- 1Laboratory of Genetic Technology, Department of Genetics, Ecology and Evolution, Institute of Biological Sciences, Federal University of Minas Gerais (UFMG), Belo Horizonte, Brazil
- 2Laboratory of Cellular and Molecular Genetics, Department of Genetics, Ecology and Evolution, Institute of Biological Sciences, Federal University of Minas Gerais (UFMG), Belo Horizonte, Brazil
- 3Laboratory of Biotechnological Innovation, Research and Development Directorate, Ezequiel Dias Foundation (FUNED), Belo Horizonte, Brazil
The development of a new vaccine strategy against tuberculosis is urgently needed and has been greatly encouraged by the scientific community worldwide. In this work, we constructed a lactococcal DNA vaccine based on the fusion of two Mycobacterium tuberculosis antigens, ESAT-6 and Ag85A, and examined its immunogenicity. The coding sequences of the ESAT-6 and Ag85A genes were fused and cloned into the eukaryotic expression pValac vector, and the functionality of the vector was confirmed in vitro. Then, L. lactis FnBPA+ (pValac:e6ag85a) was obtained and used for oral immunization of mice. This strain induced significant increases in IFN-γ, TNF-α, and IL-17 cytokines in stimulated splenocyte cultures, and significant production of antigen-specific sIgA was observed in the colonic tissues of immunized mice. We demonstrated that L. lactis FnBPA+ (pValac:e6ag85a) generated a cellular and humoral immune response after oral immunization of mice. The strategy developed in this work may represent an interesting DNA mucosal vaccine candidate against tuberculosis, using the fusion of two highly immunogenic antigens delivered by safe lactic acid bacteria.
Introduction
Tuberculosis (TB), an infectious disease caused by Mycobacterium tuberculosis, remains a serious public health problem worldwide, especially in developing countries. The only vaccine available for clinical use is Bacillus Calmette-Guérin (BCG) and, although BCG is widely used, its efficacy against pulmonary TB is controversial (Kaufmann et al., 2015). Hence, the investigation of strategies for the development of more effective and economically viable vaccines against tuberculosis is necessary and has been greatly encouraged. Over the last few years, the international scientific community has made a substantial effort to search for more effective vaccine alternatives to BCG or to increase its immunogenicity. These alternatives include vaccines based on genetically modified mycobacteria lineages, subunit vaccines and DNA vaccines (Kaufmann et al., 2014). DNA vaccines have the advantages of T cell activation and antibody production without the possible limitations of immunization with live pathogenic microorganisms (Kutzler and Weiner, 2008).
The proteins secreted by M. tuberculosis are considered attractive vaccine candidate antigens (Andersen et al., 1991). Among these proteins, two are prominent: ESAT-6 and Ag85A. 6-kDa Early Secreted Antigenic Target (ESAT-6) is an immunodominant antigen secreted in the early stages of infection and is present in pathogenic M. tuberculosis but absent in BCG (Pym et al., 2002). Ag85A belongs to the antigen 85 complex (Ag85) and is considered a highly immunogenic virulence factor (Dietrich et al., 2006). Studies have revealed that DNA vaccines using these proteins can increase the T helper cell type 1 (Th1) response, which is critical in protection against tuberculosis (Romano et al., 2006; Xu et al., 2008).
Currently, despite the various available DNA vaccine delivery methods, bacterial mucosal immunization has emerged as a good strategy (Schoen et al., 2004). However, many of these microorganisms are attenuated enteropathogenic bacteria, and the potential risk of reversion may difficult their use (Dunham, 2002). Fortunately, this risk can be circumvented by the use of non-pathogenic bacteria, such as Lactococcus lactis.
Lactococcus lactis, considered the model lactic acid bacteria, is classified as Generally Recognized As Safe (GRAS) and has been used for production and delivery of antigens and cytokines to mucosal surfaces for a long time (Wells, 2011). The potential of this microorganism as a vehicle for the delivery of DNA vaccines has been investigated and good results were observed (Del Carmen et al., 2013; Pereira et al., 2015; Souza et al., 2016; Mancha-Agresti et al., 2017; Zurita-Turk et al., 2020). These results were achieved by the use of the pValac vector (Guimarães et al., 2009) and the recombinant strain L. lactis FnBPA+ that expresses fibronectin binding protein A (FnBPA) of Staphylococcus aureus, an invasin involved in adhesion and invasion of eukaryotic cells (Que et al., 2001). In vitro and in vivo assays demonstrated the invasiveness of L. lactis FnBPA+ and its ability to deliver the pValac vector to eukaryotic cells (Innocentin et al., 2009; Pontes et al., 2012; Almeida et al., 2014).
Thus, our aim was to construct L. lactis FnBPA+ harboring the pValac vector containing the fusion ORF ESAT6-Ag85A of M. tuberculosis and use this system for oral immunization of mice. Moreover, we aimed to evaluate the immune response generated by this vaccine strategy.
Materials and Methods
Bacterial Strains, Plasmids, and Growth Conditions
The plasmid and bacterial strains used are listed in Table 1. The pValac plasmid was constructed by our group in 2009 (Guimarães et al., 2009). The L. lactis FnBPA+ lineage was developed by Que et al. (2001), who kindly gave it to the group of Dr. Philippe Langella from INRAe—France. Dr. Philippe Langella passed it on to us and, currently, both the plasmid and the lineage are part of our microbiological collection.
Escherichia coli TG1 was grown in LB broth (Acumedia, San Bernardino, United States) at 37°C with shaking. Lactococcus lactis FnBPA+ was grown at 30°C without shaking in M17 broth (Sigma-Aldrich, Darmstadt, Germany) enriched with 0.5% glucose (GM17). Recombinant bacteria were selected by addition of the following antibiotics: for L. lactis FnBPA+, erythromycin at 5 μg/ml; for L. lactis FnBPA+ (pValac:e6ag85a), erythromycin at 5 μg/ml and chloramphenicol at 10 μg/ml; and for E. coli (pValac:e6ag85a), chloramphenicol at 10 μg/ml.
Plasmids from E. coli and L. lactis were isolated as described by Green and Sambrook (2012), with the following modification: for L. lactis, TE-LYS (25% sucrose, 1 mmol EDTA, 50 mmol Tris–HCl, pH 8, lysozyme 10 mg/ml) was added to the samples for 1 h at 37°C before the addition of lysis solution.
