon, A., Janoir, C., and Péchiné, S. (2016). Immunogenic properties of the surface layer precursor of Clostridium difficile and vaccination assays in animal models. Anaerobe 37, 78–84. doi: 10.1016/j.anaerobe.2015.10.010
Bruxelle, J.-F., Mizrahi, A., Hoÿs, S., Collignon, A., Janoir, C., and Péchiné, S. (2017). Clostridium difficile flagellin FliC: evaluation as adjuvant and use in a mucosal vaccine against Clostridium difficile. PLoS One 12:e0187212. doi: 10.1371/journal.pone.0187212
Burmølle, M., Thomsen, T. R., Fazli, M., Dige, I., Christensen, L., Homøe, P., et al. (2010). Biofilms in chronic infections - a matter of opportunity - monospecies biofilms in multispecies infections. FEMS Immunol. Med. Microbiol. 59, 324–336. doi: 10.1111/j.1574-695X.2010.00714.x
Carter, G. P., Des Purdy, Williams, P., and Minton, N. P. (2005). Quorum sensing in Clostridium difficile: analysis of a luxS-type signalling system. J. Med. Microbiol. 54, 119–127. doi: 10.1099/jmm.0.45817-0
Castro-Córdova, P., Díaz-Yáñez, F., Muñoz-Miralles, J., Gil, F., and Paredes-Sabja, D. (2020). Effect of antibiotic to induce Clostridioides difficile-susceptibility and infectious strain in a mouse model of Clostridioides difficile infection and recurrence. Anaerobe 62:102149. doi: 10.1016/j.anaerobe.2020.102149
Chilton, C. H., Pickering, D. S., and Freeman, J. (2018). Microbiologic factors affecting Clostridium difficile recurrence. Clin. Microbiol. Infect. 24, 476–482. doi: 10.1016/j.cmi.2017.11.017
Chong, P. M., Lynch, T., McCorrister, S., Kibsey, P., Miller, M., Gravel, D., et al. (2014). Proteomic analysis of a NAP1 Clostridium difficile clinical isolate resistant to metronidazole. PLoS One 9:e82622. doi: 10.1371/journal.pone.0082622
Chu, M., Mallozzi, M. J. G., Roxas, B. P., Bertolo, L., Monteiro, M. A., Agellon, A., et al. (2016). A Clostridium difficile cell wall glycopolymer locus influences bacterial shape, polysaccharide production and virulence. PLoS Pathog. 12:e1005946. doi: 10.1371/journal.ppat.1005946
Cox, J., and Mann, M. (2008). MaxQuant enables high peptide identification rates, individualized p.p.b.-range mass accuracies and proteome-wide protein quantification. Nat. Biotechnol. 26, 1367–1372. doi: 10.1038/nbt.1511
Crowther, G. S., Chilton, C. H., Todhunter, S. L., Nicholson, S., Freeman, J., Baines, S. D., et al. (2014). Comparison of planktonic and biofilm-associated communities of Clostridium difficile and indigenous gut microbiota in a triple-stage chemostat gut model. J. Antimicrob. Chemother. 69, 2137–2147. doi: 10.1093/jac/dku116
Cuenot, E., Garcia-Garcia, T., Douche, T., Gorgette, O., Courtin, P., Denis-Quanquin, S., et al. (2019). The Ser/Thr kinase PrkC participates in cell wall homeostasis and antimicrobial resistance in Clostridium difficile. Infect. Immun. 87, e00005–e19. doi: 10.1128/IAI.00005-19
Dannheim, H., Riedel, T., Neumann-Schaal, M., Bunk, B., Schober, I., Spröer, C., et al. (2017). Manual curation and reannotation of the genomes of Clostridium difficile 630Δerm and C. difficile 630. J. Med. Microbiol. 66, 286–293. doi: 10.1099/jmm.0.000427
Ðapa, T., Dapa, T., Leuzzi, R., Ng, Y. K., Baban, S. T., Adamo, R., et al. (2013). Multiple factors modulate biofilm formation by the anaerobic pathogen Clostridium difficile. J. Bacteriol. 195, 545–555. doi: 10.1128/JB.01980-12
Dawson, L. F., Valiente, E., Faulds-Pain, A., Donahue, E. H., and Wren, B. W. (2012). Characterisation of Clostridium difficile biofilm formation, a role for Spo0A. PLoS One 7:e50527. doi: 10.1371/journal.pone.0050527
Del Papa, M. F., and Perego, M. (2008). Ethanolamine activates a sensor histidine kinase regulating its utilization in Enterococcus faecalis. J. Bacteriol. 190, 7147–7156. doi: 10.1128/JB.00952-08
Derré, I., Rapoport, G., and Msadek, T. (1999). CtsR, a novel regulator of stress and heat shock response, controls clp and molecular chaperone gene expression in gram-positive bacteria. Mol. Microbiol. 31, 117–131. doi: 10.1046/j.1365-2958.1999.01152.x
Dineen, S. S., McBride, S. M., and Sonenshein, A. L. (2010). Integration of metabolism and virulence by Clostridium difficile CodY. J. Bacteriol. 192, 5350–5362. doi: 10.1128/JB.00341-10
Dineen, S. S., Villapakkam, A. C., Nordman, J. T., and Sonenshein, A. L. (2007). Repression of Clostridium difficile toxin gene expression by CodY. Mol. Microbiol. 66, 206–219. doi: 10.1111/j.1365-2958.2007.05906.x
Doll, M., Marra, A. R., Apisarnthanarak, A., Al-Maani, A. S., Abbas, S., and Rosenthal, V. D. (2021). Prevention of Clostridioides difficile in hospitals: a position paper of the international society for infectious diseases. Int. J. Infec. Dis. 102, 188–195. doi: 10.1016/j.ijid.2020.10.039
