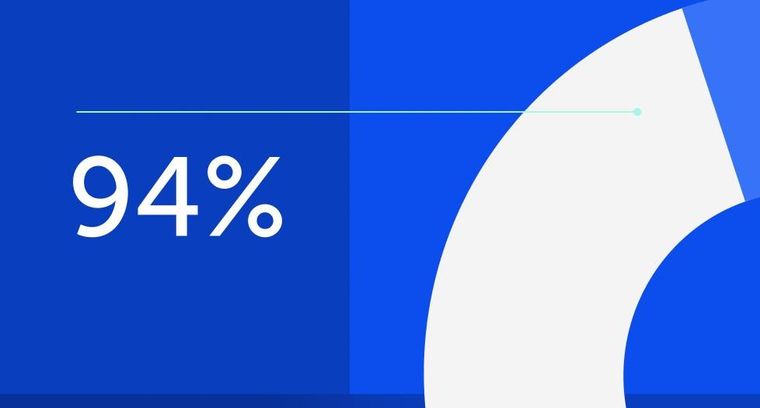
94% of researchers rate our articles as excellent or good
Learn more about the work of our research integrity team to safeguard the quality of each article we publish.
Find out more
ORIGINAL RESEARCH article
Front. Microbiol., 16 June 2021
Sec. Microbiotechnology
Volume 12 - 2021 | https://doi.org/10.3389/fmicb.2021.675763
This article is part of the Research TopicBiological Strategies to Enhance the Anaerobic Digestion Performance: Fundamentals and Process Development View all 10 articles
Volatile fatty acid accumulation is a sign of digester perturbation. Previous work showed the thermodynamic limitations of hydrogen and CO2 in syntrophic propionate oxidation under elevated partial pressure of CO2 (pCO2). Here we study the effect of directional selection under increasing substrate load as a strategy to restructure the microbial community and induce cross-protection mechanisms to improve glucose and glycerol conversion performance under elevated pCO2. After an adaptive laboratory evolution (ALE) process, viable cell density increased and predominant microbial groups were modified: an increase in Methanosaeta and syntrophic propionate oxidizing bacteria (SPOB) associated with the Smithella genus was found with glycerol as the substrate. A modest increase in SPOB along with a shift in the predominance of Methanobacterium toward Methanosaeta was observed with glucose as the substrate. The evolved inoculum showed affected diversity within archaeal spp. under 5 bar initial pCO2; however, higher CH4 yield resulted from enhanced propionate conversion linked to the community shifts and biomass adaptation during the ALE process. Moreover, the evolved inoculum attained increased cell viability with glucose and a marginal decrease with glycerol as the substrate. Results showed differences in terms of carbon flux distribution using the evolved inoculum under elevated pCO2: glucose conversion resulted in a higher cell density and viability, whereas glycerol conversion led to higher propionate production whose enabled conversion reflected in increased CH4 yield. Our results highlight that limited propionate conversion at elevated pCO2 resulted from decreased cell viability and low abundance of syntrophic partners. This limitation can be mitigated by promoting alternative and more resilient SPOB and building up biomass adaptation to environmental conditions via directional selection of microbial community.
Volatile fatty acids (VFA) are important chemical building blocks obtained by mixed culture fermentation in the carboxylate platform (Holtzapple and Granda, 2009; Agler et al., 2011). However, its accumulation is a symptom of reactor malfunctioning in anaerobic digestion (AD), attributed to dissimilarities between acidogenic, acetogenic, and methanogenic bio-conversion rates (Hickey and Switzenbaum, 1991). The identified causes leading to VFA accumulation are associated with substrate overload (Amorim et al., 2018), possible toxicity due to increasing concentrations of undissociated acids at low pH (Russell and Diez-Gonzalez, 1998), and disparities in the proportionality of acidogenic, syntrophic, and methanogenic microorganisms (McMahon et al., 2004; Li et al., 2016; Town and Dumonceaux, 2016). When VFA production is targeted under anaerobic conditions, the product spectrum selectivity is influenced by operational parameters such as temperature, substrate type, concentration, pH, solids retention time (SRT) and headspace composition (Arslan et al., 2016; Zhou et al., 2018; Wainaina et al., 2019).
Possible steering effects of headspace composition in AD could be magnified applying the auto-generative high-pressure AD technology, which was developed for simultaneous biogas production and upgrading (Lindeboom et al., 2011; Lemmer et al., 2015). Here, by applying a closed gastight vessel, the reactor pressure auto-generatively increases and a higher CH4 content in the biogas is achieved due to enlarged differences in CO2 and CH4 solubility at high operational pressure. Lindeboom et al. (2013) observed a decline in the propionate oxidation rate at an increased total pressure with a concomitantly increased partial pressure of CO2 (pCO2). As part of the explanatory mechanism, an acidification process and reversible toxicity linked to carbamate formation were proposed. Both processes occur due to increased aqueous CO2 concentration (H2CO3∗) in the liquid medium resulting from enhanced CO2 dissolution (Lindeboom et al., 2016). Recently, we investigated additional effects of elevated pCO2 on syntrophic conversions occurring in AD (Ceron-Chafla et al., 2020). The observed limited propionate and butyrate conversion under elevated pCO2 were explained by a more comprehensive mechanism that encompasses bioenergetics, kinetic and physiological effects.
The effects of elevated pCO2 on the cell viability level can be attributed to the detrimental effect that increased H2CO3∗ concentrations have on cell membrane permeability. Leakage of internal components, structural modifications, and internal acidification are part of the explanatory mechanisms (Wu et al., 2007; Garcia-Gonzalez et al., 2010). In bioreactors with a pressurized headspace, the effects of elevated pCO2 differ from inert gases, such as N2, which does not severely compromise cell viability (Wu et al., 2007). Considerably higher hydrostatic pressures of N2 are required to achieve the same inactivation levels as with elevated pCO2 (Aertsen et al., 2009). Safeguarding cell viability is necessary since reduced cell density and increased percentage of permeable cells might result in impaired specific conversion rates, causing decreased productivity.
The extent of detrimental effects of increased dissolved CO2 concentrations on cell membranes depends on localized conditions. Some microbial species have acid tolerance mechanisms involving enzymatic systems carrying out neutralization reactions, proton pumps and modifications in the cell membrane (Guan and Liu, 2020). In consequence, they can counteract ramping H+ concentrations due to H2CO3∗ intracellular dissociation. Some authors observed during sterilization experiments that a reduction in water activity in the liquid medium decreases CO2 dissolution thus diminishing its intracellular diffusion (Kumagai et al., 1997; Chen et al., 2017). In other cases, microorganisms induce the synthesis of compounds acting as compatible solutes, such as glutamate, which help to tackle changes in osmolarity and CO2 toxicity (Oger and Jebbar, 2010; Park et al., 2020). Additionally, the presence of fats in the medium affects the porosity and structure of the cell wall or membranes, thus limiting CO2 penetration (Lin et al., 1994).
Previous studies have shown that directional selection of microbial community through adaptive laboratory evolution (ALE) processes is highly effective to improve stress tolerance and selectively enhance product formation by activation of downregulated pathways (Portnoy et al., 2011; Dragosits and Mattanovich, 2013). However, in mixed culture fermentations changes in pathway predominance to enhance hydrogen production already have been observed after relatively short adaptive evolution processes (46 days) (Huang et al., 2016). In particular, ALE has shown to trigger the development of features such as acid resistance mechanisms (Kwon et al., 2011), as well as other physiological mechanisms to conserve cell membrane integrity. Such insights could act as cross-protection mechanisms against stressful pCO2 levels and improve metabolic activity.
Elevated pCO2 has also shown effects at the ecological level in anaerobic communities. In terms of structure and taxonomic diversity, the overall response to high CO2 concentrations included a decrease in richness and lowering diversity, dependent on the actual prevailing pH (Gulliver et al., 2014; Fazi et al., 2019). Moreover, there is evidence of shifts in bacterial and archaeal predominant groups since CO2 can be incorporated as a reactant to a different extent and consequently, enhances the metabolic pathways in a selective manner (Gulliver et al., 2014; Yu and Chen, 2019). Therefore, the modifying effect of elevated pCO2 in highly redundant communities, such as the ones from anaerobic digesters, might redefine the metabolic activity output and overall process performance.
Elevated CO2 concentrations could steer specific metabolic pathways in anaerobic conversion systems, particularly those reactions coupled to the phosphoenolpyruvate (PEP)–pyruvate–oxaloacetate (OAA) node in the biochemical conversions. In addition, high pCO2 could promote carboxylation reactions, i.e., propionate formation is favored over acetate formation since the latter will require a decarboxylation reaction of acetyl-CoA (Baez et al., 2009). The enhancement of methane production at high CO2 levels could occur due to the combination of homoacetogenic activity coupled with aceticlastic methanogenesis when an electron sink is required (Bajón Fernández et al., 2019). Otherwise, under the stoichiometric provision of a suitable electron donor, such as hydrogen or formate, it will directly promote hydrogenotrophic methanogenesis (Zabranska and Pokorna, 2018).
