- 1Instituto de Química Biológica, “Dr. Bernabé Bloj”, Facultad de Bioquímica, Química y Farmacia, Universidad Nacional de Tucumán (UNT) and Instituto Superior de Investigaciones Biológicas (INSIBIO), CONICET-UNT, San Miguel de Tucumán, Argentina
- 2Department of Biochemistry and Molecular Biology, Universidade Federal do Paraná, Curitiba, Brazil
- 3Centro de Referencia para Lactobacilos (CERELA-CONICET), San Miguel de Tucumán, Argentina
- 4Facultad de Agronomía y Zootecnia, Universidad Nacional de Tucumán (UNT), San Miguel de Tucumán, Argentina
Herbaspirillum seropedicae is a nitrogen-fixing endophytic bacterium associated with important cereal crops, which promotes plant growth, increasing their productivity. The understanding of the physiological responses of this bacterium to different concentrations of prevailing nutrients as phosphate (Pi) is scarce. In some bacteria, culture media Pi concentration modulates the levels of intracellular polyphosphate (polyP), modifying their cellular fitness. Here, global changes of H. seropedicae SmR1 were evaluated in response to environmental Pi concentrations, based on differential intracellular polyP levels. Cells grown in high-Pi medium (50 mM) maintained high polyP levels in stationary phase, while those grown in sufficient Pi medium (5 mM) degraded it. Through a RNA-seq approach, comparison of transcriptional profiles of H. seropedicae cultures revealed that 670 genes were differentially expressed between both Pi growth conditions, with 57% repressed and 43% induced in the high Pi condition. Molecular and physiological analyses revealed that aspects related to Pi metabolism, biosynthesis of flagella and chemotaxis, energy production, and polyhydroxybutyrate metabolism were induced in the high-Pi condition, while those involved in adhesion and stress response were repressed. The present study demonstrated that variations in environmental Pi concentration affect H. seropedicae traits related to survival and other important physiological characteristics. Since environmental conditions can influence the effectiveness of the plant growth-promoting bacteria, enhancement of bacterial robustness to withstand different stressful situations is an interesting challenge. The obtained data could serve not only to understand the bacterial behavior in respect to changes in rhizospheric Pi gradients but also as a base to design strategies to improve different bacterial features focusing on biotechnological and/or agricultural purposes.
Introduction
Beneficial plant–bacteria interactions promote plant growth and development. Plant growth-promoting bacteria (PGPB) represent a promising alternative to be used as biofertilizer and biocontrol agent, due to their ability to fix atmospheric N2, solubilize minerals, and produce phytohormones and siderophores (Bhattacharyya and Jha, 2012). Environmental conditions before, during, and after plant inoculation can influence the effectiveness of the PGPB (Berney et al., 2006; Baldani et al., 2014). It is well known that bacteria are influenced by the soil conditions including temperature, moisture, and chemicals (Glick et al., 1999). Data regarding concentration of phosphorus in the soil solution are scarce and inconsistent. An adequate phosphate (Pi) supply is a challenge for many bacteria in soil, where the level of free Pi can be affected by the pH-dependent formation of precipitates (Bagyaraj et al., 2000; Matula, 2011). Pi concentration in soil produces changes in microbial community composition (Leff et al., 2015; Fabiañska et al., 2018). In addition, Pi availability influences the plant–microbe interaction along the mutualism process (Hiruma et al., 2016).
Pi is an essential constituent of all living organisms. Biomolecules containing Pi participate in a wide range of cellular activities, including processing of cellular information, energy metabolism, signaling, regulation of protein activity, and maintenance of acid–base homeostasis (Devine, 2018). In bacteria, the mechanism that senses and responds to Pi limitation involves the high-affinity phosphate-specific transport (Pst) system, in combination with the two-component signaling system, PhoR–PhoB, collectively known as the phosphate regulon (Pho regulon) (Hsieh and Wanner, 2010). The most common genes of the Pho regulon encode extracellular enzymes capable of obtaining Pi from organic phosphates, Pi-specific transporters, and enzymes involved in nutrient storage processes (Rao and Torriani, 1990; Santos-Beneit et al., 2008; Hsieh and Wanner, 2010). The Pho regulon not only is a regulatory circuit of Pi homeostasis but also plays an important adaptive role in bacterial stress and virulence (Chekabab et al., 2014).
Microorganisms store Pi in the form of polyphosphate (polyP), a linear polymer of Pi with a chain length of 1,000 residues or more. Besides its function as a storage compound, polyP is involved in stress response (e.g., starvation, acid, or oxidative stresses) virulence, motility, or biofilm formation, among other physiological processes (Grillo-Puertas et al., 2012, 2014, 2015, 2018; Gray and Jakob, 2015; Albi and Serrano, 2016). It has been described in several bacteria that variations in environmental Pi concentrations can modulate intracellular polyP levels, affecting bacterial physiology (Schurig-Briccio et al., 2009a; Grillo-Puertas et al., 2012, 2014, 2015, 2016, 2018; Correa-Deza et al., 2017). Indeed, Escherichia coli K-12 cells maintained a high polyP level during the stationary phase in the presence of a high Pi concentration (>25–37 mM). High polyP levels were correlated to protection against oxidative stress, inhibition of biofilm formation, and tolerance to copper salts (Schurig-Briccio et al., 2009b; Grillo-Puertas et al., 2012, 2015). In the PGPB Gluconacetobacter diazotrophicus, variations in media Pi concentration modulated the intracellular polyP levels, affecting its survival, environmental stress tolerance, biofilm formation capacity, and competence as a promoter of strawberry plant growth (Grillo-Puertas et al., 2018).
Herbaspirillum seropedicae is a diazotrophic endophytic PGPB that colonizes several crops, such as rice (Oryza sativa), maize (Zea mays), sorghum (Sorghum bicolor), and sugarcane (Saccharum officinarum). Within the H. seropedicae genome, four genes coding for polyP-related proteins were found: Hsero_1254 (ppk1), Hsero_3790 (ppk2), and Hsero_4359, encoding enzymes involved in polyP synthesis, and Hsero_1891 (ppx), coding for an exopolyphosphatase (Pedrosa et al., 2011). Here, the global changes of H. seropedicae SmR1 in response to environmental Pi conditions, based on differential intracellular polyP levels, were evaluated through RNA-seq along with physiological experiments. Our results provide valuable insights into the H. seropedicae gene expression upon differential Pi conditions, and allowed to assess its role in several adaptive pathways.
Materials and Methods
Bacterial Strain, Culture Media, and Growth Conditions
H. seropedicae strain SmR1 (Souza et al., 2000) was routinely grown at 30°C with 120 rpm in NFbHPN-malate medium (Pedrosa and Yates, 1984; Klassen et al., 1997) supplemented with 80 μg ml–1 of streptomycin. NFbHPN containing 50 mM potassium phosphate buffer was denoted here as NFb50 medium. Also, NFbHPN-malate was prepared with a reduced Pi concentration of 5 mM and defined as NFb5 medium. Growth curves were performed in both media at 30°C for 24 h. Aliquots were taken at different time intervals to measure CFU ml–1 and absorbance at 600 nm.
Measurement of PolyP Levels
Intracellular polyP was measured in cell suspensions using a DAPI (4’,6-diamidino-2-phenylindole)-based fluorescence approach (Aschar-Sobbi et al., 2008). Briefly, cells were grown in NFb5 or NFb50 media at 30°C for 24 h. Cell suspensions were washed and resuspended in buffer T (100 mM Tris–HCl, pH 7.5) at an A600 nm = 0.02. For cell permeabilization, 15 μl of 0.1% SDS and chloroform were added. Finally, 17 μM DAPI (Sigma) was added to cuvettes. After stirring for 5 min at 37°C, the DAPI fluorescence spectra (excitation, 415 nm; emission, from 445 to 650 nm) were recorded using an ISS PCI spectrofluorometer (SS Inc., Champaign, IL). Fluorescence (expressed as arbitrary units) of the DAPI–polyP complex at 550 nm was used as a measure of the intracellular polyP level, since fluorescence emissions from free DAPI and DAPI-DNA are minimal at this wavelength (Aschar-Sobbi et al., 2008).
Transcriptome Profiling RNA-Seq Design and Analyses
H. seropedicae SmR1 cells were grown in NFb5 or NFb50 media and harvested after 9 h of growth with a cell density corresponding to an A600 nm ∼ 0.8 for both conditions. Three independent samples (biological replicates) of each condition were used to construct six sequencing libraries. Bacterial samples were taken, and total RNA was isolated using TRI Reagent (Sigma) followed by chloroform extraction as recommended by the manufacturer. Samples were treated with DNaseI (Ambion) and the RNA was quantified with a NanoDrop Spectrophotometer (THERMO Scientific) and gel electrophoresis. The ribosomal RNA was depleted using Ribo-Zero rRNA Removal Kit-Gram-Negative Bacteria (Illumina). The whole transcriptome libraries were constructed using the Ion total RNA-seq Kit v2 (Thermo Fisher Scientific), barcode with Ion XpressTM Barcode Adapters, following the manufacturer instructions. The libraries were amplified and enriched using the Ion PI Hi-QTM OT2 200 Kit, and sequenced with the Ion PI Hi-QTM Sequencing 200 kit on an Ion PITM Chip Kit v3. Obtained sequences were trimmed, mapped, and analyzed using CLC Genomics Workbench 7.5.1 (Qiagen) against the H. seropedicae SmR1 genome (NC_014323). The following parameters were used: reads were trimmed to a minimum of 40 bp, 90% alignment to the reference sequence and 80% identity required for inclusion as a mapped read, number of hits equal to 1, and number of additional bases downstream and upstream of the CDS equal to 50 bp. A gene was considered to be expressed with a read coverage equal to or higher than threefold and differentially expressed when the normalized RPKM (reads per kilobase per million mapped reads) value was twofold higher in NFb50 compared to NFb5 medium and with a p ≤ 0.05. Differential gene expression was accessed using the DESeq2 package, which is based on a negative binomial distribution analysis (Love et al., 2014). Those genes with a RPKM log2fold change ≥ 1 and p ≤ 0.05 were considered differentially regulated. Some genes with fold changes marginally lower than 2.0-fold were also considered regulated, if neighborhood analysis suggested that they are part of a pathway with genes regulated according to the previous criteria. Differential expression values in the text, tables, and figures are shown as fold change.
Quantitative Reverse Transcription Real-Time PCR
In order to confirm differential expression in the RNA-seq profiling, quantitative reverse transcription real-time PCR was performed on selected targets. Briefly, 2 μg of RNA extracted from H. seropedicae as described previously was used to synthesize cDNA using High Capacity cDNA Reverse Transcription Kit (Applied Biosystems) and quantified in triplicate using the Power SYBR-Green PCR MasterMix on a Step-One Plus Real Time-PCR System (Applied Biosystems). The PrimerQuest Tool of Integrated DNA Technologies® was used to design qPCR primers, as listed in Table 1. Primer efficiency was calculated through cDNA dilution curve over at least five orders of magnitude. The 16S rRNA gene was used as internal control, and the relative gene expression was determined using the 2–ΔΔCt method (Livak and Schmittgen, 2001).
Determination of Alkaline Phosphatase Activity
Alkaline phosphatase (AP) is an enzyme encoded by phoA, a Pho regulon member in several bacteria (Wanner and Latterell, 1980; Lamarche et al., 2005, 2008). AP activity was determined using the chromogenic substrate p-nitrophenylphosphate (pNPP), according to a previously described method (Lamarche et al., 2005) with modifications. Briefly, cells were grown at 30°C in the indicated media and aliquots were extracted at different times. For permeabilization, cells were resuspended at an A600 nm = 0.5 in 1 M Tris–HCl buffer (pH 8) to a final volume of 1 ml with the addition of 30 μl of 0.1% SDS and 30 μl of chloroform and incubated for 30 min at room temperature. Permeabilized cells were incubated with 2 mM pNPP (Sigma) at 37°C for 20 min or up to color development. Absorbance at 405 and 550 nm was determined. AP activity was calculated with the following equation, where t is the reaction time in minutes:
Pi Solubilization Assay
For Pi solubilization assay, cells were first grown in M1 liquid medium (Gupta et al., 1999). After 24 h of incubation at 30°C and 150 rpm, cells were harvested by centrifugation (10,000 rpm; 5 min). The pellet was washed twice and resuspended in 0.9% NaCl solution (w/v) to an A600 nm = 0.3. Aliquots of 10 μl were placed in NBRIP plates containing 5 g L–1 tricalcium phosphate [Ca3(PO4)2] (Nautiyal, 1999), and NBRIP was supplemented with 5 or 50 mM Pi buffer (denoted as NBRIP+5 or NBRIP+50, respectively). After incubation at 30°C for 72 h, the solubilization index (SI) was determined as previously described by Edi–Premono et al. (1996). A positive reaction was determined as the presence of clear halos around the colonies.
Motility and Chemotaxis Assays
Overnight NFb50 cultures were washed and resuspended in fresh NFb5 medium to an A600 nm = 0.1. Motility was evaluated according to Ulett et al. (2006) with modifications. A cell suspension was spotted onto the center of a NFb5 or NFb50 0.3% agar plates, using a sterile toothpick for inoculation. Plates were incubated at 30°C for 48 h, and swimming motility was determined by measuring diameters of growth. Data are expressed in centimeters as the mean diameter of movement for four independent experiments. Chemotaxis was evaluated by measuring the distance of the bacterial growth from the spot to the edge of the halo in the direction of chemical stimuli (malate, glucose, salicylic acid, shikimic acid, and root exudates) in 0.3% agar plates containing 0.9% NaCl without a carbon source, after 48 h at 30°C. Inoculation point was 1.5 cm away from the attractants. Solutions of 4% malate or glucose and 75 μg ml–1 shikimic or salicylic acids were used. For root exudates, a filter disc containing exudates from germinated maize seeds were placed on the plates.