Donelli, G., Vuotto, C., Cardines, R., and Mastrantonio, P. (2012). Biofilm-growing intestinal anaerobic bacteria. FEMS Immunol. Med. Microbiol. 65, 318–325. doi: 10.1111/j.1574-695X.2012.00962.x
Dubois, T., Dancer-Thibonnier, M., Monot, M., Hamiot, A., Bouillaut, L., Soutourina, O., et al. (2016). Control of Clostridium difficile physiopathology in response to cysteine availability. Infect. Immun. 84, 2389–2405. doi: 10.1128/IAI.00121-16
Dubois, T., Tremblay, Y. D. N., Hamiot, A., Martin-Verstraete, I., Deschamps, J., Monot, M., et al. (2019). A microbiota-generated bile salt induces biofilm formation in Clostridium difficile. npj Biofilms Microbio. 5, 1–12. doi: 10.1038/s41522-019-0087-4
Edwards, A. N., Nawrocki, K. L., and McBride, S. M. (2014). Conserved oligopeptide permeases modulate sporulation initiation in Clostridium difficile. Infect. Immun. 82, 4276–4291. doi: 10.1128/IAI.02323-14
El Meouche, I., Peltier, J., Monot, M., Soutourina, O., Pestel-Caron, M., Dupuy, B., et al. (2013). Characterization of the SigD regulon of C. difficile and its positive control of toxin production through the regulation of tcdR. PLoS One 8:e83748. doi: 10.1371/journal.pone.0083748
Faulds-Pain, A., Twine, S. M., Vinogradov, E., Strong, P. C. R., Dell, A., Buckley, A. M., et al. (2014). The post-translational modification of the Clostridium difficile flagellin affects motility, cell surface properties and virulence. Mol. Microbiol. 94, 272–289. doi: 10.1111/mmi.12755
Feuerstadt, P., Boules, M., Stong, L., Dahdal, D. N., Sacks, N. C., Lang, K., et al. (2021). Clinical complications in patients with primary and recurrent Clostridioides difficile infection: A real-world data analysis. SAGE Open Med. 9:2050312120986733. doi: 10.1177/2050312120986733
Fimlaid, K. A., Bond, J. P., Schutz, K. C., Putnam, E. E., Leung, J. M., Lawley, T. D., et al. (2013). Global analysis of the sporulation pathway of Clostridium difficile. PLoS Genet. 9:e003660. doi: 10.1371/journal.pgen.1003660
Fletcher, J. R., Erwin, S., Lanzas, C., and Theriot, C. M. (2018). Shifts in the gut metabolome and Clostridium difficile transcriptome throughout colonization and infection in a mouse model. mSphere 3:e00089–e18. doi: 10.1128/mSphere.00089-18
Fletcher, J. R., Pike, C. M., Parsons, R. J., Rivera, A. J., Foley, M. H., McLaren, M. R., et al. (2021). Clostridioides difficile exploits toxin-mediated inflammation to alter the host nutritional landscape and exclude competitors from the gut microbiota. Nat. Commun. 12:462. doi: 10.1038/s41467-020-20746-4
Francke, C., Groot Kormelink, T., Hagemeijer, Y., Overmars, L., Sluijter, V., Moezelaar, R., et al. (2011). Comparative analyses imply that the enigmatic Sigma factor 54 is a central controller of the bacterial exterior. BMC Genom. 12:385. doi: 10.1186/1471-2164-12-385
Ganeshapillai, J., Vinogradov, E., Rousseau, J., Weese, J. S., and Monteiro, M. A. (2008). Clostridium difficile cell-surface polysaccharides composed of pentaglycosyl and hexaglycosyl phosphate repeating units. Carbohydrate Res. 343, 703–710. doi: 10.1016/j.carres.2008.01.002
Gencic, S., and Grahame, D. A. (2020). Diverse energy-conserving pathways in Clostridium difficile: Growth in the absence of amino acid stickland acceptors and the role of the Wood-Ljungdahl Pathway. J. Bacteriol. 202:e00233–e220. doi: 10.1128/JB.00233-20
Gingichashvili, S., Duanis-Assaf, D., Shemesh, M., Featherstone, J. D. B., Feuerstein, O., and Steinberg, D. (2017). Bacillus subtilis biofilm development - a computerized study of morphology and kinetics. Front. Microbiol. 8:2072. doi: 10.3389/fmicb.2017.02072
Gómez, S., Chaves, F., and Orellana, M. A. (2017). Clinical, epidemiological and microbiological characteristics of relapse and re-infection in Clostridium difficile infection. Anaerobe 48, 147–151. doi: 10.1016/j.anaerobe.2017.08.012
Gooyit, M. D., and Janda, K. D. (2016). Modulation of the surface-layer protein of Clostridium difficile through Cwp84 inhibition. ACS Infect. Dis. 2, 465–470. doi: 10.1021/acsinfecdis.6b00061
Goulding, D., Thompson, H., Emerson, J., Fairweather, N. F., Dougan, G., and Douce, G. R. (2009). Distinctive profiles of infection and pathology in hamsters infected with Clostridium difficile strains 630 and B1. Infect. Immun. 77, 5478–5485. doi: 10.1128/IAI.00551-09
Hansen, S. K., Rainey, P. B., Haagensen, J. A. J., and Molin, S. (2007). Evolution of species interactions in a biofilm community. Nature 445, 533–536. doi: 10.1038/nature05514
Hayrapetyan, H., Tempelaars, M., Nierop Groot, M., and Abee, T. (2015). Bacillus cereus ATCC 14579 RpoN (Sigma 54) is a pleiotropic regulator of growth, carbohydrate metabolism, motility, biofilm formation and toxin production. PLoS One 10:e0134872. doi: 10.1371/journal.pone.0134872