Glucose and glycerol conversion, under anaerobic conditions, share the glycolytic pathway after glycerol has been converted to glyceraldehyde-3-phosphate and subsequently to PEP (Saint-Amans et al., 2001) (Supplementary Figure 1). These substrates differ in their degree of reduction: for glycerol, this value corresponds to –0.67 electron equivalents/C-mol, whereas for glucose this value is 0. Due to this, glycerol fermentation leads to the formation of reduced compounds and less acetate and CO2 as in the case of Propionibacterium acidipropionici (Zhang et al., 2016). With the formation of more reduced fermentation products, the redox balance is maintained and biomass growth and overall productivity are sustained (Himmi et al., 2000). In terms of biomass yields, the substrates also have contrasting differences: the theoretical values are higher for glucose than for glycerol being 0.239 vs. 0.145 C-mol biomass/C-mol electron donor, respectively (Heijnen and Kleerebezem, 2010). Moreover, the enzymatic activity associated with CO2 fixation also differs in cells grown in glycerol due to the expression of pyruvate carboxylase, which has not been observed with glucose (Parizzi et al., 2012).
These fundamental differences between glucose and glycerol fermentation make a comparative study highly relevant to elucidate the potential of elevated pCO2 as a steering parameter in anaerobic conversions when performance limitations need to be overcome. Therefore, in this work, we applied a directional selection process based on increasing substrate load to restructure the microbial community and activate cross-protection mechanisms to enhance the anaerobic conversion of glucose and glycerol under elevated pCO2. As a negative control, we investigated the effect of elevated pCO2 on the original inoculum. We expected differences in the product spectrum as a result of the dissimilar substrate oxidation state and increased pCO2 favoring propionate production. Nonetheless, further propionate oxidation (Pr-Ox) would be limited due to thermodynamic constraints on syntrophic Pr-Ox in relation to interspecies hydrogen transfer (Stams et al., 1998). Further constraints will be established due to the negative effects of elevated pCO2 on cell viability and relative abundance of methanogens and syntrophic propionate oxidation bacteria (SPOB) in the original inoculum. The evolved inoculum would feature enhanced cell viability, a higher proportion of fermenters and more resilient SPOB and methanogenic groups. These factors could help to circumvent thermodynamic and performance limitations present in the original inoculum, thereby enabling propionate conversion under elevated pCO2.
Flocculent anaerobic sludge from an anaerobic membrane bioreactor (AnMBR) treating wastewater from a chocolate and pet food industry was used as the starting inoculum. Measured physicochemical parameters are presented in Table 1.
Table 1. Physicochemical characterization of the original anaerobic inoculum used for the experiments of glucose and glycerol conversion under elevated pCO2 and evolved inoculum after adaptive laboratory evolution (ALE) using glucose and glycerol (1 g COD L–1) at T = 35°C, initial pCO2 = 0.3 bar and initial pH in the range 7.5–8.0.
Batch experiments with original inoculum were conducted at four different pCO2, namely 0.3, 3, 5, and 8 bar initial pressures. Schott bottles with a working volume of 250 mL, air-tight sealed with rubber stoppers were used for the experiments at atmospheric conditions, i.e., 0.3 bar pCO2. The employed gas to liquid ratio was 2:3. Stainless steel reactors working in a pressure range of 1–600 bar (Nantong Vasia, China) were used for the experiments at moderately elevated pressure, i.e., 3, 5, and 8 bar. These reactors are fitted with gas and liquid sampling ports in the head, as well as a glycerin manometer. The working liquid volume, in this case, was 120 mL, and the same gas to liquid ratio as before was kept. Reactors were inoculated with 2 g VSS L–1. The liquid medium added to each reactor contained 1 g glucose or glycerol as COD L–1, macronutrients, micronutrients both prepared according to García Rea et al. (2020) and buffer solution (100 mM as HCO3–). The initial pH of all the reactors was not adjusted and values were in the range 7.5–8.0.
After filling and closure, atmospheric reactors were flushed for 2 min with 100% N2 and sequentially with N2:CO2, 70:30%. Pressure reactors were initially flushed with the same gas mixture as the atmospheric reactors and afterward, consecutive pressurization-release cycles with >99% CO2 were applied to ensure initial headspace composition. Reactors were operated for approximately 10 days, kept at 35°C and continuously shaken at 110 rpm. All the experimental treatments were conducted in triplicates. Pressurized controls with only nitrogen in the headspace were additionally included. To diminish sampling interference during the experiment, we applied the same sampling strategy as previously described (Ceron-Chafla et al., 2020). A graphical description of the experimental design is presented in Figure 1.
Figure 1. Graphical summary of experimental conditions for the anaerobic conversion experiments at elevated pCO2 using glucose and glycerol as substrates. Experiments are organized according to substrate and inoculum conditions.
The total length of the atmospheric ALE process was 61 days divided into four cycles with duration as follows: the two first cycles lasted 7 days, which corresponded to complete substrate conversion for initial atmospheric experiments at 0.3 bar pCO2. However, due to the limited growth and conversion of intermediates identified after the second cycle, it was decided to perform the third and fourth cycles at 0.3 bar pCO2 for 21 and 26 days, respectively, to achieve complete conversion. Schott bottles with a working volume of 2,000 mL were used for the ALE incubation. After every cycle, 240 mL were removed, replaced by fresh medium and the bottles were flushed with N2:CO2, 70:30%. The substrate concentration in the medium refreshing solution was fixed for all the cycles at 1 g COD L–1 glucose or glycerol. It should be noted that owing to the serial dilution procedure, biomass was exposed to a deliberately increased substrate load per cycle. The evolved inoculum was harvested after the fourth cycle, employing low-speed centrifugation and resuspension with macro and micronutrient solution. After this, the obtained biomass was characterized in terms of physicochemical parameters (Table 1) and used to inoculate pressure reactors at 5 bar pCO2 and controls at 5 bar pN2 to evaluate anaerobic conversion performance of evolved microbial biomass at a higher Food to Mass ratio (F:M ratio) (phase 3; Figure 1). The pressurized experiments with evolved inoculum lasted approximately 10 days.
Biomass samples stored at –80°C from the endpoint of the batch experiments were used to evaluate Microbial community dynamics. After thawing, DNA was extracted according to the instructions included in the DNeasy UltraClean Microbial Kit (Qiagen, Germany). The quality and quantity of the obtained DNA were checked through Qubit® 3.0 DNA detection (Qubit dsDNA HS Assay Kit, Life Technologies, United States). Library construction and sequencing by the Illumina platform were conducted by Novogene (Hong Kong). A summary of the internal protocol is presented in Supplementary Information.
Secondary metabolites in the liquid medium (acetate, propionate, butyrate, and valerate) were measured by gas chromatography (7890A GC; Agilent Technologies, United States) according to the method described by Muñoz Sierra et al. (2020). The gas composition of samples stabilized at atmospheric conditions was determined via gas chromatography (7890A GC; Agilent Technologies, United States) using a thermal conductivity detector operated at 200°C and oven temperature ramping from 40 to 100°C. The system operated with two columns: an HP-PLOT Molesieve GC Column 30 m × 0.53 mm × 25.00 μm and an HP-PLOT U GC Column, 30 m, 0.53 mm, and 20.00 μm (Agilent Technologies, United States). The carrier gas was helium at a constant flow rate of 10 mL min–1.
Total cell numbers and live/dead cells were assessed by flow cytometry (BD Accuri® C6, BD Biosciences, Belgium) using Milli-Q as sheath fluid. Before measurement, samples were pre-treated as follows: First, samples were diluted (×500) with 0.22-μm filtered phosphate-buffered-saline (PBS) solution. Diluted samples were sonicated in three cycles of 45 s at 100 W and the amplitude at 50%. Subsequently, samples were diluted (×500) and filtered at 22 μm. Immediately after the pre-treatment, the samples were stained with 5% SYBR® Green I or SYBR® Green I combined with propidium iodide (Invitrogen) and incubated at 37°C for 10 min.
pH, total and soluble COD, TSS, and VSS, ammonium and total phosphorus were measured according to standard methods (American Public Health Association, 2005).
Statistical analyses were carried out in the R software (R Core Team, 2019). After processing the amplicon sequencing data, a table was generated with relative abundances of the different OTUs and their taxonomic assignment of each sample. Normalization of the samples was carried out based on the flow cytometry data (Props et al., 2017). The R packages vegan (Oksanen et al., 2016) and phyloseq (McMurdie and Holmes, 2013) were used for community analysis. Significant differences (p < 0.05) in microbial community composition were identified employing pair-wise Permutational ANOVA (PERMANOVA) with Bonferroni correction using the adonis function included in the vegan package.
The order-based Hill’s numbers were used to evaluate the alpha diversity in terms of richness (number of OTUs, H0), the exponential of the Shannon diversity index (H1) and the Inverse Simpson index (H2) (Hill, 1973). Beta diversity was evaluated via the Bray–Curtis distance measure (Bray and Curtis, 1957). Spearman’s correlation analysis was performed using the function cor.test ().
The main results of the glucose and glycerol conversion experiments at elevated pCO2 using the evolved and original inoculum are summarized in Table 2. Observations are categorized in terms of cell viability, microbial community, and product spectrum and explained in detail in the following sections.
Table 2. Summary of the observed effects of the Adaptive Laboratory Evolution (ALE) strategy in the performance of anaerobic conversion of glucose and glycerol under 5 bar pCO2.
The total and viable cell density of the starting inoculum is presented in Figure 2A. During the experiments of glucose conversion at pCO2 of 0.3 bar using this inoculum, there was a negligible reduction in the final viable cell density, expressed as percentage change, after 10 days (Figure 2B). However, at moderately high pressures, i.e., 3, 5, and 8 bar initial pCO2, the treatments showed a more substantial decrease of approximately 66% in viable cell density for the same period (Figure 2B). It should be noted that nitrogen controls at 5 bar showed a comparable decrease of 73% in viable cell density.