Quantification of Biofilm Formation
Biofilm formation was assayed by growing cells on polystyrene microtiter plates and stained with crystal violet solution (O’Toole and Kolter, 1998). Overnight cultures in the NFb50 medium were diluted to an A600 nm = 0.1 with fresh NFb5 or NFb50 media. Two hundred microliters of cells were grown in a 96-well microtiter plate under static conditions at 30°C for 48 h. Then, unattached cells were removed by washing the plates with deionized water. Two hundred microliters of 0.1% crystal violet solution were added to each well, and plates were incubated at room temperature for 15 min. Then, wells were rinsed three times with water. The absorbed crystal violet was extracted with 200 μl of 95% ethanol, and A595 nm was measured (Spectra MaxPlus384 Absorbance Microplate Reader, United States).
Maize Root Colonization Assay
Assays of maize (Z. mays) root colonization by H. seropedicae SmR1 strain were performed according to Balsanelli et al. (2013) with modifications. Briefly, seeds of Z. mays cv. 30F53 (Dow AgroScience, Argentina) were surface-sterilized as described previously (Döbereiner et al., 1995; Balsanelli et al., 2010) and pre-germinated in 1% (w/v) water-agar Petri dishes in the dark for 48 h at 30°C. The seedlings were then transferred to glass tubes (one seedling in each tube) with 5 ml of Plant Medium (Egener et al., 1999) containing a final Pi concentration of 5 or 50 mM (named as PM5 or PM50, respectively) and about 2 ml in volume of sterile perlite (Perlomeoi®, Imerys, Brazil) as support. Each tube was inoculated with 1 ml of H. seropedicae cell suspension (∼107 CFU ml–1). The inoculated maize seedlings were incubated at 26°C with a light cycle of 14/10 h (light/dark) for 5 days. Free living, root epiphytic and endophytic bacterial populations were quantified. Epiphytic bacteria were recovered by vortexing roots for 30 s in 1 ml of 0.9% NaCl solution. Endophytic bacteria were recovered by homogenizing surface-sterilized roots with a pestle and mortar. Homogenates were serially diluted and plated on NFb50 medium containing streptomycin (80 μg ml–1). Colonies were counted after 2 days of incubation at 30°C and expressed as colony-forming units (CFU) per milliliter of medium or per gram of fresh root tissue.
Poly-3-Hydroxybutyrate (PHB) Quantification by Flow Cytometry
PHB quantification was carried out by flow cytometry, according to Alves et al. (2017). Briefly, H. seropedicae SmR1 and ΔphaC1, a PHB synthesis-deficient mutant (Tirapelle et al., 2013), were cultivated in NFb5 or NFb50 media for 24 h. At different time points, 100 ml of bacterial culture (∼106–107 cells) was centrifuged for 1 min at 13,000 rpm. The supernatant was discarded, and the cell pellet was resuspended in 1 ml of TBAC buffer [PBS buffer containing 1 mM EDTA and 0.01% (v/v) Tween 20] and 50% of ethanol (v/v). After 1 min of incubation, samples were stained with 31 mM of Nile Red for 1 min in the dark and centrifuged 1 min at 13,000 rpm, and the supernatant was discarded. The pellet was then resuspended in 1 ml of TBAC solution and immediately analyzed in a BD Accuri C5s Flow Cytometer (BD Biosciences, United States), equipped with a 488-nm laser for fluorescence excitation. For each sample, 100,000 events were acquired. The fluorescence intensities were obtained from histograms of FL2-H 585/40 nm channel.
Statistical Analysis
When indicated, data were subjected to analysis of variance (ANOVA) followed by Tukey’s test with Infostat Analytical Software 2020 for Windows. Differences at p-value 0.05 were considered significant.
Results and Discussion
PolyP Levels in H. seropedicae Growing Under Different Pi Concentrations
Intracellular polyP was measured in H. seropedicae SmR1 throughout the growth curves to establish whether media Pi concentration influences the polymer levels. Figure 1 shows growth and polyP levels in sufficient or high Pi concentration media, containing 5 or 50 mM Pi (NFb5 or NFb50, respectively). Bacterial growth was similar in both media up to early stationary phase. At 24 h, cells grown in NFb50 reached higher A600 nm values than those grown in NFb5, corresponding to ∼108 and 107 CFU ml–1, respectively. SmR1 cells grown in NFb50 medium accumulated polyP up to 9 h, maintaining high levels during the stationary phase. However, cells grown in NFb5 medium accumulated the polymer up to 6 h and, thereafter, degraded it. Thus, an improved stationary phase survival of H. seropedicae cells was observed in NFb50, where high polyP levels were maintained. Fluctuation of polyP levels by environmental Pi has been previously reported in E. coli K12, uropathogenic E. coli, Lactobacillus rhamnosus, and G. diazotrophicus (Schurig-Briccio et al., 2009a; Grillo-Puertas et al., 2015, 2018; Correa-Deza et al., 2017). A common feature among these bacteria is the improvement of their stationary phase fitness when cells present high intracellular polyP levels, which can be achieved adjusting media Pi concentration, according to the studied microorganism.
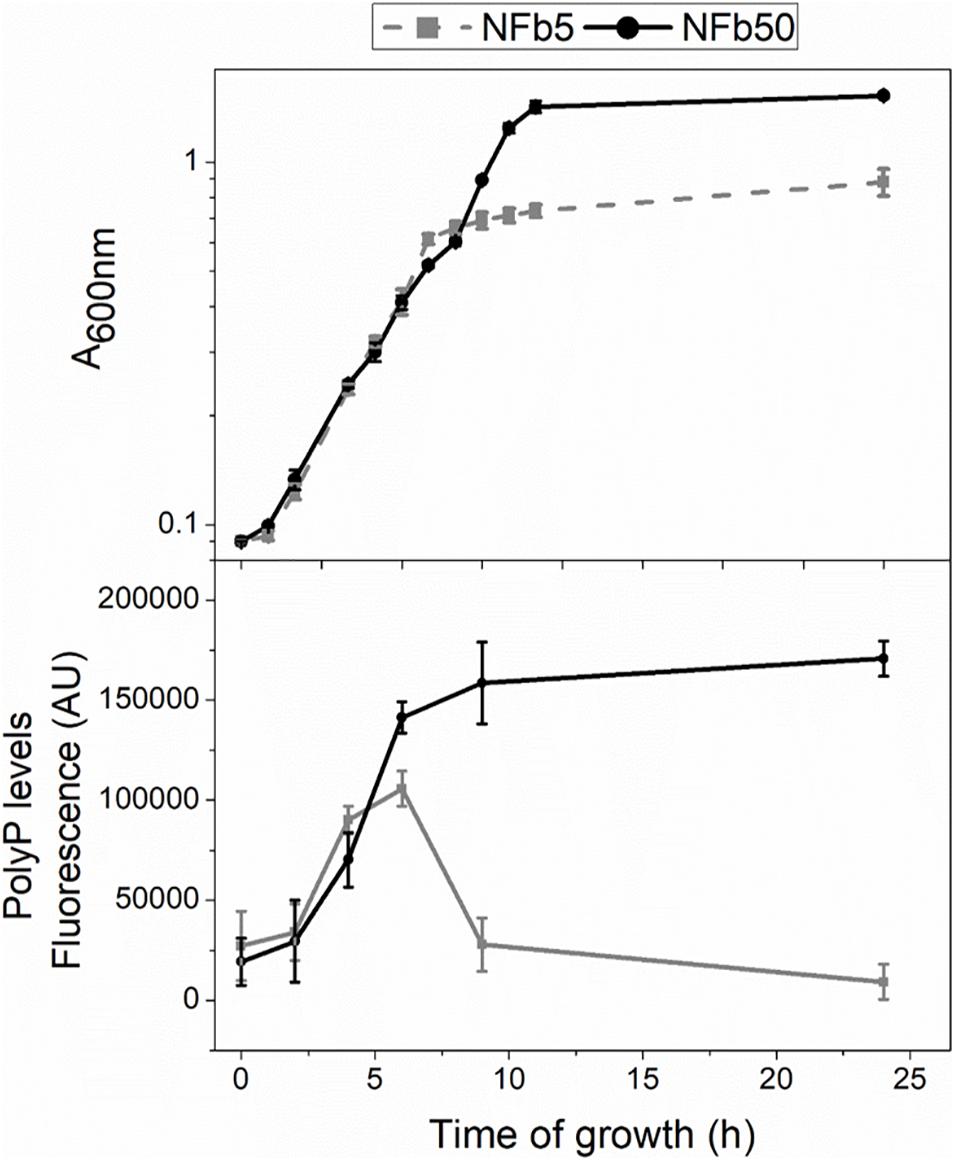
Figure 1. Cell growth and polyP levels in sufficient and high Pi media. Cells were grown at 30°C for 24 h in NFb5 or NFb50 media. Bacterial growth and polyP levels were measured at indicated times. Growth was determined by measuring the A600 nm (upper panel). PolyP levels were determined using DAPI fluorescence and data were expressed in fluorescence arbitrary units (AU) (bottom panel). Data represent the mean ± SD of at least three independent experiments with a p < 0.05.
RNA-Seq of H. seropedicae Grown Under Differential Pi Conditions
Considering that environmental Pi concentration modulates polyP levels in H seropedicae SmR1 and to analyze the global changes generated by these variations, a comparative transcriptomic analysis by RNA-seq was carried out in cells grown in NFb5 and NFb50 media at 9 h. The selection of this particular time was based on the facts that OD and CFU (∼107 CFU ml–1 for both cultures) values were similar at 9 h and polyP levels were remarkably different when comparing both conditions (Figure 1). After sequencing, 26 and 35 total million reads were obtained for NFb50 and NFb5 conditions, respectively, and from those, 12 million and 16 million reads were uniquely mapped to the H. seropedicae SmR1 genome. The transcriptomic raw data presented in this study can be found in GEO repository (GSE168341)1. Biological replicates showed a very high level of correlation (r2 > 0.98) (Table 2); thus, all the libraries of each condition were used for further analysis. Among the 4805 genes of the H. seropedicae SmR1 genome, 3976 genes were expressed considering a read coverage equal to or higher than threefold. Supplementary Table 1A shows the 1330 genes whose expression levels were statistically different (p ≤ 0.05), from which 670 had fold changes of 2 or higher and were considered differentially expressed genes (DEGs) in NFb50 vs. NFb5 transcriptome (Supplementary Table 1B), with 385 being downregulated and 285 being upregulated. To confirm the differential expression observed by the RNA-seq data, the regulation of flhA, cheD, and rfbD genes was confirmed by RT-qPCR (Table 3).
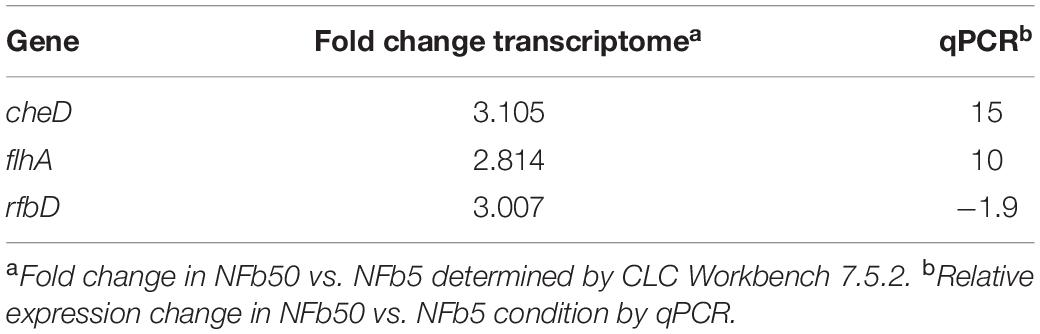
Table 3. Comparison of the gene expression of H. seropedicae SmR1 under Pi differential conditions between reverse transcription-quantitative PCR and RNA-seq analysis.
Clusters of orthologous groups (COGs) classification of DEG showed that 20 biological processes were involved in the response to Pi concentration. Figure 2 shows functional classification of upregulated and downregulated genes of cells grown in Pi differential conditions. Most DEGs belong to the following functional categories: Cell motility, intracellular trafficking, and secretion (22.7%); Translation, ribosomal structure, and biogenesis (18.7%); Energy production and conversion (16.9%); Inorganic ion transport and metabolism (15%); Nucleotide transport and metabolism (14.6%); Transcription (13.5%); and Carbohydrate transport and metabolism (13.5%) (Figure 2).