Hernandez, B. G., Vinithakumari, A. A., Sponseller, B., Tangudu, C., and Mooyottu, S. (2020). Prevalence, colonization, epidemiology, and public health significance of Clostridioides difficile in companion animals. Front. Veter. Sci. 7:512551. doi: 10.3389/fvets.2020.512551
Hirschman, J., Wong, P. K., Sei, K., Keener, J., and Kustu, S. (1985). Products of nitrogen regulatory genes ntrA and ntrC of enteric bacteria activate glnA transcription in vitro: evidence that the ntrA product is a sigma factor. Proc. NatL. Acad. Sci. U S A. 82, 7525–7529. doi: 10.1073/pnas.82.22.7525
Ho, T. D., and Ellermeier, C. D. (2015). Ferric uptake regulator Fur control of putative iron acquisition systems in Clostridium difficile. J. Bacteriol. 197, 2930–2940. doi: 10.1128/JB.00098-15
Hofmann, J. D., Biedendieck, R., Michel, A.-M., Schomburg, D., Jahn, D., and Neumann-Schaal, M. (2021). Influence of L-lactate and low glucose concentrations on the metabolism and the toxin formation of Clostridioides difficile. PLoS One 16:e0244988. doi: 10.1371/journal.pone.0244988
Hofmann, J. D., Otto, A., Berges, M., Biedendieck, R., Michel, A.-M., Becher, D., et al. (2018). Metabolic reprogramming of Clostridioides difficile during the stationary phase with the induction of toxin production. Front. Microbiol. 9:1970. doi: 10.3389/fmicb.2018.01970
Høiby, N., Bjarnsholt, T., Givskov, M., Molin, S., and Ciofu, O. (2010). Antibiotic resistance of bacterial biofilms. Int. J. Antimicrobial. Agent 35, 322–332. doi: 10.1016/j.ijantimicag.2009.12.011
Hussain, H. A., Roberts, A. P., and Mullany, P. (2005). Generation of an erythromycin-sensitive derivative of Clostridium difficile strain 630 (630Deltaerm) and demonstration that the conjugative transposon Tn916DeltaE enters the genome of this strain at multiple sites. J. Med. Microbiol. 54, 137–141. doi: 10.1099/jmm.0.45790-0
Iyer, V. S., and Hancock, L. E. (2012). Deletion of σ(54) (rpoN) alters the rate of autolysis and biofilm formation in Enterococcus faecalis. J. Bacteriol. 194, 368–375. doi: 10.1128/JB.06046-11
Jackson, S., Calos, M., Myers, A., and Self, W. T. (2006). Analysis of proline reduction in the nosocomial pathogen Clostridium difficile. J. Bacteriol. 188, 8487–8495. doi: 10.1128/JB.01370-06
Jagannathan, A., Constantinidou, C., and Penn, C. W. (2001). Roles of rpoN, fliA, and flgR in expression of flagella in Campylobacter jejuni. J. Bacteriol. 183, 2937–2942. doi: 10.1128/JB.183.9.2937-2942.2001
Jain, S., Graham, C., Graham, R. L. J., McMullan, G., and Ternan, N. G. (2011). Quantitative proteomic analysis of the heat stress response in Clostridium difficile strain 630. J. Proteome Res. 10, 3880–3890. doi: 10.1021/pr200327t
Jain, S., Smyth, D., O’Hagan, B. M. G., Heap, J. T., McMullan, G., Minton, N. P., et al. (2017). Inactivation of the dnaK gene in Clostridium difficile 630 Δerm yields a temperature-sensitive phenotype and increases biofilm-forming ability. Sci. Rep. 7:17522. doi: 10.1038/s41598-017-17583-9
Jamal, M., Ahmad, W., Andleeb, S., Jalil, F., Imran, M., Nawaz, M. A., et al. (2018). Bacterial biofilm and associated infections. J. Chinese Med. Assoc. 81, 7–11. doi: 10.1016/j.jcma.2017.07.012
James, G. A., Chesnel, L., Boegli, L., deLancey Pulcini, E., Fisher, S., and Stewart, P. S. (2018). Analysis of Clostridium difficile biofilms: imaging and antimicrobial treatment. J. Antimicrobial. Agents Chemother. 73, 102–108. doi: 10.1093/jac/dkx353
Janoir, C., Denève, C., Bouttier, S., Barbut, F., Hoys, S., Caleechum, L., et al. (2013). Adaptive strategies and pathogenesis of Clostridium difficile from in vivo transcriptomics. Infect. Immun. 81, 3757–3769. doi: 10.1128/IAI.00515-13
Jenior, M. L., Leslie, J. L., Young, V. B., and Schloss, P. D. (2017). Clostridium difficile colonizes alternative nutrient niches during infection across distinct murine gut microbiomes. mSystems 2:e00063–e17. doi: 10.1128/mSystems.00063-17
Jenior, M. L., Leslie, J. L., Young, V. B., and Schloss, P. D. (2018). Clostridium difficile alters the structure and metabolism of distinct cecal microbiomes during initial infection to promote sustained colonization. mSphere 3:e00261–e18. doi: 10.1128/mSphere.00261-18
Karygianni, L., Ren, Z., Koo, H., and Thurnheer, T. (2020). Biofilm matrixome: Extracellular components in structured microbial communities. Trends Microbiol. 28, 668–681. doi: 10.1016/j.tim.2020.03.016
Kesel, S., Bronk, B., von, Falcón García, C., Götz, A., Lieleg, O., et al. (2017). Matrix composition determines the dimensions of Bacillus subtilis NCIB 3610 biofilm colonies grown on LB agar. RSC Adv. 7, 31886–31898. doi: 10.1039/C7RA05559E
Khader, K., Munoz-Price, L. S., Hanson, R., Stevens, V., Keegan, L. T., Thomas, A., et al. (2021). Transmission dynamics of Clostridioides difficile in 2 high-acuity hospital units. Clin. Infec. Dis. 72, S1–S7. doi: 10.1093/cid/ciaa1580