Figure 2. (A) Total and viable cell density for the original and evolved inoculum with glucose and glycerol. Percentage of change of total and viable cell density for the (B) glucose and (C) glycerol conversion experiments at different initial pCO2 in the headspace (0.3, 3, 5, and 8 bar) using original inoculum and at 5 bar initial pCO2 using evolved inoculum. Pressure controls with N2 headspace for the original and evolved inoculum at 5 bar (5C and E5C in the graphs) are included for reference.
Total and viable cell density increased in comparison to the original inoculum after the ALE cycle using glucose as a substrate. In particular, there was a 2.2-fold increase in viable cell density in comparison to the original inoculum (Figure 2A). After the exposure to 5 bar pCO2, the viable cell density of the evolved inoculum showed an increase of 163% compared to the original inoculum. Nitrogen controls at 5 bar presented a smaller increase of 67% in viable cell density after 10 days (Figure 2B).
The proportion of bacteria and archaea, based on the total number of processed reads, corresponded to 79 and 21%, respectively, in the original inoculum. The bacterial community of the original inoculum was majorly composed of the phyla Chloroflexi (52%), Actinobacteriota (22%), Firmicutes (10%), and Proteobacteria (5%). In terms of the relative abundance of the bacterial community at the genus level, there were a representative proportion of SJA-15_ge (35%) and unclassified Micrococcales (19%) (Figure 3A). The proportion of genera associated with syntrophic Pr-Ox namely Smithella was low (<1%). The archaeal community was mainly composed of members of the aceticlastic genus Methanosaeta (68%) and the hydrogenotrophic genus Methanobacterium (31%) (Figure 3B).
Figure 3. Heatmap presenting the relative abundance of (A) Bacterial community and (B) Archaeal community at the genus level. Experimental treatments correspond to glucose conversion at elevated pCO2 using original (0.3, 3, 5, and 8 bar) and evolved inoculum (5 bar–E5). I corresponds to the original inoculum and E to the evolved inoculum. Pressure corresponds to initial values before equilibrium.
In the experiments at 3, 5, and 8 bar initial pCO2, bacterial genera from the class Anaerolineae: SJA-15_ge (38–45%), unclassified Micrococcales (11–16%) were predominant (Figure 3A). The relative abundance of syntrophic groups remained low (<1%). The proportion of total archaea was below 45% in all treatments. Additionally, Figure 3B suggests a contrasting relationship in the relative abundance of Methanobacterium and Methanosaeta in the treatments when compared to the original inoculum.
After the ALE cycles, the proportion of bacteria and archaea was 70 and 30%, respectively. The directional selection of microbial community favored the relative abundance of bacterial phyla Actinobacteria, increasing its abundance to 48% and decreased the proportion of Chloroflexi to 20% of total bacterial reads for this inoculum. At the genus level, the relative abundance of unclassified Micrococcales and SJA-15_ge corresponded to 44 and 12%, respectively (Figure 3A). Smithella increased to 4% of the total bacterial reads. At the archaeal level, the proportions of the two methanogenic genera Methanosaeta and Methanobacterium corresponded to 68 and 31% of the archaeal reads, respectively (Figure 3B).
For the experiments at 5 bars initial pCO2 with evolved inoculum, at the genus level, unclassified Micrococcales remained predominant (28%), as well as SJA-15_ge (15%) (Figure 3A). Under the imposed experimental conditions, the abundance of syntrophic groups, e.g., Smithella (Figure 3A) and Syntrophobacter, cumulatively increased to 8% of the total bacterial reads. The proportion of total archaea after exposure to pCO2 corresponded to 39% of the total reads. The relative abundance of Methanosaeta increased to 77%, whereas Methanobacterium remained around 22% of total processed archaeal reads (Figure 3B).
The product spectrum of the anaerobic conversion of glucose under different initial pCO2 levels was mainly composed of propionate, acetate, butyrate and CH4. Propionate production was predominant under initial pCO2 of 0.3 bar, reaching 399 mg COD–Pr L–1, which in terms of the mass balance corresponded to 44% of the initial COD (Figure 4A). In the experiments at 3, 5, and 8 bar pCO2, propionate production peaked at approximately 407 ± 32 mg COD–Pr L–1, which accounted for 38% of the initial COD. Small discrepancies in the total amount being fed to atmospheric and pressure reactors were experienced since the effective volume differed among reactors to keep the liquid to gas ratio comparable. Acetate and butyrate amounts were higher at initial pCO2 of 8 bar compared to atmospheric conditions, whereas at 3 and 5 bar a noteworthy accumulation of these metabolites was not detected (Figures 4B–D). Propionate conversion was hindered by elevated pCO2 and in consequence, decreased CH4 production was observed in the treatments at high initial pCO2. The experiments at 3, 5, and 8 bar pCO2 resulted in an average 30% decrease in the final amount of COD–CH4 produced in comparison to the atmospheric control at 0.3 bar pCO2 evaluated after 10 days (Figures 4A–D).
Figure 4. Volatile fatty acid (VFA) and CH4 production (mg COD) over time for the glucose conversion experiments using original inoculum at initial pCO2 of (A) 0.3 bar, (B) 3 bar, (C) 5 bar, and (D) 8 bar. Pressure corresponds to initial values before equilibrium. Data points represent experimental data. Bars represent the standard deviation of three biological replicates measured at the beginning, middle, and end of the experiment.
Figure 5A shows the composition of the product spectrum in the experiments with evolved inoculum which remained similar to the experiments with original inoculum; however, less accumulation of intermediates, particularly propionate, was detected. Propionate production peaked after 92 h (Supplementary Figure 6) and it was no longer detected at significant amounts at the end of the experiment (Supplementary Table 1). A preliminary 33% decrease in the final COD–CH4 was calculated when comparing to the treatment at the same pCO2, i.e., 5 bar, using the original inoculum (Figure 4C).
Figure 5. Volatile fatty acid and CH4 production (mg COD) over time for (A) glucose and (B) glycerol conversion experiments at elevated pCO2 using evolved inoculum at 5 bar initial pCO2. Pressure corresponds to initial values before equilibrium. Data points represent experimental data. Bars represent the standard deviation of three biological replicates measured at the beginning, middle, and end of the experiment.
In the experiments of glycerol conversion at elevated pCO2 using the starting inoculum at 0.3 bar pCO2, there was a negligible 10% increase in final viable cell density compared to initial conditions. However, at initial pCO2 of 3, 5, and 8 bar there was an increase of approximately 50% for the two lowest pressures and a comparable percentage decrease for the highest pCO2. Nitrogen controls at 5 bar did not present a considerable change in viable cell density, just accounting for a 6% increase (Figure 2C).
After the ALE process with increasing glycerol load, there was a 5.2-fold increase in viable cell density in comparison to the original inoculum and a 0.9-fold increase compared to the ALE with glucose as substrate (Figures 2A,B). After exposure to 5 bar pCO2, the evolved inoculum showed a negligible 5% decrease in viable cell density. Nitrogen controls at 5 bar showed a 20% decrease compared to the initial conditions after 10 days (Figure 2C).
In the experiments with the original inoculum at 3, 5, and 8 bar initial pCO2, results showed a predominance of bacterial genus SJA-15_ge, whose relative abundance calculated based on processed reads varied between 34 and 42%, and other genera, such as Clostridium_sensu_stricto_12 (8–12%), as well as unclassified Micrococcales and Mesotoga with relative abundances <14% (Figure 6A). The relative abundance of SPOB remained low (<1%) in all cases. There was a descending trend regarding the changes in the proportion of total archaea for the high pCO2 experiments using the original inoculum: for experiments at 3, 5, and 8 bar pCO2, the archaeal presence corresponded to 35, 22, and 18% of the total number of processed reads, respectively. Methanosaeta had the highest relative abundance at the genus level, varying between 58 and 82%, while Methanobacterium ranged between 17 and 41% of total processed archaeal reads (Figure 6B).
Figure 6. Heatmap presenting the relative abundance of (A) Bacterial community and (B) Archaeal community at the genus level. Experimental treatments correspond to glycerol conversion at elevated pCO2 using original (0.3, 3, 5, and 8 bar) and evolved inoculum (5 bar–E5). I corresponds to the original inoculum and E to the evolved inoculum. Pressure corresponds to initial values before equilibrium.
After ALE with glycerol, the proportion of Bacteria and Archaea corresponded to 81 and 19% of processed reads, respectively. The bacterial community composition was dominated by phyla Chloroflexi (35%), Actinobacteriota (19%), Desulfobacterota (14%), and Synergistota (14%). At the genus level, SJA-15 (27%), unclassified Micrococcales (17%), Smithella (13%), and Thermovirga (11%) showed the highest relative abundances (Figure 6A). The archaeal community was majorly composed of genera Methanosaeta and Methanobacterium at a corresponding relative abundance of 76 and 22% (Figure 6B).
For the experiments at 5 bar pCO2 with evolved inoculum, the relative abundance of SJA-15_ge decreased to 19% and Clostridium_sensu_stricto_12 (21%) was predominant. At this condition, the relative abundance of syntrophic groups, e.g., Smithella (Figure 6A) and Syntrophobacter, cumulatively increased to 18%. The proportion of total archaea, in this case, showed an increase to 35% of total processed reads. In terms of community composition, it differed from the glucose experiments: a slightly higher proportion of Methanosaeta (89%) was observed, whereas Methanobacterium represented 10% of processed archaeal reads (Figure 6B).