DNA Vaccine Construction
The ESAT-6 and the Ag85A coding sequences were amplified by PCR using Pfx Platinum® High-Fidelity DNA Polymerase (Life Technologies, Carlsbad, United States) and the genomic DNA of M. tuberculosis H37Rv strain (ATCC 27294) as a template. The oligonucleotides used for ESAT-6 were as follows: 5′ - CGGGATCCCCACCATGGAGCAGCAGTGGAATTTCGCG-3′ (forward) containing the BamHI restriction site (underlined) and the customized Kozak sequence (in bold) and 5′ - CTAGTCTAGATGCGAACATCCCAGTGACG-3′ (reverse) containing the XbaI restriction site (underlined). The reverse primer was designed without a stop codon. The oligonucleotides used for Ag85A were as follows: 5′ -CTAGTCTAGAATGCAGCTTGTTGACAGGGTTC-3′ (forward) containing the XbaI restriction site (underlined) and 5′ CGGAATTCCTAGGCGCCCTGGGGCGC-3′ (reverse) containing the EcoRI restriction site (underlined).
PCR products were purified from agarose gels using illustra™ GFX™ PCR DNA and Gel Band Purification kit (GE Healthcare, Chicago, United States) and individually digested with XbaI. Then, fragments were ligated using T4 DNA ligase (Life Technologies, Carlsbad, United States). The fusion fragment ESAT6-Ag85A was digested with BamHI and EcoRI enzymes and inserted into the corresponding sites of the eukaryotic expression vector pValac (Guimarães et al., 2009), which was previously cleaved with the same restriction enzymes.
The pValac:e6ag85a plasmid (Figure 1) was transformed into E. coli TG1, producing the E. coli TG1 (pValac:e6ag85a) strain. The insert integrity was confirmed by DNA sequence analysis, using a BigDye Terminator v3.1 Cycle Sequencing kit (Applied Biosystems, Foster City, United States) and ABI3130 sequencing equipment (Applied Biosystems, Foster City, United States). Finally, pValac:e6ag85a was transformed by electroporation (Langella et al., 1993) into L. lactis FnBPA+, producing the L. lactis FnBPA+ (pValac:e6ag85a) strain.
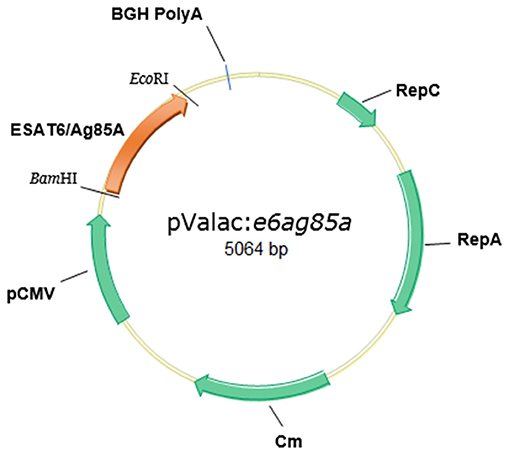
Figure 1. Structure of the pValac:e6ag85a vector. Tick marks indicate BamHI and EcoRI restriction sites and BGH polyadenylation region (polyA). Arrows indicate cytomegalovirus promoter (pCMV), ORF of the ESAT6/Ag85A fusion protein, replication origin of E. coli (RepC), L. lactis (RepA), and chloramphenicol resistance gene (Cm).
Cell Transfection and Protein Expression Assays
The pValac:e6ag85a vector was transfected into the Chinese hamster ovarian cell line (Flp-In™-CHO; Life Technologies, Carlsbad, United States) to evaluate its functionality by analyzing the expression of the protein by confocal microscopy and flow cytometry. CHO cells were cultured in F12 Ham media (Gibco, Dublin, Ireland) supplemented with 10% fetal bovine serum, 1% L-glutamine, zeocin (100 ng/ml) and 2.5% HEPES [4-(2-hydroxyethyl)-1-piperazineethanesulfonic acid]. The cells were transfected with 4 μg of pValac:e6ag85a vector using Lipofectamine™ 2000 transfection reagent (Life Technologies, Carlsbad, United States) as described by the supplier. Cells that received the pValac:gfp vector served as the positive control; cells that did not receive plasmids served as the negative control. DNA plasmids used in transfection were prepared using Qiagen® Plasmid Midi kit (Qiagen, Hilden, Germany), according to the manufacturer's instruction.
Forty-eight hours post transfection, pValac:e6ag85a-transfected and control cells were fixed with 4% paraformaldehyde (Sigma-Aldrich, Darmstadt, Germany) for 15 min and permeabilized with 0.1% Triton X-100 (Sigma-Aldrich, Darmstadt, Germany) for 10 min. Then, the cells were incubated for 2 h with a specific mouse monoclonal immunoglobulin G1 (IgG1) anti-ESAT-6 antibody 1 mg/ml, diluted 1/1000 in PBS/BSA 1% (Abcam ab26246; Abcam, Cambridge, United Kingdom). Next, the cells were incubated with goat anti-mouse IgG (H+L) Alexa Fluor® 488 (4 μg/ml, diluted 1/500 in PBS/BSA 1%; Life Technologies, Carlsbad, United States) for 1 h in darkness. Nuclear labeling was performed concurrently with secondary antibody labeling by incubation with the fluorochrome DAPI 2 μg/ml (Life Technologies, Carlsbad, United States), diluted 1:300 in PBS/BSA 1%. Samples were mounted and images were captured using a Zeiss LSM 510 META inverted confocal laser-scanning microscope (Zeiss, Oberkochen, Germany). Images were collected and analyzed using Zeiss LSM Image Browser software (Zeiss, Oberkochen, Germany).