Kim, J., Darley, D., Selmer, T., and Buckel, W. (2006). Characterization of (R)-2-hydroxyisocaproate dehydrogenase and a family III coenzyme A transferase involved in reduction of L-leucine to isocaproate by Clostridium difficile. Appl. Environ. Microbiol. 72, 6062–6069. doi: 10.1128/AEM.00772-06
Kint, N., Janoir, C., Monot, M., Hoys, S., Soutourina, O., Dupuy, B., et al. (2017). The alternative sigma factor σB plays a crucial role in adaptive strategies of Clostridium difficile during gut infection. Environ. Microbiol. 19, 1933–1958. doi: 10.1111/1462-2920.13696
Kirby, J. M., Ahern, H., Roberts, A. K., Kumar, V., Freeman, Z., Acharya, K. R., et al. (2009). Cwp84, a surface-associated cysteine protease, plays a role in the maturation of the surface layer of Clostridium difficile. J. Biol. Chem. 284, 34666–34673. doi: 10.1074/jbc.M109.051177
Kirk, J. A., Banerji, O., and Fagan, R. P. (2017a). Characteristics of the Clostridium difficile cell envelope and its importance in therapeutics. Microbial. Biotechnol. 10, 76–90. doi: 10.1111/1751-7915.12372
Kirk, J. A., Gebhart, D., Buckley, A. M., Lok, S., Scholl, D., Douce, G. R., et al. (2017b). New class of precision antimicrobials redefines role of Clostridium difficile S-layer in virulence and viability. Sci. Transl. Med. 9:eaah6813. doi: 10.1126/scitranslmed.aah6813
Köpke, M., Straub, M., and Dürre, P. (2013). Clostridium difficile is an autotrophic bacterial pathogen. PLoS One 8:e62157. doi: 10.1371/journal.pone.0062157
La Riva, L., de, Willing, S. E., Tate, E. W., and Fairweather, N. F. (2011). Roles of cysteine proteases Cwp84 and Cwp13 in biogenesis of the cell wall of Clostridium difficile. J. Bacteriol. 193, 3276–3285. doi: 10.1128/JB.00248-11
Laemmli, U. K. (1970). Cleavage of structural proteins during the assembly of the head of bacteriophage T4. Nature 227, 680–685. doi: 10.1038/227680a0
Lawley, T. D., Clare, S., Walker, A. W., Goulding, D., Stabler, R. A., Croucher, N., et al. (2009). Antibiotic treatment of clostridium difficile carrier mice triggers a supershedder state, spore-mediated transmission, and severe disease in immunocompromised hosts. Infec. Immunol. 77, 3661–3669. doi: 10.1128/IAI.00558-09
Lawley, T. D., Clare, S., Walker, A. W., Stares, M. D., Connor, T. R., Raisen, C., et al. (2012). Targeted restoration of the intestinal microbiota with a simple, defined bacteriotherapy resolves relapsing Clostridium difficile disease in mice. PLoS Pathog. 8:e1002995. doi: 10.1371/journal.ppat.1002995
Levin, B. R., and Cornejo, O. E. (2009). The population and evolutionary dynamics of homologous gene recombination in bacterial populations. PLoS Genet. 5:e1000601. doi: 10.1371/journal.pgen.1000601
Maldarelli, G. A., Piepenbrink, K. H., Scott, A. J., Freiberg, J. A., Song, Y., Achermann, Y., et al. (2016). Type IV pili promote early biofilm formation by Clostridium difficile. Pathog. Dis. 74:ftw061. doi: 10.1093/femspd/ftw061
Martínez-Meléndez, A., Morfin-Otero, R., Villarreal-Treviño, L., Baines, S. D., Camacho-Ortíz, A., and Garza-González, E. (2020). Molecular epidemiology of predominant and emerging Clostridioides difficile ribotypes. J. Microbiol. Methods 175:105974. doi: 10.1016/j.mimet.2020.105974
Mathur, H., Rea, M. C., Cotter, P. D., Hill, C., and Ross, R. P. (2016). The efficacy of thuricin CD, tigecycline, vancomycin, teicoplanin, rifampicin and nitazoxanide, independently and in paired combinations against Clostridium difficile biofilms and planktonic cells. Gut Pathog. 8:20. doi: 10.1186/s13099-016-0102-8
McBride, S. M., and Sonenshein, A. L. (2011). The dlt operon confers resistance to cationic antimicrobial peptides in Clostridium difficile. Microbiology 157, 1457–1465. doi: 10.1099/mic.0.045997-0
McKee, R. W., Aleksanyan, N., Garrett, E. M., and Tamayo, R. (2018a). Type IV pili promote Clostridium difficile adherence and persistence in a mouse model of infection. Infec. Immun. 86:e00943–e17. doi: 10.1128/IAI.00943-17
McKee, R. W., Harvest, C. K., and Tamayo, R. (2018b). Cyclic diguanylate regulates virulence factor genes via multiple riboswitches in Clostridium difficile. mSphere 3:e00423–e18. doi: 10.1128/mSphere.00423-18
McKee, R. W., Mangalea, M. R., Purcell, E. B., Borchardt, E. K., and Tamayo, R. (2013). The second messenger cyclic Di-GMP regulates Clostridium difficile toxin production by controlling expression of sigD. J. Bacteriol. 195, 5174–5185. doi: 10.1128/JB.00501-13
McLure, A., Clements, A. C. A., Kirk, M., and Glass, K. (2019). Modelling diverse sources of Clostridium difficile in the community: importance of animals, infants and asymptomatic carriers. Epidemiol. Infec. 147:e152. doi: 10.1017/S0950268819000384
