- 1Fujian Provincial Key Laboratory of Agroecological Processing and Safety Monitoring, College of Life Sciences, Fujian Agriculture and Forestry University, Fuzhou, China
- 2Key Laboratory for Genetics, Breeding and Multiple Utilization of Crops, Ministry of Education, College of Crop Sciences, Fujian Agriculture and Forestry University, Fuzhou, China
- 3Department of Crop Science, National University of Lesotho, Maseru, Lesotho
- 4College of Crop Sciences, Fujian Agriculture and Forestry University, Fuzhou, China
- 5Fujian Provincial Key Laboratory of Soil Environmental Health and Regulation, College of Resources and Environment, Fujian Agriculture and Forestry University, Fuzhou, China
The complex composition and interaction of root-associated microbes are critical to plant health and performance. In this study, we presented a detailed characterization of three rhizocompartment (rhizosphere, rhizoplane, and root) microbiomes of Achyranthes bidentata under different years of consecutive monoculture by deep sequencing in order to determine keystone microorganisms via co-occurrence network analysis. The network analysis showed that multiple consecutive monoculture (MCM, represented 5Y and 10Y) soils generated some distinct beneficial bacterial taxa such as Bacillus, Fictibacillus, Bradyrhizobium, Shinella, and Herbaspirillum. For fungi, Mortierella substituted for Fusarium in occupying an important position in different rhizocompartments under A. bidentate monoculture. Quantitative PCR analysis confirmed a significant increase in Bacillus, Pseudomonas, and Burkholderia spp. The results of the inoculation assay showed that addition of beneficial bacteria Bacillus subtilis 74 and Bacillus halodurans 75 significantly increased the root length and fresh weight of A. bidentata. Furthermore, three types of phytosterones, as the main allochemicals, were identified both in the rhizosphere soil and in culture medium under sterile conditions by LC-MS/MS. When looking at in vitro interactions, it was found that phytosterones displayed a positive interaction with dominant beneficial species (Bacillus amyloliquefaciens 4 and B. halodurans 75) and had a negative effect on the presence of the pathogenic fungi Fusarium solani and Fusarium oxysporum. Overall, this study demonstrated that consecutive monoculture of A. bidentata can alter the bacterial and fungal community by secreting root exudates, leading to recruitment of beneficial microbes and replacement of plant-specific pathogenic fungi with plant beneficial fungi.
Introduction
Chinese medicine resources are a key point for human disease treatment. Rehmannia glutinosa, Radix pseudostellariae, and Achyranthes bidentata are perennial herbaceous plants that are highly valued in traditional Chinese medicine with a surging market demand (Li et al., 2015; Sun et al., 2015; Wu et al., 2017). However, many medicinal plant species, such as R. glutinosa and R. pseudostellariae, are grown under a continuous cropping system in a geo-authentic production zone because the synthesis of bioactive constituents of certain Chinese medicinal plants is closely correlated to ecological factors (Zhang et al., 2010). In general, this system can have a detrimental effect on soil quality leading to a significant decline in plant biomass and vigor as well as increasing occurrence of plant pests (Wu et al., 2015, 2019). Such “replanting disease” have seriously affected the sustainable development of medicinal plant production (Qian et al., 2016). In contrast, A. bidentata (family Amaranthaceae) is very suitable for consecutive monoculture (Hao et al., 2008; Chen et al., 2015; Wang et al., 2019). Interestingly, the plant biomass and the main active ingredients (includes phytosterone and saponin) in monoculture plots were higher than those in newly planted plots (Li et al., 2010). There is an urgent need to fully understand the complex reasons why different crops respond differently to continuous cropping in the managed farming system.
Numerous studies have suggested that below-ground microbes play crucial roles in soil quality and plant health (Macdonald and Singh, 2013; Lakshmanan et al., 2014). Wu et al. (2015) found that R. glutinosa monoculture led to a serious decline in tuberous root biomass, which was associated with a significant increase in the amount of Fusarium oxysporum, and a decrease in beneficial Pseudomonas spp. in the rhizosphere. In addition, previous studies demonstrated that the occurrence of soil-borne diseases was responsible for either a decrease in soil microbial diversity or an increase in population size of antagonistic bacteria (Latz et al., 2012; Shen et al., 2014). However, our research (Wang et al., 2019) found that in rhizosphere soil, beneficial groups conferring “biocontrol” effects on plant growth increased with increasing years of A. bidentata planting. Therefore, the structure of below-ground microbial community was shown to be a determining constraint to consecutive monoculture.
The environment including the soil and plant root can be divided into three compartments: endosphere (ER, inside the root), rhizoplane (RP, the soil on the surface of the root), and rhizosphere (RS, the soil around the root) (Edwards et al., 2015), which are considered as ecological niches colonized by different microorganisms that play different roles in the soil ecosystem. It was noted that the established endosphere microbiome contributes significantly to several functional characteristics in plants including immune system modulation, quorum sensing, and type V and VI protein secretion systems (Barbara et al., 2015). Moreover, the rhizoplane was shown to play a critical gating role for controlling microbial entry into the host tissue (Ofek-Lalzar et al., 2013). It has been documented that more intimate microbe–host interactions occurred and a more specialized community was further enriched on the rhizoplane than the rhizosphere (Zhang et al., 2017). Studies have indicated that the microbial community in the rhizosphere contained up to 1011 microbial cells per gram of root (Egamberdieva et al., 2007) and more than 30,000 prokaryotic species (Mendes et al., 2011), and these were referred to as “the second genome” of the plant (Berendsen et al., 2012; Rout and Southworth, 2013). The narrow zone rhizosphere was also referred to as a fierce battleground for microorganisms competing for plant-derived nutrients (Martínez-Viveros et al., 2010). Previous studies have revealed that plant species and genotypes alongside environmental and edaphic factors determine the distribution and diversity of the three root-associated microbiota (Van der Heijden and Schlaeppi, 2015; Poudel et al., 2019). Among them, root exudates are vital for the interactions between roots and microbes. It has been established that plants recruit plant-beneficial microbes by secretion of the exudates from their roots in response to an attack. For example, root exudates of Arabidopsis were shown to enhance colonization by a growth-promoting bacterium (Bacillus cereus) with subsequent chitinase production; consequently, the PGPR B. cereus was able to significantly increase biomass and induce systemic resistance in A. thaliana (Zhou et al., 2015). However, there is limited information on the microbial community changes of three root-associated compartments (endosphere, rhizoplane and rhizosphere) in A. bidentata under monoculture and the ecological effects of root exudates.
Recently, with the development of high-throughput sequencing and bioinformatic analysis, studies on the co-occurrence network analysis have provided new insights into the bacterial and fungal composition, distribution, and microbial interaction patterns in environments ranging from soil (Berendsen et al., 2012; Ma et al., 2016) to water (Hu et al., 2017; Mikhailov et al., 2019) and even to human gut (Li et al., 2019). Unlike traditional analytical approaches, network analysis-based approaches, as measured by simultaneous changes in pairwise taxa abundance, can disentangle the structure and assembly of the microbial community, map the pathways of potential interactions between microorganisms, and offer meaningful information beyond sample-level comparison (Cardinale et al., 2015, 2020; Jiang et al., 2017). Based on this analysis, researchers were also able to identify the keystone microbial groups that have the largest influence on community structure and possible function regardless of their population size (Lupatini et al., 2014; Araya et al., 2020). Moreover, the analysis can yield a holistic view of linkages between microbial groups and environmental variables (Banerjee et al., 2018; Fan et al., 2018).
This study aimed to analyze the fungal and bacterial communities associated with the rhizosphere, rhizoplane, and endosphere of A. bidentata using high-throughput sequencing. We hypothesized that the microbial community composition and network structure would differ from each other in the three rhizocompartments, thereby exhibiting their different eco-niche functions in soil ecosystem due to distinct microenvironment filtering, and response to consecutive monoculture. Furthermore, we aimed to isolate key microorganisms so that they could be applied to verify the relationship with root exudates. Therefore, this study will not only enhance the understanding in ecological interactions of plant associated bacterial and fungal communities, but is intended to lay the foundation for further exploitation and application of this core community for sustainable agriculture.
Materials and Methods
Field Sites
The experiment was performed at a long-term orientation station of Fujian Agriculture and Forest University (FAFU) located in Xitao town (34.99 N, 113.26 E), Wuzhi, Henan Province, China. The test plant material was A. bidentata cultivar “Hetaowen,” which is common in the main production region. A. bidentata was planted in the last 10 days of June and harvested in the middle of November in the same year. After harvest, fields were cultivated with wheat from December to June of the following year. The six soil treatments were as follows: (i) newly planted (1Y), (ii) 2-year consecutive monoculture (2Y), (iii) 3-year consecutive monoculture (3Y), (iv) 5-year consecutive monoculture (5Y), (v) 10-year consecutive monoculture (10Y), and (vi) control with no A. bidentata plant (CK).
Sample Preparation
The tuberous roots (at about 40 cm deep) were harvested at the most productive phase of plant development (on November 5, 2017) to evaluate the microbial communities. Samples from each treatment were collected by a five-point sampling method. Briefly, five plots (2 m ∗ 2 m) were selected randomly in each treatment. From each plot, three plants were dug out from the soil and then proceeded by shaking off the bulk soil from the root. The three root-associated compartment samples were collected by the method described by Edwards et al. (2015). Briefly, approximately 1 mm of rhizosphere (RS) soil attached to the roots was collected. The rhizoplane (RP) soil was then obtained after sonicating the root for 30 s at 50–60 Hz in a sterile container with sufficient sterile phosphate buffered saline (PBS) solution. Then, the roots were used to extract the root (RT) microbiome. Root microbiome represented the microorganisms attached to and inside the root, which included the endosphere microbiome. The samples obtained from each treatment were mixed and then divided into three parts for analysis.
Each rhizocompartment sample was then stored at −80°C until DNA extraction. The physicochemical characteristics of air-dried RS soil was assayed in three technical replicates as described by Wu et al. (2013). Briefly, the total N, P, and K (TN, TP, and TK) was calculated by first digesting the soil using the H2SO4–H2O2. Then, TN was measured using the Kjeldahl method. TP and Available P (AP, extracted with NaHCO3 solution) were examined by a colorimetric method. TK and Available K (AK, extracted in ammonium acetate) were determined by flame atomic absorption spectrometry. Available N (AN) was calculated by converting available nitrogen into ammonia. Soil pH was measured by using a pH meter in a 1:2.5 soil/water suspension.