A basic analysis of alpha diversity via calculation of the Hill numbers showed an overall decrease in the richness (H0) of the bacterial community associated with the directional selection process at increasing substrate concentrations, which seemed to be more noticeable in the case of glycerol compared to glucose (Supplementary Figure 2). The inoculum condition (original vs. evolved) only exposed significant differences in terms of the bacterial community structure for the case of richness, H0 (p = 0.032) when all treatments were analyzed together. In the case of Pielou’s evenness, significant differences were explained by the type of substrate (p = 0.044) and not by elevated pCO2.
Beta diversity analysis via calculation of the Bray Curtis distance measures revealed significant differences in the community at the highest taxonomic level (Kingdom) because of exposure to elevated pCO2 (p = 0.01 and p = 0.04, respectively). The inoculum condition was significant only to explain the variability of the bacterial community (p = 0.07) among all experimental treatments.
The glycerol anaerobic conversion experiments showed a similar final product spectrum to glucose in terms of VFA. The difference was observed in the overall production: propionate in each condition of glycerol fermentation was around two times higher than during glucose fermentation and butyrate production was, on average, four times lower. Under atmospheric conditions, propionate production reached 600 mg COD–Pr L–1 at 0.3 bar pCO2. In terms of the mass balance, this accounts for 67% of the initial COD (Figure 7A). In the elevated pCO2 experiments, the propionate concentration reached its plateau around 647 ± 41 mg COD–Pr L–1, corresponding in mass to 57–66% of the initial COD input. Similar to the glucose experiments, propionate conversion was seemingly affected by elevated pCO2 leading to its accumulation after approximately 70 h and until the end of the experimental period (Figures 7B–D). Consequently, CH4 production was majorly impacted in the glycerol treatments at elevated pCO2. On average, methane production was lowered by 69%, compared to the atmospheric control at 0.3 bar pCO2. CH4 production in the pressurized treatments was, on average, 48% lower in the case of glycerol compared to glucose.
Figure 7. Volatile fatty acid and CH4 production (mg COD) over time for the glycerol conversion experiments using original inoculum at initial pCO2 of (A) 0.3 bar, (B) 3 bar, (C) 5 bar, and (D) 8 bar. Pressure corresponds to initial values before equilibrium. Data points represent experimental data. Bars represent the standard deviation of three biological replicates measured at the beginning, middle and end of the experiment.
In the case of glycerol conversion at 5 bar pCO2 using evolved inoculum, propionate peaked after 69 h (Supplementary Figure 6) and decreased until complete conversion was observed by the end of the experiment (Figure 5B). An increment of 55% in final CH4 production was achieved when compared to the treatments at the same pCO2 using the original inoculum (Figure 7C). Contrary to what was observed with the original inoculum experiments, CH4 production from glycerol with evolved inoculum was 23% higher than from glucose at 5 bar pCO2.
The processed sequencing data were used to estimate the proportions of bacteria and archaea in the incubations at atmospheric and pressurized conditions with glucose and glycerol. These proportions together with the results of FCM analysis were used to estimate the theoretical CH4 yield in ng of COD per viable archaeal cell for the experimental treatments (Figure 8). Based on these results, we calculated that in the 5 bar pCO2 experiment using evolved inoculum, the CH4 yield per viable archaeal cell was approximately 2.6 and 4.4 times higher compared to the same condition using the original inoculum for glucose and glycerol, respectively. When comparing glucose and glycerol incubations with evolved inoculum and 5 bar pCO2, the CH4 yield per cell in the incubation with glycerol was slightly higher than with glucose (1.3 times), which contrasts with the results of the original inoculum. In all cases, elevated pCO2 treatments evidenced lower CH4 yield than pN2 controls. The increased CH4 production is likely associated with changes in total archaeal proportion, resulting from the directional microbial community selection process. Furthermore, enhanced product formation is remarkable, since an elevated pCO2 of 5 bar seemed to constrain cell growth in the case of the glycerol evolved inoculum (Figure 2C), but not for glucose (Figure 2B). This might suggest the development of different stress-response strategies to elevated pCO2 depending on the type of substrate and selected microbial community. Moreover, the relative abundance of Smithella + Syntrophobacter is 2.3 times higher in glycerol than glucose evolved inoculum (Figures 3A, 6A), which in turn might help to explain higher CH4 production due to community and pathway selection despite limited growth. It is postulated that due to the ALE process, a higher F:M ratio employed during the elevated pCO2 experiments with evolved inoculum did not constrained bioconversion activity. The F:M ratio was 4–5 times higher in elevated pCO2 experiments with evolved inoculum (phase 3, Figure 1) compared to original inoculum (phase 1, Figure 1). However, due to the differences in viable cell concentration, the substrate loads (calculated as mg COD per viable cell), were actually 10 and three times higher compared to the ones using original inoculum for glucose and glycerol, respectively. These differences at the “biomass” and “cell” level could have caused additional inhibition of the biochemical activity of the evolved inoculum, however, results indicate that the ALE process selected for more resilient microorganisms able to cope with the imposed conditions.
Figure 8. CH4 yield normalized by the viable archaeal cell density at the end of the experiment for the glucose and glycerol conversion experiments at elevated pCO2 using original inoculum and evolved inoculum at 5 bar. Pressure corresponds to initial values before equilibrium. Nitrogen controls (5 bar) are included as a reference.
From the directional selection of microbial community via ALE, the three main achievements were: (i) an increase in the overall total and viable cell density, (ii) a higher proportion of archaea compared to the original inoculum for glucose and (iii) a larger proportion of SPOB for both substrates (Table 2). These achievements provide a reasonable explanation for enabling propionate conversion and consequently improving CH4 production under elevated pCO2.
The ALE process could have contributed to the development of protective mechanisms associated with the conservation of cell membrane integrity. It is known that changes in environmental conditions, such as temperature and osmotic pressure, trigger modifications in the structure and fluidity of cell membranes (Beney and Gervais, 2001). Bacteria and archaea differ in the chemical composition of their membrane lipids (Albers and Meyer, 2011), which confers them a distinctive degree of protection toward changes in total pressure and pCO2. If membrane fluidity and permeability are being compromised, both groups of microorganisms are capable of adjusting their lipid composition to control ion leakage (Van De Vossenberg et al., 2000). However, previous works suggest that the lipid core of bacterial membranes can adjust itself better to regulate membrane fluidity (Siliakus et al., 2017). This adaptation might confer a survival advantage to bacterial spp. under conditions compromising membrane fluidity and in turn intracellular fluxes, such as the exposure to elevated pCO2.
According to Wu et al. (2007), the application of high-pressure CO2, i.e., >20 bar, has shown a strong bactericidal effect in cultures of the model organism E. coli. The mechanisms contributing to the bactericidal effect included: (i) compromised membrane integrity due to facilitated intracellular diffusion of increased H2CO3∗ concentrations, (ii) drainage of internal cell components such as DNA, and cations as K+, Na+ that are linked to a more permeable membrane, and (iii) possible internal acidification jeopardizing enzymatic activity as a consequence of a surpassed cytoplasmic buffering capacity (Yao et al., 2014). In our experiments, final pH measurements after decompression (Supplementary Table 1) did not show dramatic differences at the studied pCO2 levels because of elevated buffer concentration. It should be realized that the external pH only partly determines cytoplasmic pH, which depends on the physiological features of each microorganism and the internal buffer capacity of the cell. The latter could be compromised by additional neutralization requirements to keep pH homeostasis when H2CO3∗ dissociation occurs in the cytoplasm (Slonczewski et al., 2009). Thus, some degree of cytoplasmic acidification cannot be discarded compromising cell growth in the case of glucose experiments with original inoculum (Figure 2B) and inhibition of microbial activity leading to intermediate propionate accumulation at elevated pCO2 for both substrates with the inoculum before the ALE process (Figures 4, 7).
It can be hypothesized that the increased load of an acidifying substrate, such as glucose, in every ALE cycle will lead to the development of protective measures against accumulating acidity, which could help to minimize effects on cell membrane integrity by elevated pCO2 (Sun et al., 2005) and could help to explain the increase in cell viability after ALE with glucose (Figure 2B). The noticeable positive effects of using glycerol as the substrate on cell viability (Figure 2C) could be attributed to several factors. Firstly, changes in the medium viscosity can affect the diffusion rate of CO2 and could lead to lower microbial inactivation rates by high-pressure CO2. A similar observation previously has been reported for a growth medium with increased fat content (Lin et al., 1994). Secondly, researchers recently described that compatible solutes can also act as piezolytes to increase tolerance to pressure exposure (Martin et al., 2002; Scoma and Boon, 2016). Since glycerol can also act as a compatible solute, it might as well confer a temporary piezotolerance depending on its specific uptake and conversion rate. Thirdly, because of its non-ionic nature, glycerol may reduce water activity (aw) in the medium, which in turn could contribute to lower microbial inactivation rates by high-pressure CO2 as a result of decreased H2CO3∗ formation and a stabilization effect on membrane proteins (Wu et al., 2007; Kish et al., 2012). Regarding the effects of substrate concentration on the aw in the experimental treatments, theoretical calculations performed in the hydrogeochemical software Phreeqc® indeed showed that glycerol lowered the water activity compared to glucose but at elevated substrate concentrations (Supplementary Table 3). At the applied low substrate concentrations of 5 and 9 mM for glycerol and glucose, respectively, it is questionable whether changes in aw would have significantly impacted cell viability in our experiments. Nonetheless, the observed differences in cell viability between glycerol and glucose treatments with the original inoculum (Figure 2), where a higher biomass concentration was applied, might suggest the occurrence of CO2 diffusion limitation associated with the presence of glycerol in the medium.