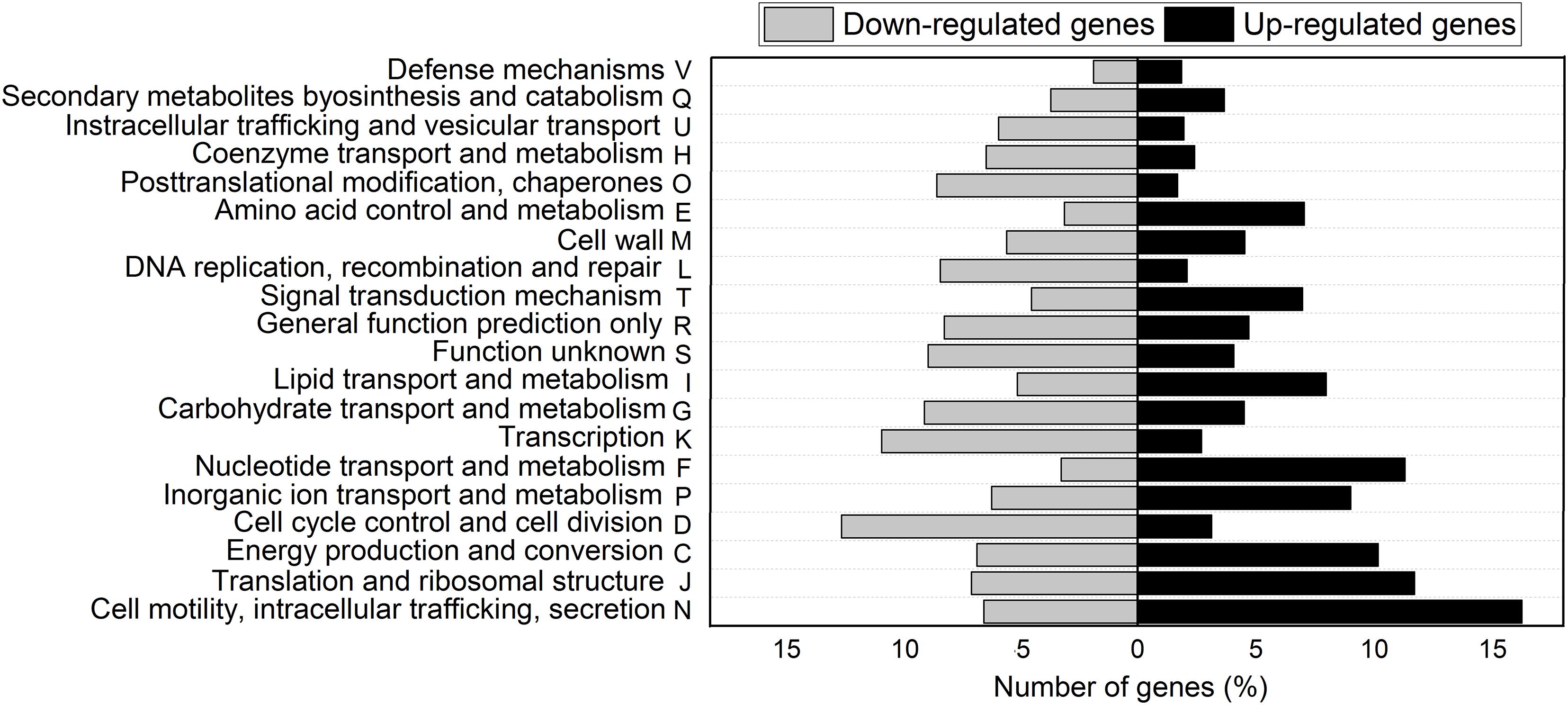
Figure 2. COGs classification of differentially expressed genes in NFb50 condition relative to NFb5 condition. COG functional categories are shown. Black bars indicate upregulated genes and gray bars denote downregulated genes.
A detailed knowledge-driven DEG analysis of pathways involved in relevant aspects of H. seropedicae SmR1 as PGPB is depicted below.
Phosphate Metabolism
Several genes involved in phosphorus metabolism were found in the H. seropedicae SmR1 genome (NC_014323, Pedrosa et al., 2011), corresponding to approximately 70% of the phosphorus related genes that were identified in the metagenomics approach of soils microbiome performed by Dai et al. (2020). Figure 3A shows that high Pi concentration in culture media unusually increased the expression of some Pi-starvation response components: the Pi regulon two-component response regulator (phoB), the AP gene (phoA), two Pi starvation-inducible genes (phoH and psiF), the low-affinity Pi transporter gene (pitA), and another Pi transporter encoded by the alkylphosphonate uptake gene (phnA). It is noted that, with regard to polyP-related genes, only ppk2 was induced under the high Pi condition. Regulation of genes involved in polyP synthesis and degradation is complex. It was previously described that fluctuations in polymer levels were not regulated at a genetic level, but by modulation of their enzymatic activity (Rudat et al., 2018).
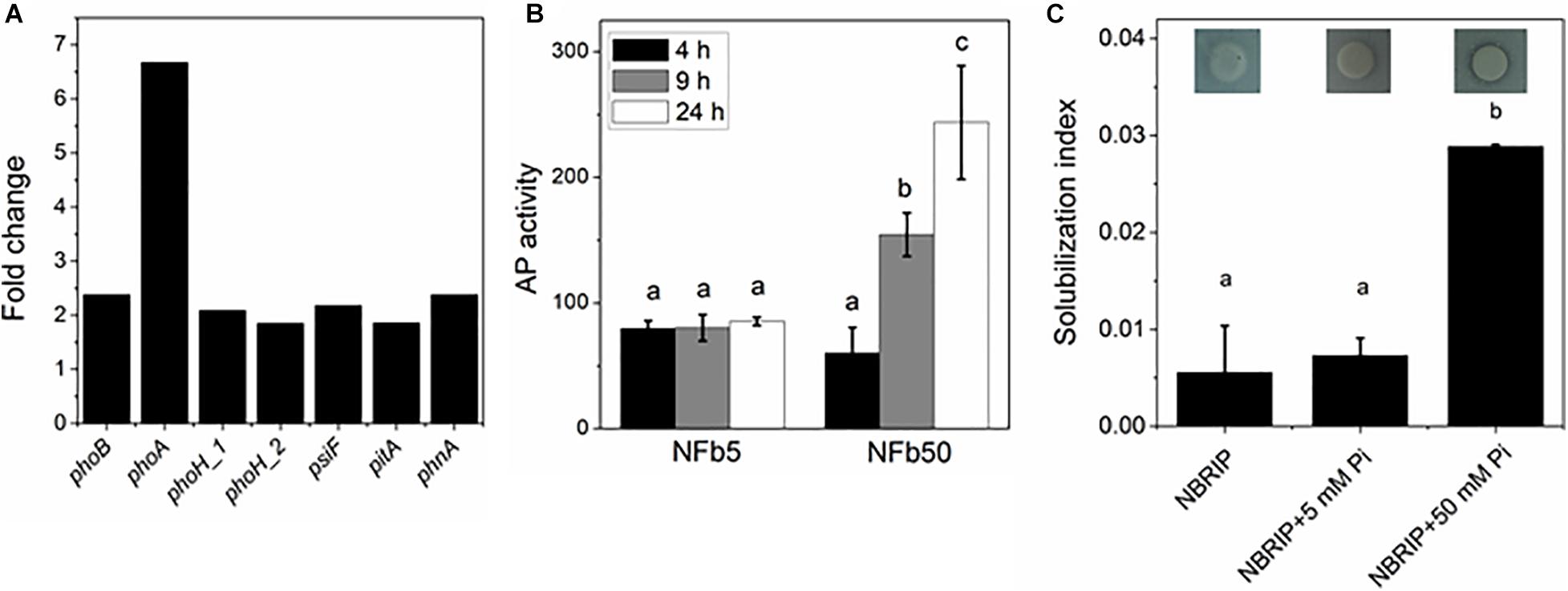
Figure 3. Expression of Pi metabolism-related genes, AP activity, and Pi solubilization in NFb50 condition relative to NFb5 condition. (A) Fold changes of differentially expressed genes. (B) Cells of the SmR1 strain were grown at 30°C in the indicated media. At 4, 9, and 24 h, aliquots were removed and AP activities were determined according to Materials and Methods section. (C) Cells of the SmR1 strain were grown at 30°C in M1 medium for 24 h and then spotted in NBRIP plates containing the indicated Pi concentration. Pi solubilization index was calculated as described in the Materials and Methods section. Results represent the mean ± SD of three independent experiments. For each panel, different letters indicate significant differences between conditions according to Tukey’s test with a p < 0.05.
To analyze whether the observed phoA upregulation was correlated with the protein activation, the AP activity was measured in cells grown in NFb5 and in NFb50 media at 4, 9, and 24 h (Figure 3B). A low AP activity was observed when cells grown in NFb5, while, in NFb50, an increased activity was obtained from 9 h. In several bacteria, phoA gene transcription is positively regulated only by PhoB, being largely used the AP activity as a reporter of PhoB activation (Wanner and Latterell, 1980; Lamarche et al., 2005, 2008). Interestingly, cells cultivated in NFb50 medium (high polyP condition) overexpressed Pi metabolism genes as if they were sensing Pi deficiency. Previous studies in E. coli demonstrated that the maintenance of high polyP levels in stationary phase induced an unexpected activation of PhoB, via acetyl phosphate (AcP), in high Pi conditions (Grillo-Puertas et al., 2016). In agreement, when AcP metabolism genes were analyzed in H. seropedicae SmR1, an upregulation of the phosphotransacetylase and acetate kinase genes, encoded by pta [1.77-fold (p-value 0.001)] and ackA (3.06-fold), was observed, inferring a possible role of AcP in PhoB activation in H. seropedicae SmR1. Considering the scarce previous knowledge about regulation of the Pi genes in H. seropedicae, the induction of the classical Pi starvation response in high Pi medium is intriguing. This is in agreement with previous results in E. coli (Grillo-Puertas et al., 2016). In addition, it was found that H. seropedicae SmR1 was able to solubilize tricalcium phosphate only when 50 mM Pi was added to NBRIP plates (Figure 3C). Although tricalcium phosphate is inappropriate as a universal selection factor for testing Pi-solubilizing bacteria (Bashan et al., 2013), the solubilization of insoluble phosphorus observed here is surprising, not only because previously studied H. seropedicae strains did not have phosphorus-solubilizing capacity (Estrada et al., 2013; Wagh et al., 2014), but also due to the observed phenomenon in cells surrounded by excess of environmental Pi. Bagyaraj et al. (2000) suggested the existence of shared regulatory controls between the pho regulon and the phosphorus solubilization in Gram-negative rhizobacteria. Phosphorus can be released from organic compounds in soil by different mechanisms, such as the secretion of gluconic acid synthesized by a pathway involving pyrroloquinoline quinone (PQQ)-dependent periplasmic glucose dehydrogenase or the secretion of non-specific phosphatases (Tarafdar and Jung, 1987; Tarafdar and Claassen, 1988; Rossolini et al., 1998). H. seropedicae SmR1 is deficient in PQQ biosynthesis genes (Pedrosa et al., 2011); therefore, the aforementioned slight Pi solubilization (Figure 3C) could involve the expression of another organic acid since RNA-seq analysis showed an upregulation of most of the TCA cycle-related genes (aceE, adhA, lpdA, prpC, icd, fumA, mqo, and maeB) in NFb50 medium (see Supplementary Table 1) and/or the activation of non-specific phosphatases, still uncharacterized in this bacterium. Based on our data, it could be inferred that H. seropedicae senses a Pi-deficiency state generated in NFb50 medium, where polyP was not degraded. Indeed, several authors suggested that polyP participates in the balance of the intracellular Pi (Nesmeyanova, 2000; Motomura et al., 2011; Grillo-Puertas et al., 2016).
Motility and Chemotaxis
H. seropedicae SmR1 has 41 genes involved in the chemotaxis pathway in its genome (Pedrosa et al., 2011). Figure 4A shows that 22 of 29 motility and chemotaxis DEGs (tar, tsr, and che genes) were upregulated in the NFb50 medium. A wide range (17 from 21 DEG) of flagella biosynthesis, assembly, and structure genes were also induced under the mentioned condition (Figure 4B). For instance, fliA gene, encoding a sigma factor necessary for the transcription of flagella and chemotaxis genes (Iriarte et al., 1995; Aizawa, 2013), was significantly induced (2.02). Upregulation of flagella and chemotaxis genes suggests that high Pi concentrations would increase motility and chemotaxis in H. seropedicae. Indeed, Figure 4C shows that SmR1 cells grown in NFb50 medium presented a significantly higher motility than those grown in NFb5. Moreover, the cell taxis to carbon sources (malate and glucose), root exudates, and phytohormones (salicylic and shikimic acids) was significantly higher in NFb50 medium than in NFb5 (Table 4). These results suggest that high polyP levels reached in the NFb50 condition could regulate motility in H. seropedicae. Consistently, Grillo-Puertas et al. (2015) reported that the presence of polyP is necessary for motility in uropathogenic E. coli isolated from acute prostatitis. In addition, previous studies with polyP-deficient strains demonstrated that the polymer is required for motility of several bacteria such as P. aeruginosa PAO1, V. cholerae 92A1552, Salmonella enterica serovar Typhimurium FIRN, Mycobacterium smegmatis, and Sulfolobales (Rashid et al., 2000; Shi et al., 2011; Recalde et al., 2021). Chemotaxis and motility differential effects observed with H. seropedicae gain attention considering their importance in the properties of beneficial soil microorganisms.