For flow cytometry analysis, 1 × 106 pValac:e6ag85a-transfected and control cells (non-transfected cells) were fixed and permeabilized using a Mouse Foxp3 Buffer Set kit (BD PharmingenTM, San Jose, United States). The cells were then incubated for 30 min with mouse monoclonal IgG1 anti-ESAT-6 antibody Abcam ab26246 diluted 1/1000 in PBS/BSA 1% or with mouse monoclonal anti-Ag85A antibody (H-2b haplotype mice—Mab DT-17/4, source: Professor Kris Huygens, Pasteur Institute of Brussels, Belgium) diluted 1/50 in PBS/BSA 1%. Next, the cells were incubated with secondary goat anti-mouse IgG (H+L) Alexa Fluor® 488 diluted 1/500 in PBS/BSA 1% for 30 min. Finally, the cells were centrifuged and suspended in PBS, and quantification of ESAT-6- and Ag85A-producing CHO cells was performed by BD Accuri™ C6 Flow Cytometer equipment (BD Biosciences, San Jose, United States). The acquired data were analyzed using FCAP array software (BD Biosciences, San Jose, United States).
Animals and Ethical Approval
Conventional female BALB/c mice (6–7 weeks old) were used for the immunization assays. Mice were obtained from the Central Bioterium of Federal University of Minas Gerais (UFMG, Belo Horizonte, Brazil). They were maintained in mini-isolators housed in ventilated racks with controlled conditions (temperature of 22 ± 2°C, humidity 50 ± 10%, air flow 35 exchanges/hour and light exposure 12-h light/dark cycle) and free access to water and rodent food. All the experiments and handling of the mice were performed in accordance with the ethical principles for animal experimentation adopted by the Animal Use Ethics Commission (CEUA, Registration Number 66/11) of Federal University of Minas Gerais (UFMG, Belo Horizonte, Brazil).
Immunization
BALB/c mice were randomly divided into the following experimental groups: negative control (saline 0.9%) (-), L. lactis FnBPA+ (invasive L. lactis strain, negative control) (FN) and L. lactis FnBPA+ (pValac:e6ag85a) (FEA). Each animal was orally immunized by gavage with 1 × 108 CFU in a final volume of 100 μl of saline 0.9%. The immunizations were performed at three different time points (days 1, 15, and 29) for three consecutive days at each time point. On day 43, all animals were anesthetized by intraperitoneal injection with a ketamine (60 mg/kg) and xylazine (8 mg/kg) cocktail and then euthanized. Two individual experiments were performed for each individual protocol (six animals/group).
Cytokine Measurement
Cytokine levels in the splenocyte supernatants were measured using a commercially available enzyme-linked immunosorbent assay (ELISA) kit (R&D DuoSet kit; R&D SystemsTM, Minneapolis, United States) according to the manufacturer's instructions to characterize the cellular immune response profile. Briefly, spleens were aseptically removed on the day of sacrifice and macerated; red blood cells were lysed. A total of 1 × 106 splenocytes from each immunized animal were plated (96-well microtiter plates) in complete RPMI-1640 (Sigma-Aldrich, Darmstadt, Germany) medium [10% fetal bovine serum (Gibco, Dublin, Ireland), sodium pyruvate 1 mmol, non-essential amino acids 1 mmol (Gibco, Dublin, Ireland), gentamicin 25 μg/ml (Gibco, Dublin, Ireland), and L-glutamine 2 mmol (LGC Biotecnologia, Cotia, Brazil)]. These cells were submitted to treatment with only RPMI (non-stimulated cells; negative control) or with RPMI containing 5 μg/ml of rESAT-6 (Pereira et al., 2015) and then incubated at 37°C in 5% CO2. As a positive control, one sample of each experimental group was stimulated with RPMI containing Concanavalin A (ConA—Sigma-Aldrich, Darmstadt, Germany) 16 μg/ml (data not shown).
Cell supernatants were collected to measure the levels of the cytokines interferon-gamma (INF-γ), tumor necrosis factor alpha (TNF-α), interleukin 17 (IL-17), 10 (IL-10), and 4 (IL-4) by sandwich ELISA using the described kit, after 60 hours of stimulation. Absorbance was measured at 492 nm using an Expert Plus Microplate Reader (Biochrom Asys, Cambridge, United Kingdom). Results for all cytokines were calculated by subtracting the basal values of cytokines from cells that received only RPMI medium from the values of cytokines from rESAT-6-stimulated cells.
Characterization of the Humoral Immune Response Pattern
The humoral immune response of immunized animals was evaluated by measuring mucosal anti-ESAT-6 sIgA levels in macerated mouse colons. For this, colons were macerated in buffer solution containing anti-proteases in a ratio of 1 ml of solution to 100 mg of tissue. The IKA T10 Basic Homogenizer workcenter (IKA® Brasil, Campinas, Brazil) was employed for this purpose. The homogenates were centrifuged, the supernatant was collected and the measurement of sIgA was performed by indirect ELISA as follows. Recombinant ESAT-6 (5 μg/ml) was used to coat microliter plates overnight (Pereira et al., 2015). Then, samples without dilution were added and incubated for 1 h. Next, horseradish peroxidase (HRP)-conjugated goat anti-mouse antibodies (Sigma-Aldrich, Darmstadt, Germany) diluted in PBS/casein (1/8000) were added and incubated for 2 h. Finally, orthophenylenediamine (OPD−1 mg/ml; Sigma Aldrich, Darmstadt, Germany) was used for color development as an indicator. Absorbance was measured at 492 nm using an ELISA Expert Plus Microplate Reader (Biochrom Asys, Cambridge, United Kingdom).
Statistical Analysis
Statistical analyses were performed using one-way analysis of variance (ANOVA) followed by Bonferroni post-test using GraphPad Prism 5.0 software (San Diego, CA, United States). The data are expressed as the mean ± standard error of the mean (SEM); p < 0.05, p < 0.01, and p < 0.001 were considered statistically significant.
Results
Construction of the Recombinant Strain L. lactis FnBPA+ (pValac:e6ag85a)
The fused ORF ESAT6-Ag85A (1,322 bp) (Gen Bank number ESAT-6: 886209; Ag85A: 886132) was directionally cloned into the pValac vector. pValac comprises the cytomegalovirus promoter (pCMV), the polyadenylation sequence of bovine growth hormone (BGH) (unit of eukaryotic expression), the origins of replication for both E. coli and L. lactis, and the chloramphenicol (Cm) resistance gene (prokaryotic region). The final pValac:e6ag85a construct (Figure 1) was confirmed by molecular biology methods such as PCR, enzymatic digestion, and sequencing (data not shown). Recombinant L. lactis FnBPA+ (pValac:e6ag85a) was constructed by transformation of the invasive L. lactis FnBPA+ strain with the pValac:e6ag85a plasmid.