DNA Extraction, PCR Purification, and High-Throughput Sequencing
Total DNA were extracted from 0.7 g of soil samples and 0.5 g of roots using BioFast Soil Genomic DNA Extraction kit (BioFlux, Hangzhou, China) according to the manufacturer’s instructions. DNA concentration was detected by using Nanodrop 2000C Spectrophotometer (Thermo Fisher Scientific, United States). For the bacterial community, the V4 amplification of the 16S rRNA gene in RS and RP samples was carried out with the specific primers 515F (5′-GTGCCAGCMGCCGCGGTAA-3′) and 806R (5′-GGACTACHVGGGTWTCTAAT-3′) (Panke-Buisse et al., 2014). Variable regions 5 to 7 (V5–V7) in RT samples were selected and amplified with the specific primers 799F (5′-AACMGGATTAGATACCCKG-3′) and 1193R (5′-ACGTCATCCCCACCTTCC-3′) with the barcode (Beckers et al., 2016). For the fungal community, the primer set ITS5-1737F (5′-GGAAGTAAAAGTCGTAACAAGG-3′)/ITS2-2053R (5′-GCTGCGTTCTTCATCGATGC-3′) for RS and RP samples (Lu et al., 2013) and the primer set ITS1-1F-F (5′-CTTGGTCATTTAGAGGAAGTAA-3′)/ITS2-2053R (5′-GCTGCGTTCTTCATCGATGC-3′) for RT samples (Xiong et al., 2016) were chosen to target the fungal ITS1 region. The primer pairs selected in RT samples can effectively filter mitochondrial DNA or plastid (chloroplast) DNA, thereby improving the accuracy of the results. The PCR reactions were carried out by Novogene Co., Ltd. (Beijing, China) using Phusion® High-Fidelity PCR Master Mix with GC Buffer (New England Biolabs, Ipswich, United States). The PCR products were purified with Qiagen Gel Extraction Kit (Qiagen, Germany). The library was then sequenced on an Illumina HiSeq2500 platform. The sequences were submitted to the NCBI database under the accession number PRJNA606926.
Sequence Data and Co-occurrence Network Analyses
Raw sequences were processed using the Quantitative Insights Into Microbial Ecology (QIIME) toolkit (V1.9.11) (Caporaso et al., 2010) and UPARSE pipeline (Uparse v7.0.10012) (Edgar, 2013). Then Usearch software was used to perform the operations of data filtering chimeras and clustering to generate operational taxonomic units (OTUs) at the 97% similarity level. Species annotation analysis was performed (set threshold value 0.8–1) using Mothur method and the SSUrRNA database of SILVA (v1323) to obtain taxonomic information and each classification level (Wang et al., 2007; Quast et al., 2012). The co-occurrence network analysis was implemented to demonstrate the relationship of different species among several samples and find out the keystone species (Li and Wu, 2018). The co-occurrence patterns in this study were performed using the 50 most abundant genera of bacterial and fungal communities based on strong (r > 0.6) and significant Spearman correlations (p < 0.01) in R software. The R scripts are found at https://github.com/RichieJu520/Co-occurrence_Network_Analysis. Moreover, the networks visualization, topological properties, and modular analysis were performed in Gephi. Heatmap was used to perform the correlations between soil physical–chemical properties and the keystone species in the network.
Quantitative PCR in situ for Dominant Genera in the Consecutive Monoculture Rhizosphere Soil
A quantitative PCR in situ was used to determine the variation tendency of total bacteria (Eub338/Eub518), total fungi (ITS1F/ITS4), and dominant genera and species including Pseudomonas spp. (Ps-for/Ps-rev), Burkholderia spp. (Burk3/BurkR), Bacillus spp. (BacF/1378), and F. oxysporum (ITS1F/AFP308R) using the CFX96 Real-Time system (Bio-RDA, United States) in rhizosphere soil (Lievens et al., 2005; Drigo et al., 2009). The mixture volume of PCR reaction was 15 μl containing 7.5 μl of 2 × TransStart Tip Green qPCR Supermix (TransGen, Beijing, China), 0.6 μl of each primer (10 μmol L–1), 5.3 μl of RNase-free H2O, and 1 μl of template DNA (20 ng μl–1 of total soil DNA or a serial dilution of plasmid DNA for standard curves). Four independent quantitative PCR assays were performed for each treatment.
Identification of the Main Substance Phytosterones in Culture Medium and Rhizosphere Soil and Its Interaction With Specific Bacterium in vitro
LC-MS/MS Analysis of Phytosterones in Different Rhizosphere Soil
Tissue cultures of A. bidentata were incubated for 44, 140, 248, 294, 320, 373, and 396 days. After removing the plants root, 90 ml of culture medium was collected and extracted with 50% aqueous methanol and dissolved in 2 ml of methanol. Soil phytosterones of each sample were extracted with 70% aqueous methanol and dissolved in 4 ml of methanol. The extraction procedure of phytosterones and detection using LC-MS/MS was previously described by Wang et al. (2019).
Screening and Functional Verification of the Specific Beneficial Bacteria
Bacillus spp. in soil were isolated on nutrient agar (NA) media following the methods of Ahmad et al. (2008). A total of 200 isolates were selected for further in vitro antagonism assays. Monitoring the antagonistic activity of single colonies of bacteria against F. oxysporum and F. solani were recorded by the method described by Wu et al. (2015). Furthermore, the 16S rRNA gene of Bacillus isolates were amplified by universal primers 27F/1522R and the PCR products were subsequently visualized on an agarose gel and sequenced by Shanghai Biosune Co. Ltd (Shanghai, China). The results were compared to the sequences on NCBI using the BlastN. The sequences were submitted to the NCBI database under accession number SUB9495955.
The pots (13 cm bottom diameter, 18 cm top diameter, and 24 cm height) containing soil were sown with the seeds of A. bidentata and placed in a greenhouse on March 5, 2018. The isolated bacteria Bacillus amyloliquefaciens 4, Bacillus subtilis 35, B. subtilis 74, and Bacillus halodurans 75 were grown in LB medium at 37°C and 200 rpm, respectively. When the OD600 value reached 0.8, 10 ml of bacterial fermented broth was centrifuged and washed with double-distilled water twice after removing the supernatant. The cells with equal volume of double-distilled water were added into the soil at the four-leaf stage of A. bidentata; meanwhile, the same volume of double-distilled water was added to the control. The treatment was repeated four times on April 7, May 7, May 20, and June 7, 2018, respectively. Each treatment had three replicates. We harvested the plants on July 15, and immediately measured plant height, root length, and fresh weight.
In vitro Interaction Between the Beneficial Bacterium and Specific Phytosterones
Based on the measurement results of phytosterones in the A. bidentata rhizosphere soil, a concentration gradient series of single phytosterone and mixed phytosterone (β-ecdysterone:25R-inokosterone:25S-inokosterone = 20:2:3) were prepared at 0, 0.01, 0.05, 0.1, 0.5, 1, and 2 μg/ml. The isolated bacterial BA 4, BS 35, BS 74, and BH 75 strains were cultured by adding the single or mixed phytosterone to LB medium with eightfold dilution. After 8 h of incubation at 30°C and shaking at 200 rpm, the optical density (OD) values were determined at 600 nm using a microplate reader (Thermo Scientific Multiskan MK3, Shanghai, China). The isolated F. oxysporum or F. solani was inoculated onto the center of 9-cm-diameter Petri dishes filled with an eightfold dilution of potato–sugar–agar (PSA) medium containing different final concentrations of single or mixtures of phytosterone (0, 0.01, 0.05, 0.1, 0.5, 1, and 2 μg/ml). After 7 days of incubation at 30°C, colony diameter was measured.
Statistical Analyses
Alpha diversity analyses including Observed-OTUs, Shannon index, Simpson index, Chao1, and ACE were conducted according to the normalized data by QIIME (V1.9.1) (Caporaso et al., 2010). Venn diagrams of the exclusive and shared OTUs between different samples were displayed with R software (version 3.0.3). Analysis of variance (ANOVA) was conducted for significance of difference by Tukey’s test (p < 0.05) using DPS 7.05 software.
Results
The Morphology and Biomass of A. bidentata Under Consecutive Monoculture
The observation of morphology and measurement of yield showed plants displayed well with less adventitious fibrous roots and more below-ground biomass when A. bidentata were harvested from consecutive monocultured soil in the period of tuber enlargement. Additionally, compared with results from a newly planted plot (1Y), the dry weight of A. bidentata tuberous roots displayed a 2.19 times increase in 10 years monoculture plot (10Y) (Supplementary Figure 1).
Assessment of Physicochemical Properties in the Rhizosphere Soil
Physicochemical properties of rhizosphere soil samples were summarized in Supplementary Table 1. The results revealed that compared to the less continuously planted plot (1Y and 3Y), the contents of total phosphorus (TP) and available nitrogen (AN) in rhizospheric soils were significantly (p < 0.05) higher in the multiple continuous monoculture soil systems (5Y and 10Y). Meanwhile, the pH value does not decrease with increasing years of monoculture. However, the newly planted soil had a higher total potassium (TK) than the monoculture soils. It was found that the total nitrogen (TN) displayed a slightly increasing trend in the monoculture system even if there was no significant difference.
Diversity Indices of the Soil Microbial Community
The results generated 885,359, 888,537, and 611,754 high-quality effective tags of each root-associated layer with a length of 253 bp (RS, RP) and 376 bp (RT), and tags were clustered to 45,959, 52,378, and 7192 bacterial OTUs, respectively. With respect to fungi, a total of 650,006, 865,696, and 850,159 high-quality ITS1 effective tags were obtained with a length of 260 bp (RS, RP) and 250 bp (RT). Subsequently, at a 97% sequence similarity cutoff, the tags were clustered to 8938, 6166, and 3553 fungal OTUs across the 12 samples. The alpha-diversity indices of bacterial and fungal communities were observed including Shannon index, Simpson index, Chao1, and ACE (Supplementary Tables 2, 3). It can be seen that the Chao1 and ACE index of bacteria and fungi displayed a trend in the order rhizosphere > rhizoplane > root. Further, it was found that the Simpson, Chao1, and ACE indices of bacterial and fungal communities were not significantly different to different continuous cropping years in the rhizosphere and rhizoplane. However, the number of OTUs and ACE indices of the bacterial community increased with increasing years of monoculture in root. The reverse was true with respect to fungal community (Supplementary Table 3).
Venn Diagram Analysis
The exclusive and shared OTUs between different samples were presented by Venn diagrams (Supplementary Figures 2, 3). Interestingly, for bacterial communities, the number of OTUs shared in 5Y and 10Y samples was 519, 214, and 73 from rhizosphere to root and higher than those shared in 1Y and 3Y samples (285, 190, and 22, respectively). It can be seen from the pie chart structure that the microorganisms shared by 5Y and 10Y are completely different from those between 1Y and 3Y samples. Moreover, the OUTs shared in 5Y and 10Y in the rhizosphere layer (Supplementary Figure 2a) were mainly assigned to the class Proteobacteria (28.5%), Planctomycetes (16.6%), and Bacteroidetes (13.1%), while in root (Supplementary Figure 2c), the Proteobacteria (58.9%) accounted for more than half of the total OTUs. For fungal communities (Supplementary Figure 3), the number of shared OTUs in 5Y and 10Y samples was less than those in 1Y and 3Y samples, which was opposite the trend displayed in bacteria except RS. Accordingly, the number of OTUs shared in 5Y and 10Y samples was 17 and 3 in rhizoplane and root, which are by far lower than those shared in 1Y and 3Y samples (101 and 57). The OTUs shared in 1Y and 3Y classified at the phylum level were similar in these three root-associated layers.