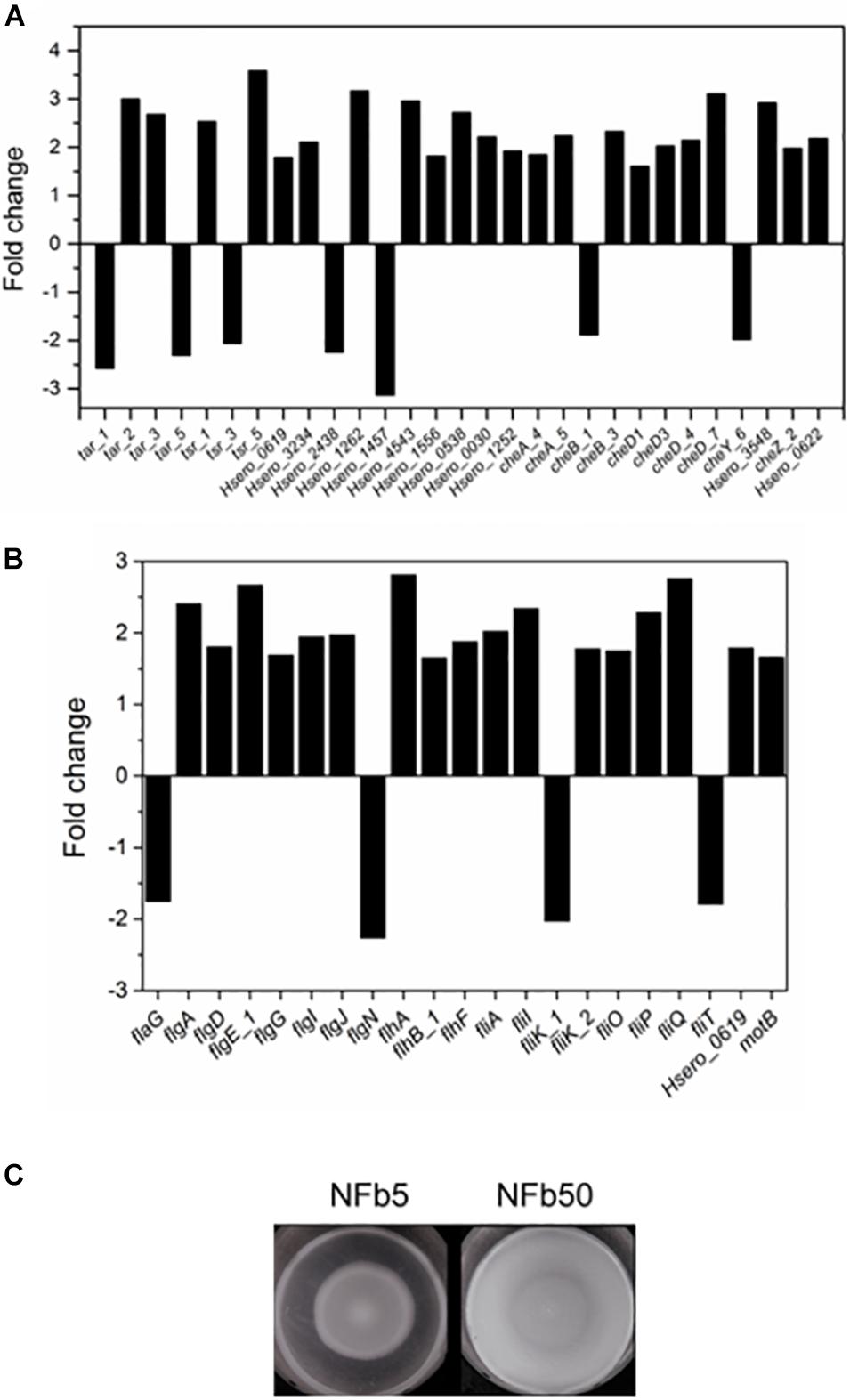
Figure 4. Chemotaxis and motility in NFb50 condition relative to NFb5 condition. Fold changes of differentially expressed genes related to chemotaxis (A) and motility (B), comparing both conditions. SmR1 strain cells were cultured on semisolid NFb5 and NFb50 agar plates (0.3%) and incubated for 48 h (C). In all cases, the results represent four independent experiments performed in triplicate.
Biofilm Formation and Adhesion
Genes related to biofilm formation and adhesion were downregulated in cells grown in NFb50 medium: type IV pili genes [pilI, pilJ, and pilQ, with fold change values of −8.71, −1.81 (p-value 0.05), and −1.83 (p-value 0.003), respectively], the entire cluster for lipopolysaccharide [LPS biosynthesis, comprising CDS Hsero_4197 to Hsero_4222 (see Supplementary Table 1)]; and a gene encoding a sugar phosphate isomerase involved in capsule formation, Hsero_3968 (−2.59). On the other hand, phoB was upregulated under the mentioned condition. It was previously demonstrated that PhoB activation was correlated with the inhibition of biofilm formation in P. fluorescens, P. aureofaciens, and E. coli (Monds et al., 2001, 2007; Grillo-Puertas et al., 2016). Thus, the obtained molecular changes suggest that biofilm formation would be impaired in the high Pi condition. To corroborate this hypothesis, biofilm formation was determined in NFb50 and NFb5 media. As expected, it was observed that biofilm formation on abiotic surfaces at 48 h by cells grown in NFb50 medium was around five-fold lower than that of cells grown in NFb5 (Supplementary Figure 1). The fact that biofilm formation was induced in NFb5 medium, where polyP was degraded, suggests that this event is necessary to trigger the cellular adhesion phenotype, as previously observed in K-12 and uropathogenic E. coli strains and G. diazotrophicus (Grillo-Puertas et al., 2012, 2015, 2018).
Besides the genes mentioned above involved in adhesion, rfbD and galE genes, which have been suggested to be involved in maize colonization (Balsanelli et al., 2010), were downregulated in NFb50 medium (−3.00 and −2.66, respectively). Thus, a plant–bacteria interaction assay was performed to compare the epiphytic and endophytic association capacity of H. seropedicae cells to maize roots in plant medium containing either 5 or 50 mM Pi (PM5 or PM50, respectively). Figure 5 shows that epiphytic cell population in the maize root surface was higher in PM5 in respect to PM50, in agreement with data obtained in abiotic adhesion assays. Endophytic population after 5 days of inoculation was approximately 1.5 orders of magnitude higher in cells grown in PM5 when compared to those grown in PM50. It should be noted that the number of planktonic cells was significantly lower in the PM5 medium than that in the PM50 (108 and 1010 UFC ml–1, respectively), suggesting that the microorganism prefers a sessile lifestyle when grown in the sufficient Pi condition. Taken together, in the sufficient Pi condition (5 mM Pi), H. seropedicae cells activate the molecular machinery necessary to improve bacterial adherence to surfaces and root colonization. This is an interesting finding and represents an important outcome to consider in plant–bacteria interaction assays.
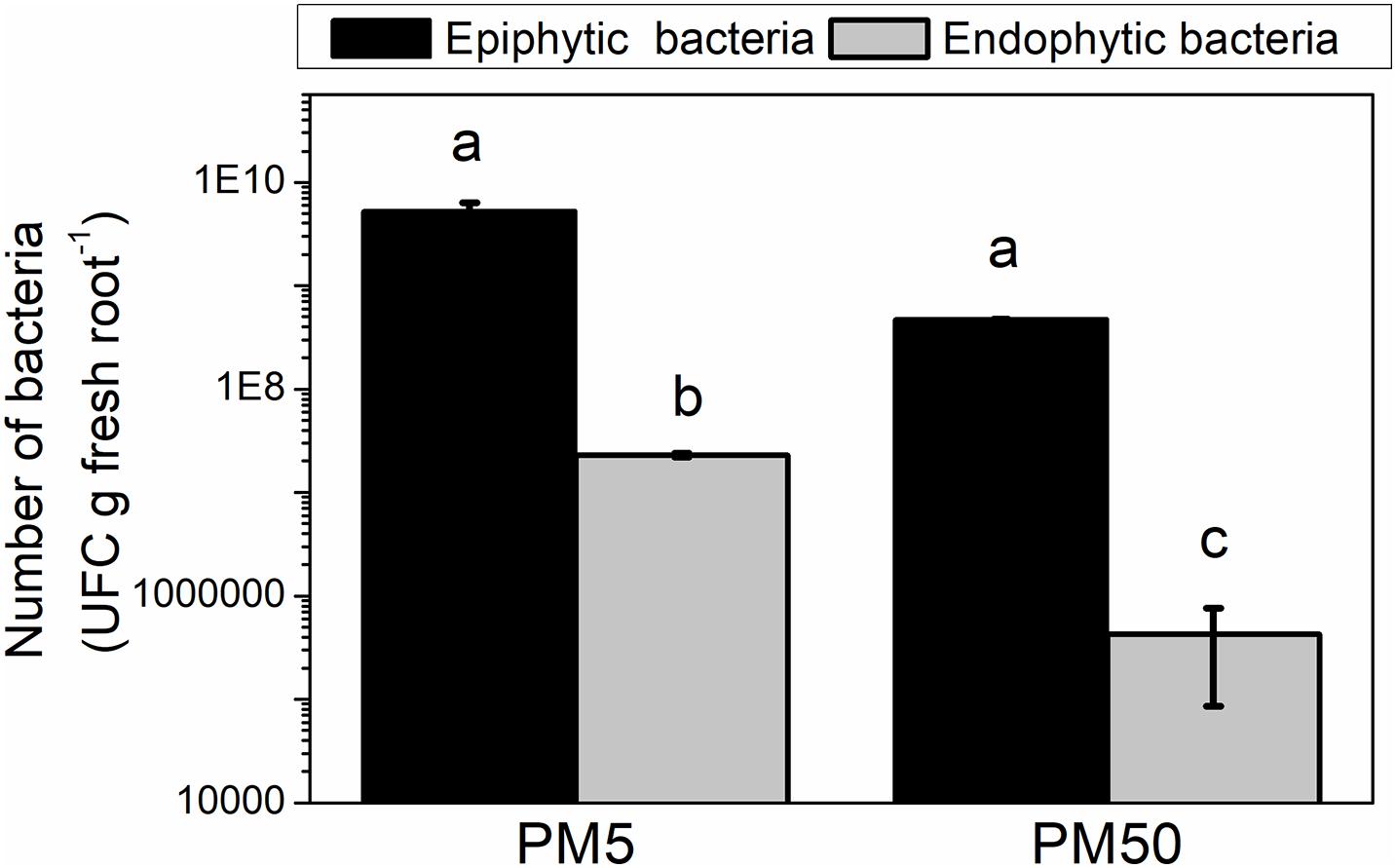
Figure 5. H. seropedicae colonization of maize seedlings in the high and sufficient Pi conditions. H. seropedicae SmR1 cells were grown in NFb50 medium for 24 h, washed, and inoculated on maize plantlets in PM5 or PM50 (10 replicates per condition). The number of epiphytic and endophytic bacteria was determined after 5 days of infection as mentioned in Materials and Methods. Results represent the mean ± SD of three independent experiments. Different letters indicate significant differences between conditions according to Tukey’s test with a p < 0.05.
Polyhydroxybutyrate Metabolism
Polyhydroxyalkanoates (PHAs) are a group of carbon and energy storage compounds accumulated by many bacteria to increase survival and stress tolerance in changing environments. Among PHAs, polyhydroxybutyrate (PHB) is a biodegradable polymer used as a possible substitute for petroleum-based plastics (Suriyamongkol et al., 2007; Li et al., 2016). It is well known that polyP form complexes with PHB, generating ion channels in lipid bilayers with high selectivity for cations (Lundgren et al., 1965; Reusch et al., 1995; Das et al., 1997; Pavlov et al., 2005b; Yilmaz and Beyatli, 2005; Zakharian and Reusch, 2007; Reusch, 2012). It has been reported that phbABC and phaC1 genes are responsible for PHB synthesis in H. seropedicae SmR1 (Catalan et al., 2007; Kadowaki et al., 2011; Tirapelle et al., 2013). Here, RNA-seq data related to PHB biosynthesis show that phbA2 and phbA genes, encoding two acetyl-CoA acyl transferases, and phbB gene, encoding a NADPH-dependent acetoacetyl-CoA reductase, were upregulated in cells grown in NFb50 medium [1.84 (p-value 0.0003), 2.5, and 1.51 (p-value 0.01), respectively] (Supplementary Table 1). In addition, phaC1 and phaC2, encoding putative PHB synthases, were increased 1.86-fold (p-value 0.0001) and 2.18-fold, respectively. The phaC2 is located in the operon with the pta-ackA genes, involved in AcP synthesis (Pedrosa et al., 2011). In cyanobacteria, AcP seems to play a role controlling PHA synthase (Sundaramoorthy et al., 2013). In the studied conditions, the co-expression of the pta-ackA with phaC2 genes suggests the participation of AcP in PHB metabolism in H. seropedicae.
To analyze the physiological response to the aforementioned genes’ differential expression, PHB levels were measured through time during H. seropedicae SmR1 growth in NFb5 and NFb50 (Figure 6). In both media, PHB levels were increased up to 12 h, and thereafter decreased. It should be noted that the highest values of PHB were reached in NFb50, in agreement with the RNA-seq data. In Azospirillum brasilense, accumulation of PHA has been related to chemotaxis, motility, and cell multiplication, becoming a more efficient inoculant (Kadouri et al., 2005; Fibach-Paldi et al., 2012). In fact, it has been described that PHB increased plant growth promotion in H. seropedicae and A. brasilense (Kadouri et al., 2003; Alves et al., 2019). However, previous studies with H. seropedicae SmR1 and A. brasilense Sp7 found no significant differences in epiphytic or endophytic root colonization, when inoculated with wild-type strains or their PHB-deficient mutants (Kadouri et al., 2003; Balsanelli et al., 2016; Alves et al., 2019). Even though a higher PHB peak was observed in NFb50 cells at 12 h, the synthesis and degradation profiles of the polymer were similar in both conditions. Further studies would be necessary to shed light on the possible relationship between PHB and the observed differential phenotypes. Moreover, the possibility of increasing polymer production in a given time and condition would represent a satisfactory approach for the production of environmentally friendly plastics.