The detrimental effects of high pCO2 cannot be solely attributed to a pressure effect. A comparable loss of viability as the one observed at elevated pCO2 has up till now only been achieved at hydrostatic pressures higher than 100 MPa (Pagán and Mackey, 2000). When using a non-reactive gas such as N2, strong biocidal effects have not been observed even if the pH is significantly lowered to emulate pH levels due to CO2 dissolution (Wu et al., 2007). However, we observed a detrimental effect of pressurized N2 on the cell viability of evolved inoculum with glycerol (Figure 2C) which suggests a negative effect of headspace pressure at low biomass concentration. Moreover, an increased amount of non-viable cells following pressurized conditions can also be explained by reactor depressurization. It has been shown that pressure release, even if performed gradually, can increase the amount of permeabilized cells (Park and Clark, 2002), and thus, compromising their viability. Further investigations are needed to thoroughly quantify possible decompression effects on cell viability and metabolic activity when using reactive and inert gases such as CO2 and N2.
At high propionate concentrations and low pH, a microbial community shift toward increased proportions of hydrogenotrophic methanogens has been evidenced, which contributes to maintaining a low partial pressure of hydrogen (pH2) to enable Pr-Ox under syntrophic conditions (Li et al., 2018; Han et al., 2020). Apparently, low pH2 conditions favor the production of H2 instead of NADH from the oxidation of reduced ferredoxin (Fdred) (Lee et al., 2008). Furthermore, at low pH2, hydrogenotrophic methanogenesis has been described as kinetically (Liu et al., 2016) and thermodynamically more feasible than homoacetogenesis at increasing pCO2 (Supplementary Figure 4). This would imply that, in principle, we should have observed an increased proportion of hydrogenotrophic methanogens in the glucose experiments. However, this occurred only in the treatment with the original inoculum at the lowest pCO2 of 0.3 bar (Figure 3B). Zhang et al. (2011) described a possible detrimental effect of elevated CO2 concentration on the transcription levels of functional [FeFe] hydrogenases of the moderate thermophile Thermoanaerobacterium thermosaccharolyticum W16. The reduction in the ratio mRNA expression to 16S DNA gene depended on the type of substrate employed, glucose or xylose, and varied between 66 and 98% (Zhang et al., 2011). Considering this as a plausible hypothesis, H2 production might have been hindered in our elevated pCO2 experiments, leaving the production of reduced compounds as the main route for NADH consumption.
The biochemical pathways of anaerobic conversion of glucose and glycerol share PEP and pyruvate as central intermediates (Zhang et al., 2015). Pathway steering toward particular electron sinks such as propionate will depend on the environmental conditions and type of microorganism (Supplementary Figure 1). Under the assumption of CO2 fixation, the carboxylation from PEP or pyruvate to OAA, which is subsequently further reduced to fumarate, is favored at the reductive branch of the PEP-pyruvate-OAA node (Sauer and Eikmanns, 2005; Stams and Plugge, 2009). Without the presence of sufficient reducing equivalents, a presumed CO2 fixation will have a more limited impact on the production of more reduced compounds during glucose fermentation. It should be realized that, compared to glucose, the metabolism of glycerol requires balancing double the amount of reducing equivalents per mole of pyruvate or PEP produced. Under limited hydrogen production, NADH will act as electron carrier, channeling the reducing equivalents toward products such as propionate, whose formation stoichiometrically consumes the NADH from glycerol conversion (Zhang et al., 2015). Concomitantly, acetate production from the acetyl-CoA pathway is downregulated to limit reductive stress due to presence of excess NADH (Doi and Ikegami, 2014). Propionate production from pyruvate in glucose metabolism requires an additional electron donor or extra NADH input. Thus, the simultaneous production of a more oxidized compound, namely acetate, helps to satisfy the redox balance. However, this will occur at the expense of a decreased propionate yield, due to diverged carbon flux (Zhang et al., 2015), helping to explain the differences in propionate levels between the used substrates (Figures 4, 5, 7). Increased propionate production could also be attributed to enhanced enzymatic activities as a result of the substrate choice for the ALE cycles. The activity of pyruvate carboxylase and succinyl CoA: propionyl CoA transferase, both enzymes with a significant role in propionate production, has been enhanced in cultures of Propionibacterium acidipropionici using glycerol as the substrate (Zhang et al., 2016). Conversely, this was not observed by these authors when the culture was grown using glucose as the sole carbon and energy source.
The premise of compromised hydrogenase activity because of elevated CO2 concentrations could help to explain the decreased proportions of hydrogenotrophic methanogens, particularly in the treatments with glucose. Moreover, the initially high proportion of Methanosaeta in the original inoculum (Figure 6B), the reduced H2 production when using glycerol as the substrate according to stoichiometry, and a plausible detrimental effect of pCO2 on hydrogenase activity, support the idea of the enhancement of a Pr-Ox pathway where thermodynamic limitations associated with interspecies H2 transfer plays a less significant role, i.e., the dismutation pathway of Smithella, whose relative abundance considerably increased following the ALE process (Figures 3A, 6A). Members of the genus Smithella are metabolically active in a broader range of propionate concentrations (Ariesyady et al., 2007), low HRT (Ban et al., 2015) and acidic pH (Li et al., 2018) than members of the genus Syntrophobacter. The conditions imposed during the directional selection, i.e., serial transfers to fresh medium, exposed the microorganisms to increased substrate loadings per cell. This possibly contributed to the enrichment of this genus, particularly in the glycerol experiments at 5 bar pCO2 using evolved inoculum (Figure 6A). Additionally, if thermodynamic feasibility is considered, the dismutation pathway, i.e., propionate conversion to butyrate and acetate, is less sensitive to the effects of increasing pH2 and pCO2 than the methyl malonyl-CoA pathway, where propionate is converted to acetate and H2, which undergo further conversion by methanogenic archaea (Dolfing, 2013) (Supplementary Figure 5). For further reference, we have summarized the stoichiometries of possible metabolic pathways for the anaerobic conversion of glucose and glycerol including either the dismutation or the methyl malonyl-CoA pathways (Supplementary Table 2).
It is postulated that the occurrence of the Smithella pathway in the incubation with both substrates relates well with enhanced CH4 yield and might ameliorate end-product inhibition due to elevated pCO2 in the case of glucose (Supplementary Table 2). Moreover, less CO2 is being produced in the glycerol treatments either by methyl malonyl-CoA pathway or by the dismutation pathway from Smithella (Supplementary Table 2). Theoretically, this could enable a substantial CO2 fixation via the OAA route (Supplementary Figure 1) and enhanced propionate production if enough reducing equivalents are available. Regulation of the redox balance could be achieved in this pathway by the consumption of reducing equivalents in the intermediate steps, forming malate and succinate. Due to these two reasons, moderately high pCO2 levels have likely affected glycerol conversion to a lesser extent.
The here presented higher CH4 yields at 5 bar pCO2 using evolved inoculum have to be interpreted with caution since significant differences were found in the initial viable cell density of treatments with evolved and original inoculum (p = 0.004), as a consequence of the higher microbial F:M ratio imposed to keep the experimental liquid volume comparable in all treatments. The observed lower initial cell density in the experiments with evolved inoculum is attributed to the followed harvesting and resuspension procedure for biomass recovery. These initial differences did not necessarily lead to statistically significant higher proportions of viable biomass (p = 0.43) at the end of the experiments using original inoculum, thus comparisons in terms of active biomass are fairly reasonable. The CH4-yield calculated per viable cell showed that the methanogenic activity under elevated pCO2 was moderately enhanced due to the directional selection process and might be indicative of microbial community resilience to elevated pCO2. It was not possible to extrapolate these yields in terms of VSS concentration since this parameter only showed a moderate positive correlation with the log-transformed viable cell density data (rs = 0.65, p = 0.005) (Supplementary Figure 3) and did not constitute a good proxy for microbial biomass in the experiments here presented. We would not have been able to evidence subtle changes in total cell density and cell viability compromising overall metabolic activity by only relying on this measurement, since it includes dead/non-viable cells and extracellular compounds besides the active biomass (Foladori et al., 2010).
At the microbial ecology level, high CO2 concentrations shift the community structure and reduce the taxonomic diversity in different environmental systems (Yu and Chen, 2019), with effects beyond acidification (Gulliver et al., 2014). In our experiments, the directional selection process, prior exposure to elevated pCO2, proved to be preponderant for changes in community structure (Hill number H0–Richness Supplementary Figure 2A) and overall diversity (Figures 3, 6), which can benefit reactor start-up. Changes in alpha diversity could be linked to a community reorganization as a consequence of the selection pressure (increased substrate load) or an applied disturbance, namely the elevated pCO2 (Werner et al., 2011). In our experiments, as expected, microbial community richness decreased due to the ALE process, but VFA production was not compromised. Fermentative activity tends to be conserved even if decreased richness is observed, most likely due to the resilience of this process to stress conditions (Mota et al., 2017). However, conservation of functionality features of the evolved community and its prevalence as the core microbiome in long-term AD-operation will depend on process operation and control (Tonanzi et al., 2018). Concerning the archaeal community structure, CO2 enrichment in anaerobic digesters at atmospheric conditions can modify the ratio aceticlastic: hydrogenotrophic methanogens favoring the abundance of Methanosaeta (Bajón Fernández et al., 2019). This finding is in alignment with the observations here described and the study by Lindeboom et al. (2016), where a high relative abundance of Methanosaeta is reported at increased pCO2 levels during high-pressure AD.