Merrigan, M. M., Venugopal, A., Roxas, J. L., Anwar, F., Mallozzi, M. J., Roxas, B. A. P., et al. (2013). Surface-layer protein A (SlpA) is a major contributor to host-cell adherence of Clostridium difficile. PLoS One 8:e78404. doi: 10.1371/journal.pone.0078404
Mizrahi, A., Bruxelle, J. F., Péchiné, S., and Le, M. A. (2018). Prospective evaluation of the adaptive immune response to SlpA in Clostridium difficile infection. Anaerobe 54, 164–168. doi: 10.1016/j.anaerobe.2018.09.008
Molin, S., and Tolker-Nielsen, T. (2003). Gene transfer occurs with enhanced efficiency in biofilms and induces enhanced stabilisation of the biofilm structure. Curr. Opin. Biotechnol. 14, 255–261. doi: 10.1016/S0958-1669(03)00036-3
Mullany, P., Wilks, M., Lamb, I., Clayton, C., Wren, B., and Tabaqchali, S. (1990). Genetic analysis of a tetracycline resistance element from Clostridium difficile and its conjugal transfer to and from Bacillus subtilis. J. General Microbiol. 136, 1343–1349. doi: 10.1099/00221287-136-7-1343
Müller, V., Imkamp, F., Biegel, E., Schmidt, S., and Dilling, S. (2008). Discovery of a ferredoxin:NAD+-oxidoreductase (Rnf) in Acetobacterium woodii: a novel potential coupling site in acetogens. Ann. N.Y. Acad. Sci. 1125, 137–146. doi: 10.1196/annals.1419.011
Nasiri, M. J., Goudarzi, M., Hajikhani, B., Ghazi, M., Goudarzi, H., and Pouriran, R. (2018). Clostridioides (Clostridium) difficile infection in hospitalized patients with antibiotic-associated diarrhea: A systematic review and meta-analysis. Anaerobe 50, 32–37. doi: 10.1016/j.anaerobe.2018.01.011
Nawrocki, K. L., Wetzel, D., Jones, J. B., Woods, E. C., and McBride, S. M. (2018). Ethanolamine is a valuable nutrient source that impacts Clostridium difficile pathogenesis. Environ. Microbiol. 20, 1419–1435. doi: 10.1111/1462-2920.14048
Neumann-Schaal, M., Hofmann, J. D., Will, S. E., and Schomburg, D. (2015). Time-resolved amino acid uptake of Clostridium difficile 630Δerm and concomitant fermentation product and toxin formation. BMC Microbiol. 15:281. doi: 10.1186/s12866-015-0614-2
Neumann-Schaal, M., Metzendorf, N. G., Troitzsch, D., Nuss, A. M., Hofmann, J. D., Beckstette, M., et al. (2018). Tracking gene expression and oxidative damage of O2-stressed Clostridioides difficile by a multi-omics approach. Anaerobe 53, 94–107. doi: 10.1016/j.anaerobe.2018.05.018
Nie, X., Dong, W., and Yang, C. (2019). Genomic reconstruction of σ 54 regulons in Clostridiales. BMC Genom. 20, 1–14. doi: 10.1186/s12864-019-5918-4
Normington, C., Moura, I. B., Bryant, J. A., Ewin, D. J., Clark, E. V., Kettle, M. J., et al. (2021). Biofilms harbour Clostridioides difficile, serving as a reservoir for recurrent infection. npj Biofilms Microbio. 7:16. doi: 10.1038/s41522-021-00184-w
Oka, K., Osaki, T., Hanawa, T., Kurata, S., Okazaki, M., Manzoku, T., et al. (2012). Molecular and microbiological characterization of Clostridium difficile isolates from single, relapse, and reinfection cases. J. Clin. Microbiol. 50, 915–921. doi: 10.1128/JCM.05588-11
Oliveira, N. M., Oliveria, N. M., Martinez-Garcia, E., Xavier, J., Durham, W. M., Kolter, R., et al. (2015). Biofilm formation as a response to ecological competition. PLoS Biol. 13:e1002191. doi: 10.1371/journal.pbio.1002191
Onderdonk, A. B., Lowe, B. R., and Bartlett, J. G. (1979). Effect of environmental stress on Clostridium difficile toxin levels during continuous cultivation. Appl. Environ. Microbiol. 38, 637–641. doi: 10.1128/AEM.38.4.637-641.1979
Otto, A., Maaß, S., Lassek, C., Becher, D., Hecker, M., Riedel, K., et al. (2016). The protein inventory of Clostridium difficile grown in complex and minimal medium. Proteomics Clin. Applicat. 10, 1068–1072. doi: 10.1002/prca.201600069
Pantaléon, V., Monot, M., Eckert, C., Hoys, S., Collignon, A., Janoir, C., et al. (2018). Clostridium difficile forms variable biofilms on abiotic surface. Anaerobe 53, 34–37. doi: 10.1016/j.anaerobe.2018.05.006
Pantaléon, V., Soavelomandroso, A. P., Bouttier, S., Briandet, R., Roxas, B., Chu, M., et al. (2015). The Clostridium difficile protease Cwp84 modulates both biofilm formation and cell-surface properties. PLoS One 10:e0124971. doi: 10.1371/journal.pone.0124971
Péchiné, S., Bruxelle, J. F., Janoir, C., and Collignon, A. (2018). Targeting Clostridium difficile surface components to develop immunotherapeutic strategies against Clostridium difficile infection. Front. Microbiol. 9:1009. doi: 10.3389/fmicb.2018.01009
Péchiné, S., Denève, C., Le Monnier, A., Hoys, S., Janoir, C., and Collignon, A. (2011). Immunization of hamsters against Clostridium difficile infection using the Cwp84 protease as an antigen. FEMS Immunol. Med. Microbiol. 63, 73–81. doi: 10.1111/j.1574-695X.2011.00832.x
Pereira, F. C., Wasmund, K., Cobankovic, I., Jehmlich, N., Herbold, C. W., Lee, K. S., et al. (2020). Rational design of a microbial consortium of mucosal sugar utilizers reduces Clostridiodes difficile colonization. Nat. Commun. 11:5104. doi: 10.1038/s41467-020-18928-1