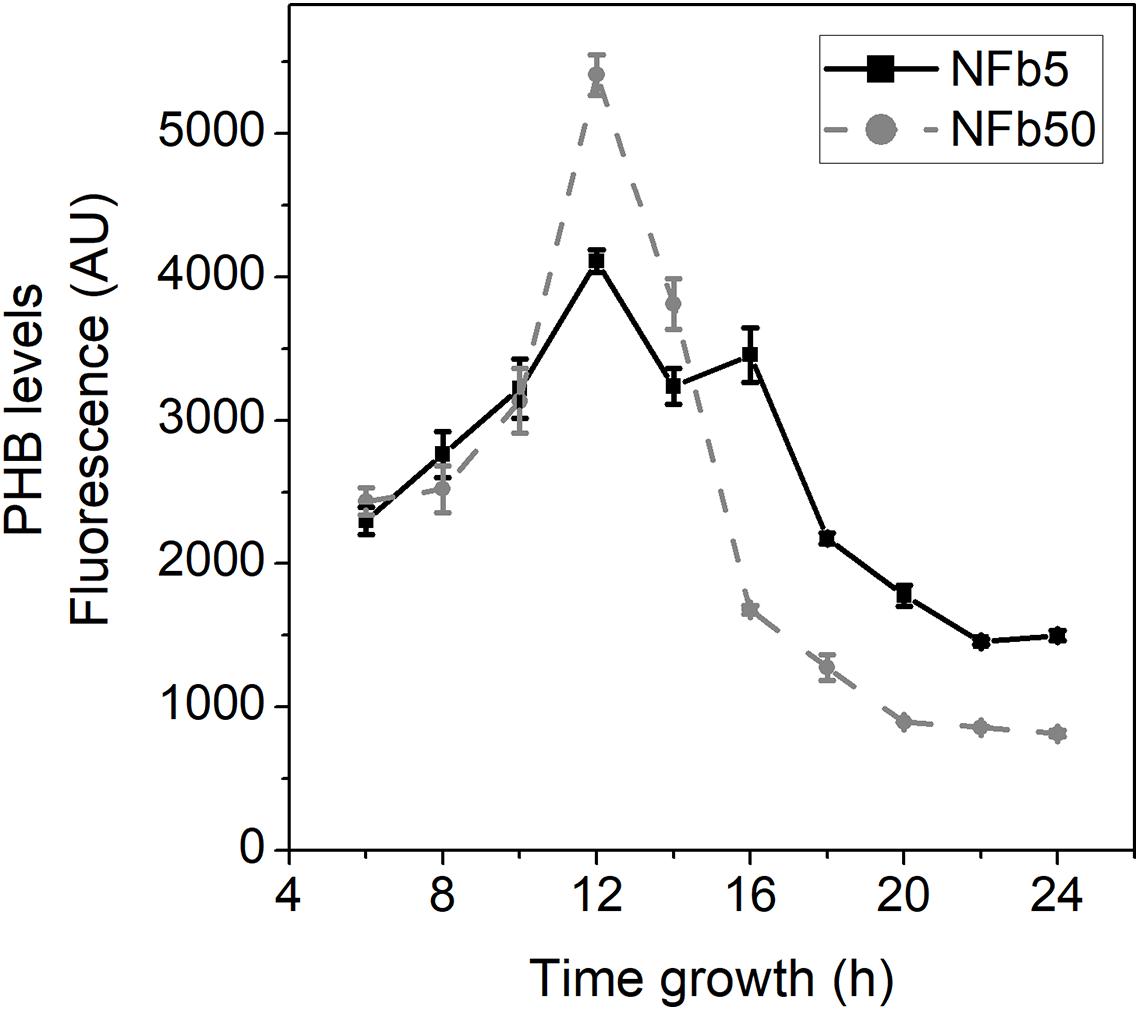
Figure 6. PHB levels in SmR1 cells grown in NFb50 and NFb5 condition. PHB measurements by flow cytometry in ΔphaC1 and SmR1 strains cultivated in NFb5 and NFb50 media. At the indicated times, culture aliquots were extracted, stained with Nile Red, and analyzed by flow cytometry as mentioned in the Materials and Methods section. Data are expressed as arbitrary units (AU) of fluorescence. Results represent the mean ± SD of three independent experiments with a p < 0.05.
Other Traits Affected by Pi
Nitrogen metabolism and its regulation have been extensively studied in H. seropedicae SmR1 (Chubatsu et al., 2012). Nitrogen assimilation in this bacterium is regulated by the NTR system, which is responsible for activating transcription of genes involved in alternative nitrogen sources in nitrogen-depleted conditions (Persuhn et al., 2000; Wassem et al., 2002; Schwab et al., 2007; Chubatsu et al., 2012). Supplementary Table 1 shows that H. seropedicae cells grown in NFb50 medium induced some of the NTR system genes such as ntrC (2.63), ntrB [1.99 (p-value 0.0006)], and glnA [1.63 (p-value 0.01)] and the operon amtBglnK [2.24 and 1.54 (p-value 0.006)]. Also, the gene involved in ammonium uptake amtH (4.60) was induced. Considering that, in the present work, cells were grown in sufficient nitrogen conditions, differential expression of these genes could be in response to unknown mechanisms that may be related to functions other than nitrogen/nitrate metabolism in the high Pi condition. For instance, it has been reported that an increase in AcP could activate NtrC, inducing Ntr regulon in several bacteria (Feng et al., 1992; Verhamme et al., 2002), since, in certain conditions, this molecule is sufficient for direct phosphorylation of two-component response regulators (Kim et al., 1996; Klein et al., 2007).
ATP-binding cassette (ABC) transporters catalyze the translocation of various substrates across the bacterial membrane. They enable the uptake of nutrients and important molecules and facilitate the extrusion of toxins (Dawson and Locher, 2006). The RNA-seq analysis showed a significant regulation of ABC-transporter genes (42 DEGs) in response to Pi concentration, with 16 being downregulated and 26 being upregulated in NFb50 medium (Supplementary Table 1). Among the downregulated genes, the most relevant were those involved in carbohydrate and ion transport (i.e., glycerol-3-phosphate, Fe3+, and sulfate/molybdate transporters). On the other hand, among the upregulated ABC transporters genes, the operon tauABC, responsible for taurine (aliphatic organosulfonate) transport inside the cell for further degradation into alanine, was significantly induced (fold change 4.5–10). The importance of this sulfonate transport system has been described for several bacterial species, such as S. Typhimurium (Turnbull and Surette, 2010), P. aeruginosa (Hummerjohann et al., 1998), Bacillus subtilis (Grundy and Henkin, 1998), and Acidithiobacillus ferrooxidans (Valdés et al., 2003). In addition, sulfated metabolites have been implicated in the interactions between bacteria and their eukaryotic hosts, including species of the plant symbiont genus Rhizobium (Cronan and Keating, 2002), Mycobacterium tuberculosis (Mougous et al., 2002), and Xanthomonas oryzae (Da Silva et al., 2004). Several DEGs predicted for amino acid transport and metabolism were upregulated in NFb50 medium (livH_4, livK_5, livK_3, livF_1, Hsero_4792, livK_1, and hisJ_1), together with the ABC transport genes for putrescine (potCHDA, Hsero_1078-1079-1080-1081, and Hsero_0600). Putrescine or 1,4-diaminobutane is a biogenic polyamine present in nearly all living cells and is involved in bacterial response to osmotic stress (Miller and Wood, 1996). The rapid bacterial adaptation to the osmolarity would facilitate the colonization of the rhizosphere (Miller and Wood, 1996). Also, the amount of this compound in plant cells increases in some stress situations (Flores and Galston, 1982). Therefore, putrescine transporters could also be involved in the bacterial protection from the putrescine produced by the colonized plant. Interestingly, three multidrug ABC transporter genes (mdlB, Hsero_4073, and Hsero_3566) were also upregulated by 1.60 (p-value 0.03), 1.57 (p-value 0.008), and 1.58 (p-value 0.03), respectively. Multidrug transporters mediate the extrusion of structurally unrelated drugs from prokaryotic and eukaryotic cells (Blackmore et al., 2001). Upregulation of these transporters would be important to H. seropedicae defense against different compounds present in the rhizosphere. According to the observed robust response of ABC transporter systems, it can be suggested that Pi is an important signal regulating bacterial transport processes in response to environmental conditions.
Central metabolism requires a fine regulation to ensure optimal use of carbon sources and proper allocation of resources to the metabolic pathways (Neidhardt et al., 1990; Heinrich and Schuster, 1998; Karp et al., 2007). It has been reported that polyP acts as a buffer for free Pi level (Kornberg et al., 1999). Since Pi buffering is essential for general metabolism, the synthesis or degradation of the polymer has been associated with the regulation of primary metabolism and general fitness in prokaryotic and eukaryotic cells (Freimoser et al., 2006; Schurig-Briccio et al., 2008, 2009a,b; Varela et al., 2010; Livermore et al., 2016). Within carbon metabolism, the tricarboxylic acid (TCA) cycle is an amphibolic pathway involved in energy production and precursor biosynthesis in aerobic organisms (Sauer and Eikmanns, 2005). Through its anabolic function, the TCA cycle produces 2-oxoglutarate and oxaloacetate as precursors of the glutamate family (glutamate, glutamine, arginine, and proline) and the aspartate family (aspartate and asparagine) of amino acids, respectively (Bott, 2007). Before it enters the TCA cycle, acetyl-CoA is generated by the pyruvate dehydrogenase complex (PDC) (Patel and Roche, 1990). Here, genes encoding for the pyruvate dehydrogenase components (aceE1, lpdA_1, lpdA_2, and aceF) were upregulated in NFb50 growing cells [6.69, 2.55, 2.00, and 1.56 (p-value 0.004), respectively]. In addition, most of the genes encoding for TCA cycle enzymes were upregulated in the high Pi condition, such as prpC (11.01), acnA_1 [1.46 (p-value 0.02)], acnA_2 [1.66 (p-value 0.003)], icd_2 (2.57), sucB [1.68 (p-value 0.001)], lpdA_1 (2.55), lpdA_2 (2.00), sucD [1.77 (p-value 0.001)], fumA (2.09), and mqo (4.59). No significant DEGs related to the oxidative phosphorylation pathway were found, suggesting that the upregulation of the TCA cycle genes in the high Pi condition would supply intermediates for biosynthetic pathways. Actually, several genes related to amino acid metabolism were upregulated in the aforementioned condition. For instance, ansB_1, argJ, argE, argH, Hsero_0061, Hsero_4778, and Hsero_4377 genes, involved in arginine and proline metabolism; ansB_1, ansB_2, gltB, gltD, and goaG genes, involved in alanine, aspartate and glutamate metabolism; thrC and thrA genes belonging to threonine metabolism; and dadA, Hsero_0324, Hsero_1676, Hsero_1447, Hsero_0324, Hsero_1684, Hsero_2240, and Hsero_4778 from phenylalanine metabolism (see Supplementary Table 1). In particular, Hsero_4778 gene, described to be essential for in vitro growth of H. seropedicae (Rosconi et al., 2016), encoded for an enzyme that catalyzes the interconversion of pyruvate and D-glutamate to D-alanine and 2-oxoglutarate, providing a carbon skeleton for the TCA cycle. Moreover, the TCA cycle shares common intermediates with the glyoxylate cycle, with both pathways being coordinately regulated. The glyoxylate shunt is known to be upregulated when acetyl-CoA is a direct product of a metabolic pathway, for example, via degradation of acetate, fatty acids, and alkanes (Maloy and Nunn, 1982; Chung et al., 1993). Note that glcD, glcE, and aceA genes, coding for the specific enzymes of the glyoxylate cycle, were downregulated in the high Pi condition (−5.42, −5.39, and −7.60, respectively). There is evidence suggesting that the glyoxylate shunt also plays an important role in pathogenesis and the response to oxidative stress in microorganisms (Ahn et al., 2016). Although it is not possible to predict metabolic fluxes from transcriptomic data, these results highlight the fact that environmental Pi is capable of modulating the expression of most of the genes from certain central metabolism pathways in H. seropedicae.
Many bacteria respond to oxidative stress by changing the expression of genes encoding enzymes that regulate the cellular concentration of superoxide and hydrogen peroxide, the formation of iron–sulfur clusters, and the reparation of oxidative damage to DNA (Imlay, 2008; Gray and Jakob, 2015). Here, different stress global regulators, such as soxR (-4.91), norR (-4.55), acrR (-3.10), ompR (-2.67), and risA (-2.06); two genes involved in the SOS response for DNA repair, recX (-2.61) and umuD (-2.21); and a gene encoding a superoxide dismutase protein, sodB (-1.98), were downregulated in NFb50 medium (Supplementary Table 1). Also, around 11 LysR-type transcriptional regulator (LTTR) genes were significantly downregulated in the same medium (Hsero_3928, Hsero_4799, Hsero_3540, cysB, Hsero_2318, Hsero_4110, Hsero_0047, Hsero_4802, Hsero_4528, Hsero_3808, and Hsero_4346). LTTRs are a well-characterized group of transcriptional regulators that control key adaptive phenotypes, such as virulence, biofilm formation, quorum sensing, motility, signaling, secondary metabolite production, and oxidative stress (Russell et al., 2004; Tropel and van der Meer, 2004; Heroven and Dersch, 2006; Maddocks and Oyston, 2008; O’Grady et al., 2011). In addition, genes encoding different bacterial chaperones, such as ibpA, groES, htpX, Hsero_1726, Hsero_0435, and hfj, were also significantly downregulated in NFb50 (Supplementary Table 1). Our results indicate that an important stress response is observed only in cells grown in NFb5 medium (low polyP condition), suggesting that cells sense and respond to the usual deprived state of the early stationary phase. It was widely reported that polyP protects bacteria from cellular stresses (Jahid et al., 2006; Rao et al., 2009; Nikel et al., 2013; Alcántara et al., 2014; Gray et al., 2014). PolyP can act directly by a protein-stabilizing chaperone activity and by interactions with redox-active metals, or indirectly controlling stress response pathways (Gray and Jakob, 2015). Previous reports from our laboratory indicate that high polyP levels in stationary phase improve bacterial fitness and resistance to different stresses (Schurig-Briccio et al., 2009a; Grillo-Puertas et al., 2014, 2018). Thus, the downregulation in the NFb50 condition of these stress-related components may be due to the chaperone capacity of the polyP that could provide the cytoplasmic protein quality to overcome a stress condition or due to the fact that cells grown in high Pi medium do not sense the typically early stationary phase-stress condition (Zhaoa and Drlica, 2014).
Conclusion
The present study provides a comprehensive analysis of the H. seropedicae SmR1 transcriptome in Pi differential conditions. Figure 7 shows a general scheme of some of the molecular adaptations that occurred in cells grown in medium containing 50 mM Pi when compared to those grown in 5 mM Pi. Transcriptional analysis revealed that 670 genes were differentially expressed, with 57% repressed (i.e., adhesion and stress response) and 43% induced (i.e., Pi metabolism, bacterial flagella biosynthesis, chemotaxis, energy production processes, and PHB metabolism) in the high Pi condition. Based on transcriptomic and phenotypic data, H. seropedicae cells grown in high Pi medium seem to have a better general fitness, which could be an important feature to consider when the bacterium is used as plant inoculant. On the other hand, under sufficient Pi concentrations, cell adhesion and colonization capacity increased, leading to a higher root colonization. The present data shed light on the bacterial behavior in respect to changes in environmental Pi concentrations that could be useful for the development of strategies to benefit their biotechnological and agricultural potential.
