- 1Department of Earth and Planetary Sciences, Harvard University, Cambridge, MA, United States
- 2Princeton Environmental Institute, Princeton University, Princeton, NJ, United States
The reconstruction of modern and paleo-sulfur cycling relies on understanding the long-term relative contribution of its main actors; these include microbial sulfate reduction (MSR) and microbial sulfur disproportionation (MSD). However, a unifying theory is lacking for how MSR and MSD, with the same enzyme machinery and intimately linked evolutionary histories, perform two drastically different metabolisms. Here, we aim at shedding some light on the distribution, diversity, and evolutionary histories of MSR and MSD, with a focus on the Desulfobulbales as a test case. The Desulfobulbales is a diverse and widespread order of bacteria in the Desulfobacterota (formerly Deltaproteobacteria) phylum primarily composed of sulfate reducing bacteria. Recent culture- and sequence-based approaches have revealed an expanded diversity of organisms and metabolisms within this clade, including the presence of obligate and facultative sulfur disproportionators. Here, we present draft genomes of previously unsequenced species of Desulfobulbales, substantially expanding the available genomic diversity of this clade. We leverage this expanded genomic sampling to perform phylogenetic analyses, revealing an evolutionary history defined by vertical inheritance of sulfur metabolism genes with numerous convergent instances of transition from sulfate reduction to sulfur disproportionation.
Introduction
Microbial sulfur metabolisms drive the biogeochemical sulfur cycle over geologic timescales and couple it to the carbon, oxygen, and iron cycles (Johnston et al., 2005; Fike et al., 2015). Though the contribution of these metabolisms to net global carbon fixation rates is low relative to that of photosynthesis (e.g., Ward and Shih, 2019), carbon and sulfur fluxes through dissimilatory sulfur metabolisms are large and provide a significant control on net oxidation-reduction (redox) balance, in turn driving changes in Earth surface conditions (Berner and Raiswell, 1984; Berner and Canfield, 1989; Canfield, 2001; Fike et al., 2015). The main microbial metabolisms that drive the sulfur cycle are microbial sulfate reduction (MSR), sulfide oxidation (SO), and microbial sulfur disproportionation (MSD) (Johnston et al., 2005; Fike et al., 2015) (Figure 1). MSR couples the oxidation of simple organic molecules – including H2 for some organisms – to the reduction of sulfate, thiosulfate, and in some cases sulfite (Rosenberg et al., 2014, and references within). This reductive sulfur reaction promotes the burial of sedimentary pyrite and the remineralization of organic matter, which are major controls on Earth’s surface redox conditions (Jørgensen, 1982Canfield and Teske, 1996; Canfield, 2001; Fike et al., 2015). MSD, heavily involved in the oxidative sulfur cycle (Canfield, 2001), is a chemolithotrophic process by which sulfur species of intermediate valence – thiosulfate, sulfite, and/or elemental sulfur – act as both electron acceptor and donor, producing sulfate and sulfide as final products (Thamdrup et al., 1993; Canfield and Thamdrup, 1994; Canfield et al., 1998; Finster et al., 1998, 2013; Habicht and Canfield, 1998; Frederiksen and Finster, 2003; Finster, 2008; for a review on this metabolic pathway).