Eukaryotic Cells Transfected With the Plasmid pValac:e6ag85a Can Express ESAT6-Ag85A Protein
The functionality of pValac:e6ag85a was confirmed by confocal microscopy and flow cytometry. In confocal microscopy analysis, pValac:e6ag85a-transfected CHO cells labeled with a specific mouse anti-ESAT-6 monoclonal antibody and secondary antibody conjugated to Alexa 488 showed green fluorescence in the cytoplasm, thus confirming the expression of ESAT-6 protein (Figure 2A). Protein expression was not detected in untransfected CHO cells labeled with the same primary and secondary antibodies (negative control) (Figure 2B). Cells transfected with the pValac:gfp plasmid were assayed as a positive control, which showed specific green fluorescence protein (GFP) expression (Figure 2C).
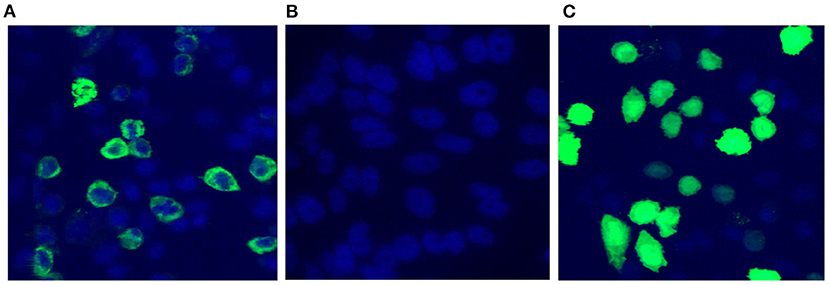
Figure 2. Confirmation of pValac:e6ag85a functionality. Confocal Microscopy. (A) CHO cells transfected with pValac:e6ag85a labeled with anti-ESAT-6 primary antibody, Alexa 488 secondary antibody and DAPI; (B) Untransfected CHO cells labeled with anti-ESAT-6 primary antibody, Alexa 488 secondary antibody and DAPI (negative control); (C) CHO cells transfected with pValac:gfp labeled with DAPI (positive control). Images obtained using a Zeiss LSM 510 META inverted confocal laser-scanning microscope with a 63X objective.
In flow cytometry, cells transfected with the plasmid pValac:e6ag85a and labeled with the antibody against ESAT-6 (Figure 3A) or Ag85A (Figure 3C) antigens showed protein expression in 6.3 and 5.6% of the analyzed events, respectively. Indeed, no expression was observed in non-transfected cells (Figures 3B,D). Taken together, these results confirmed the functionality of the pValac:e6ag85a vector.
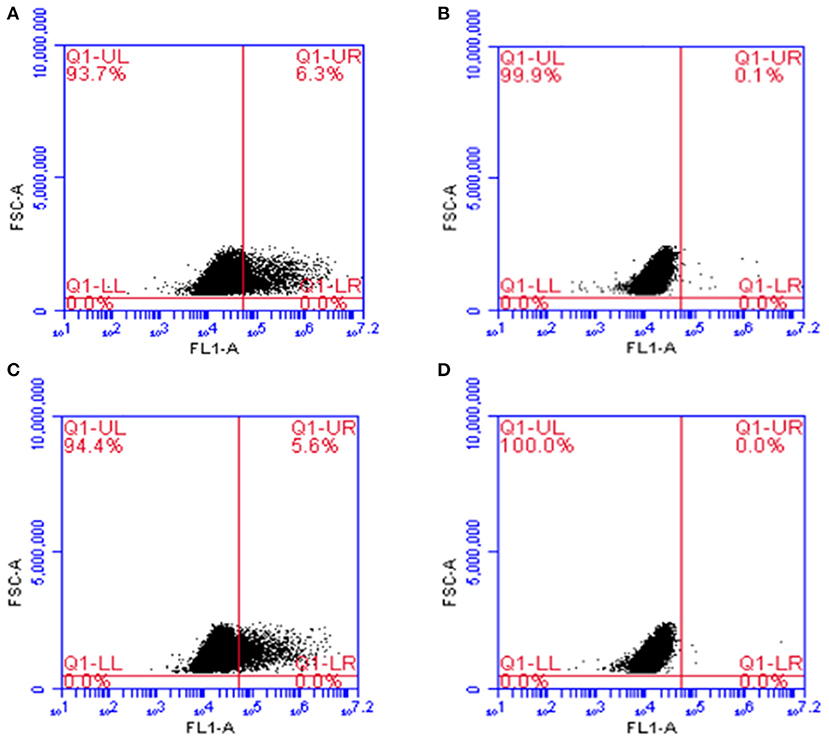
Figure 3. Expression of ESAT-6 and Ag85A proteins by CHO cells transfected with the pValac:e6ag85a plasmid. Flow Cytometry. (A) Transfected cells labeled with primary anti-ESAT-6 and secondary Alexa 488 antibodies; (B) Non-transfected cells labeled with primary anti-ESAT-6 and secondary Alexa 488 antibodies; (C) Transfected cells labeled with primary anti-Ag85A and secondary Alexa 488 antibodies; (D) Non-transfected cells labeled with primary anti-Ag85A and secondary Alexa 488 antibodies. Images obtained using FCAP array software (BD Biosciences).
Oral Immunization With L. lactis FnBPA+ (pValac:e6ag85a) Can Induce IFN-γ, TNF-α, and IL-17 Cytokines
To determine the cellular immune response pattern after mouse immunization, we measured the levels of IFN-γ, TNF-α, IL-17, IL-4, and IL-10 cytokines in the supernatant of rESAT-6-stimulated splenocytes. Compared with control mice immunized with L. lactis FnBPA+ (FN) or saline (-), mice immunized with L. lactis FnBPA+ (pValac:e6ag85a) (FEA) exhibited a significant increase in IFN-γ and TNF- α levels (Figures 4A,B).