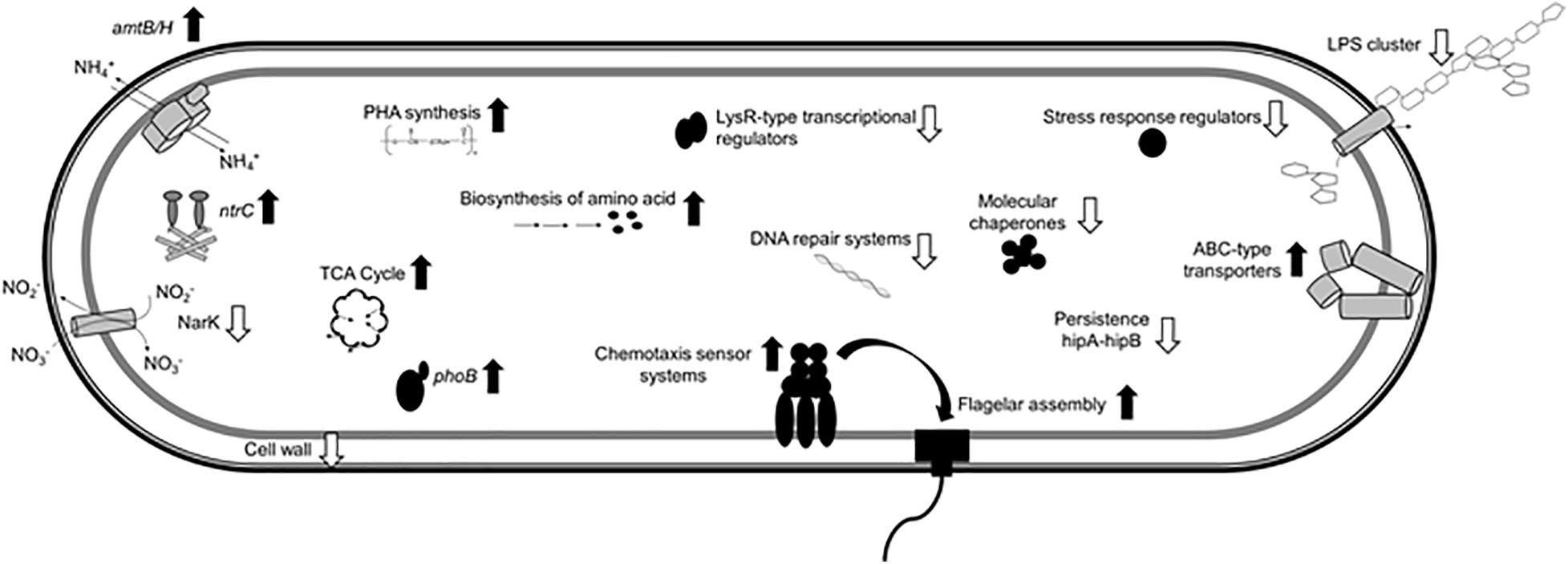
Figure 7. Molecular differences of H. seropedicae cells in the high Pi condition relative to the sufficient Pi condition. The scheme shows induced (black up arrows) and repressed (white down arrows) traits in H. seropedicae SmR1.
Data Availability Statement
The datasets presented in this study can be found in online repositories. The names of the repository/repositories and accession number(s) can be found below: https://www.ncbi.nlm.nih.gov/geo/, GSE168341.
Author Contributions
MG-P, ES, and VAR conceived and designed research. MG-P, JMV, VP, MT-S, EH, and FT conducted experiments. LB-S contributed to DESeq analysis. RP contributed to general ideas and formulation of the research aims. MG-P, JV, and VAR analyzed the data. FP, ES, and VAR provided financial support. MG-P, JMV, VAR, and ES wrote the manuscript. All authors read, discussed, and approved the manuscript.
Funding
This work was supported by grants from INCT-FBN/CNPq, CONICET (PIP 2015-611), and Universidad Nacional de Tucumán (PIUNT2018-26/D627). MG-P was supported by a postdoctoral fellowship from CONICET and the Programa Nacional de Pós-Doutorado (PNPD-2747/2011).
Conflict of Interest
The authors declare that the research was conducted in the absence of any commercial or financial relationships that could be construed as a potential conflict of interest.
Acknowledgments
We thank Roseli Prado and Valter Baura (Department of Biochemistry and Molecular Biology, UFPR, Curitiba, Brasil) for technical assistance and Rafael Gutiérrez (CCT CONICET Tucumán, Argentina) for maintenance of different lab equipment.
Supplementary Material
The Supplementary Material for this article can be found online at: https://www.frontiersin.org/articles/10.3389/fmicb.2021.666277/full#supplementary-material
Footnotes
References
Ahn, S., Jung, J., Jang, I. A., Madsen, E. L., and Park, W. (2016). Role of glyoxylate shunt in oxidative stress response. J. Biol. Chem. 291, 11928–11938. doi: 10.1074/jbc.M115.708149
Aizawa, S. I. (2013). “Flagella,” in Brenner’s Encyclopedia of Genetics, 2nd Edn, eds S. Maloy and K. Hughes (San Diego, CA: Academic Press), 60–63.
Albi, T., and Serrano, A. (2016). Inorganic polyphosphate in the microbial world. emerging roles for a multifaceted biopolymer. World J. Microbiol. Biotechnol. 32:27. doi: 10.1007/s11274-015-1983-2
Alcántara, C., Blasco, A., Zuniga, M., and Monedero, V. (2014). Polyphosphate in Lactobacillus: accumulation and involvement in resistance against stress. Appl. Environ. Microbiol. 80, 1650–1659. doi: 10.1128/aem.03997-13
Alves, L. P., Plucani do Amaral, F., Kim, D., Todo Bom, M., Piñero Gav dia, M., Silvano Teixeira, C., et al. (2019). Importance of poly-3-hydroxybutyrate metabolism to the ability of herbaspirillum seropedicae to promote plant growth. Appl. Environ. Microbiol. 6, e02586–18. doi: 10.1128/AEM.02586-18
Alves, L. P. S., Almeida, A. T., Cruz, L. M., Pedrosa, F. O., de Souza, E. M., Chubatsu, L. S., et al. (2017). A simple and efficient method for poly-3-hydroxybutyrate quantification in diazotrophic bacteria within 5 minutes using flow cytometry. Braz. J. Med. Biol. Res. 50:e5492. doi: 10.1590/1414-431X20165492
Aschar-Sobbi, R., Abramov, A. Y., Diao, C., Kargacin, M. E., Kargacin, G. J., French, R. J., et al. (2008). High sensitivity, quantitative measurements of polyphosphate using a new DAPI-based approach. J. Fluoresc. 18, 859–866. doi: 10.1007/s10895-008-0315-4
Bagyaraj, D. J., Krishnaraj, P. U., and Khanuja, S. P. (2000). Mineral phosphate solubilization: agronomic implication, mechanism and molecular genetics. Proc. Indian natn. Sci. Acad. 66, 69–82. doi: 10.16943/ptinsa/2014/v80i2/55116
Baldani, J. I., Reis, V. M., Videira, S. S., Boddey, L. H., and Divan Baldani, V. L. (2014). The art of isolating nitrogen-fixing bacteria from non-leguminous plants using N-free semi-solid media: a practical guide for microbiologists. Plant Soil 384, 413–431. doi: 10.1007/s11104-014-2186-6
Balsanelli, E., Serrato, R. V., de Baura, V. A., Sassaki, G., Yates, M. G., Rigo, L. U., et al. (2010). Herbaspirillum seropedicae rfbB and rfbC genes are required for maize colonization. Environ. Microbiol. 12, 2233–2244. doi: 10.1111/j.1462-2920.2010.02187.x
Balsanelli, E., Tadra-Sfeir, M. Z., Faoro, H., Pankievicz, V. C., de Baura, V. A., Pedrosa, F. O., et al. (2016). H. seropedicae transcript profiles in maize rhizosphere. Environ. Microbiol. 18, 2343–2356. doi: 10.1111/1462-2920.12887
Balsanelli, E., Tuleski, T. R., de Baura, V. A., Yates, M. G., Chubatsu, L. S., Pedrosa Fde, O., et al. (2013). Maize root lectins mediate the interaction with Herbaspirillum seropedicae via N-Acetyl glucosamine residues of lipopolysaccharides. PLoS One 8:e77001. doi: 10.1371/journal.pone.0077001
Bashan, Y., Kamnev, A., and de-Bashan, L. (2013). Tricalcium phosphate is inappropriate as a universal selection factor for isolating and testing phosphate-solubilizing bacteria that enhance plant growth: a proposal for an alternative procedure. Biol. Fertility Soils 49, 465–469. doi: 10.1007/s00374-012-0737-7
Berney, M., Weilenmann, H. U., Ihssen, J., Bassin, C., and Egli, T. (2006). Specific growth rate determines the sensitivity of Escherichia coli to thermal, UVA, and solar disinfection. Appl. Environ. Microbiol. 72, 2586–2593. doi: 10.1128/AEM.72.4.2586-2593.2006
Bhattacharyya, P. N., and Jha, D. K. (2012). Plant growth-promoting rhizobacteria (PGPR): emergence in agriculture. World J. Microbiol. Biotechnol. 28, 1327–1350. doi: 10.1007/s11274-011-0979-9
Blackmore, C. G., McNaughton, P. A., and van Veen, H. W. (2001). Multidrug transporters in prokaryotic and eukaryotic cells: physiological functions and transport mechanisms. Mol. Membr. Biol. 18, 97–103. doi: 10.1080/09687680010030200
Bott, M. (2007). Offering surprises: TCA cycle regulation in Corynebacterium glutamicum. Trends Microbiol. 15, 417–425. doi: 10.1016/j.tim.2007.08.004
Catalan, A. I., Ferreira, F., Gill, P. R., and Batista, S. (2007). Production of polyhydroxyalkanoates by Herbaspirillum seropedicae grown with different sole carbon sources and on lactose when engineered to express the lacZ lacY genes. Enzyme Microb. Tech. 40, 1352–1357. doi: 10.1016/j.enzmictec.2006.10.008
Chekabab, S. M., Harel, J., and Dozois, C. M. (2014). Interplay between genetic regulation of phosphate homeostasis and bacterial virulence. Virulence 5, 786–793. doi: 10.4161/viru.29307
Chubatsu, L., Monteiro, R., Souza, E., Oliveira, M., Yates, M., Wassem, R., et al. (2012). Nitrogen fixation control in Herbaspirillum seropedicae. Plant Soil 356, 197–207. doi: 10.1007/s11104-011-0819-6
Chung, T., Resnik, E., Stueland, C., and LaPorte, D. C. (1993). Relative expression of the products of glyoxylate bypass operon: contributions of transcription and translation. J. Bacteriol. 175, 4572–4575. doi: 10.1128/jb.175.14.4572-4575
Correa-Deza, M. A., Grillo-Puertas, M., Salva, S., Rapisarda, V. A., Gerez, C. L., and Font de Valdez, G. (2017). Inorganic salts and intracellular polyphosphate inclusions play a role in the thermotolerance of the immunobiotic Lactobacillus rhamnosus CRL 1505. PLoS One 12:e0179242. doi: 10.1371/journal.pone.0179242
Cronan, G. E., and Keating, D. H. (2002). A Sinorhizobium melliloti sulfotransferase that modifies lipopolissacharide. J. Bacteriol. 186, 4168–4176. doi: 10.1128/JB.186.13.4168-4176
Da Silva, F. G., Shen, Y., Dardick, C., Burdman, S., Yadav, R. C., de Leon, A. L., et al. (2004). Bacterial genes involved in type I secretion and sulfation are required to elicit the rice Xa21-mediated innate immune response. Mol. Plant Microbe. Interact. 17, 593–601. doi: 10.1094/MPMI.2004.17.6.593
Dai, Z., Liu, G., Chen, H., Chen, C., Wang, J., Ai, S., et al. (2020). Long-term nutrient inputs shift soil microbial functional profiles of phosphorus cycling in diverse agroecosystems. ISME J. 14, 757–770. doi: 10.1038/s41396-019-0567-9
Das, S., Lengweiler, U. D., Seebach, D., and Reusch, R. N. (1997). Proof for a nonproteinaceous calcium-selective channel in Escherichia coli by total synthesis from (R)-3-hydroxybutanoic acid and inorganic polyphosphate. Proc. Natl. Acad. Sci. U.S.A. 94, 9075–9079. doi: 10.1073/pnas.94.17.9075
Dawson, R. J., and Locher, K. P. (2006). Structure of a bacterial multidrug ABC transporter. Nature 443, 180–185. doi: 10.1038/nature05155
Devine, K. M. (2018). Activation of the PhoPR-mediated response to phosphate limitation is regulated by wall teichoic acid metabolism in bacillus subtilis. Front. Microbiol. 9:2678. doi: 10.3389/fmicb.2018.02678
Döbereiner, J., Baldani, V. L. D., and Baldani, J. I. (1995). Como Isolar e Identificar Bacterias Diazotroficas de Plantas no-Leguminosas. Brasılia: EMBRAPA–SPI.
Edi–Premono, M., Moawad, M. A., and Vleck, P. L. G. (1996). Effect of phosphate solubilizing Pseudomonas putida on the growth of maize and its survival in the rhizosphere. Indonesian J. Crop Sci. 11, 13–23.