Structure and Composition of Soil Microbial Community
As shown in Figures 1, 2, the proportions of various phyla and genus were different across the compartments. The bacterial OTUs in RP were predominantly associated with five phyla, Proteobacteria, Firmicutes, Actinobacteria, Acidobacteria, and Gemmatimonadetes, and accounted for 80.8%, 80.9%, 77.7%, and 86.8% of the total population in the 1Y, 3Y, 5Y, and 10Y sample, respectively. Proteobacteria was the dominant microbial taxon in RT samples (Figures 1B,C). It is worth mentioning that each of the compartments was found to harbor a distinct microbiome, and the most relative abundance Bacillus (RP) by the phyla Firmicutes and Pseudomonas (RT) belong to Proteobacteria (more than 50% in most samples) increased with the increasing years of monoculture (Figures 2A,B). Fungal OTUs were comprised mainly of the phyla Ascomycota, Basidiomycota, and Zygomycota, and these three phyla accounted for exceeding 70% in each sample of each layer. Other minor phyla including Chytridiomycota, Rozellomycota, and Glomeromycota were mostly found in the RS compartments (Figures 1D–F). Variation in community composition among the three plant compartments was also observed at the genus level (Figures 2D–F). The three most abundant genera (Mortierella, Microidium, and Cladosporium) showed different patterns of relative abundance. Further, the trends of Mortierella (Mucoromycota) were similar in each plant compartment, and the proportion in 10Y was higher than those in the other soil samples (1Y, 3Y, and 10Y). Although the content of Fusarium (Ascomycota) was increased with increasing years of monoculture in RS and RP, the proportion was lower in 10Y compared to 1Y in RT.
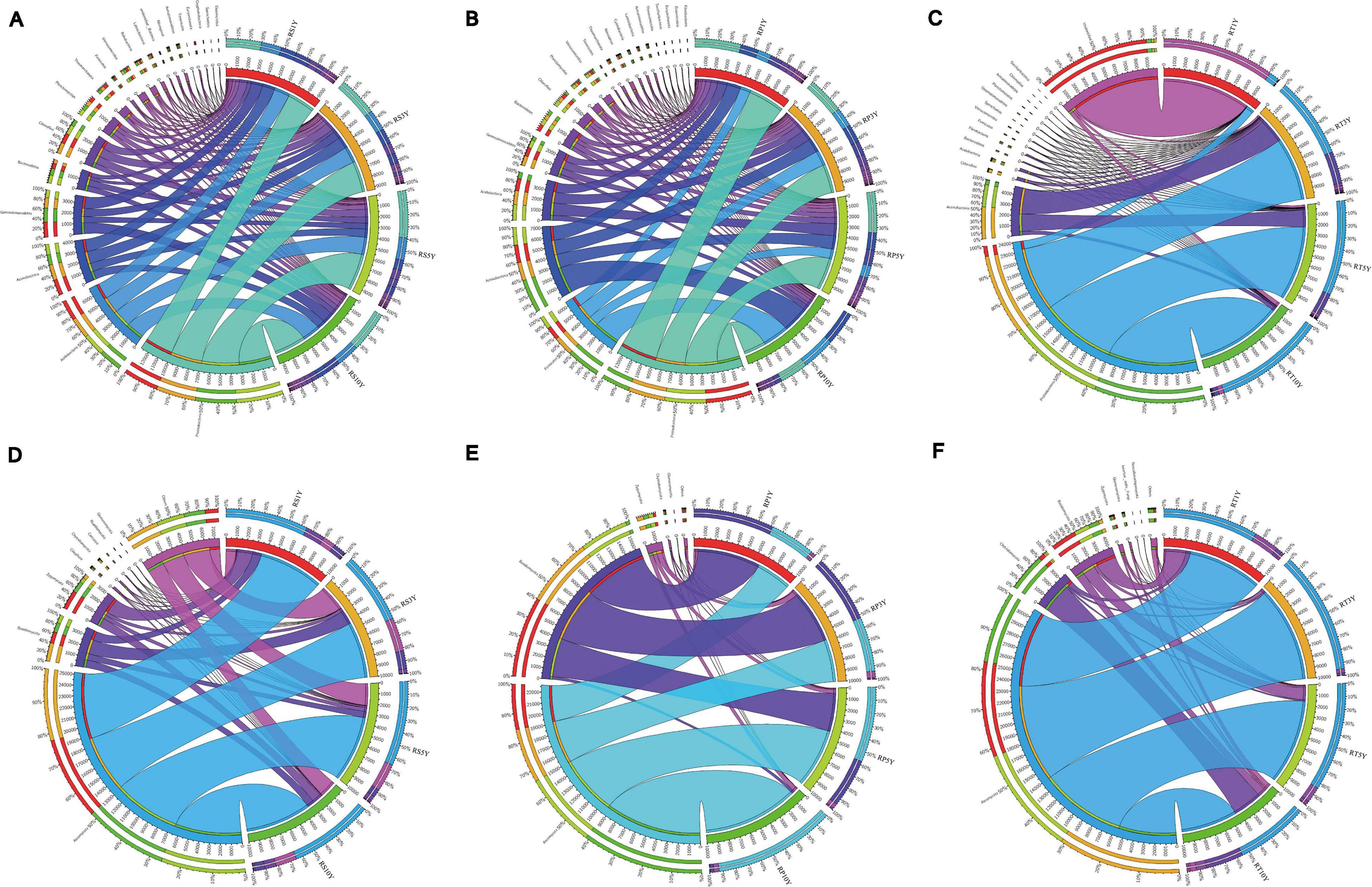
Figure 1. Distribution of dominant (top 20) phyla in four different samples in three root-associated compartments. [(A–C) represented the bacterial community in rhizosphere, rhizoplane, and root, respectively; (D–F) represented the fungal community in rhizosphere, rhizoplane, and endosphere, respectively]. The thickness of each ribbon represents the abundance of each taxon. The relative tick above the outer segment stands for the relative abundance of each taxon. The data were visualized using Circos (Version 0.69, http://circos.ca/).
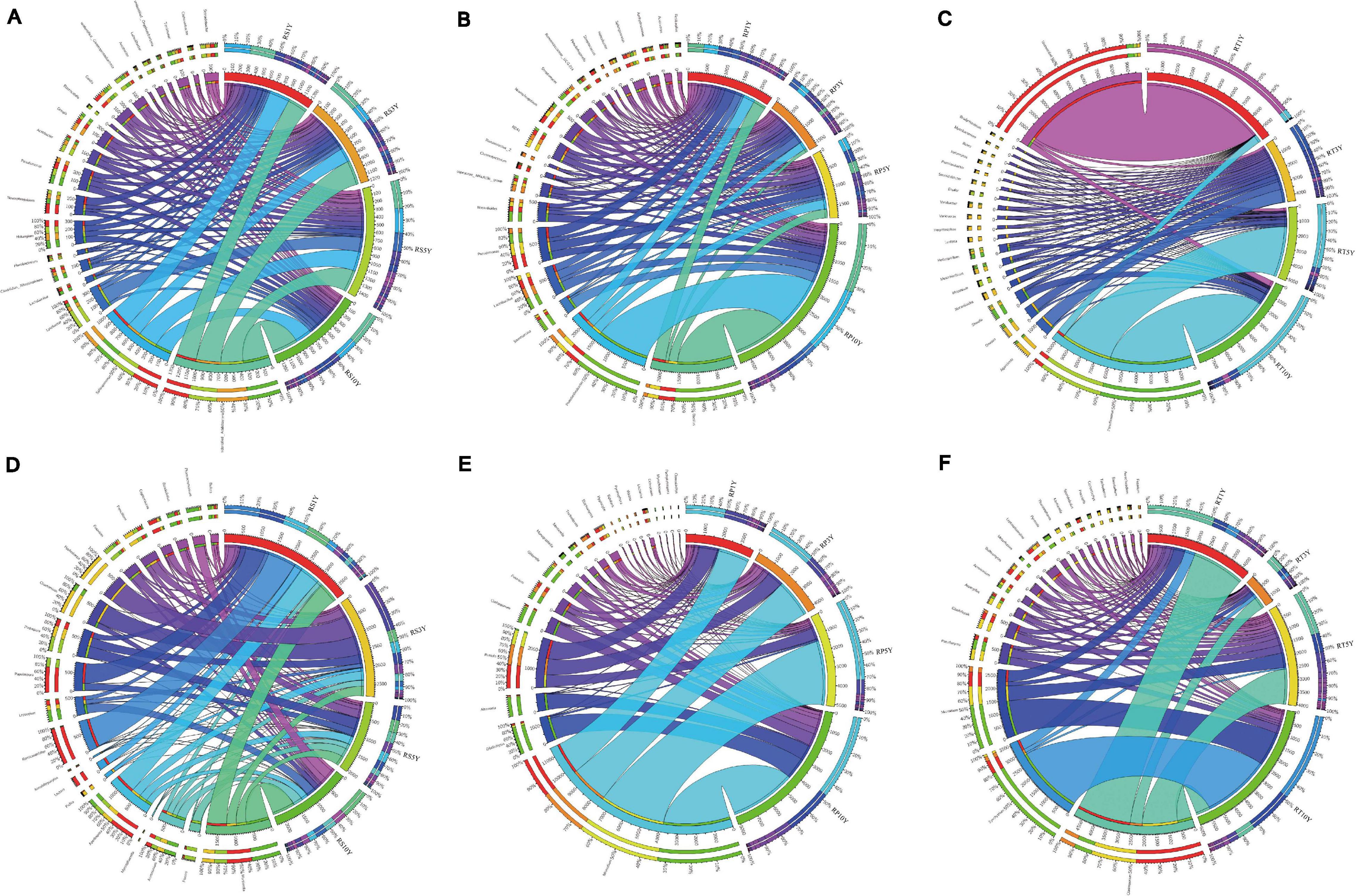
Figure 2. Distribution of dominant (top 20) genera in four different samples in three root-associated compartments. [(A–C) represent the bacterial community in rhizosphere, rhizoplane, and root, respectively; (D–F) represented the fungal community in rhizosphere, rhizoplane, and root, respectively]. The thickness of each ribbon represents the abundance of each taxon. The relative tick above the outer segment stands for the relative abundance of each taxon. The data were visualized using Circos (Version 0.69, http://circos.ca/).
Co-occurrence Network Analysis
In this study, 12 networks were constructed to illustrate differences in soil bacterial and fungal communities between less continuous planted (LCM) plot systems (1Y and 3Y) and multiple continuous monoculture (MCM) plot systems (5Y and 10Y) in three compartments (Figures 3, 4). The network properties of soil bacterial and fungal communities are summarized in Supplementary Table 4. In the bacterial networks, LCM soils and MCM soils resulted in networks with similar sizes in RS (19 and 19 nodes, 31 and 36 edges, respectively; Figure 3 and Supplementary Table 4). MCM soil [1 (RP) and 3.157 (RT)] had a lower value of average path length (path length is the shortest path between two nodes) than the LCM soils [2.502 (RP) and 4.658 (RT)]. The higher modularity value indicates the denser connections between the nodes within the module. The results showed that in both RS and RP rhizocompartment, the modularity value of MCM soil (0.773 and 0.917, respectively) was higher than the value in LCM soil (0.765 and 0.533, respectively). The RS and RT were mainly composed of Proteobacteria, while the RP was mainly Proteobacteria and Firmicute (Figure 3). The keystone bacterial genera in these systems are listed in Table 1. It is worth mentioning that unidentified Nitrosomonadaceae, Bacillus, Fictibacillus, Lysinibacillu, Bradyrhizobium, Shinella, and Herbaspirillum occupied an important position in MCM soil.