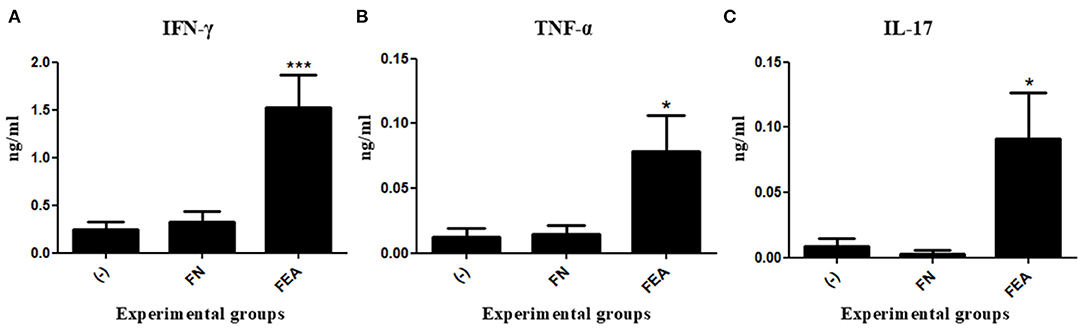
Figure 4. Production of cytokines after oral immunization of mice. Levels of (A) IFN-γ, (B) TNF-α, and (C) IL-17 in the supernatant of splenocyte cultures stimulated with rESAT-6 and analyzed by ELISA. Experimental groups: Saline (-; negative control), Lactococcus lactis FnBPA+ (FN; negative control), and Lactococcus lactis FnBPA+ (pValac:e6ag85a) (FEA). Data are shown as the mean ± SEM of two independent experiments (n = 12). *P < 0.05 or ***P < 0.001.
Regarding IL-17, mice immunized with L. lactis FnBPA+ (pValac:e6ag85a) showed significantly higher levels of this cytokine than did mice immunized with L. lactis FnBPA+ or saline (Figure 4C). The other cytokines measured, IL-4 and IL- 10, which are specific to the Th2 immune response, were not detected in the supernatants of the splenocytes culture.
Increased sIgA Is Detected After Immunization With L. lactis FnBPA+ (pValac:e6ag85a)
The principal immunoglobulin in mucosa immunity is secretory IgA (sIgA). The results obtained in this study showed that anti-ESAT-6 sIgA levels in the macerated colons of mice immunized with L. lactis FnBPA+ (pValac:e6ag85a) were significantly higher than those observed in the colons of mice immunized with L. lactis FnBPA+ or saline (Figure 5). Indeed, these results demonstrated that administration of the L. lactis FnBPA+ (pValac:e6ag85a) strain induced a mucosal immune response in vivo.
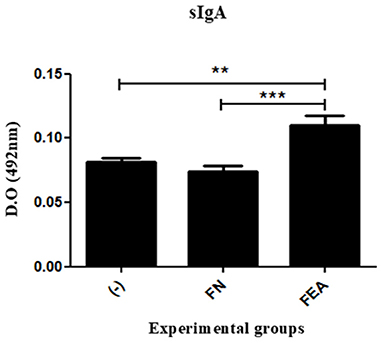
Figure 5. Production of sIgA after oral immunization of mice. Levels of anti-ESAT6 sIgA in the colonic tissues of mice orally immunized with L. lactis FnBPA+ (pValac:e6ag85a) and analyzed by ELISA. Experimental groups: Saline (-; negative control), Lactococcus lactis FnBPA+ (FN; negative control), and Lactococcus lactis FnBPA+ (pValac:e6ag85a) (FEA). Data are shown as the mean ± SEM of two independent experiments (n = 12). **P < 0.01 or ***P < 0.001.
Discussion
The use of Lactococcus lactis FnBPA+ for mucosal DNA delivery is a promising strategy for immunization. The main mucosae exploited for immunization with DNA vaccines are intranasal (Dou et al., 2012) and oral (Wang et al., 2009; Pereira et al., 2015, 2017). Perhaps the most advantageous is the oral route, considering that the gastrointestinal tract contains more cells of the immune system than any other tissue, representing the largest immunological compartment of the organism (Mowat and Agace, 2014). Thus, the development of DNA vaccines suitable for oral immunization, aiming at reaching the intestinal mucosa and, consequently, their inherent immunological advantages, is particularly interesting.
Recently, using the same vaccination strategy employed in this work but exploring only the role of ESAT-6 as a candidate antigen, Pereira et al. reported a significant increase in IFN-γ production in mice orally immunized with L. lactis FnBPA+ (pValac:esat6). However, no differences were observed in the expression of other equally important cytokines, such as TNF-α, in the immune response against M. tuberculosis (Pereira et al., 2015).
Moreover, Mancha-Agresti et al. (2017) developed the L. lactis FnBPA+ (pValac:ag85a) DNA vaccine, which encodes the Ag85A antigen. Following intranasal administration of the vaccine to mice, they found a significant increase in production of IFN-γ and TNF-α by the animals, similar to the present study, but with smaller magnitudes. In addition, the increase in IL-17, which is important in the early stages of TB control, was not verified (Mancha-Agresti et al., 2017).
In this study, we used the coding sequence of these two highly immunogenic proteins in a construct in which they were fused and cloned into the pValac vector. The pValac:e6ag85a functionality was confirmed, and this plasmid was transformed into the invasive L. lactis FnBPA+ strain. L. lactis FnBPA+ (pValac:e6ag85a) was used to immunize BALB/c mice by the oral route, and cellular and humoral immune responses were evaluated.
Concerning the cellular immune response, compared with the control groups, the group immunized with the L. lactis FnBPA+ (pValac:e6ag85a) strain showed increased IFN-γ, TNF-α, and IL-17 levels. IFN-γ is considered the most relevant cytokine in the immune response against M. tuberculosis and is mainly secreted in response to the activation of naive CD4+ T cells in Th1-type effector cells. Th1 cells represent a primordial defense against intracellular microorganisms, such as M. tuberculosis (Lyadova and Panteleev, 2015). In a previous study, mice deficient in IFN-γ died in the early stages of M. tuberculosis infection and presented a high mycobacterial burden in their bodies (Cooper et al., 1993). Similar effects have been observed in humans who have defective IFN-γ signaling pathways, which results in severe infections caused by this microorganism (van de Vosse et al., 2004).