Pettit, L. J., Browne, H. P., Yu, L., Smits, W. K., Fagan, R. P., Barquist, et al. (2014). Functional genomics reveals that Clostridium difficile Spo0A coordinates sporulation, virulence and metabolism. BMC Genom. 15, 1–15. doi: 10.1186/1471-2164-15-160
Pike, C. M., and Theriot, C. M. (2020). Mechanisms of colonization resistance against Clostridioides difficile. J. Infec. Dis. 2020:jiaa408. doi: 10.1093/infdis/jiaa408
Pizarro-Guajardo, M., Calderón-Romero, P., and Paredes-Sabja, D. (2016). Ultrastructure variability of the exosporium layer of Clostridium difficile spores from sporulating cultures and biofilms. Appl. Environ. Microbiol. 82, 5892–5898. doi: 10.1128/AEM.01463-16
Plaza-Garrido, Á, Miranda-Cárdenas, C., Castro-Córdova, P., Olguín-Araneda, V., Cofré-Araneda, G., Hernández-Rocha, C., et al. (2015). Outcome of relapsing Clostridium difficile infections do not correlate with virulence-, spore- and vegetative cell-associated phenotypes. Anaerobe 36, 30–38. doi: 10.1016/j.anaerobe.2015.09.005
Poquet, I., Saujet, L., Canette, A., Monot, M., Mihajlovic, J., Ghigo, J.-M., et al. (2018). Clostridium difficile biofilm: Remodeling metabolism and cell surface to build a sparse and heterogeneously aggregated architecture. Front. Microbiol. 9:2084. doi: 10.3389/fmicb.2018.02084
Purcell, E. B., McKee, R. W., Bordeleau, E., Burrus, V., and Tamayo, R. (2016). Regulation of type IV pili contributes to surface behaviors of historical and epidemic strains of Clostridium difficile. J. Bacteriol. 198, 565–577. doi: 10.1128/JB.00816-15
Purcell, E. B., McKee, R. W., Courson, D. S., Garrett, E. M., McBride, S. M., Cheney, R. E., et al. (2017). A nutrient-regulated cyclic diguanylate phosphodiesterase controls Clostridium difficile biofilm and toxin production during stationary phase. Infec. Immun. 85:e00347–e17. doi: 10.1128/IAI.00347-17
Purcell, E. B., McKee, R. W., McBride, S. M., Waters, C. M., and Tamayo, R. (2012). Cyclic diguanylate inversely regulates motility and aggregation in Clostridium difficile. J. Bacteriol. 194, 3307–3316. doi: 10.1128/JB.00100-12
Reid, C. W., Vinogradov, E., Li, J., Jarrell, H. C., Logan, S. M., and Brisson, J.-R. (2012). Structural characterization of surface glycans from Clostridium difficile. Carbohydrate Res. 354, 65–73. doi: 10.1016/j.carres.2012.02.002
Ren, D., Madsen, J. S., Sørensen, S. J., and Burmølle, M. (2015). High prevalence of biofilm synergy among bacterial soil isolates in cocultures indicates bacterial interspecific cooperation. ISME J. 9, 81–89. doi: 10.1038/ismej.2014.96
Reynolds, C. B., Emerson, J. E., La Riva, L., de, Fagan, R. P., and Fairweather, N. F. (2011). The Clostridium difficile cell wall protein CwpV is antigenically variable between strains, but exhibits conserved aggregation-promoting function. PLoS Pathog. 7:e1002024. doi: 10.1371/journal.ppat.1002024
Richards, E., Bouché, L., Panico, M., Arbeloa, A., Vinogradov, E., Morris, H., et al. (2018). The S-layer protein of a Clostridium difficile SLCT-11 strain displays a complex glycan required for normal cell growth and morphology. J. Biol. Chem. 293, 18123–18137. doi: 10.1074/jbc.RA118.004530
Riedel, T., Wetzel, D., Hofmann, J. D., Plorin, Simon Paul, Erich Otto, et al. (2017). High metabolic versatility of different toxigenic and non-toxigenic Clostridioides difficile isolates. Int. J. Med. Microbiol. 307, 311–320. doi: 10.1016/j.ijmm.2017.05.007
Ryder, V. J., Chopra, I., and O’Neill, A. J. (2012). Increased mutability of Staphylococci in biofilms as a consequence of oxidative stress. PLoS One 7:e47695. doi: 10.1371/journal.pone.0047695
Saldías, M. S., Lamothe, J., Wu, R., and Valvano, M. A. (2008). Burkholderia cenocepacia requires the RpoN sigma factor for biofilm formation and intracellular trafficking within macrophages. Infec. Immun. 76, 1059–1067. doi: 10.1128/IAI.01167-07
Saujet, L., Monot, M., Dupuy, B., Soutourina, O., and Martin-Verstraete, I. (2011). The key sigma factor of transition phase, SigH, controls sporulation, metabolism, and virulence factor expression in Clostridium difficile. J. Bacteriol. 193, 3186–3196. doi: 10.1128/JB.00272-11
Schade, J., and Weidenmaier, C. (2016). Cell wall glycopolymers of firmicutes and their role as nonprotein adhesins. FEBS Lett. 590, 3758–3771. doi: 10.1002/1873-3468.12288
Schiffels, J., and Selmer, T. (2019). Combinatorial assembly of ferredoxin-linked modules in Escherichia coli yields a testing platform for Rnf-complexes. Biotechnol. Bioeng. 116, 2316–2329. doi: 10.1002/bit.27079
Schulz, A., and Schumann, W. (1996). hrcA, the first gene of the Bacillus subtilis dnaK operon encodes a negative regulator of class I heat shock genes. J. Bacteriol. 178, 1088–1093.