Our observations associated with changes in structure and diversity are only indicative of the effects of elevated CO2 in fermentative and methanogenic communities and their syntrophic interactions at the bioreactor level, because of the short duration of our experiments. Further support for these conclusions needs to come from longer incubations under pressurized CO2 conditions with different types of inocula. Longer incubations leading to increased growth of adapted biomass could enable a more accurate quantification with standard methods (VSS). If a more thorough characterization of the biomass at cell level in terms of average cell dimension and biovolume would be performed, it could permit a better correlation between VSS and FCM results. Similar to previous work, a correlation between protein measurements following the Lowry method and VSS could also provide additional characterization (Lindeboom et al., 2018). Moreover, if these incubations are monitored with online pH, pressure measurements and intensive sampling for microbial community dynamics, a clearer correlation could be obtained between changes in the community and operational variables modified by the pressurized operation. But, even then, the accounted effects might depend on specific system characteristics and operational strategy. Observations of natural soil communities exposed to elevated atmospheric pCO2 show that there is not a common agreement over the effects on the microbial ecology. Recent studies have reported either no significant changes (Bruce et al., 2000; Ahrendt et al., 2014) or major shifts in the microbial community (Xu et al., 2013; Šibanc et al., 2014). These shifts include, for example, the predominance of acid-resistant groups such as Chloroflexi and Firmicutes or acetogenic spore-forming Clostridia (Conrad, 2020), which agrees with the results here reported (Figures 3, 6). From these investigations, it can be deduced that other environmental factors such as temperature, pH, nutrient availability as well as CO2 final concentration and exposure time will determine the overall fate of the microbial community after exposure to elevated pCO2.
A microbial community directional selection strategy was employed in this study to overcome limited syntrophic Pr-Ox in glucose and glycerol anaerobic conversions under elevated pCO2. The pressurized incubations using evolved inoculum showed a dissimilarly enhanced final cell viability, with a stronger positive effect of the ALE in cell viability of glucose incubations. Our results suggest that directional selection of microbial community with increased substrate load per cell triggered mechanisms to preserve cell viability. Moreover, it increased the proportions and enhanced the metabolic activity of microorganisms resilient to increasing propionate concentrations such as SPOB and the interrelated methanogenic community. The increased abundance of the genus Smithella accompanied by higher proportions of Methanosaeta after incubation with glycerol proved to be beneficial for propionate conversion at elevated pCO2. The highest CH4 yield per cell at conditions elevated pCO2 was observed in the glycerol experiments, which was attributed to enhanced propionate formation, as a way to incorporate CO2 and maintain the redox balance via metabolic regulation. Overall, using an evolved inoculum with higher viable cell density and restructured community with a predominance of key microbial groups proved to be a right course of action into surmounting reduced metabolic performance associated with elevated pCO2.
The raw fastq files used to create the OTU table for the microbial community analysis, have been deposited in the National Center for Biotechnology Information (NCBI) database (Accession number PRJNA704575).
PC-C designed the experiment, supervised the experiment execution, and wrote the manuscript in its majority. Y-tC performed the experiments, analytical measurements, and contributed to the manuscript writing and editing process. PC-C and Y-tC analyzed the data. JL provided feedback to the experimental design and critically revised the final version of the manuscript. KR constructively revised the final version of the manuscript. RL helped to design, supervised the development of the experiment, and provided constructive feedback to the manuscript. All authors contributed to the article and approved the submitted version.
This research was funded by the European Union’s Horizon 2020 Research and Innovation Program under the Marie Skłodowska-Curie grant agreement no. 676070 (SuPER-W). This communication reflects only the author’s view, and the Research Executive Agency of the EU is not responsible for any use that may be made of the information it contains.
The authors declare that the research was conducted in the absence of any commercial or financial relationships that could be construed as a potential conflict of interest.
The authors kindly acknowledge Lais Soares for her technical assistance during the experiments and Ingrid Pinel for her technical assistance with the flow cytometry measurements. Tim Lacoere and Jo de Vrieze are acknowledged for their contribution to the Illumina sequencing data processing and statistical analysis.
The Supplementary Material for this article can be found online at: https://www.frontiersin.org/articles/10.3389/fmicb.2021.675763/full#supplementary-material
Aertsen, A., Meersman, F., Hendrickx, M. E. G., Vogel, R. F., and Michiels, C. W. (2009). Biotechnology under high pressure: applications and implications. Trends Biotechnol. 27, 434–441. doi: 10.1016/j.tibtech.2009.04.001
Agler, M. T., Wrenn, B. A., Zinder, S. H., and Angenent, L. T. (2011). Waste to bioproduct conversion with undefined mixed cultures: the carboxylate platform. Trends Biotechnol. 29, 70–78. doi: 10.1016/j.tibtech.2010.11.006
Ahrendt, S. R., Mobberley, J. M., Visscher, P. T., Koss, L. L., and Foster, J. S. (2014). Effects of elevated carbon dioxide and salinity on the microbial diversity in lithifying microbial mats. Minerals 4, 145–169. doi: 10.3390/min4010145
Albers, S. V., and Meyer, B. H. (2011). The archaeal cell envelope. Nat. Rev. Microbiol. 9, 414–426. doi: 10.1038/nrmicro2576
American Public Health Association. (2005). Standard Methods for the Examination of Water and Wastewater. 21st Edn. Washington, D.C: American Public Health Association.
Amorim, N. C. S., Amorim, E. L. C., Kato, M. T., Florencio, L., and Gavazza, S. (2018). The effect of methanogenesis inhibition, inoculum and substrate concentration on hydrogen and carboxylic acids production from cassava wastewater. Biodegradation 29, 41–58. doi: 10.1007/s10532-017-9812-y
Ariesyady, H. D., Ito, T., Yoshiguchi, K., and Okabe, S. (2007). Phylogenetic and functional diversity of propionate-oxidizing bacteria in an anaerobic digester sludge. Appl. Microbiol. Biotechnol. 75, 673–683. doi: 10.1007/s00253-007-0842-y
Arslan, D., Steinbusch, K. J. J., Diels, L., Hamelers, H. V. M., Strik, D. P. B. T. B., Buisman, C. J. N., et al. (2016). Selective short-chain carboxylates production: a review of control mechanisms to direct mixed culture fermentations. Crit. Rev. Environ. Sci. Technol. 46, 592–634. doi: 10.1080/10643389.2016.1145959
Baez, A., Flores, N., Bolívar, F., and Ramírez, O. T. (2009). Metabolic and transcriptional response of recombinant Escherichia coli to elevated dissolved carbon dioxide concentrations. Biotechnol. Bioeng. 104, 102–110. doi: 10.1002/bit.22379
Bajón Fernández, Y., Soares, A., Vale, P., Koch, K., Masse, A. L., and Cartmell, E. (2019). Enhancing the anaerobic digestion process through carbon dioxide enrichment: initial insights into mechanisms of utilization. Environ. Technol. 40, 1744–1755. doi: 10.1080/09593330.2019.1597173
Ban, Q., Zhang, L., and Li, J. (2015). Shift of propionate-oxidizing bacteria with HRT decrease in an UASB reactor containing propionate as a sole carbon source. Appl. Biochem. Biotechnol. 175, 274–286. doi: 10.1007/s12010-014-1265-8
Beney, L., and Gervais, P. (2001). Influence of the fluidity of the membrane on the response of microorganisms to environmental stresses. Appl. Microbiol. Biotechnol. 57, 34–42. doi: 10.1007/s002530100754
Bray, J. R., and Curtis, J. T. (1957). An ordination of the upland forest communities of southern Wisconsin. Ecol. Monogr. 27, 325–349. doi: 10.2307/1942268
Bruce, K. D., Jones, T. H., Bezemer, T. M., Thompson, L. J., and Ritchie, D. A. (2000). The effect of elevated atmospheric carbon dioxide levels on soil bacterial communities. Glob. Change Biol. 6, 427–434. doi: 10.1046/j.1365-2486.2000.00320.x
Ceron-Chafla, P., Kleerebezem, R., Rabaey, K., van Lier, J. B., and Lindeboom, R. E. F. (2020). Direct and Indirect Effects of increased CO2 partial pressure on the bioenergetics of syntrophic propionate and butyrate conversion. Environ. Sci. Technol. 54, 12583–12592. doi: 10.1021/acs.est.0c02022
Chen, Y. Y., Temelli, F., and Gänzle, M. G. (2017). Mechanisms of inactivation of dry Escherichia coli by high-pressure carbon dioxide. Appl. Environ. Microbiol. 83:e00062. doi: 10.1128/AEM.00062-17
Conrad, R. (2020). Importance of hydrogenotrophic, aceticlastic and methylotrophic methanogenesis for methane production in terrestrial, aquatic and other anoxic environments: a mini review. Pedosphere 30, 25–39. doi: 10.1016/S1002-0160(18)60052-9
Doi, Y., and Ikegami, Y. (2014). Pyruvate formate-lyase is essential for fumarate-independent anaerobic glycerol utilization in the Enterococcus faecalis strain W11. J. Bacteriol. 196, 2472–2480. doi: 10.1128/JB.01512-14
Dolfing, J. (2013). Syntrophic propionate oxidation via butyrate: a novel window of opportunity under methanogenic conditions. Appl. Environ. Microbiol. 79, 4515–4516. doi: 10.1128/AEM.00111-13
Dragosits, M., and Mattanovich, D. (2013). Adaptive laboratory evolution – principles and applications for biotechnology. Microb. Cell Fact. 12:64. doi: 10.1186/1475-2859-12-64
Fazi, S., Ungaro, F., Venturi, S., Vimercati, L., Cruz Viggi, C., Baronti, S., et al. (2019). Microbiomes in soils exposed to naturally high concentrations of CO2 (Bossoleto Mofette Tuscany, Italy). Front. Microbiol. 10:2238. doi: 10.3389/fmicb.2019.02238
Foladori, P., Bruni, L., Tamburini, S., and Ziglio, G. (2010). Direct quantification of bacterial biomass in influent, effluent and activated sludge of wastewater treatment plants by using flow cytometry. Water Res. 44, 3807–3818. doi: 10.1016/j.watres.2010.04.027
García Rea, V. S., Muñoz Sierra, J. D., Fonseca Aponte, L. M., Cerqueda-Garcia, D., Quchani, K. M., Spanjers, H., et al. (2020). Enhancing phenol conversion rates in saline anaerobic membrane bioreactor using acetate and butyrate as additional carbon and energy sources. Front. Microbiol. 11:604173. doi: 10.3389/fmicb.2020.604173
Garcia-Gonzalez, L., Geeraerd, A. H., Mast, J., Briers, Y., Elst, K., Van Ginneken, L., et al. (2010). Membrane permeabilization and cellular death of Escherichia coli, Listeria monocytogenes and Saccharomyces cerevisiae as induced by high pressure carbon dioxide treatment. Food Microbiol. 27, 541–549. doi: 10.1016/j.fm.2009.12.004
Guan, N., and Liu, L. (2020). Microbial response to acid stress: mechanisms and applications. Appl. Microbiol. Biotechnol. 104, 51–65. doi: 10.1007/s00253-019-10226-1
Gulliver, D. M., Lowry, G. V., and Gregory, K. B. (2014). CO2 concentration and pH alters subsurface microbial ecology at reservoir temperature and pressure. RSC Adv. 4, 17443–17453. doi: 10.1039/C4RA02139H
Han, Y., Green, H., and Tao, W. (2020). Reversibility of propionic acid inhibition to anaerobic digestion: inhibition kinetics and microbial mechanism. Chemosphere 255:126840. doi: 10.1016/j.chemosphere.2020.126840
Heijnen, J. J., and Kleerebezem, R. R. (2010). “Bioenergetics of Microbial Growth,” in Encyclopedia of Industrial Biotechnology, ed. M. C. Flickinger (Hoboken, NJ: John Wiley & Sons, Inc.), 1–66.