TNF-α, in turn, is a potent activator of macrophages, acting in synergy with IFN-γ in the induction of its antimicrobial activity (Mootoo et al., 2009). Knock-out mice for TNF-α developed a lethal M. tuberculosis infection (Jacobs et al., 2000). In humans, the importance of TNF-α has been demonstrated notably by the increased risk of reactivation of latent TB in patients with chronic inflammatory diseases who underwent anti-TNF-α therapies (Feldmann and Maini, 2001; Harris and Keane, 2010).
The IL-17 cytokine, secreted by Th17 cells, acts on the stimulation of the granulocytic lineage, the recruitment of neutrophils to sites of infection and the induction of pro-inflammatory molecules secretion (Lyadova and Panteleev, 2015). In the human bronchial epithelium, Th17 cells induce the expression of antimicrobial mucins and peptides, which together with neutrophil activity, provide mechanisms to combat M. tuberculosis (Kao et al., 2004; Tsai et al., 2013). Low levels of IL-17 in TB patient serum is related to high mortality (Lyadova and Panteleev, 2015).
Taken together, these findings support the concept that the generation of Th1 and Th17 cellular immune response profiles with production of their signature cytokines is necessary for TB defense; these patterns were observed in this work. When comparing the results of the present study with those obtained by Mancha-Agresti et al. (2017) and Pereira et al. (2015), we believe that the results obtained here are due to a greater amount of target epitopes coexisting in the same vaccine plasmid, which generated stronger immune responses.
Regarding the mucosal immune response generated after vaccination with L. lactis FnBPA+ (pValac:e6ag85a), there was a significant difference between the group that received the DNA vaccine and the control groups. Among the functions of this immunoglobulin, the neutralization of antigens and pathogens in the extracellular environment, with the consequent inhibition of mucosal colonization (Corthésy, 2013), is notable. Furthermore, IgA knock-out mice were more susceptible to infection, which was evidenced by the high mycobacterial load found in the lungs of these animals (Williams et al., 2004; Rodriguez et al., 2005). Thus, a vaccine that induces antigen-specific sIgA responses is particularly desirable for protective immunity, especially for diseases in which the mucosa is their primary site of induction, as is the case of tuberculosis.
In other studies that adopted the approach of multigenic vaccines, results similar to those of this study were obtained. Wang et al. constructed a DNA vaccine encoding a fusion protein composed of ESAT-6 and Ag85B antigens (belonging to the same Ag85A family), which was orally delivered to mice through an attenuated strain of Salmonella typhimurium. The animals immunized with this vaccine showed high production of antigen-specific IFN-γ as well as increased levels of sIgA and were protected from a M. tuberculosis challenge (Wang et al., 2009). Although interesting, this strategy used pathogenic bacteria as a DNA delivery vector; despite attenuation of the pathogenicity, the risks of reversion to its wild phenotype were not eliminated, which may restrict its use.
Dou et al. tested a DNA vaccine encoding the Ag85A/ESAT-6 fusion protein in association with the pro-inflammatory cytokine IL-21. They used a prime boost scheme, with naked DNA intranasally administered first, followed by BCG as the booster. The researchers observed that vaccinated animals had elevated levels of IFN-γ, measured in the supernatant of the splenocyte culture, as well as activation of the cytotoxic activity of NK cells and increased levels of sIgA in the bronchoalveolar lavage. This vaccine also demonstrated protection against a challenge with M. tuberculosis (Dou et al., 2012). However, naked DNA delivery may expose the plasmids to a nuclease-rich environment, which may eventually degrade them, rendering the process unfeasible.
Our vaccine strategy can, then, overcome the abovementioned obstacles since it uses bacteria with a recognized safety profile as a delivery vector for the DNA vaccine. In this way, the plasmid is protected against degradation by nucleases, which may lower the required dose for administration. In addition, the oral route explored in this work allows the DNA vaccine to induce a mucosal immune response, reaching the intestinal mucosa, which is considered the body's largest immune compartment (Mowat and Agace, 2014). In turn, mucosal immunization is extremely important for defense against pathogens that access the host organism through this route, as is the case of M. tuberculosis.
In conclusion, L. lactis FnBPA+ (pValac:e6ag85a) was functional after oral immunization and presented features indicative of its potential protective capacity; these results have encouraged us to perform challenge trials with M. tuberculosis in the future.
Data Availability Statement
The datasets presented in this study can be found in online repositories. The names of the repository/repositories and accession number(s) can be found in the article/supplementary material.
Ethics Statement
The animal study was reviewed and approved by Animal Use Ethics Commission (CEUA, Registration Number 66/11) of Federal University of Minas Gerais (UFMG, Belo Horizonte, Brazil).
Author Contributions
CC contributed to experimental work, data analysis, manuscript writing, and editing. BS contributed to experimental work, manuscript writing, and revision. PM-A contributed to experimental work and data analysis. VP, MZ-T, TP, VC, JS, and SL contributed to experimental work. VA contributed to conception and design of the study. AM contributed to conception and design of the study and manuscript revision. All authors contributed to the article and approved the submitted version.
Funding
This work was supported by the Coordenação de Aperfeiçoamento de Pessoal de Nível Superior (CAPES), Conselho Nacional de Desenvolvimento Científico e Tecnológico (CNPq), and Fundação de Amparo à Pesquisa do Estado de Minas Gerais (FAPEMIG).
Conflict of Interest
The authors declare that the research was conducted in the absence of any commercial or financial relationships that could be construed as a potential conflict of interest.
Acknowledgments
The authors would like to thank Dr. Kris Huygen of the Pasteur Institute of Brussels, Belgium, for having kindly provided the Ag85A antibody.