Egener, T., Hurek, T., and Reinhold-Hurek, B. (1999). Endophytic expression of nif genes of Azoarcus sp. Strain BH72 in rice roots. Mol. Plant Microbe Interact. 12, 813–819. doi: 10.1094/MPMI.1999.12.9.813
Estrada, G. A., Baldani, V. L. D., de Oliveira, D. M., Urquiaga, S., and Baldani, J. I. (2013). Selection of phosphate-solubilizing diazotrophic Herbaspirillum and Burkholderia strains and their effect on rice crop yield and nutrient uptake. Plant Soil 369, 115–129. doi: 10.1007/s11104-012-1550-7
Fabiañska, I., Gerlach, N., Almario, J., and Bucher, M. (2018). Plant-mediated effects of soil phosphorus on the root associated fungal microbiota in Arabidopsis thaliana. New Phytol. 221, 2123–2137. doi: 10.1111/nph.15538
Feng, J., Atkinson, M. R., McCleary, W., Stock, J. B., Wanner, B. L., and Ninfa, A. J. (1992). Role of phosphorylated metabolic intermediates in the regulation of glutamine synthetase synthesis in Escherichia coli. J. Bacteriol. 174, 6061–6070. doi: 10.1128/jb.174.19.6061-6070.1992
Fibach-Paldi, S., Burdman, S., and Okon, Y. (2012). Key physiological properties contributing to rhizosphere adaptation and plant growth promotion abilities of Azospirillum brasilense. FEMS Microbiol. Lett. 326, 99–108. doi: 10.1111/j.1574-6968.2011.02407.x
Flores, H. E., and Galston, A. W. (1982). Polyamines and plant stress: activation of putrescine biosynthesis by osmotic shock. Science 217, 1259–1261. doi: 10.1126/science.217.4566.1259
Freimoser, F. M., Hürlimann, H. C., Jakob, C. A., Werner, T. P., and Amrhein, N. (2006). Systematic screening of polyphosphate (polyP) levels in yeast mutant cells reveals strong interdependence with primary metabolism. Genome Biol. 7:R109. doi: 10.1186/gb-2006-7-11-r109
Glick, B. R., Patten, C. L., Holguin, G., and Penrose, D. M. (1999). Biochemical and Genetic Mechanisms Used by Plant Growth Promoting Bacteria. London: Imperial College Press, doi: 10.1142/p130
Gray, M. J., and Jakob, U. (2015). Oxidative stress protection by polyphosphate-new roles for an old player. Curr. Opin. Microbiol. 24, 1–6. doi: 10.1016/j.mib.2014.12.004
Gray, M. J., Wholey, W. Y., Wagner, N. O., Cremers, C. M., Mueller-Schickert, A., Hock, N. T., et al. (2014). Polyphosphate is a primordial chaperone. Mol. Cell 53, 689–699. doi: 10.1016/j.molcel.2014.01.012
Grillo-Puertas, M., Rintoul, M. R., and Rapisarda, V. (2016). PhoB activation in non-limiting phosphate condition by the maintenance of high polyphosphate levels in the stationary phase inhibits biofilm formation in Escherichia coli. Microbiology-UK 162, 1000–1008. doi: 10.1099/mic.0.000281
Grillo-Puertas, M., Delaporte-Quintana, P., Pedraza, R. O., and Rapisarda, V. A. (2018). Intracellular polyphosphate levels in Gluconacetobacter diazotrophicus affect tolerance to abiotic stressors and biofilm formation. Microbes Environ. 28, 440–445. doi: 10.1264/jsme2.me18044
Grillo-Puertas, M., Martínez-Zamora, M. G., Rintoul, M. R., Soto, S. M., and Rapisarda, V. A. (2015). Environmental phosphate differentially affects virulence phenotypes of uropathogenic Escherichia coli isolates causative of prostatitis. Virulence 6, 608–617. doi: 10.1080/21505594.2015.1059561
Grillo-Puertas, M., Schurig-Briccio, L. A., Rodríguez-Montelongo, L., Rintoul, M. R., and Rapisarda, V. A. (2014). Copper tolerance mediated by polyphosphate degradation and low-affinity inorganic phosphate transport system in Escherichia coli. BMC Microbiol. 14:72. doi: 10.1186/1471-2180-14-72
Grillo-Puertas, M., Villegas, J. M., Rintoul, M. R., and Rapisarda, V. A. (2012). Polyphosphate degradation in stationary phase triggers biofilm formation via LuxS quorum sensing system in Escherichia coli. PLoS One 7:e50368. doi: 10.1371/journal.pone.0050368
Grundy, F. J., and Henkin, T. M. (1998). The S box regulon: a new global transcription termination control system for methionine and cysteine biosynthesis genes in gram-positive bacteria. Mol. Microbiol. 30, 737–749. doi: 10.1046/j.1365-2958.1998.01105.x
Gupta, A., Felder, M., Verma, V., Cullum, J., and Qazi, G. N. (1999). A mutant of Gluconobacter oxydans deficient in gluconic acid dehydrogenase. FEMS Microbiol. Lett. 179, 501–506. doi: 10.1111/j.1574-6968.1999.tb08769.x
Heinrich, R., and Schuster, S. (1998). The modeling of metabolic systems. Structure, control and optimality. Biosystems 47, 61–77. doi: 10.1016/S0303-2647(98)00013-6
Heroven, A. K., and Dersch, P. (2006). RovM, a novel LysR-type regulator of the virulence activator gene rovA, controls cell invasion, virulence and motility of Yersinia pseudotuberculosis. Mol. Microbiol. 62, 1469–1483. doi: 10.1111/j.1365-2958.2006.05458.x
Hiruma, K., Gerlach, N., Sacristan, S., Nakano, R. T., Hacquard, S., Kracher, B., et al. (2016). Root Endophyte Colletotrichum tofieldiae confers plant fitness benefits that are phosphate status dependent. Cell 165, 464–474. doi: 10.1016/j.cell.2016.02.028
Hsieh, Y. J., and Wanner, B. L. (2010). Global regulation by the seven-component Pi signaling system. Curr. Opin. Microbiol. 13, 198–203. doi: 10.1016/j.mib.2010.01.014
Hummerjohann, J., Küttel, E., Quadroni, M., Ragaller, J., Leisinger, T., and Kertesz, M. A. (1998). Regulation of the sulfate starvation response in Pseudomonas aeruginosa: role of cysteine biosynthetic intermediates. Microbiology 144, 1375–1386. doi: 10.1099/00221287-144-5-1375
Imlay, J. A. (2008). Cellular defenses against superoxide and hydrogen peroxide. Annu. Rev. Biochem. 77, 755–776. doi: 10.1146/annurev.biochem.77.061606.161055
Iriarte, M., Stainier, I., Mikulskis, A., and Cornelis, G. R. (1995). fliA encoding sigma 28 in Yersinia enterocolitica. J. Bacteriol. 177, 2299–2304. doi: 10.1128/jb.177.9.2299-2304.1995
Jahid, I. K., Silva, A. J., and Benitez, J. A. (2006). Polyphosphate stores enhance the ability of Vibrio cholerae to overcome environmental stresses in a low-phosphate environment. Appl. Environ. Microbiol. 72, 7043–7049. doi: 10.1128/AEM.00924-06
Kadouri, D., Jurkevitch, E., and Okon, Y. (2003). Involvement of the reserve material poly-beta-hydroxybutyrate in Azospirillum brasilense stress endurance and root colonization. Appl. Environ. Microbiol. 69, 3244–3250. doi: 10.1128/aem.69.6.3244-3250.2003
Kadouri, D., Jurkevitch, E., Okon, Y., and Castro-Sowinski, S. (2005). Ecological and agricultural significance of bacterial polyhydroxyalkanoates. Crit. Rev. Microbiol. 31, 55–67. doi: 10.1080/10408410590899228
Kadowaki, M. A. S., Müller-Santos, M., Rego, F. G. M., Souza, E. M., Yates, M. G., Monteiro, R. A., et al. (2011). Identification and characterization of PhbF: a DNA binding protein with regulatory role in the PHB metabolism of Herbaspirillum seropedicae SmR1. BMC Microbiol. 11:230. doi: 10.1186/1471-2180-11-230
Karp, P. D., Keseler, I. M., Shearer, A., Latendresse, M., Krummenacker, M., Paley, S. M., et al. (2007). Multidimensional annotation of the Escherichia coli K-12 genome. Nucleic Acids Res. 35, 7577–7590. doi: 10.1093/nar/gkm740
Kim, S. K., Wilmes-Riesenberg, M. R., and Wanner, B. L. (1996). Involvement of the sensor kinase EnvZ in the in vivo activation of the response-regulator PhoB by acetyl phosphate. Mol. Microbiol. 22, 135–147. doi: 10.1111/j.1365-2958.1996.tb02663.x
Klassen, G., Pedrosa, F. O., Souza, E. M., Funayama, S., and Rigo, L. U. (1997). Effect of nitrogen compounds on nitrogenase activity in Herbaspirillum seropedicae strain SMR1. Can. J. Microbiol. 43, 887–891. doi: 10.1139/m97-129
Klein, A. H., Shulla, A., Reimann, S. A., Keating, D. H., and Wolfe, A. J. (2007). The intracellular concentration of acetyl phosphate in Escherichia coli is sufficient for direct phosphorylation of two-component response regulators. J. Bacteriol. 189, 5574–5581. doi: 10.1128/JB.00564-07
Kornberg, A., Rao, N. N., and Ault-Riché, D. (1999). Inorganic polyphosphate: a molecule of many functions. Annu. Rev. Biochem. 68, 89–125. doi: 10.1146/annurev.biochem.68.1.89
Lamarche, M. G., Dozois, C. M., Daigle, F., Caza, M., Curtiss, R., Dubreuil, J. D., et al. (2005). Inactivation of the pst system reduces the virulence of an avian pathogenic Escherichia coli O78 strain. Infect. Immun. 73, 4138–4145. doi: 10.1128/IAI.73.7.4138-4145.2005
Lamarche, M. G., Wanner, B. L., Crepin, S., and Harel, J. (2008). The phosphate regulon and bacterial virulence: a regulatory network connecting phosphate homeostasis and pathogenesis. FEMS Microbiol. Rev. 32, 461–473. doi: 10.1111/j.1574-6976.2008.00101.x
Leff, J. W., Jones, S. E., Prober, S. M., Barberan, A., Borer, E. T., Firn, J. L., et al. (2015). Consistent responses of soil microbial communities to elevated nutrient inputs in grasslands across the globe. Proc. Natl. Acad. Sci. U.S.A. 112, 10967–10972. doi: 10.1073/pnas.1508382112
Li, Z., Yang, J., and Loh, X. J. (2016). Polyhydroxyalkanoates: opening doors for a sustainable future. NPG Asia Mater. 8:e265. doi: 10.1038/am.2016.48
Livak, K. J., and Schmittgen, T. D. (2001). Analysis of relative gene expression data using real-time quantitative PCR and the 2(-Delta Delta C(T)) method. Methods 25, 402–408. doi: 10.1006/meth.2001.1262
Livermore, T. M., Jonathan, R. C., and Saiardi, A. (2016). Developmental accumulation of inorganic polyphosphate affects germination and energetic metabolism in Dictyostelium discoideum. PNAS 113, 996–1001. doi: 10.1073/pnas.1519440113
Love, M. I., Huber, W., and Anders, S. (2014). Moderated estimation of fold change and dispersion for RNA-seq data with DESeq2. Genome Biol. 15:550. doi: 10.1186/s13059-014-0550-8
Lundgren, D. G., Alper, R., Schnaitman, C., and Marchessault, R. H. (1965). Characterization of Poly-β-hydroxybutyrate extracted from different bacteria. J. Bacteriol. 89, 245–251. doi: 10.1128/JB.89.1.245-251.1965
Maddocks, S. E., and Oyston, P. C. (2008). Structure and function of the LysR-type transcriptional regulator (LTTR) family proteins. Microbiology 154, 3609–3623. doi: 10.1099/mic.0.2008/022772-0
Maloy, S. R., and Nunn, W. D. (1982). Genetic regulation of the glyoxylate shunt in Escherichia coli K-12. J. Bacteriol. 149, 173–180. doi: 10.1128/jb.149.1.173-180.1982
Matula, J. (2011). Relationship between phosphorus concentration in soil solution and phosphorus in shoots of barley. Plant Soil Environ. 57, 307–314. doi: 10.17221/149/2011-PSE
Miller, K. J., and Wood, J. M. (1996). Osmoadaptation by rhizosphere bacteria. Annu. Rev. Microbiol. 50, 101–136. doi: 10.1146/annurev.micro
Monds, R. D., Newell, P. D., Gross, R. H., and O’Toole, G. A. (2007). Phosphate-dependent modulation of c-di-GMP levels regulates Pseudomonas fluorescens Pf0-1 biofilm formation by controlling secretion of the adhesin LapA. Mol. Microbiol. 63, 656–679. doi: 10.1111/j.1365-2958.2006.05539.x
Monds, R. D., Silby, M. W., and Mahanty, H. K. (2001). Expression of the pho regulon negatively regulates biofilm formation by Pseudomonas aureofaciens PA147-2. Microbiology 42, 415–426. doi: 10.1046/j.1365-2958.2001.02641.x
Motomura, K., Hirota, R., Ohnaka, N., Okada, M., Ikeda, T., Morohoshi, T., et al. (2011). Overproduction of YjbB reduces the level of polyphosphate in Escherichia coli: a hypothetical role of YjbB in phosphate export and polyphosphate accumulation. FEMS Microbiol. Lett. 320, 25–32. doi: 10.1111/j.1574-6968.2011.02285.x
Mougous, J. D., Green, R. E., Williams, S. J., Brenner, S. E., and Bertozzi, C. R. (2002). Sulfotransferases and sulfatases in mycobacteria. Chem. Biol. 9, 767–776. doi: 10.1016/s1074-5521(02)00175-8
Nautiyal, C. S. (1999). An efficient microbiological growth medium for screening phosphate solubilizing microorganisms. FEMS Microbiol. Lett. 170, 265–270. doi: 10.1111/j.1574-6968.1999.tb13383.x
Neidhardt, F. C., Ingraham, J. L., and Schaechter, M. (1990). Physiology of the Bacterial Cell: A Molecular Approach. Sunderland, MA: Sinauer Associates.