Sebaihia, M., Wren, B. W., Mullany, P., Fairweather, N. F., Minton, N., Stabler, R., et al. (2006). The multidrug-resistant human pathogen Clostridium difficile has a highly mobile, mosaic genome. Nat. Genet. 38, 779–786. doi: 10.1038/ng1830
Semenyuk, E. G., Laning, M. L., Foley, J., Johnston, P. F., Knight, K. L., Gerding, D. N., et al. (2014). Spore formation and toxin production in Clostridium difficile biofilms. PLoS One 9:e87757. doi: 10.1371/journal.pone.0087757
Shin, J.-B., Krey, J. F., Hassan, A., Metlagel, Z., Tauscher, A. N., Pagana, J. M., et al. (2013). Molecular architecture of the chick vestibular hair bundle. Nat. Neurosci. 16, 365–374. doi: 10.1038/nn.3312
Sievers, S., Metzendorf, N. G., Dittmann, S., Troitzsch, D., Gast, V., Tröger, S. M., et al. (2019). Differential view on the bile acid stress response of Clostridioides difficile. Front. Microbiol. 10:258. doi: 10.3389/fmicb.2019.00258
Sirard, S., Valiquette, L., and Fortier, L.-C. (2011). Lack of association between clinical outcome of Clostridium difficile infections, strain type, and virulence-associated phenotypes. J. Clin. Microbiol. 49, 4040–4046. doi: 10.1128/JCM.05053-11
Slater, R. T., Frost, L. R., Jossi, S. E., Millard, A. D., and Unnikrishnan, M. (2019). Clostridioides difficile LuxS mediates inter-bacterial interactions within biofilms. Sci. Rep. 9:9903. doi: 10.1038/s41598-019-46143-6
Smits, W. K. (2013). Hype or hypervirulence: a reflection on problematic C. difficile strains. Virulence 4, 592–596. doi: 10.4161/viru.26297
Soavelomandroso, A. P., Gaudin, F., Hoys, S., Nicolas, V., Vedantam, G., Janoir, C., et al. (2017). Biofilm structures in a mono-associated mouse model of Clostridium difficile infection. Front. Microbiol. 8:2086. doi: 10.3389/fmicb.2017.02086
Soutourina, O., Dubois, T., Monot, M., Shelyakin, P. V., Saujet, L., Boudry, P., et al. (2020). Genome-wide transcription start site mapping and promoter assignments to a sigma factor in the human enteropathogen Clostridioides difficile. Front. Microbiol. 11:1939. doi: 10.3389/fmicb.2020.01939
Soutourina, O. A., Monot, M., Boudry, P., Saujet, L., Pichon, C., Sismeiro, O., et al. (2013). Genome-wide identification of regulatory RNAs in the human pathogen Clostridium difficile. PLoS Genet. 9:e1003493. doi: 10.1371/journal.pgen.1003493
Stickland, L. H. (1934). Studies in the metabolism of the strict anaerobes (genus Clostridium): The chemical reactions by which Cl. sporogenes obtains its energy. Biochem. J. 28, 1746–1759. doi: 10.1042/bj0281746
Stupperich, E., Hammel, K. E., Fuchs, G., and Thauer, R. K. (1983). Carbon monoxide fixation into the carboxyl group of acetyl coenzyme A during autotrophic growth of Methanobacterium. FEBS Lett. 152, 21–23. doi: 10.1016/0014-5793(83)80473-6
Ternan, N. G., Jain, S., Srivastava, M., and McMullan, G. (2012). Comparative transcriptional analysis of clinically relevant heat stress response in Clostridium difficile strain 630. PLoS One 7:e42410. doi: 10.1371/journal.pone.0042410
Theriot, C. M., Koenigsknecht, M. J., Paul, E., Carlson, Gabrielle, E., Hatton, et al. (2014). Antibiotic-induced shifts in the mouse gut microbiome and metabolome increase susceptibility to Clostridium difficile infection. Nat. Commun. 5, 1–10. doi: 10.1038/ncomms4114
Tijerina-Rodríguez, L., Villarreal-Treviño, L., Morfín-Otero, R., Camacho-Ortíz, A., and Garza-González, E. (2019). Virulence factors of Clostridioides (Clostridium) difficile linked to recurrent infections. Can. J. Infec. Dis. Med. Microbiol. 2019:7127850. doi: 10.1155/2019/7127850
Tremblay, Y. D. N., Durand, B. A. R., Hamiot, A., Martin-Verstraete, I., Oberkampf, M., Monot, M., et al. (2021). Metabolic adaption to extracellular pyruvate triggers biofilm formation in Clostridioides difficile. bioRxiv doi: 10.1101/2021.01.23.427917.