References
Almeida, J. F., Mariat, D., Azevedo, V., Miyoshi, A., de Moreno de LeBlanc, A., Del Carmen, S., et al. (2014). Correlation between fibronectin binding protein A expression level at the surface of recombinant Lactococcus lactis and plasmid transfer in vitro and in vivo. BMC Microbiol. 14:248. doi: 10.1186/s12866-014-0248-9
Andersen, P., Askgaard, D., Ljungqvist, L., Bennedsen, J., and Heron, I. (1991). Proteins released from Mycobacterium tuberculosis during growth. Infect. Immun. 59, 1905–1910. doi: 10.1128/IAI.59.6.1905-1910.1991
Cooper, A. M., Dalton, D. K., Stewart, T. A., Griffin, J. P., Russell, D. G., and Orme, I. M. (1993). Disseminated tuberculosis in interferon gamma gene-disrupted mice. J. Exp. Med. 178, 2243–2247. doi: 10.1084/jem.178.6.2243
Corthésy, B. (2013). Multi-faceted functions of secretory IgA at mucosal surfaces. Front. Immunol. 4:185. doi: 10.3389/fimmu.2013.00185
Del Carmen, S., Zurita-Turk, M., Lima, F. A., Santos, J. S. C., Leclercq, S. Y., Chatel, J. M., et al. (2013). A novel interleukin-10 DNA mucosal delivery system attenuates intestinal inflammation in a mouse model. Eur. J. Inflam. 11, 641–654. doi: 10.1177/1721727X1301100308
Dietrich, J., Lundberg, C. V., and Andersen, P. (2006). TB vaccine strategies-what is needed to solve a complex problem? Tuberculosis. 86, 163–168. doi: 10.1016/j.tube.2006.01.009
Dou, J., Wang, Y., Yu, F., Yang, H., Wang, J., He, X., et al. (2012). Protection against Mycobacterium tuberculosis challenge in mice by DNA vaccine Ag85A-ESAT-6-IL-21 priming and BCG boosting. Int. J. Immunogenet. 39, 183–190. doi: 10.1111/j.1744-313X.2011.01066.x
Dunham, S. P. (2002). The application of nucleic acid vaccines in veterinary medicine. Res. Vet. Sci. 73, 9–16. doi: 10.1016/S0034-5288(02)00032-2
Feldmann, M., and Maini, R. N. (2001). Anti-TNF-α therapy of rheumatoid arthritis: what have we learned? Annu. Rev. Immunol. 19, 163–196. doi: 10.1146/annurev.immunol.19.1.163
Green, M. R., and Sambrook, J. (2012). Molecular Cloning: A Laboratory Manual. New York, NY: Cold Spring Harbor.
Guimarães, V., Innocentin, S., Chatel, J. M., Lefèvre, F., Langella, P., Azevedo, V., et al. (2009). A new plasmid vector for DNA delivery using lactococci. Genet. Vaccines Ther. 7:4. doi: 10.1186/1479-0556-7-4
Harris, J., and Keane, J. (2010). How tumor necrosis factor blockers interfere with tuberculosis immunity. Clin. Exp. Immunol. 161, 1–9. doi: 10.1111/j.1365-2249.2010.04146.x
Innocentin, S., Guimarães, V., Miyoshi, A., Azevedo, V., Langella, P., Chatel, J. M., et al. (2009). Lactococcus lactis expressing either Staphylococcus aureus fibronectin-binding protein A or Listeria monocytogenes internalin A can efficiently internalize and deliver DNA in human epithelial cells. Appl. Environ. Microbiol. 75, 4870–4878. doi: 10.1128/AEM.00825-09
Jacobs, M., Brown, N., Allie, N., and Ryffel, B. (2000). Fatal Mycobacterium bovis BCG infection in TNF LT-alpha-deficient mice. Clin. Immunol. 94, 192–199. doi: 10.1006/clim.2000.4835
Kao, C. Y., Chen, Y., Thai, P., Whaci, S., Huang, F., Kim, C., et al. (2004). IL-17 markedly up-regulates β-defensin-2 expression in human airway epithelium via JAK and NF-κB signaling pathways. J. Immunol. 173, 3482–3491. doi: 10.4049/jimmunol.173.5.3482
Kaufmann, S. H., Evans, T. G., and Hanekom, W. A. (2015). Tuberculosis vaccines: time for a global strategy. Sci. Transl. Med. 7:276fs8. doi: 10.1126/scitranslmed.aaa4730
Kaufmann, S. H., Lange, C., Rao, M., Balaji, K. N., Lotze, M., Schito, M., et al. (2014). Progress in tuberculosis vaccine development and host-directed therapies—a state of the art review. Lancet Respir. Med. 2, 301–320. doi: 10.1016/S2213-2600(14)70033-5
Kutzler, M. A., and Weiner, D. B. (2008). DNA vaccines: ready for prime time? Nat. Rev. Genet. 9, 776–788. doi: 10.1038/nrg2432
Langella, P., Le Loir, Y., Ehrlich, S. D., and Gruss, A. (1993). Efficient plasmid mobilization by pIP501 in Lactococcus lactis subsp. lactis. J. Bacteriol. 175, 5806–5813. doi: 10.1128/JB.175.18.5806-5813.1993
Lyadova, I. V., and Panteleev, A. V. (2015). Th1 and Th17 cells in tuberculosis: protection, pathology, and biomarkers. Mediators Inflamm. 2015:854507. doi: 10.1155/2015/854507
Mancha-Agresti, P., de Castro, C. P., dos Santos, J. S. C., Araújo, M. A., Pereira, V. B., LeBlanc, J. G., et al. (2017). Recombinant invasive Lactococcus lactis carrying a DNA vaccine coding the Ag85A antigen increases INF-γ, IL-6, and TNF-α cytokines after intranasal immunization. Front. Microbiol. 8:1263. doi: 10.3389/fmicb.2017.01263
Mootoo, A., Stylianou, E., Arias, M. A., and Reljic, R. (2009). TNF-alpha in tuberculosis: a cytokine with a split personality. Inflamm. Allergy Drug Targets 8, 53–62. doi: 10.2174/187152809787582543
Mowat, A. M., and Agace, W. W. (2014). Regional specialization within the intestinal immune system. Nat. Rev. Immunol. 14, 667–685. doi: 10.1038/nri3738
Pereira, V. B., da Cunha, V. P., Preisser, T. M., Souza, B. M., Zurita-Turk, M., de Castro, C. P., et al. (2017). Lactococcus lactis carrying a DNA vaccine coding for the ESAT-6 antigen increases IL-17 cytokine secretion and boosts the BCG vaccine immune response. J. Appl. Microbiol. 22, 1657–1662. doi: 10.1111/jam.13449