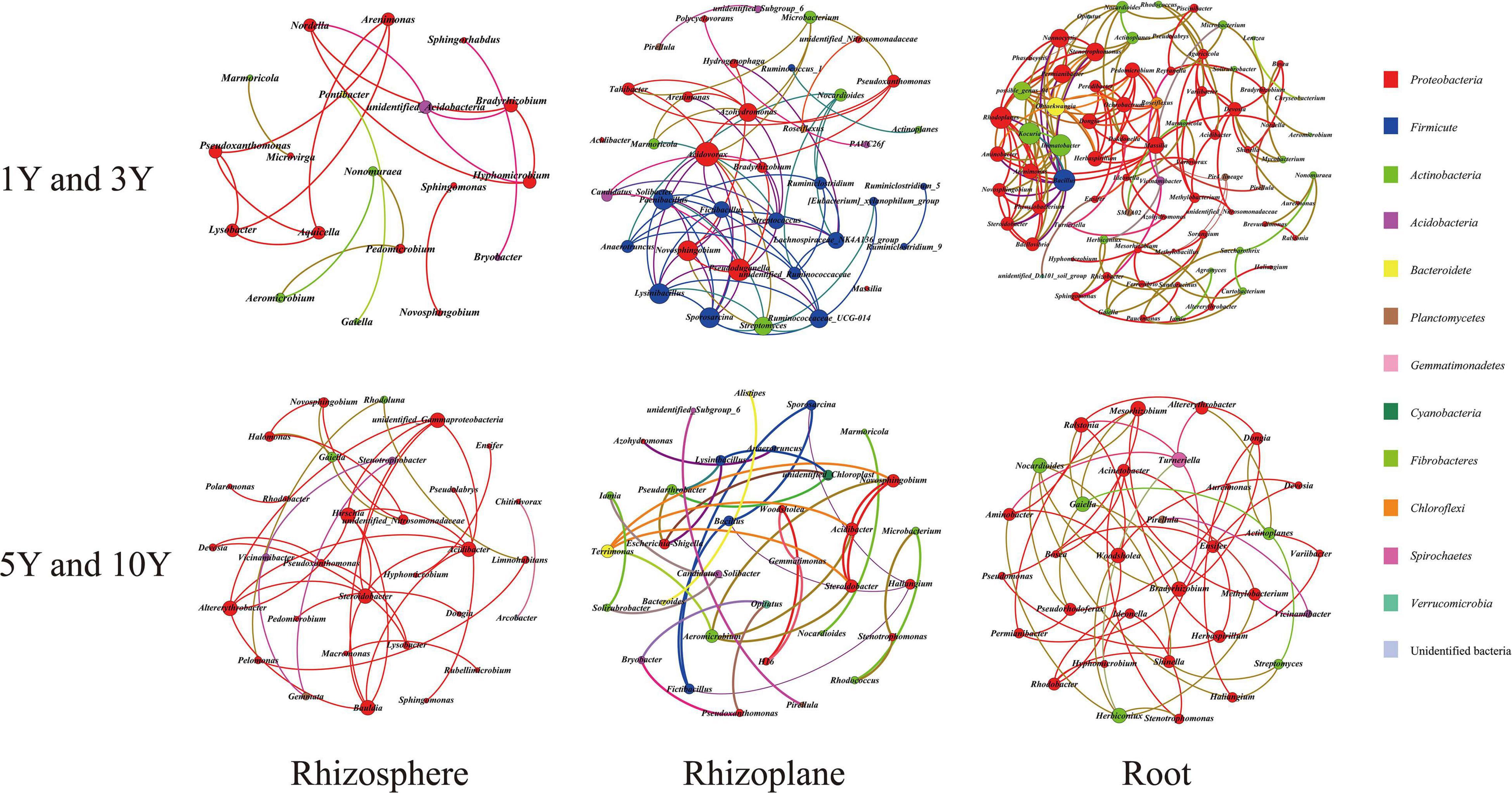
Figure 3. The co-occurrence network of bacterial genera based on correlation analysis for different samples in three root-associated compartments. A connection stands for a strong (Spearman’s r > 0.6) and significant (p < 0.01) correlation. The size of each node is proportional to the degree. Nodes colored by taxonomy.
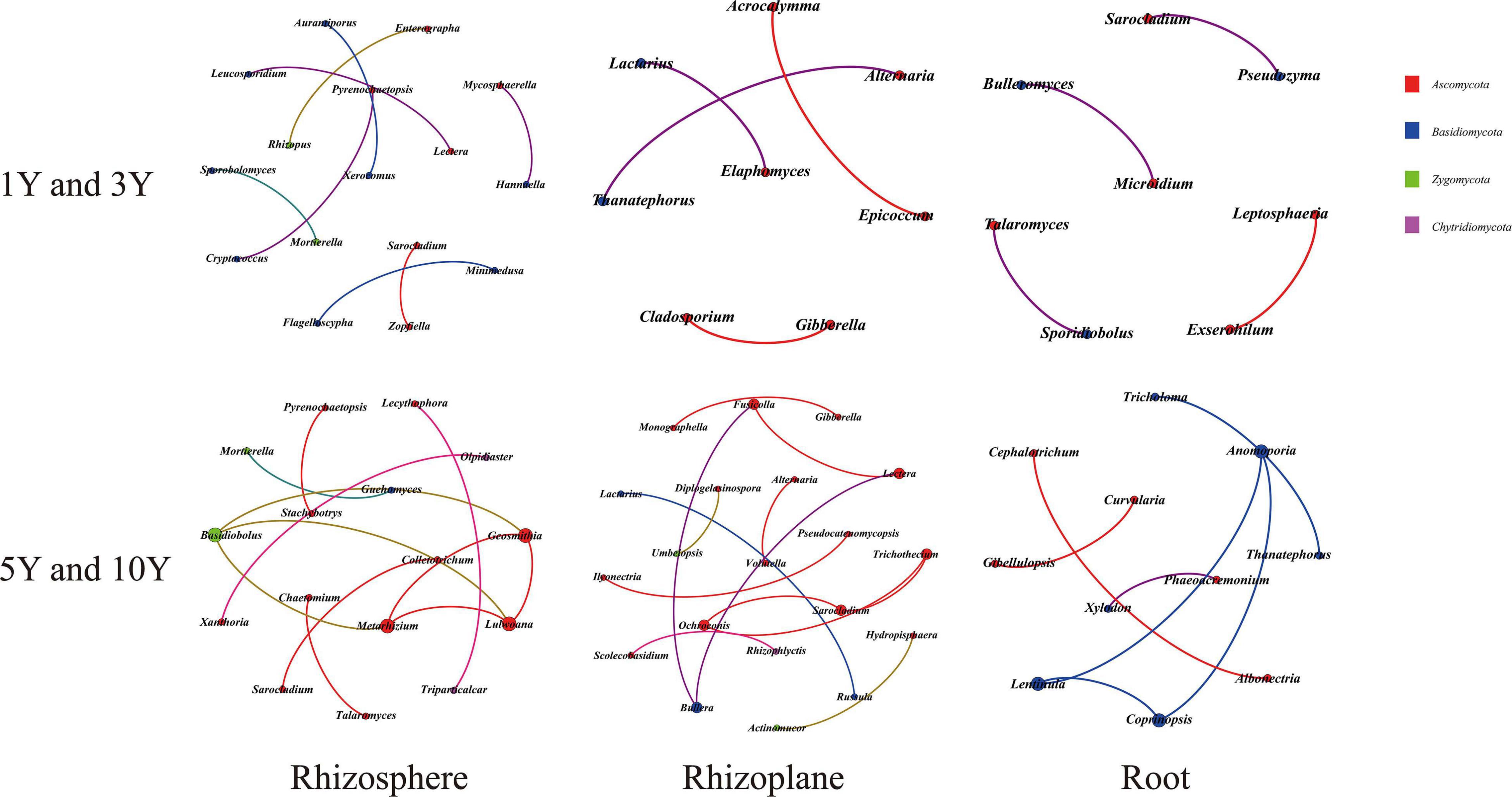
Figure 4. The co-occurrence network of fungal genera based on correlation analysis for different samples in three root-associated compartments. A connection stands for a strong (Spearman’s r > 0.6) and significant (p < 0.01) correlation. The size of each node is proportional to the degree. Nodes colored by taxonomy.
With respect to fungal networks, the nodes and edges of MCM soils were 16 and 12 (RS), 20 and 13 (RP), and 11 and 7 (RT) higher than that 16 and 8 (RS), 8 and 4 (RP), and 8 and 4 (RT) in LCM soil, respectively, indicating that MCM soils had more connections compared to LCM soil. The values of network modularity presented an opposite trend in which the values [0.875 (RS) and 0.75 (RT)] in LCM soil were higher than those in MCM soils [0.708 (RS) and 0.735 (RT)] (Supplementary Table 4). The results from Figure 4 showed that most genera (more than 80%) in three compartments of LCM soil belonged to Ascomycota and Basidiomycota. While all genera were assigned to four phyla and various phyla accounted for a different proportion in MCM soils. Mortierella species had the highest level of abundance compared to other fungi. Further, these networks were not associated with Fusarium.
The results showed that there were correlations between soil physical–chemical properties and the keystone species. Among them, Pseudoxanthomonas, Altererythrobacter, unidentified Gammaproteobacteria, Pseudolabrys, and Hirschia showed a significant positive correlation with AN, TN, and AP (Supplementary Figure 4). Novosphingobium, Gaiella, Halomonas, and Nordella showed a significant negative correlation with AN, TN, and AP (Supplementary Figure 4). With respect to fungi, Geosmithia, Talaromyces, and Guehomyces showed a significant negative correlation with TK (Supplementary Figure 5). Sporobolomyces and Sarocladium showed a significant positive correlation with TK (Supplementary Figure 5).
Abundance of Specific Microbes by Quantitative PCR
The qPCR analysis showed that the abundance of total bacteria, Bacillus, Pseudomonas, and Burkholderia spp., were significantly greater in the 5Y and 10Y (strong allelopathic activity soils) than in the 1Y and 2Y, while total fungi and F. oxysporum showed the other trend and tended to stay at a stable level with an increase of continuous cropping years (Figure 5). The results were consistent with high-throughput sequencing analysis (Figure 2).

Figure 5. Quantification of total bacteria, total fungi, and specific microbes (including Bacillus, Pseudomonas, Burkholderia, and Fusarium) in different soil samples. Small letters in figures show significant differences determined by Tukey’s test (p ≤ 0.05, n = 3).
Isolation and Functional Verification of Key Microorganisms
Among the cultured organisms, there were significantly higher occurrence of F. solani and F. oxysporum. Tests demonstrated that the tissue culture of A. bidentate under F. oxysporum treatment exhibited wilt disease symptom (Supplementary Figures 6a,b). Then, the antagonistic assay of the purified isolated Bacillus spp. against F. solani and F. oxysporum was evaluated. In Supplementary Figure 6c, the bacteria exhibited a strong antagonism against the two fungi. After molecular identification, the strains were aligned with B. amyloliquefaciens 4 (BA4), B. subtilis 35 (BS35), B. subtilis 74 (BS74), and B. halodurans 75 (BH75). Subsequently, the bacteria were added and inoculated to the pots where the A. bidentata were grown. Both the root length and the fresh weight of A. bidentata under treatment by BS 74 and BH 75 were significantly (p < 0.05) greater than the control (Supplementary Figure 7).