Troitzsch, D., Zhang, H., Dittmann, S., Düsterhöft, D., Möller, T. A., Michel, A.-M., et al. (2021). A point mutation in the transcriptional repressor PerR results in a constitutive oxidative stress response in Clostridioides difficile 630Δerm. mSphere 6:e00091–21. doi: 10.1128/mSphere.00091-21
Twine, S. M., Reid, C. W., Aubry, A., McMullin, D. R., Fulton, K. M., Austin, J., et al. (2009). Motility and flagellar glycosylation in Clostridium difficile. J. Bacteriol. 191, 7050–7062. doi: 10.1128/JB.00861-09
Tyanova, S., Temu, T., and Cox, J. (2016a). The MaxQuant computational platform for mass spectrometry-based shotgun proteomics. Nat. Prot. 11, 2301–2319. doi: 10.1038/nprot.2016.136
Tyanova, S., Temu, T., Sinitcyn, P., Carlson, A., Hein, M. Y., Geiger, T., et al. (2016b). The Perseus computational platform for comprehensive analysis of (prote)omics data. Nat. Methods 13, 731–740. doi: 10.1038/nmeth.3901
Underwood, S., Guan, S., Vijayasubhash, V., Baines, S. D., Graham, L., Lewis, R. J., et al. (2009). Characterization of the sporulation initiation pathway of Clostridium difficile and its role in toxin production. J. Bacteriol. 191, 7296–7305. doi: 10.1128/JB.00882-09
Valiente, E., Bouché, L., Hitchen, P., Faulds-Pain, A., Songane, M., Dawson, L. F., et al. (2016). Role of glycosyltransferases modifying type B flagellin of emerging hypervirulent Clostridium difficile lineages and their impact on motility and biofilm formation. J. Biol. Chem. 291, 25450–25461. doi: 10.1074/jbc.M116.749523
van Eijk, E., Anvar, S. Y., Browne, H. P., Leung, W. Y., Frank, J., Schmitz, A. M., et al. (2015). Complete genome sequence of the Clostridium difficile laboratory strain 630Δerm reveals differences from strain 630, including translocation of the mobile element CTn5. BMC Genom. 16:31. doi: 10.1186/s12864-015-1252-7
Vaz, F., Wilson, G., Kirk, J., Salgado, P., Fagan, R., and Douce, G. (2019). Unraveling the role of C. difficile S-layer in infection and disease. Access Microbiol. 1:86. doi: 10.1099/acmi.ac2019.po0086
Vlamakis, H., Aguilar, C., Losick, R., and Kolter, R. (2008). Control of cell fate by the formation of an architecturally complex bacterial community. Genes Dev. 22, 945–953. doi: 10.1101/gad.1645008
Vuotto, C., Donelli, G., Buckley, A., and Chilton, C. (2018). Clostridium difficile biofilm. Adv. Exper. Med. Biol. 1050, 97–115. doi: 10.1007/978-3-319-72799-8_7
Waligora, A. J., Hennequin, C., Mullany, P., Bourlioux, P., Collignon, A., and Karjalainen, T. (2001). Characterization of a cell surface protein of Clostridium difficile with adhesive properties. Infec. Immun. 69, 2144–2153. doi: 10.1128/IAI.69.4.2144-2153.2001
Walter, B. M., Cartman, S. T., Minton, N. P., Butala, M., and Rupnik, M. (2015). The SOS response master regulator LexA is associated with sporulation, motility and biofilm formation in Clostridium difficile. PLoS One 10:e0144763. doi: 10.1371/journal.pone.0144763
Weiss, V., and Magasanik, B. (1988). Phosphorylation of nitrogen regulator I (NRI) of Escherichia coli. Proc. Natl. Acad. Sci. U S A. 85, 8919–8923.
Werner, A., Mölling, P., Fagerström, A., Dyrkell, F., Arnellos, D., Johansson, K., et al. (2020). Whole genome sequencing of Clostridioides difficile PCR ribotype 046 suggests transmission between pigs and humans. PLoS One 15:e0244227. doi: 10.1371/journal.pone.0244227
Wiegand, P. N., Nathwani, D., Wilcox, M. H., Stephens, J., Shelbaya, A., and Haider, S. (2012). Clinical and economic burden of Clostridium difficile infection in Europe: a systematic review of healthcare-facility-acquired infection. J. Hospital Infec. 81, 1–14. doi: 10.1016/j.jhin.2012.02.004
Willing, S. E., Candela, T., Shaw, H. A., Seager, Z., Mesnage, S., Fagan, R. P., et al. (2015). Clostridium difficile surface proteins are anchored to the cell wall using CWB2 motifs that recognise the anionic polymer PSII. Mol. Microbiol. 96, 596–608. doi: 10.1111/mmi.12958
Woods, E. C., Edwards, A. N., Childress, K. O., Jones, J. B., and McBride, S. M. (2018). The C. difficile clnRAB operon initiates adaptations to the host environment in response to LL-37. PLoS Pathog. 14:e1007153. doi: 10.1371/journal.ppat.1007153
Xu, Y.-B., Chen, M., Zhang, Y., Wang, M., Wang, Y., Huang, Q.-B., et al. (2014). The phosphotransferase system gene ptsI in the endophytic bacterium Bacillus cereus is required for biofilm formation, colonization, and biocontrol against wheat sharp eyespot. FEMS Microbiol. Lett. 354, 142–152. doi: 10.1111/1574-6968.12438
Yu, N. Y., Wagner, J. R., Laird, M. R., Melli, G., Rey, S., Lo, R., et al. (2010). PSORTb 3.0: improved protein subcellular localization prediction with refined localization subcategories and predictive capabilities for all prokaryotes. Bioinformatics 26, 1608–1615. doi: 10.1093/bioinformatics/btq249
Keywords: biofilm, colony biofilm, aggregate biofilm, Clostridioides difficile, proteomics, cell surface antigens, RpoN signaling
Citation: Brauer M, Lassek C, Hinze C, Hoyer J, Becher D, Jahn D, Sievers S and Riedel K (2021) What’s a Biofilm?—How the Choice of the Biofilm Model Impacts the Protein Inventory of Clostridioides difficile. Front. Microbiol. 12:682111. doi: 10.3389/fmicb.2021.682111
Received: 17 March 2021; Accepted: 12 May 2021;
Published: 10 June 2021.
Edited by:
George Grant, University of Aberdeen, United KingdomReviewed by:
Thomas Candela, Université Paris-Saclay, FranceAnthony Buckley, University of Leeds, United Kingdom
Copyright © 2021 Brauer, Lassek, Hinze, Hoyer, Becher, Jahn, Sievers and Riedel. This is an open-access article distributed under the terms of the Creative Commons Attribution License (CC BY). The use, distribution or reproduction in other forums is permitted, provided the original author(s) and the copyright owner(s) are credited and that the original publication in this journal is cited, in accordance with accepted academic practice. No use, distribution or reproduction is permitted which does not comply with these terms.
*Correspondence: Katharina Riedel, cmllZGVsYUB1bmktZ3JlaWZzd2FsZC5kZQ==