Hickey, R. F., and Switzenbaum, M. S. (1991). Thermodynamics of volatile fatty acid accumulation in anaerobic digesters subject to increases in hydraulic and organic loading. Res. J. Water Pollut. Control Fed. 63, 141–144.
Hill, M. O. (1973). Diversity and evenness: a unifying notation and its consequences. Ecology 54, 427–432. doi: 10.2307/1934352
Himmi, E. H., Bories, A., Boussaid, A., and Hassani, L. (2000). Propionic acid fermentation of glycerol and glucose by Propionibacterium acidipropionici and Propionibacterium freudenreichii ssp. shermanii. Appl. Microbiol. Biotechnol. 53, 435–440. doi: 10.1007/s002530051638
Holtzapple, M. T., and Granda, C. B. (2009). Carboxylate platform: the MixAlco process part 1: comparison of three biomass conversion platforms. Appl. Biochem. Biotechnol. 156, 95–106. doi: 10.1007/s12010-008-8466-y
Huang, Z., Yu, J., Xiao, X., Miao, H., Ren, H., Zhao, M., et al. (2016). Directional regulation of the metabolic heterogeneity in anaerobic mixed culture to enhance fermentative hydrogen production by adaptive laboratory evolution. Int. J. Hydrogen Energy 41, 10145–10151. doi: 10.1016/j.ijhydene.2016.05.012
Kish, A., Griffin, P. L., Rogers, K. L., Fogel, M. L., Hemley, R. J., and Steele, A. (2012). High-pressure tolerance in Halobacterium salinarum NRC-1 and other non-piezophilic prokaryotes. Extremophiles 16, 355–361. doi: 10.1007/s00792-011-0418-8
Kumagai, H., Hata, C., and Nakamura, K. (1997). CO2 sorption by microbial cells and sterilization by high-pressure CO2. Biosci. Biotechnol. Biochem. 61, 931–935. doi: 10.1271/bbb.61.931
Kwon, Y. D., Kim, S., Lee, S. Y., and Kim, P. (2011). Long-term continuous adaptation of Escherichia coli to high succinate stress and transcriptome analysis of the tolerant strain. J. Biosci. Bioeng. 111, 26–30. doi: 10.1016/j.jbiosc.2010.08.007
Lee, H.-S., Salerno, M. B., and Rittmann, B. E. (2008). Thermodynamic evaluation on H2 production in glucose fermentation. Environ. Sci. Technol. 42, 2401–2407. doi: 10.1021/es702610v
Lemmer, A., Chen, Y., Lindner, J., Wonneberger, A. M., Zielonka, S., Oechsner, H., et al. (2015). Influence of different substrates on the performance of a two-stage high pressure anaerobic digestion system. Bioresour. Technol. 178, 313–318. doi: 10.1016/j.biortech.2014.09.118
Li, L., He, Q., Ma, Y., Wang, X., and Peng, X. (2016). A mesophilic anaerobic digester for treating food waste: process stability and microbial community analysis using pyrosequencing. Microb. Cell Fact. 15:65. doi: 10.1186/s12934-016-0466-y
Li, Y., Sun, Y., Li, L., and Yuan, Z. (2018). Acclimation of acid-tolerant methanogenic propionate-utilizing culture and microbial community dissecting. Bioresour. Technol. 250, 117–123. doi: 10.1016/j.biortech.2017.11.034
Lin, H., Cao, N., and Chen, L.-F. (1994). Antimicrobial effect of pressurized carbon dioxide on listeria monocytogenes. J. Food Sci. 59, 657–659. doi: 10.1111/j.1365-2621.1994.tb05587.x
Lindeboom, R. E. F., Fermoso, F. G., Weijma, J., Zagt, K., and van Lier, J. B. (2011). Autogenerative high pressure digestion: anaerobic digestion and biogas upgrading in a single step reactor system. Water Sci. Technol. 64, 647–653. doi: 10.2166/wst.2011.664
Lindeboom, R. E. F., Ferrer, I., Weijma, J., and van Lier, J. B. (2013). Effect of substrate and cation requirement on anaerobic volatile fatty acid conversion rates at elevated biogas pressure. Bioresour. Technol. 150, 60–66. doi: 10.1016/j.biortech.2013.09.100
Lindeboom, R. E. F., Ilgrande, C., Carvajal-Arroyo, J. M., Coninx, I., Van Hoey, O., Roume, H., et al. (2018). Nitrogen cycle microorganisms can be reactivated after space exposure. Sci. Rep. 8, 8–14. doi: 10.1038/s41598-018-32055-4
Lindeboom, R. E. F., Shin, S. G., Weijma, J., van Lier, J. B., and Plugge, C. M. (2016). Piezo-tolerant natural gas-producing microbes under accumulating pCO2. Biotechnol. Biofuels 9, 98–127. doi: 10.1186/s13068-016-0634-7
Liu, R., Hao, X., and Wei, J. (2016). Function of homoacetogenesis on the heterotrophic methane production with exogenous H2/CO2 involved. Chem. Eng. J. 284, 1196–1203. doi: 10.1016/j.cej.2015.09.081
Martin, D. D., Bartlett, D. H., and Roberts, M. F. (2002). Solute accumulation in the deep-sea bacterium Photobacterium profundum. Extremophiles 6, 507–514. doi: 10.1007/s00792-002-0288-1
McMahon, K. D., Zheng, D., Stams, A. J. M., Mackie, R. I., and Raskin, L. (2004). Microbial population dynamics during start-up and overload conditions of anaerobic digesters treating municipal solid waste and sewage sludge. Biotechnol. Bioeng. 87, 823–834. doi: 10.1002/bit.20192
McMurdie, P. J., and Holmes, S. (2013). Phyloseq: an R package for reproducible interactive analysis and graphics of microbiome census data. PLoS One 8:e61217. doi: 10.1371/journal.pone.0061217
Mota, M. J., Lopes, R. P., Koubaa, M., Roohinejad, S., Barba, F. J., Delgadillo, I., et al. (2017). Fermentation at non-conventional conditions in food- and bio-sciences by application of advanced processing technologies. Crit. Rev. Biotechnol. 38, 122–140. doi: 10.1080/07388551.2017.1312272
Muñoz Sierra, J. D., García Rea, V. S., Cerqueda-García, D., Spanjers, H., and van Lier, J. B. (2020). Anaerobic conversion of saline phenol-containing wastewater under thermophilic conditions in a membrane bioreactor. Front. Bioeng. Biotechnol. 8:565311. doi: 10.3389/fbioe.2020.565311
Oger, P. M., and Jebbar, M. (2010). The many ways of coping with pressure. Res. Microbiol. 161, 799–809. doi: 10.1016/j.resmic.2010.09.017
Oksanen, J., Blanchet, F. G., Kindt, R., Legendre, P., Minchin, P. R., O’Hara, B., et al. (2016). Vegan: Community Ecology Package. R Package Version 2.3-4. (Vienna, Austria: R Foundation for Statistical Computing).