Identification and Analysis of Main Phytosterones in Mediums and Its Interaction With Microbes in vitro
The results showed that phytosterone levels tended to increase as the growth of the tissue culture plant increased. In particular, the content of β-ecdysterone after 373 and 396 days (2.34, 1.84 μg/ml media) tissue culture was 6.3 and 4.9 times higher than after 140 days (0.37 μg/ml), respectively (Supplementary Figure 8a). Although it was found that no phytosterone was detected in 1Y and CK soils, the content of β-ecdysterone did not accumulate in consecutively monocultured soil (Supplementary Figure 8b). This may be the result of the combined effect of root exudation intensity and soil microbial catabolism in monocultured soil.
Further, the effects of phytosterone allelochemicals in root exudates on the growth of beneficial bacteria (BA4, BS35, BS74, and BH75) and pathogenic fungi (F. solani and F. oxysporum) were determined (Supplementary Figures 6, 7, 9). A mixture of phytosterones significantly promoted the growth of beneficial bacteria especially BA74 and BH75 (Figure 6). Among the three phytosterone compounds identified in the soil, β-ecdysterone displayed the most stimulatory effect. What is more, mycelial growth of F. solani was inhibited by a mixture of phytosterones (Figure 7). Likewise, the various single phytosterones displayed different influences on the mycelial growth.

Figure 6. The effects of a mixture of phytosterone on the growth of beneficial bacteria [including Bacillus amyloliquefaciens 4 (BA4), Bacillus subtilis 35 (BS35), Bacillus subtilis 74 (BS74), and Bacillus halodurans 75 (BH75)]. Small letters in figures show significant differences determined by Tukey’s test (p ≤ 0.05, n = 3).
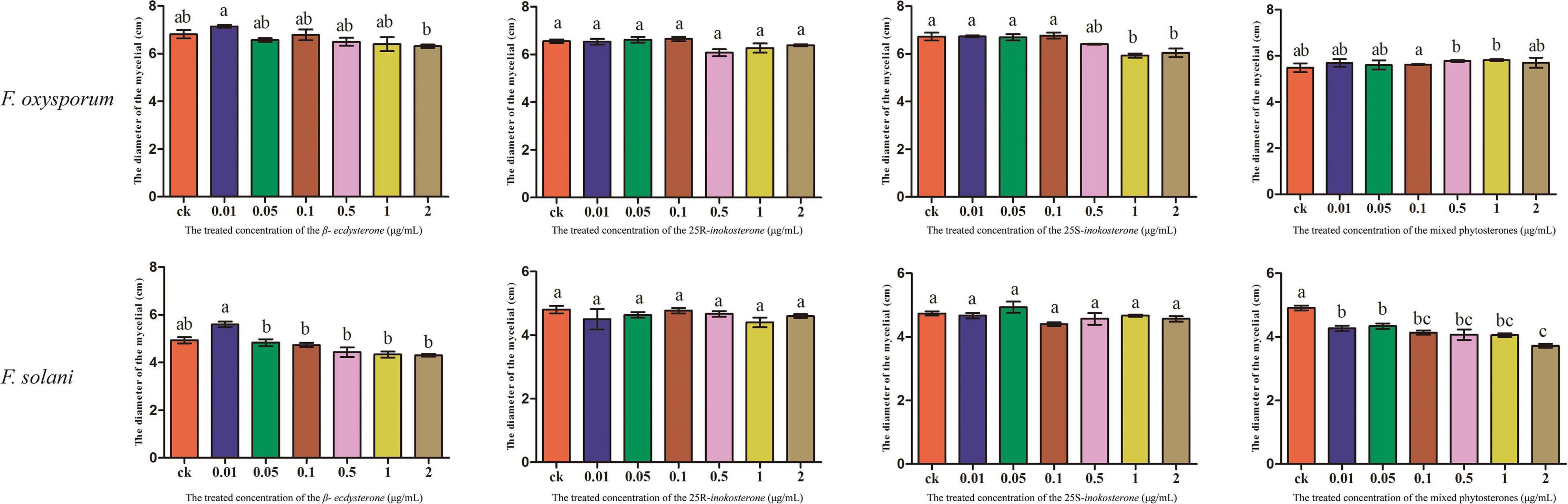
Figure 7. The effects of single (β-ecdysterone, 25R-inokosterone, and 25S-inokosterone) and a mixture of phytosterone on the mycelial growth of F. oxysporum and F. solani. Small letters in figures show significant differences determined by Tukey’s test (p ≤ 0.05, n = 3).
Discussion
It can be clearly seen that both plant and soil environment provided an ideal habitat for some microorganisms, and these species had a great impact on plant productivity (Van der Heijden et al., 2008; Schlaeppi and Bulgarelli, 2015). Among them, the role of the microbiome including bacteria and fungi must be taken into consideration. On the one hand, soil microbes are important positive catalysts of plant productivity (Raaijmakers et al., 2002). Precisely, mechanisms through which microorganisms can enhance plant productivity have been identified and include triggering inducing systemic resistance by priming for accelerated defense-related gene expression (Van Der Ent et al., 2009), fixation of nitrogen from atmospheric N (Unkovich et al., 2008), and solubilization of nutrients that are inaccessible to plant roots (Zahir et al., 2003). On the other hand, soil pathogens grow rapidly to obtain sufficient numbers on their host and be able to infect host tissue resulting in a reduction of plant biomass (Raaijmakers et al., 1995; Beattie, 2006).
The data presented here provide a characterization of the bacterial and fungal community in three root-associated layers of A. bidentata. The structure, composition, and microbial abundance of soil fungal and bacterial communities differed in relation to different consecutive monoculture years. From the viewpoint of diversity indices, it could be shown that the diversity decreases from rhizosphere to rhizoplane and then to root for both bacteria and fungi. The shifts in community structure from rhizosphere toward roots was a result of a three-step selective filter by each of these compartments (Edwards et al., 2015; Beckers et al., 2017). From the viewpoint of structure and composition, the various proportions of certain microbes (such as Sphingomonas, Bacillus, and Pseudomonas) across the three rhizocompartments in our study indicated that microbes were attracted to suitable niche. When rhizoplane microbiome data were taken into consideration, the most dominant genus, Bacillus spp., exhibited higher relative abundance in the MCM soils compared to LCM soils. This selection by the plant may be explained by the fact that this bacterium was known to have the ability to form biofilms that helped to generate antagonistic effects on pathogenic fungi (Chen et al., 2013). With respect to the root, Pseudomonas spp. became the dominant bacterium with the same trend of Bacillus spp. in different continuous cropping samples. The change from rhizoplane to root further clarified that Bacillus spp. when bound to the rhizoplane served as a critical gating role. Moreover, other studies found that Pseudomonas isolates from the endosphere engaged in more metabolic pathways for plant signaling compounds, bacterial–plant interactions, and root growth promotion when compared to isolates obtained from the rhizosphere (Timm et al., 2015). The roles of specific species of Pseudomonas app. need to be discussed in-depth in future research. The results reported here also showed that the relative abundance of Proteobacteria was increased in root compared to rhizosphere soil, which was similar to studies of Arabidopsis and rice (Bulgarelli et al., 2012; Edwards et al., 2015). This suggested that the distribution of different bacterial phyla inside the roots might be similar for many land plants. The plant cell-wall feature provided a cue that this A. bidentata-unspecific root-inhabiting microbiota might play a vital role in the decomposition of organic matter after plant death (Bulgarelli et al., 2012). Therefore, the niche-specific settings among different rhizocompartments can exhibit different eco-niche functions in an ecosystem.
By employing network analysis, new insights were revealed regarding the bacterial and fungal microbiota associated with different consecutive monocultures. Twelve network graphs were constructed to display interactions among different genera. The bacterial community was found to have a greater number of functionally interrelated members (231 nodes and 402 edges) than those in the fungal community (79 nodes and 48 edges). The ecosystem characteristics and processes were determined by interactions among species that are able to modify energy pathways or the abundance of species, not simply the presence of species (Chapin et al., 2000; Faust and Raes, 2012). Our results suggest that bacteria may play a more important role in continuous cropping systems. For the bacterial network, the keystone bacterial species were unidentified: Nitrosomonadaceae, Bacillus, Fictibacillus, Bradyrhizobium, Shinella, and Herbaspirillum. In addition, most of these functional bacteria exhibited different eco-niche functions in the soil ecosystem, which have been shown to be involved in plant growth-promoting activities including production of different plant hormones, soil nutrient bioavailability, and generating antagonistic effects (Han et al., 2014; Li B. et al., 2014). It is worth mentioning that there were many nitrogen-fixing bacteria (including Bradyrhizobium, Shinella, and Herbaspirillum), which are responsible for the nitrogen input into various ecological niches in this system (Perret et al., 2000; Elbeltagy et al., 2001; Lin et al., 2008). For example, Baldani et al. (1986) successfully isolated and verified nitrogen fixation activity of Herbaspirillum from cereal roots. For fungi, Mortierella were the most prevalent genus in this system. Previous studies have shown that some species of Mortierella are antagonistic to pathogens, and several isolates have been determined to be potential antagonistic agents against major potato scab pathogens (Tagawa et al., 2010). However, Fusarium was absent in the network graph, which was the opposite observation to that obtained from previous reports on the monoculture of medicinal plants (R. glutinosa and R. pseudostellariae) and disease-conducive soil (Wu et al., 2015, 2016; Xiong et al., 2017). Previous studies have shown the significant correlations between key environmental factors (such as available nutrients, soil pH, soil texture, and climatic conditions) and the keystone soil microbes (Fierer and Jackson, 2006; Banerjee et al., 2016). The vital participation of soil microbiomes in soil nutrient cycling was also reported previously, especially in nitrogen fixing and phosphate solubilization, which were key steps for promoting plant growth (Lugtenberg and Kamilova, 2009; Banerjee et al., 2018). In this study, our result provided evidence that AN, TP, and AP contents were closely related to bacterial community while TK was correlated with fungal community. The suitable eco-niche differentiation in each compartment and beneficial plant microbiome interactions are avenues to help plant growth and development (Compant et al., 2019). It was therefore suggested that the recruitment of beneficial microorganisms and disappearance of harmful microorganisms may in turn contribute to a higher disease-suppressive ability of the specific medicinal plant A. bidentata to adapt to the consecutive monoculture system. This would be different from most medicinal plants severely suffering from replanting disease in the monoculture system.
In addition, plants along with their root system can provide a unique ecological niche for soil microbiota and transport up to 40% of their photosynthates to the soil in order to feed microorganisms, which are mostly carbon starved in the soil (Bais et al., 2006; Garbeva et al., 2011). The evidence that intercropping systems or rhizosphere soil have a higher microbial population density indicates that plant species are able to affect species-specific shift as well as the composition of microbial community through the production of specific root exudates (Berg et al., 2006; Li L. et al., 2014). In the present study, we found that the phytosterone produced by tissue culture plantlet roots of A. bidentata accumulated in the culture medium. However, phytosterone did not accumulate in the rhizosphere soil over all the years of monoculture. Therefore, we propose that root exudates were involved in biological processes involving microbial metabolism. The experimental results also showed that exogenously added phytosterones could significantly promote the growth of beneficial bacteria especially Bacillus 4 and Bacillus 75 and inhibit mycelial growth of F. solani and F. oxysporum. An increasing number studies have shown that root exudates could select microorganisms in the rhizosphere, which manifested as an increased density of pathogenic F. oxysporum and a low abundance of beneficial bacteria (Zhou and Wu, 2012; Li X. et al., 2014). It should be noted that the positive or negative effects of root-released allelochemicals in our study displayed an opposite trend from previous studies. Furthermore, certain doses of phytoecdysteroid were able to cause abnormal molting, immobility, weakened invasion, impaired development, and death of nematodes on plants, which resulted in protecting spinach from plant-parasitic nematodes (Soriano et al., 2004). Cui et al. (2015) found that a 1% dose of plant ecdysterone could increase the number of probiotic C. coccoides in the intestine, and a 2% dose increased the proportion of Enterobacteriaceae. The ideal population structure with a higher abundance of beneficial microorganisms (Bacillus) but lower abundance of harmful microorganism was influenced by the phytoecdysteroid exudates, which may partially account for the healthy growth of A. bidentata.