Pereira, V. B., Saraiva, T. D., Souza, B. M., Zurita-Turk, M., Azevedo, M. S., De Castro, C. P., et al. (2015). Development of a new DNA vaccine based on mycobacterial ESAT-6 antigen delivered by recombinant invasive Lactococcus lactis FnBPA+. Appl. Microbiol. Biotechnol. 99, 1817–1826. doi: 10.1007/s00253-014-6285-3
Pontes, D., Innocentin, S., Del Carmen, S., Almeida, J. F., LeBlanc, J. G., Moreno de LeBlanc, A., et al. (2012). Production of fibronectin binding protein A at the surface of Lactococcus lactis increases plasmid transfer in vitro and in vivo. PLoS ONE. 7:e44892. doi: 10.1371/journal.pone.0044892
Pym, A. S., Brodin, P., Brosch, R., Huerre, M., and Cole, S. T. (2002). Loss of RD1 contributed to the attenuation of the live tuberculosis vaccines Mycobacterium bovis BCG and Mycobacterium microti. Mol. Microbiol. 46, 709–717. doi: 10.1046/j.1365-2958.2002.03237.x
Que, Y. A., François, P., Haefliger, J. A., Entenza, J. M., Vaudaux, P., and Moreillon, P. (2001). Reassessing the role of Staphylococcus aureus clumping factor and fibronectin-binding protein by expression in Lactococcus lactis. Infect. Immun. 69, 6296–6302. doi: 10.1128/IAI.69.10.6296-6302.2001
Rodriguez, A., Tjärnlund, A., Ivanji, J., Singh, M., García, I., Williams, A., et al. (2005). Role of IgA in the defense against respiratory infections IgA deficient mice exhibited increased susceptibility to intranasal infection with Mycobacterium bovis BCG. Vaccine. 23, 2565–2572. doi: 10.1016/j.vaccine.2004.11.032
Romano, M., D'Souza, S., Adnet, P. Y., Laali, R., Jurion, F., Palfliet, K., et al. (2006). Priming but not boosting with plasmid DNA encoding mycolyltransferase Ag85A from Mycobacterium tuberculosis increases the survival time of Mycobacterium bovis BCG vaccinated mice against low dose intravenous challenge with M. tuberculosis H37Rv. Vaccine 24, 3353–3364. doi: 10.1016/j.vaccine.2005.12.066
Schoen, C., Stritzker, J., Goebel, W., and Pilgrim, S. (2004). Bacteria as DNA vaccine carriers for genetic immunization. Int. J. Med. Microbiol. 294, 319–335. doi: 10.1016/j.ijmm.2004.03.001
Souza, B. M., Preisser, T. M., Pereira, V. B., Zurita-Turk, M., de Castro, C. P., da Cunha, V. P., et al. (2016). Lactococcus lactis carrying the pValac eukaryotic expression vector coding for IL-4 reduces chemically-induced intestinal inflammation by increasing the levels of IL-10-producing regulatory cells. Microb. Cell Fact. 15, 150–168. doi: 10.1186/s12934-016-0548-x
Tsai, H. C., Velichko, S., Hung, L. Y., and Wu, R. (2013). IL-17A and Th17 cells in lung inflammation: an update on the role of Th17 cell differentiation and IL-17R signaling in host defense against infection. Clin. Dev. Immunol. 2013:267971. doi: 10.1155/2013/267971
van de Vosse, E., Hoeve, M. A., and Ottenhoff, T. H. (2004). Human genetics of intracellular infectious diseases: molecular and cellular immunity against mycobacteria and salmonellae. Lancet Infect. Dis. 4, 739–749. doi: 10.1016/S1473-3099(04)01203-4
Wang, Q. L., Pan, Q., Ma, Y., Wang, K., Sun, P., Liu, S., et al. (2009). An attenuated Salmonella-vectored vaccine elicits protective immunity against Mycobacterium tuberculosis. Vaccine. 27, 6712–6722. doi: 10.1016/j.vaccine.2009.08.096
Wells, J. (2011). Mucosal vaccination and therapy with genetically modified lactic acid bacteria. Annu. Rev. Food Sci. Technol. 2, 423–445. doi: 10.1146/annurev-food-022510-133640
Williams, A., Reljic, R., Naylor, I., Clark, S. O., Falero-Diaz, G., Singh, M., et al. (2004). Passive protection with immunoglobulin A antibodies against tuberculosis early infection of the lungs. Immunology 111, 328–333. doi: 10.1111/j.1365-2567.2004.01809.x
Xu, J., Xu, W., Chen, X., Zhao, D., and Wang, Y. (2008). Recombinant DNA vaccine of the early secreted antigen ESAT-6 by Mycobacterium tuberculosis and Flt3 ligand enhanced the cell-mediated immunity in mice. Vaccine 26, 4519–4525. doi: 10.1016/j.vaccine.2008.06.044
Keywords: lactic acid bacteria, Lactococcus lactis, DNA vaccine, tuberculosis, ESAT6/Ag85A
Citation: de Castro CP, Souza BM, Mancha-Agresti P, Pereira VB, Zurita-Turk M, Preisser TM, da Cunha VP, dos Santos JSC, Leclercq SY, Azevedo V and Miyoshi A (2021) Lactococcus lactis FNBPA+ (pValac:e6ag85a) Induces Cellular and Humoral Immune Responses After Oral Immunization of Mice. Front. Microbiol. 12:676172. doi: 10.3389/fmicb.2021.676172
Received: 05 March 2021; Accepted: 23 April 2021;
Published: 20 May 2021.
Edited by:
Silvia Beatriz Boscardin, University of São Paulo, BrazilReviewed by:
José Ronnie Carvalho Vasconcelos, Federal University of São Paulo, BrazilYing Xu, Fudan University, China
Copyright © 2021 de Castro, Souza, Mancha-Agresti, Pereira, Zurita-Turk, Preisser, da Cunha, dos Santos, Leclercq, Azevedo and Miyoshi. This is an open-access article distributed under the terms of the Creative Commons Attribution License (CC BY). The use, distribution or reproduction in other forums is permitted, provided the original author(s) and the copyright owner(s) are credited and that the original publication in this journal is cited, in accordance with accepted academic practice. No use, distribution or reproduction is permitted which does not comply with these terms.
*Correspondence: Camila Prósperi de Castro, Y2FtaWxhcHJvc3BlcmljJiN4MDAwNDA7Z21haWwuY29t
†These authors have contributed equally to this work