Pagán, R., and Mackey, B. (2000). Relationship between membrane damage and cell death in pressure-treated Escherichia coli cells: differences between exponential- and stationary-phase cells and variation among strains. Appl. Environ. Microbiol. 66, 2829–2834. doi: 10.1128/AEM.66.7.2829-2834.2000
Parizzi, L. P., Grassi, M. C. B., Llerena, L. A., Carazzolle, M. F., Queiroz, V. L., Lunardi, I., et al. (2012). The genome sequence of propionibacterium acidipropionici provides insights into its biotechnological and industrial potential. BMC Genom. 13:562. doi: 10.1186/1471-2164-13-562
Park, C. B., and Clark, D. S. (2002). Rupture of the cell envelope by decompression of the deep-sea methanogen methanococcus jannaschii. Appl. Environ. Microbiol. 68, 1458–1463. doi: 10.1128/AEM.68.3.1458-1463.2002
Park, S., Kwon, H. S., Lee, C. H., and Ahn, I. S. (2020). Correlation between fixation of high-concentration CO2 and glutamate accumulation in sulfurovum lithotrophicum 42BKTT. J. Ind. Eng. Chem. 92, 56–61. doi: 10.1016/j.jiec.2020.08.015
Portnoy, V. A., Bezdan, D., and Zengler, K. (2011). Adaptive laboratory evolution-harnessing the power of biology for metabolic engineering. Curr. Opin. Biotechnol. 22, 590–594. doi: 10.1016/j.copbio.2011.03.007
Props, R., Kerckhof, F. M., Rubbens, P., De Vrieze, J., Hernandez Sanabria, E., Waegeman, W., et al. (2017). Absolute quantification of microbial taxon abundances. ISME J. 11, 584–587. doi: 10.1038/ismej.2016.117
R Core Team. (2019). R: A language and Environment for Statistical Computing. Available online at: https://www.r-project.org/ (accessed January 15, 2020).
Russell, J. B., and Diez-Gonzalez, F. (1998). The effects of fermentation acids on bacterial growth. Adv. Microb. Physiol. 39, 228–234. doi: 10.1016/s0065-2911(08)60017-x
Saint-Amans, S., Girbal, L., Andrade, J., Ahrens, K., and Soucaille, P. (2001). Regulation of carbon and electron flow in Clostridium butyricum VPI 3266 grown on glucose-glycerol mixtures. J. Bacteriol. 183, 1748–1754. doi: 10.1128/JB.183.5.1748
Sauer, U., and Eikmanns, B. J. (2005). The PEP-pyruvate-oxaloacetate node as the switch point for carbon flux distribution in bacteria. FEMS Microbiol. Rev. 29, 765–794. doi: 10.1016/j.femsre.2004.11.002
Scoma, A., and Boon, N. (2016). Osmotic stress confers enhanced cell integrity to hydrostatic pressure but impairs growth in Alcanivorax borkumensis SK2. Front. Microbiol. 7:729. doi: 10.3389/fmicb.2016.00729
Šibanc, N., Dumbrell, A. J., Mandič-Mulec, I., and Maćek, I. (2014). Impacts of naturally elevated soil CO2 concentrations on communities of soil archaea and bacteria. Soil Biol. Biochem. 68, 348–356. doi: 10.1016/j.soilbio.2013.10.018
Siliakus, M. F., van der Oost, J., and Kengen, S. W. M. (2017). Adaptations of archaeal and bacterial membranes to variations in temperature, pH and pressure. Extremophiles 21, 651–670. doi: 10.1007/s00792-017-0939-x
Slonczewski, J. L., Fujisawa, M., Dopson, M., and Krulwich, T. A. (2009). Cytoplasmic pH measurement and homeostasis in bacteria and archaea. Adv. Microb. Physiol. 55:317. doi: 10.1016/S0065-2911(09)05501-5
Stams, A. J. M., Dijkema, C., Plugge, C. M., and Lens, P. (1998). Contribution of 13C-NMR spectroscopy to the elucidation of pathways of propionate formation and degradation in methanogenic environments. Biodegradation 9, 463–473. doi: 10.1023/A:1008342130938
Stams, A. J. M., and Plugge, C. M. (2009). Electron transfer in syntrophic communities of anaerobic bacteria and archaea. Nat. Rev. Microbiol. 7, 568–577. doi: 10.1038/nrmicro2166
Sun, L., Fukamachi, T., Saito, H., and Kobayashi, H. (2005). Carbon dioxide increases acid resistance in Escherichia coli. Lett. Appl. Microbiol. 40, 397–400. doi: 10.1111/j.1472-765X.2005.01714.x
Tonanzi, B., Gallipoli, A., Gianico, A., Montecchio, D., Pagliaccia, P., Di Carlo, M., et al. (2018). Long-term anaerobic digestion of food waste at semi-pilot scale: relationship between microbial community structure and process performances. Biomass Bioenergy 118, 55–64. doi: 10.1016/j.biombioe.2018.08.001
Town, J. R., and Dumonceaux, T. J. (2016). Laboratory-scale bioaugmentation relieves acetate accumulation and stimulates methane production in stalled anaerobic digesters. Appl. Microbiol. Biotechnol. 100, 1009–1017. doi: 10.1007/s00253-015-7058-3
Van De Vossenberg, J. L. C. M., Driessen, A. J. M., and Konings, W. N. (2000). “Adaptations of the cell membrane for life in extreme environments,” in Environmental Stressors and Gene Responses, eds K. B. Storey and J. M. Storey (Netherlands: University of Groningen), 71–88.
Wainaina, S., Lukitawesa, L., Kumar Awasthi, M., and Taherzadeh, M. J. (2019). Bioengineering of anaerobic digestion for volatile fatty acids, hydrogen or methane production: a critical review. Bioengineered 10, 437–458. doi: 10.1080/21655979.2019.1673937
Werner, J. J., Knights, D., Garcia, M. L., Scalfone, N. B., Smith, S., Yarasheski, K., et al. (2011). Bacterial community structures are unique and resilient in full-scale bioenergy systems. Proc. Natl. Acad. Sci. U.S.A. 108, 4158–4163. doi: 10.1073/pnas.1015676108
Wu, Y., Yao, S.-J., and Guan, Y.-X. (2007). Inactivation of microorganisms in carbon dioxide at elevated pressure and ambient temperature. Ind. Eng. Chem. Res. 46, 6345–6352. doi: 10.1021/ie0702330
Xu, M., He, Z., Deng, Y., Wu, L., Van Nostrand, J. D., Hobbie, S. E., et al. (2013). Elevated CO2 influences microbial carbon and nitrogen cycling. BMC Microbiol 13:124. doi: 10.1186/1471-2180-13-124
Yao, C., Li, X., Bi, W., and Jiang, C. (2014). Relationship between membrane damage, leakage of intracellular compounds, and inactivation of Escherichia coli treated by pressurized CO2. J. Basic Microbiol. 54, 858–865. doi: 10.1002/jobm.201200640
Yu, T., and Chen, Y. (2019). Effects of elevated carbon dioxide on environmental microbes and its mechanisms: a review. Sci. Total Environ. 655, 865–879. doi: 10.1016/j.scitotenv.2018.11.301
Zabranska, J., and Pokorna, D. (2018). Bioconversion of carbon dioxide to methane using hydrogen and hydrogenotrophic methanogens. Biotechnol. Adv. 36, 707–720. doi: 10.1016/j.biotechadv.2017.12.003
Zhang, A., Sun, J., Wang, Z., Yang, S.-T., and Zhou, H. (2015). Effects of carbon dioxide on cell growth and propionic acid production from glycerol and glucose by Propionibacterium acidipropionici - sciencedirect. Bioresour. Technol. 175, 374–381. doi: 10.1016/J.BIORTECH.2014.10.046
Zhang, K., Ren, N. Q., Cao, G. L., and Wang, A. J. (2011). Biohydrogen production behavior of moderately thermophile Thermoanaerobacterium thermosaccharolyticum W16 under different gas-phase conditions. Int. J. Hydrogen Energy 36, 14041–14048. doi: 10.1016/j.ijhydene.2011.04.056
Zhang, L., Zhu, K., and Li, A. (2016). Differentiated effects of osmoprotectants on anaerobic syntrophic microbial populations at saline conditions and its engineering aspects. Chem. Eng. J. 288, 116–125. doi: 10.1016/j.cej.2015.11.100
Keywords: high-pressure anaerobic digestion, elevated CO2 partial pressure, syntrophic propionate oxidation, Smithella, adaptive laboratory evolution
Citation: Ceron-Chafla P, Chang Y-t, Rabaey K, van Lier JB and Lindeboom REF (2021). Front. Microbiol. 12:675763. doi: 10.3389/fmicb.2021.675763
Received: 03 March 2021; Accepted: 24 May 2021;
Published: 16 June 2021.
Edited by:
Irini Angelidaki, Technical University of Denmark, DenmarkReviewed by:
Bipro Ranjan Dhar, University of Alberta, CanadaCopyright © 2021 Ceron-Chafla, Chang, Rabaey, van Lier and Lindeboom. This is an open-access article distributed under the terms of the Creative Commons Attribution License (CC BY). The use, distribution or reproduction in other forums is permitted, provided the original author(s) and the copyright owner(s) are credited and that the original publication in this journal is cited, in accordance with accepted academic practice. No use, distribution or reproduction is permitted which does not comply with these terms.
*Correspondence: Pamela Ceron-Chafla, cC5zLmNlcm9uY2hhZmxhQHR1ZGVsZnQubmw=
Disclaimer: All claims expressed in this article are solely those of the authors and do not necessarily represent those of their affiliated organizations, or those of the publisher, the editors and the reviewers. Any product that may be evaluated in this article or claim that may be made by its manufacturer is not guaranteed or endorsed by the publisher.
Research integrity at Frontiers
Learn more about the work of our research integrity team to safeguard the quality of each article we publish.