In summary (Figure 8), we provide an insight into soil bacterial and fungal community structure in three root-associated layers of A. bidentata and found key microorganisms as being affected by long-term monoculture via network interactions, which included both basic inventory descriptions of species richness and abundance and coexistence and modularity among populations in the microbial community. Our results showed that the MCM soil apparently generated some distinct bacterial taxa such as plant growth-promoting bacteria and nitrogen-fixing bacteria inhabiting the corresponding root-associated layers. However, there were no potential pathogens in the system. Root exudate phytosterones were found to play important roles in the formation of this structure resulting in the presence of more plant-beneficial bacteria and fewer pathogenic fungi, indicating that different plants can shape the microbial community via secreting a variety of substances. These findings provide a clue necessary to open a new avenue for modulating the root microbiome to enhance medicinal plant production and sustainability. The key microorganisms obtained in this study can lay the foundation for the development of microbial fertilizer in the future. At the same time, the results also have important theoretical significance and potential application value to elucidate the underground interaction mechanism of A. bidentata resistant to continuous cropping for solving the “sick soil syndrome” of most medicinal plants. However, we still need to be aware that further experiments are required to elucidate the intrinsic mechanism of interaction between specific microbes and plant root exudates leading to the tolerance to consecutive monoculture of A. bidentata.
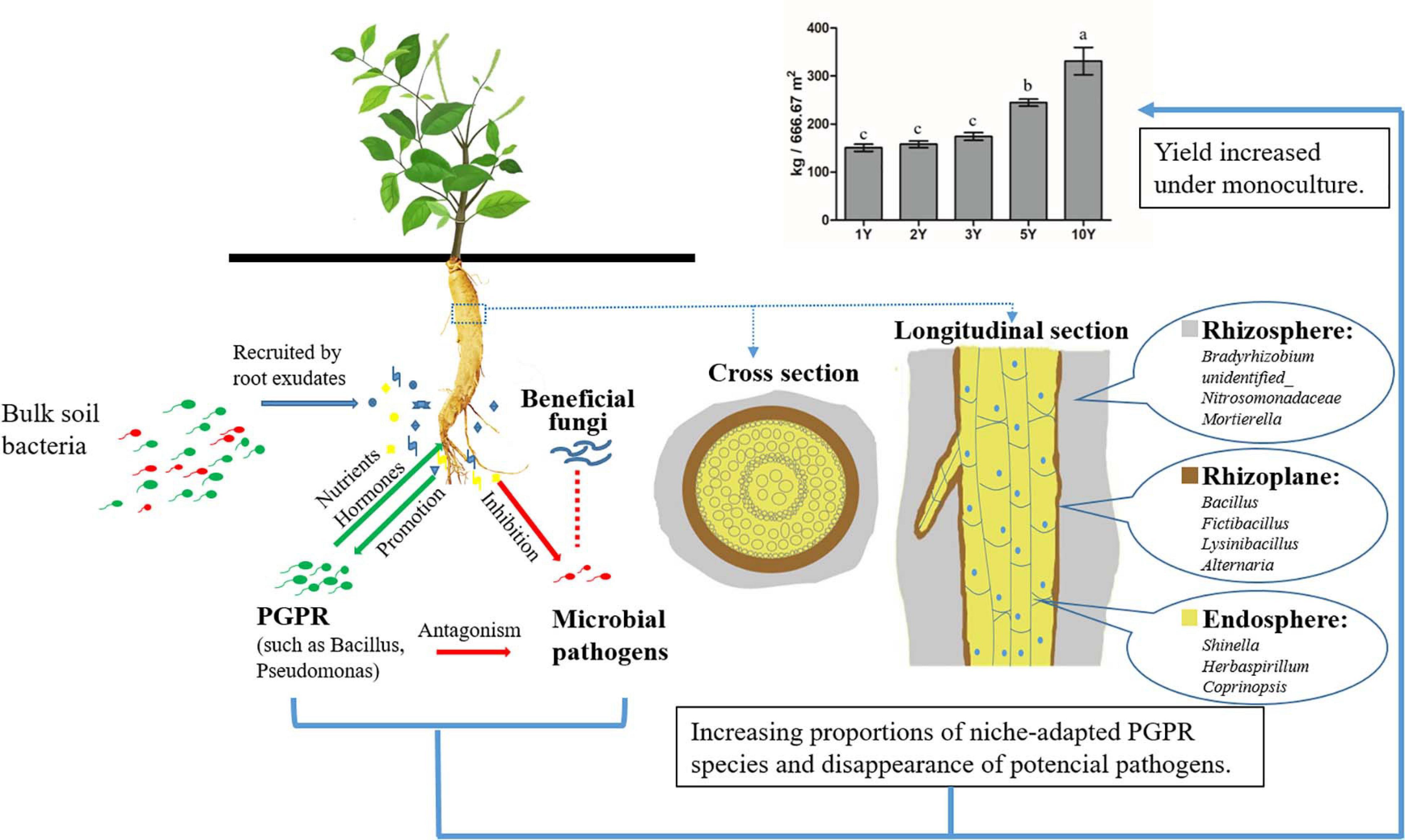
Figure 8. Schematic representation of microbiome interactions and eco-niche distribution in three rhizocompartments of A. bidentata in a consecutive monoculture system. The red dotted line represented that no experiments have been obtained in vitro.
Data Availability Statement
The datasets presented in this study can be found in online repositories. The names of the repository/repositories and accession number(s) can be found below: https://www.ncbi.nlm.nih.gov/, PRJNA606926.
Author Contributions
SL and WL conceived and directed the project. JW, YL, TC, and XQ did all of the experiments. JW, LW, and HW performed the integrated data analysis. JW and WL wrote the manuscript. CR and PL provided linguistic and scientific assistance. All authors contributed to the article and approved the submitted version.
Funding
This work was supported by grants from the National Key Research and Development Plan (2017YFE0121800), the National Natural Science Foundation of China (81973412 and 81573530), Fujian-Taiwan Joint Innovative Center for Germplasm Resources and Cultivation of Crop (FJ 2011 Program, no. 2015-75), and the Research Project of the Education Department of Fujian Province (JAT170200).
Conflict of Interest
The authors declare that the research was conducted in the absence of any commercial or financial relationships that could be construed as a potential conflict of interest.
Supplementary Material
The Supplementary Material for this article can be found online at: https://www.frontiersin.org/articles/10.3389/fmicb.2021.677654/full#supplementary-material
Footnotes
- ^ http://qiime.org/scripts/split_libraries_fastq.html
- ^ http://drive5.com/uparse/
- ^ http://www.arb-silva.de/
References
Ahmad, F., Ahmad, I., and Khan, M. S. (2008). Screening of free-living rhizospheric bacteria for their multiple plant growth promoting activities. Microbiol. Res. 163, 173–181. doi: 10.1016/j.micres.2006.04.001
Araya, J. P., González, M., Cardinale, M., Schnell, S., and Stoll, A. (2020). Microbiome dynamics associated with the Atacama flowering desert. Front. Microbiol. 10:3160. doi: 10.3389/fmicb.2019.03160
Bais, H. P., Weir, T. L., Perry, L. G., Gilroy, S., and Vivanco, J. M. (2006). The role of root exudates in rhizosphere interactions with plants and other organisms. Annu. Rev. Plant Biol. 57, 233–266. doi: 10.1146/annurev.arplant.57.032905.105159
Baldani, J. I., Baldani, V. L. D., Seldin, L., and Dobereiner, J. (1986). Characterization of Herbaspirillum seropedicae gen. nov., sp. nov., a root-associated nitrogen-fixing bacterium. Int. J. Syst. Evol. Microbiol. 36, 86–93. doi: 10.1099/00207713-36-1-86
Banerjee, S., Baah-Acheamfour, M., Carlyle, C. N., Bissett, A., Richardson, A. E., Siddique, T., et al. (2016). Determinants of bacterial communities in Canadian agroforestry systems. Environ. Microbiol. 18, 1805–1816. doi: 10.1111/1462-2920.12986
Banerjee, S., Thrall, P. H., Bissett, A., van der Heijden, M. G. A., and Richardson, A. E. (2018). Linking microbial co-occurrences to soil ecological processes across a woodland-grassland ecotone. Ecol. Evol. 8, 1–14. doi: 10.1002/ece3.4346
Barbara, R. H., Wiebke, B., Claudia, S. B., Mugdha, S., and Thomas, H. (2015). Roots shaping their microbiome: global hotspots for microbial activity. Annu. Rev. Phytopathol. 53, 403–424. doi: 10.1146/annurev-phyto-082712-102342
Beattie, G. A. (2006). “Plant-associated bacteria: Survey, molecular phylogeny, genomics and recent,” in, ed. S. S. Gnanamanickam (Netherlands: Springer), 1–56. doi: 10.1007/978-1-4020-4538-7_1
Beckers, B., Beeck, M. O. D., Thijs, S., Truyens, S., Weyens, N., Boerjan, W., et al. (2016). Performance of 16s rDNA primer pairs in the study of rhizosphere and endosphere bacterial microbiomes in metabarcoding studies. Front. Microbiol. 7:650. doi: 10.3389/fmicb.2016.00650
Beckers, B., Beeck, M. O. D., Weyens, N., Boerjan, W., and Vangronsveld, J. (2017). Structural variability and niche differentiation in the rhizosphere and endosphere bacterial microbiome of field-grown poplar trees. Microbiome 5, 1–17. doi: 10.1186/s40168-017-0241-2
Berendsen, R. L., Pieterse, C. M., and Bakker, P. A. (2012). The rhizosphere microbiome and plant health. Trends Plant Sci. 17, 478–486. doi: 10.1016/j.tplants.2012.04.001
Berg, G., Opelt, K., Zachow, C., Lottmann, J., Gotz, M., Costa, R., et al. (2006). The rhizosphere effect on bacteria antagonistic towards the pathogenic fungus Verticillium differs depending on plant species and site. FEMS Microbiol. Ecol. 56, 250–261. doi: 10.1111/j.1574-6941.2005.00025.x
Bulgarelli, D., Rott, M., Schlaeppi, K., Van Themaat, E. V. L., Ahmadinejad, N., Assenza, F., et al. (2012). Revealing structure and assembly cues for Arabidopsis root-inhabiting bacterial microbiota. Nature 488, 91–95. doi: 10.1038/nature11336
Caporaso, J. G., Kuczynski, J., Stombaugh, J., Bittinger, K., Bushman, F. D., Costello, E. K., et al. (2010). QIIME allows analysis of high-throughput community sequencing data. Nat. Methods 7, 335–336. doi: 10.1038/nmeth.f.303
Cardinale, M., Grube, M., Erlacher, A., Quehenberger, J., and Berg, G. (2015). Bacterial networks and co-occurrence relationships in the lettuce root microbiota. Environ. Microbiol. 17, 239–252. doi: 10.1111/1462-2920.12686
Cardinale, M., Ratering, S., Sadeghi, A., Pokhrel, S., and Schnell, S. (2020). The response of the soil microbiota to long-term mineral and organic nitrogen fertilization is stronger in the bulk soil than in the rhizosphere. Genes 11:456. doi: 10.3390/genes11040456
Chapin, F. S., Zavaleta, E. S., Eviner, V. T., Naylor, R. L., Vitousek, P. M., Reynolds, H. L., et al. (2000). Consequences of changing biodiversity. Nature 405, 234–242. doi: 10.1038/35012241
Chen, T., Li, J., Wu, L. K., Lin, S., Wang, J. H., Li, Z. F., et al. (2015). Effects of continuous monoculture of Achyranthes bidentata on microbial community structure and functional diversity in soil. Allelopathy J. 36, 197–211.