Nikel, P. I., Chavarria, M., Martinez-Garcia, E., Taylor, A. C., and de Lorenzo, V. (2013). Accumulation of inorganic polyphosphate enables stress endurance and catalytic vigour in Pseudomonas putida KT2440. Microb. Cell Fact. 12:50. doi: 10.1186/1475-2859-12-50
O’Grady, E. P., Nguyen, D. T., Weisskopf, L., Eberl, L., and Sokol, P. A. (2011). The Burkholderia cenocepacia LysR-type transcriptional regulator ShvR influences expression of quorum-sensing, protease, type II secretion, and afc genes. J. Bacteriol. 193, 163–176. doi: 10.1128/JB.00852-10
O’Toole, G. A., and Kolter, R. (1998). Initiation of biofilm formation in Pseudomonas fluorescens WCS365 proceeds via multiple, convergent signaling pathways: a genetic analysis. Mol. Microbiol. 42, 449–461. doi: 10.1046/j.1365-2958.1998.00797.x
Patel, M. S., and Roche, T. E. (1990). Molecular biology and biochemistry of pyruvate dehydrogenase complexes. FASEB J. 4, 3224–3233. doi: 10.1096/fasebj.4.14.2227213
Pavlov, E., Zakharian, E., Bladen, C., Diao, C. T. M., Grimbly, C., Reusch, R. N., et al. (2005b). A large, voltage-dependent channel, isolated from mitochondria by water-free chloroform extraction. Biophys. J. 88, 2614–2625. doi: 10.1529/biophysj.104.057281
Pedrosa, F. O., and Yates, M. G. (1984). Regulation of nitrogen-fixation (nif) genes of Azospirillum brasilense by NifA and Ntr (Gln) type gene-products. FEMS Microbiol. Lett. 23, 95–101. doi: 10.1111/j.1574-6968.1984.tb01042.x
Pedrosa, F. O., Monteiro, R. A., Wassem, R., Cruz, L. M., Ayub, R. A., and Colauto, N. B. (2011). Genome of Herbaspirillum seropedicae strain SmR1, a specialized diazotrophic endophyte of tropical grasses. PLoS Genet. 7:e1002064. doi: 10.1371/journal.pgen.1002064
Persuhn, D. C., Souza, E. M., Steffens, M. B., Pedrosa, F. O., Yates, M. G., and Rigo, L. U. (2000). The transcriptional activator NtrC controls the expression and activity of glutamine synthetase in Herbaspirillum seropedicae. FEMS Microbiol. Lett. 192, 217–221. doi: 10.1111/j.1574-6968.2000.tb09385.x
Rao, N. N., and Torriani, A. (1990). Molecular aspects of phosphate transport in Escherichia coli. Mol. Microbiol. 4, 1083–1090. doi: 10.1111/j.1365-2958.1990.tb00682.x
Rao, N. N., Gomez-Garcia, M. R., and Kornberg, A. (2009). Inorganic polyphosphate: essential for growth and survival. Annu. Rev. Biochem. 78, 605–647. doi: 10.1146/annurev.biochem.77.083007.093039
Rashid, M. H., Rao, N. N., and Kornberg, A. (2000). Inorganic polyphosphate is required for motility of bacterial pathogens. J. Bacteriol. 182, 225–227. doi: 10.1128/jb.182.1.225-227.2000
Recalde, A., van Wolferen, M., Sivabalasarma, S., Albers, S. V., Navarro, C. A., and Jerez, C. A. (2021). The role of polyphosphate in motility, adhesion, and biofilm formation in sulfolobales. Microorganisms 9:193. doi: 10.3390/microorganisms9010193
Reusch, R. N. (2012). Physiological importance of poly-(R)-3-hydroxybutyrates. Chem. Biodivers. 9, 2343–2366. doi: 10.1002/cbdv.201200278
Reusch, R. N., Huang, R., and Bramble, L. L. (1995). Poly-3-hydroxybutyrate/polyphosphate complexes form voltage-activated Ca2+ channels in the plasma membranes of Escherichia coli. Biophys. J. 69, 754–766. doi: 10.1016/S0006-3495(95)79958-1
Rosconi, F., de Vries, S. P. W., Baig, A., Fabiano, E., and Grant, A. J. (2016). Essential genes for In Vitro growth of the Endophyte Herbaspirillum seropedicae SmR1 as revealed by transposon insertion site sequencing. Appl. Environ. Microbiol. 82, 6664–6671. doi: 10.1128/AEM.02281-16
Rossolini, G., Schippa, S., Riccio, M., Berlutti, F., Macaskie, L., and Thaller, M. (1998). Bacterial nonspecific acid phosphohydrolases: physiology, evolution and use as tools in microbial biotechnology. Cell Mol. Life Sci. CMLS 54, 833–850. doi: 10.1007/s000180050212
Rudat, A. K., Pokhrel, A., Green, T. J., and Gray, M. J. (2018). Mutations in Escherichia coli polyphosphate kinase that lead to dramatically increased in vivo polyphosphate levels. J. Bacteriol. 200, e697–17. doi: 10.1128/JB.00697-17
Russell, D. A., Byrne, G. A., O’Connell, E. P., Boland, C. A., and Meijer, W. G. (2004). The LysR-type transcriptional regulator VirR is required for expression of the virulence gene vapA of Rhodococcus equi ATCC 33701. J. Bacteriol. 186, 5576–5584. doi: 10.1128/JB.186.17.5576-5584.2004
Santos-Beneit, F., Rodríguez-García, A., Franco-Domínguez, E., and Martín, J. F. (2008). Phosphate-dependent regulation of the low- and high-affinity transport systems in the model actinomycete Streptomyces coelicolor. Microbiology 154, 2356–2370. doi: 10.1099/mic.0.2008/019539-0
Sauer, U., and Eikmanns, B. J. (2005). The PEP–pyruvate–oxaloacetate node as the switch point for carbon flux distribution in bacteria. FEMS Microbiol. Rev. 29, 765–794. doi: 10.1016/j.femsre.2004.11.002
Schurig-Briccio, L. A., Farías, R. N., Rintoul, M. R., and Rapisarda, V. A. (2009a). Phosphate-enhanced stationary-phase fitness of Escherichia coli is related to inorganic polyphosphate level. J. Bacteriol. 191, 4478–4481. doi: 10.1128/JB.00082-09
Schurig-Briccio, L. A., Farías, R. N., Rodríguez-Montelongo, L., Rintoul, M. R., and Rapisarda, V. A. (2009b). Protection against oxidative stress in Escherichia coli stationary phase by a phosphate concentration dependent genes expression. Arch. Biochem. Biophys. 483, 106–110. doi: 10.1016/j.abb.2008.12.009
Schurig-Briccio, L. A., Rintoul, M. R., Volentini, S. I., Farías, R. N., Baldoma, L., Badía, J., et al. (2008). A critical phosphate concentration in the stationary phase maintains ndh gene expression and aerobic respiratory chain activity in Escherichia coli. FEMS Microbiol. Lett. 284, 76–83. doi: 10.1111/j.1574-6968.2008.01188.x
Schwab, S., Souza, E. M., Yates, M. G., Persuhn, D. C., Steffens, M. B., Chubatsu, L. S., et al. (2007). The glnAntrBC operon of Herbaspirillum seropedicae is transcribed by two oppositely regulated promoters upstream of glnA. Can. J. Microbiol. 53, 100–105. doi: 10.1139/w06-113
Shi, T., Fu, T., and Xie, J. (2011). Polyphosphate deficiency affects the sliding motility and biofilm formation of mycobacterium smegmatis. Curr. Microbiol. 63:470. doi: 10.1007/s00284-011-0004-4
Nesmeyanova, M. A. (2000). Polyphosphates and enzymes of polyphosphate metabolism in Escherichia coli. Biochemistry 65, 309–314.
Souza, E. M., Pedrosa, F. O., Rigo, L. U., Machado, H. B., and Yates, M. G. (2000). Expression of the nifA gene of Herbaspirillum seropedicae: role of the NtrC and NifA binding sites and of the -24/-12 promoter element. Microbiology 146, 1407–1418. doi: 10.1099/00221287-146-6-1407
Sundaramoorthy, B., Kadiyala, G., and Muthuvelan, B. (2013). Production of Poly Hydroxybutyrates from cyanobacteria for the production of bioplastics. Algal Res. 2, 278–285. doi: 10.1016/j.algal.2013.03.002
Suriyamongkol, P., Weselake, R., Narine, S., Moloney, M., and Shah, S. (2007). Biotechnological approaches for the production of polyhydroxyalkanoates in microorganisms and plants - a review. Biotechnol. Adv. 2, 148–175. doi: 10.1016/j.biotechadv.2006.11.007
Tarafdar, J. C., and Claassen, N. (1988). Organic phosphorus compounds as a phosphorus source for higher plants through the activity of phosphatases produced by plant roots and microorganisms. Biol. Fertil. Soils 5, 308–312. doi: 10.1007/BF00262137
Tarafdar, J. C., and Jung, A. (1987). Phosphatase activity in the rhizosphere and its relation to the depletion of soil organic phosphorus. Biol. Fertil. Soils 3, 199–204. doi: 10.1007/BF00640630
Tirapelle, E. F., Muller-Santos, M., Tadra-Sfeir, M. Z., Kadowaki, M. A., Steffens, M. B., Monteiro, R. A., et al. (2013). Identification of proteins associated with polyhydroxybutyrate granules from Herbaspirillum seropedicae SmR1 - old partners, new players. PLoS One 8:e75066. doi: 10.1371/journal.pone.0075066
Tropel, D., and van der Meer, J. R. (2004). Bacterial transcriptional regulators for degradation pathways of aromatic compounds. Microbiol. Mol. Biol. Rev. 68, 474–500. doi: 10.1128/MMBR.68.3.474-500.2004
Turnbull, A. L., and Surette, M. G. (2010). Cysteine biosynthesis, oxidative stress and antibiotic resistance in Salmonella typhimurium. Res. Microbiol. 161, 643–650. doi: 10.1016/j.resmic.2010.06.004
Ulett, G. C., Webb, R. I., and Schembri, M. A. (2006). Antigen-43-mediated autoaggregation impairs motility in Escherichia coli. Microbiology 152, 2101–2110. doi: 10.1099/mic.0.28607-0
Valdés, J., Veloso, F., Jedlicki, E., and Holmes, D. (2003). Metabolic reconstruction of sulfur assimilation in the extremophile Acidithiobacillus ferrooxidans based on genome analysis. BMC Genomics 4:51. doi: 10.1186/1471-2164-4-51
Varela, C., Mauriaca, C., Paradela, A., Albar, J. P., Jerez, C. A., and Chávez, F. P. (2010). New structural and functional defects in polyphosphate deficient bacteria: A cellular and proteomic study. BMC Microbiol. 10:7. doi: 10.1186/1471-2180-10-7
Verhamme, D. T., Arents, J. C., Postma, P. W., Crielaard, W., and Hellingwerf, K. J. (2002). Investigation of in vivo cross-talk between key two-component systems of Escherichia coli. Microbiology 148, 69–78. doi: 10.1099/00221287-148-1-69
Wagh, J., Bhandari, P., Shah, S., Archana, G., and Kumar, G. N. (2014). Overexpression of citrate operon in Herbaspirillum seropedicae Z67 enhances organic acid secretion, mineral phosphate solubilization and growth promotion of Oryza sativa. Plant Soil 383, 73–86. doi: 10.1007/s11104-014-2161-2
Wanner, B. L., and Latterell, P. (1980). Mutants affected in alkaline phosphatase expression: evidence for multiple positive regulators of the phosphate regulon in Escherichia coli. Genetics 96, 353–366. doi: 10.1093/genetics/96.2.353
Wassem, R., Pedrosa, F. O., Yates, M. G., Rego, F. G., Chubatsu, L. S., Rigo, L. U., et al. (2002). Control of autogenous activation of Herbaspirillum seropedicae nifA promoter by the IHF protein. FEMS Microbiol. Lett. 212, 177–182. doi: 10.1111/j.1574-6968.2002.tb11263.x
Yilmaz, M., and Beyatli, Y. (2005). Poly-β-hydroxybutyrate (PHB) production by a Bacillus cereus MS strain in sugarbeet molasses. Zuckerindustrie 130, 109–112.
Zakharian, E., and Reusch, R. N. (2007). Haemophilus influenzae outer membrane protein P5 is associated with inorganic polyphosphate and polyhydroxybutyrate. Biophys. J. 92, 588–593. doi: 10.1529/biophysj.106.095273
Keywords: Herbaspirillum seropedicae, polyphosphate, adhesion, phosphate, plant-microbe interaction
Citation: Grillo-Puertas M, Villegas JM, Pankievicz VCS, Tadra-Sfeir MZ, Teles Mota FJ, Hebert EM, Brusamarello-Santos L, Pedraza RO, Pedrosa FO, Rapisarda VA and Souza EM (2021) Transcriptional Responses of Herbaspirillum seropedicae to Environmental Phosphate Concentration. Front. Microbiol. 12:666277. doi: 10.3389/fmicb.2021.666277
Received: 10 February 2021; Accepted: 29 April 2021;
Published: 10 June 2021.
Edited by:
Anton Hartmann, Ludwig Maximilian University of Munich, GermanyReviewed by:
Michael J. Gray, University of Alabama at Birmingham, United StatesStephanie A. Smith, Washington State University, United States
Copyright © 2021 Grillo-Puertas, Villegas, Pankievicz, Tadra-Sfeir, Teles Mota, Hebert, Brusamarello-Santos, Pedraza, Pedrosa, Rapisarda and Souza. This is an open-access article distributed under the terms of the Creative Commons Attribution License (CC BY). The use, distribution or reproduction in other forums is permitted, provided the original author(s) and the copyright owner(s) are credited and that the original publication in this journal is cited, in accordance with accepted academic practice. No use, distribution or reproduction is permitted which does not comply with these terms.
*Correspondence: Viviana A. Rapisarda, dml2aWFuYS5yYXBpc2FyZGFAZmJxZi51bnQuZWR1LmFy; Emanuel M. Souza, c291emFlbUB1ZnByLmJy