Chen, Y., Yan, F., Chai, Y., Liu, H., Kolter, R., Losick, R., et al. (2013). Biocontrol of tomato wilt disease by Bacillus subtilis isolates from natural environments depends on conserved genes mediating biofilm formation. Environ. Microbiol. 15, 848–864. doi: 10.1111/j.1462-2920.2012.02860.x
Compant, S., Samad, A., Faist, H., and Sessitsch, A. (2019). A review on the plant microbiome: ecology, functions, and emerging trends in microbial application. J. Adv. Res. 19, 29–37. doi: 10.1016/j.jare.2019.03.004
Cui, Z., Meng, Q. X., Ma, W., Zhang, X., Zhou, Z., and Ren, L. P. (2015). Diversity of the Intestinal Bacteria of Cattle Fed on Diets with Different Doses of Gelatinized Starch-Urea. Indian J. Microbiol. 55, 269–277. doi: 10.1007/s12088-015-0526-8
Drigo, B., Van Veen, J. A., and Kowalchuk, G. A. (2009). Specific rhizosphere bacterial and fungal groups respond differently to elevated atmospheric CO2. ISME J. 3, 1204–1217. doi: 10.1038/ismej.2009.65
Edgar, R. C. (2013). UPARSE: highly accurate OTU sequences from microbial amplicon reads. Nat. Methods 10, 996–998. doi: 10.1038/NMETH.2604
Edwards, J., Johnson, C., Santos-Medellin, C., Lurie, E., Podishetty, N. K., Bhatnagar, S., et al. (2015). Structure, variation, and assembly of the root-associated microbiomes of rice. Proc. Natl. Acad. Sci. U. S. A. 112, E911–E920. doi: 10.1073/pnas.1414592112
Egamberdieva, D., Kamilova, F., Validov, S., Gafurova, L., Kucharova, Z., and Lugtenberg, B. J. J. (2007). High incidence of plant growth-stimulating bacteria associated with the rhizosphere of wheat grown on salinated soil in Uzbekistan. Environ. Microbiol. 10, 1–9. doi: 10.1111/j.1462-2920.2007.01424.x
Elbeltagy, A. S., Nishioka, K., Sato, T., Suzuki, H., Ye, B., Hamada, T., et al. (2001). Endophytic Colonization and In Planta Nitrogen Fixation by a Herbaspirillum sp. Isolated from Wild Rice Species. Appl. Environ. Microbiol. 67, 5285–5293. doi: 10.1128/AEM.67.11.5285-5293.2001
Fan, K. K., Weisenhornc, P., Gilbertc, J. A., and Chua, H. Y. (2018). Wheat rhizosphere harbors a less complex and more stable microbial co-occurrence pattern than bulk soil. Soil Biol. Biochem. 125, 251–260. doi: 10.1016/j.soilbio.2018.07.022
Faust, K., and Raes, J. (2012). Microbial interactions: from networks to models. Nat. Rev. Microbiol. 10, 538–550. doi: 10.1038/nrmicro2832
Fierer, N., and Jackson, R. B. (2006). The diversity and biogeography of soil bacterial communities. Proc. Natl. Acad. Sci. U. S. A. 103, 626–631. doi: 10.1073/pnas.0507535103
Garbeva, P., Hol, W. H. G., Termorshuizen, A. J., Kowalchuk, G. A., and De Boer, W. (2011). Fungistasis and general soil biostasis - A new synthesis. Soil Biol. Biochem. 43, 469–477. doi: 10.1016/j.soilbio.2010.11.020
Han, Q., Lu, X., Bai, J., Qiao, Y., Pare, P. W., Wang, S., et al. (2014). Beneficial soil bacterium Bacillus subtilis (GB03) augments salt tolerance of white clover. Front. Plant Sci. 5:525–525. doi: 10.3389/fpls.2014.00525
Hao, H. R., Li, Z. F., Xiong, J., Chen, H., Zhang, Z. Y., and Lin, W. X. (2008). Variation of microbial flora and enzyme activity in rhizospheric soil under continuous cropping of Achyranthes bidentata. Chin. J. Eco Agric. 16, 301–311. doi: 10.3724/SP.J.1011.2008.00307
Hu, A., Ju, F., Hou, L., Li, J., Yang, X., Wang, H., et al. (2017). Strong impact of anthropogenic contamination on the co-occurrence patterns of a riverine microbial community. Environ. Microbiol. 19, 4993–5009. doi: 10.1111/1462-2920.13942
Jiang, Y. J., Li, S. Z., Li, R. P., Zhang, J., Liu, Y. H., Lv, L. F., et al. (2017). Plant cultivars imprint the rhizosphere bacterial community composition and association networks. Soil Biol Biochem. 109, 145–155. doi: 10.1016/j.soilbio.2017.02.010
Lakshmanan, V., Selvaraj, G., and Bais, H. P. (2014). Functional soil microbiome: belowground solutions to an aboveground problem. Plant Physiol. 166, 689–700. doi: 10.1104/pp.114.245811
Latz, E., Eisenhauer, N., Rall, B. R. C., Allan, E., Roscher, C., Scheu, S., et al. (2012). Plant diversity improves protection against soil-borne pathogens by fostering antagonistic bacterial communities. J Ecol. 100, 597–604. doi: 10.1111/j.1365-2745.2011.01940.x
Li, B., Li, Q., Xu, Z., Zhang, N., Shen, Q., and Zhang, R. (2014). Responses of beneficial Bacillus amyloliquefaciens SQR9 to different soilborne fungal pathogens through the alteration of antifungal compounds production. Front. Microbiol. 5:636. doi: 10.3389/fmicb.2014.00636
Li, L., Tilman, D., Lambers, H., and Zhang, F. S. (2014). Plant diversity and overyielding: insights from belowground facilitation of intercropping in agriculture. New Phytol. 203, 63–69. doi: 10.1111/nph.12778
Li, X., Ding, C., Hua, K., Zhang, T., Zhang, Y., Zhao, L., et al. (2014). Soil sickness of peanuts is attributable to modifications in soil microbes induced by peanut root exudates rather than to direct allelopathy. Soil Biol Biochem. 78, 149–159. doi: 10.1016/j.soilbio.2014.07.019
Li, G., Li, J., Kohl, K. D., Yin, B., Wei, W., Wan, X., et al. (2019). Dietary shifts influenced by livestock grazing shape the gut microbiota composition and co-occurrence networks in a local rodent species. J. Anim. Ecol. 88, 302–314. doi: 10.1111/1365-2656.12920
Li, J., Huang, J., Li, P., and Zhang, Z. Y. (2010). The changes on the contents of steroids and three terpenoids in Achyranthes bidentata under continuous cropping. Lishizhen Medicine Materia Medica Res. 21, 2433–2434. doi: 10.3969/j.issn.1008-0805.2010.10.006
Li, S., and Wu, F. (2018). Diversity and Co-occurrence Patterns of Soil Bacterial and Fungal Communities in Seven Intercropping Systems. Front. Microbiol. 9:1521. doi: 10.3389/fmicb.2018.01521
Li, Y., Zhang, W., Fu, Z. Z., Wang, W., and Li, Y. H. (2015). Isolation and characterization of microsatellite markers for Achyranthes bidentata (amaranthaceae) using next-generation sequencing platform. Biochem. Syst. Ecol. 61, 437–440. doi: 10.1016/j.bse.2015.06.032
Lievens, B., Brouwer, M., Vanachter, A. C. R. C., Levesque, C. A., Cammue, B. P. A., and Thomma, B. P. H. J. (2005). Quantitative assessment of phytopathogenic fungi in various substrates using a DNA macroarray. Environ. Microbiol. 7, 1698–1710. doi: 10.1111/j.1462-2920.2005.00816.x
Lin, D. X., Wang, E. T., Tang, H., Han, T. X., He, Y. R., Guan, S. H., et al. (2008). Shinella kummerowiae sp. nov., a symbiotic bacterium isolated from root nodules of the herbal legume Kummerowia stipulacea. Int. J. Syst. Evol. Microbiol. 58, 1409–1413. doi: 10.1099/ijs.0.65723-0
Lu, L. H., Yin, S. X., Liu, X., Zhang, W. M., Gu, T. Y., Shen, Q. R., et al. (2013). Fungal networks in yield-invigorating and -debilitating soils induced by prolonged potato monoculture. Soil Biol. Biochem. 65, 186–194. doi: 10.1016/j.soilbio.2013.05.025
Lugtenberg, B., and Kamilova, F. (2009). Plant-growth-promoting rhizobacteria. Annu. Rev. Microb. 63, 541–556. doi: 10.1146/annurev.micro.62.081307.162918
Lupatini, M., Suleiman, A. K. A., Jacques, R. J. S., Antoniolli, Z. I., Ferreira, A. O. D. S., Kuramae, E. E., et al. (2014). Network topology reveals high connectance levels and few key microbial genera within soils. Front. Environ. Sci. 2:10. doi: 10.3389/fenvs.2014.00010
Ma, B., Wang, H., Dsouza, M., Lou, J., He, Y., Dai, Z., et al. (2016). Geographic patterns of co-occurrence network topological features for soil microbiota at continental scale in eastern China. ISME J. 10, 1891–1901. doi: 10.1038/ismej.2015.261
Macdonald, C., and Singh, B. (2013). Harnessing plant-microbe interactions for enhancing farm productivity. Bioengineered 5, 5–9. doi: 10.4161/bioe.25320
Martínez-Viveros, O., Jorquera, M. A., Crowley, D. E., Gajardo, G., and Mora, M. L. (2010). Mechanisms and practical considerations involved in plant growth promotion by rhizobacteria. J. Soil Sci. Plant Nutr. 10, 293–319. doi: 10.4067/S0718-95162010000100006
Mendes, R., Kruijt, M., De Bruijn, I., Dekkers, E., Van Der Voort, M., Schneider, J. H. M., et al. (2011). Deciphering the Rhizosphere Microbiome for Disease-Suppressive Bacteria. Science 332:1097. doi: 10.1126/science.1203980
Mikhailov, I. S., Zakharova, Y. R., Bukin, Y. S., Galachyants, Y. P., Petrova, D. P., Sakirko, M. V., et al. (2019). Co-occurrence Networks Among Bacteria and Microbial Eukaryotes of Lake Baikal During a Spring Phytoplankton Bloom. Microbi. Ecol. 77, 96–109. doi: 10.1007/s00248-018-1212-2
Ofek-Lalzar, M., Sela, N., Goldman-Voronov, M., Green, S. J., Hadar, Y., and Minz, D. (2013). Niche and host-associated functional signatures of the root surface microbiome. Nat. Commun. 5:4950. doi: 10.1038/ncomms5950
Panke-Buisse, K., Poole, A. C., Goodrich, J. K., Ley, R. E., and Kao-Kniffin, J. (2014). Selection on soil microbiomes reveals reproducible impacts on plant function. ISME J. 9, 980–989. doi: 10.1038/ismej.2014.196
Perret, X., Staehelin, C., and Broughton, W. J. (2000). Molecular Basis of Symbiotic Promiscuity. Microbiol. Mol. Biol. Rev. 64, 180–201. doi: 10.1128/MMBR.64.1.180-201.2000
Poudel, R., Jumpponen, A., Kennelly, M. M., Rivard, C. L., Gomez-Montano, L., and Garrett, K. A. (2019). Rootstocks Shape the Rhizobiome: rhizosphere and Endosphere Bacterial Communities in the Grafted Tomato System. bioRxiv [Preprint]. doi: 10.1101/375444
Qian, P., Mi, C. Y., Jiang, K., Yang, R., Li, O., and Hu, X. F. (2016). Phylogenetic and metabolic responses of rhizosphere microbes to the cultivation of Panax notoginseng. J. Biobased Mater. Bioenergy 10, 370–377. doi: 10.1166/jbmb.2016.1616
Quast, C., Pruesse, E., Yilmaz, P., Gerken, J., Schweer, T., Yarza, P., et al. (2012). The SILVA ribosomal RNA gene database project: improved data processing and web-based tools. Nucleic Acids Res. 41, 590–596. doi: 10.1093/nar/gks1219
Raaijmakers, J. M., Leeman, M., Oorschot, M. M. P. V., Sluis, I. V. D., and Bakker, P. A. H. M. (1995). Dose-response relationships in biological control of Fusarium wilt of Radish by Pseudomonas spp. Phytopathology 85, 1075–1081. doi: 10.1094/Phyto-85-1075
Raaijmakers, J. M., Vlami, M., and Souza, J. T. D. (2002). Antibiotic production by bacterial biocontrol agents. Antonie Van Leeuwenhoek 81, 537–547. doi: 10.1023/A:1020501420831
Rout, M. E., and Southworth, D. (2013). The root microbiome influences scales from molecules to ecosystems: the unseen majority1. Am. J. Bot. 100, 1689–1691. doi: 10.3732/ajb.1300291
Schlaeppi, K., and Bulgarelli, D. (2015). The plant microbiome at work. Mol. Plant Microbe Interact. 28, 212–217. doi: 10.1094/MPMI-10-14-0334-FI
Shen, Z., Wang, D., Ruan, Y., Xue, C., Zhang, J., Li, R., et al. (2014). Deep 16S rRna pyrosequencing reveals a bacterial community associated with banana Fusarium wilt disease suppression induced by bio-organic fertilizer application. PLoS One 9:e98420. doi: 10.1371/journal.pone.0098420
Soriano, I. R., Riley, I. T., Potter, M. J., and Bowers, W. S. (2004). Phytoecdysteroids: a novel defense against plant-parasitic nematodes. J. Chem. Ecol. 30, 1885–1899. doi: 10.1023/B:JOEC.0000045584.56515.11
Sun, P., Xiao, X. G., Duan, L. S., Guo, Y. H., Qi, J. J., Liao, D. Q., et al. (2015). Dynamic transcriptional profiling provides insights into tuberous root development in Rehmannia glutinosa. Front. Plant Sci. 6:396. doi: 10.3389/fpls.2015.00396
Tagawa, M., Tamaki, H., Manome, A., Koyama, O., and Kamagata, Y. (2010). Isolation and characterization of antagonistic fungi against potato scab pathogens from potato field soils. FEMS Microbiol. Lett. 305, 136–142. doi: 10.1111/j.1574-6968.2010.01928.x
Timm, C. M., Campbell, A. G., Utturkar, S. M., Jun, S., Parales, R. E., Tan, W. A., et al. (2015). Metabolic functions of Pseudomonas fluorescens strains from Populus deltoides depend on rhizosphere or endosphere isolation compartment. Front. Microbiol. 6:1118. doi: 10.3389/fmicb.2015.01118
Unkovich, M., Herridge, D., Peoples, M., Cadisch, G., Boddey, B., Giller, K., et al. (2008). Measuring Plant-Associated Nitrogen Fixation In Agricultural Systems. ACIAR Monograph. 136. Canberra: Australian Centre for International Agricultural Research, 258.
Van Der Ent, S., Van Wees, S. C. M., and Pieterse, C. M. J. (2009). Jasmonate signaling in plant interactions with resistance-inducing beneficial microbes. Phytochemistry 69, 1581–1588. doi: 10.1016/j.phytochem.2009.06.009
Van der Heijden, M. G., Bardgett, R. D., and Van Straalen, N. M. (2008). The unseen majority: soil microbes as drivers of plant diversity and productivity in terrestrial ecosystems. Ecol. Lett. 11, 296–310. doi: 10.1111/j.1461-0248.2007.01139.x
Van der Heijden, M. G., and Schlaeppi, K. (2015). Root surface as a frontier for plant microbiome research. Proc. Natl. Acad. Sci. U. S. A. 112, 2299–2300. doi: 10.1073/pnas.1500709112
Wang, J., Wu, L., Tantai, H., Khan, M. U., Letuma, P., Wu, H., et al. (2019). Properties of bacterial community in the rhizosphere soils of Achyranthes bidentata tolerant to consecutive monoculture. Plant Growth Regul. 89, 167–178. doi: 10.1007/s10725-019-00523-0
Wang, Q., Garrity, G. M., Tiedje, J. M., and Cole, J. R. (2007). Naïve Bayesian classifier for rapid assignment of rRNA sequences into the new bacterial taxonomy. Appl. Environ. Microbiol. 73, 5261–5267. doi: 10.1128/AEM.00062-07
Wu, H. M., Qin, X. J., Wang, J. Y., Wu, L. K., Chen, J., Fan, J., et al. (2019). Rhizosphere responses to environmental conditions in Radix pseudostellariae under continuous monoculture regimes. Agric. Ecosyst. Environ. 27, 19–31. doi: 10.1016/J.AGEE.2018.10.014
Wu, H. M., Wu, L. K., Wang, J. Y., Zhu, Q., Lin, S., Xu, J. J., et al. (2016). Mixed Phenolic Acids Mediated Proliferation of Pathogens Talaromyces helicus and Kosakonia sacchari in Continuously Monocultured Radix pseudostellariae Rhizosphere Soil. Front Microbiol. 7:335. doi: 10.3389/fmicb.2016.00335
Wu, H. M., Xu, J. J., Wang, J. Y., Qin, X. J., Wu, L. K., Li, Z. C., et al. (2017). Insights into the mechanism of proliferation on the special microbes mediated by phenolic acids in the Radix pseudostellariae rhizosphere under continuous monoculture regimes. Front. Plant Sci. 8:659. doi: 10.3389/fpls.2017.00659
Wu, L., Li, Z., Li, J., Khan, M. A., Huang, W., Zhang, Z., et al. (2013). Assessment of shifts in microbial community structure and catabolic diversity in response to Rehmannia glutinosa monoculture. Appl. Soil Ecol. 67, 1–9. doi: 10.1016/j.apsoil.2013.02.008
Wu, L., Wang, J., Huang, W., Wu, H., Chen, J., Yang, Y., et al. (2015). Plant-microbe rhizosphere interactions mediated by Rehmannia glutinosa root exudates under consecutive monoculture. Sci. Rep. 5:15871. doi: 10.1038/srep19101
Xiong, W., Li, R., Ren, Y., Liu, C., Zhao, Q., Wu, H., et al. (2017). Distinct roles for soil fungal and bacterial communities associated with the suppression of vanilla Fusarium wilt disease. Soil Biol. Biochem. 107, 198–207. doi: 10.1016/j.soilbio.2017.01.010
Xiong, W., Zhao, Q. Y., Xue, C., Xun, W. B., Zhao, J., Wu, H. S., et al. (2016). Comparison of fungal community in black pepper-vanilla and vanilla monoculture systems associated with vanilla fusarium wilt disease. Front. Microbiol. 7:117. doi: 10.3389/fmicb.2016.00117
Zahir, Z. A., Arshad, M., and Frankenberger, W. T. (2003). Plant growth promoting rhizobacteria: applications and perspectives in agriculture. Adv. Agron. 81, 97–168. doi: 10.1016/S0065-2113(03)81003-9
Zhang, B. G., Peng, Y., Zhang, Z., Liu, H. T., Qi, Y. D., Liu, S., et al. (2010). GAP production of TCM herbs in China. Planta Med. 76, 1948–1955. doi: 10.1055/s-0030-1250527
Zhang, Y., Xu, J., Riera, N., Jin, T., Li, J., and Wang, N. (2017). Huanglongbing impairs the rhizosphere-to-rhizoplane enrichment process of the citrus root-associated microbiome. Microbiome 5:97. doi: 10.1186/s40168-017-0304-4
Zhou, D., Huang, X. F., Chaparro, J. M., Badri, D. V., and Guo, J. (2015). Root and bacterial secretions regulate the interaction between plants and PGPR leading to distinct plant growth promotion effects. Plant Soil 401, 259–272. doi: 10.1007/s11104-015-2743-7
Keywords: co-occurrence network, rhizosphere, rhizoplane, root, plant–soil interactions
Citation: Wang J, Wu H, Wu L, Liu Y, Letuma P, Qin X, Chen T, Rensing C, Lin S and Lin W (2021) Revealing Microbiome Structure and Assembly Process in Three Rhizocompartments of Achyranthes bidentata Under Continuous Monoculture Regimes. Front. Microbiol. 12:677654. doi: 10.3389/fmicb.2021.677654
Received: 08 March 2021; Accepted: 10 May 2021;
Published: 14 June 2021.
Edited by:
Sabine Dagmar Zimmermann, Délégation Languedoc Roussillon (CNRS), FranceReviewed by:
Massimiliano Cardinale, University of Salento, ItalyMonica Calvo-Polanco, University of Salamanca, Spain
Xiaogang Li, Nanjing Forestry University, China
Copyright © 2021 Wang, Wu, Wu, Liu, Letuma, Qin, Chen, Rensing, Lin and Lin. This is an open-access article distributed under the terms of the Creative Commons Attribution License (CC BY). The use, distribution or reproduction in other forums is permitted, provided the original author(s) and the copyright owner(s) are credited and that the original publication in this journal is cited, in accordance with accepted academic practice. No use, distribution or reproduction is permitted which does not comply with these terms.
*Correspondence: Sheng Lin, bGluc2hAZmFmdS5lZHUuY24=; Wenxiong Lin, bHd4QGZhZnUuZWR1LmNu