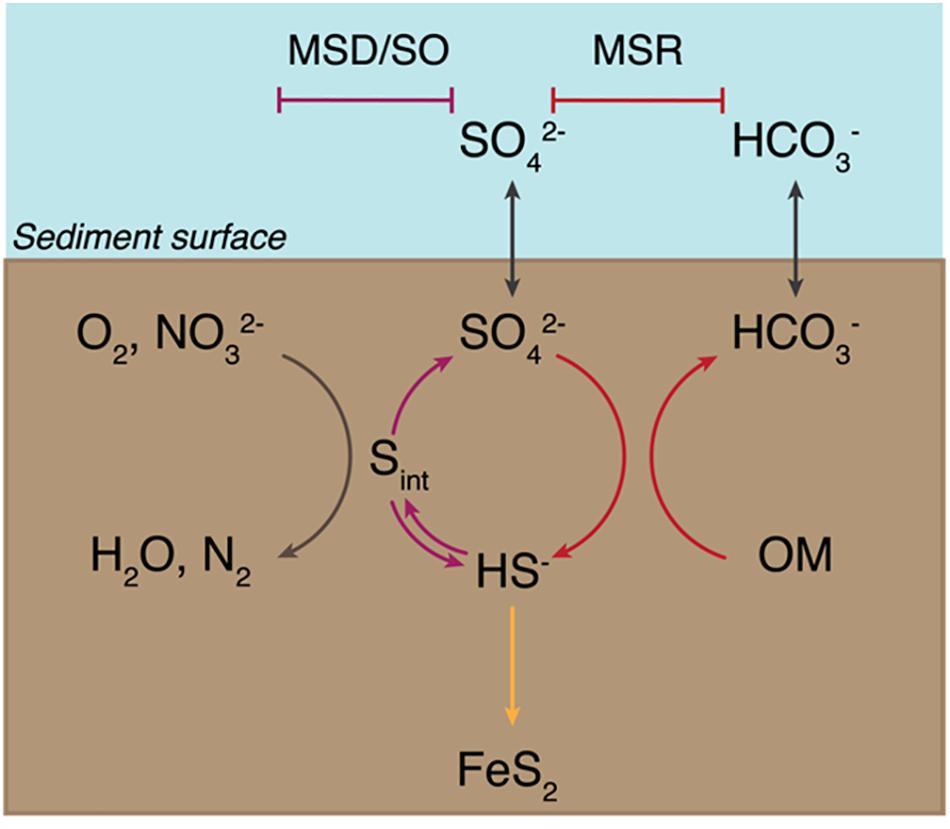
Figure 1. Sedimentary biogeochemical sulfur cycle. The sediment-water interface is indicated at the “Sediment surface.” First, seawater sulfate (SO42– ) diffuses into the sediments and enters the reductive sulfur cycle promoted by microbial sulfate reduction (MSR) (indicated with red arrows), which couples the reduction of sulfate to sulfide (HS– ) to the oxidation of organic matter (OM). A fraction of the produced sulfide precipitates with iron to form pyrite (FeS2) and is ultimately buried (yellow arrow). A portion of biogenic sulfide is oxidized – either biotically through sulfide oxidation (SO, shown with a blue arrow) or abiotically – using common oxidants – oxygen (O2) or nitrate (NO32– ) – to yield intermediate sulfur species (Sint). These are then disproportionated via microbial sulfur disproportionation (MSD) to release sulfate and sulfide (depicted with a purple arrow).
It has long been understood that MSR and MSD share core reactions and enzymatic machineries (Frederiksen and Finster, 2003), yet yield drastically different net pathways and a mechanistic argument for this conundrum is lacking. MSR is a respiratory pathway while MSD is fermentative and the energetic yields for each differ significantly. Sulfate reduction is a vastly more thermodynamically favorable metabolism than sulfur disproportionation under standard conditions (Finster, 2008; Wing and Halevy, 2014). As an example, in the absence of a sulfide sink, elemental sulfur disproportionation is effectively an endergonic reaction (Finster et al., 1998; Finster, 2008). It was then surprising when early pure culture experiments, as well as full genome sequencing and enzyme extract studies, revealed sulfate reduction and sulfur disproportionation share the same sulfur metabolism enzymes – sulfate adenylyltransferase, adenylylsulfate reductase (subunits A and B), dissimilatory sulfite reductase (subunits A, B, and C), and the sulfite reduction-associated DsrMKJOP complex – (Frederiksen and Finster, 2003; Finster et al., 2013). These are also dramatically different from the enzymes driving SO. It would thus be expected for sulfur disproportionating microbes to be capable of using sulfate as an electron acceptor in the presence of organic matter, and for sulfate reducers to disproportionate sulfur species of intermediate valence when conditions permit it. This expectation on the metabolic plasticity of sulfate reducers and sulfur disproportionators is not met and most sulfur disproportionators are incapable of MSR (Finster et al., 1998). To date, only two exceptions to this phenomenon have been reported: Desulfocapsa thiozymogenes (Janssen et al., 1996; Canfield et al., 1998; Rosenberg et al., 2014; Junghare and Schink, 2015) and Desulfobulbus propionicus (Widdel, 1980; Widdel and Pfennig, 1982; Kramer and Cypionka, 1989; Lovley and Phillips, 1994; Fuseler and Cypionka, 1995; Janssen et al., 1996; Böttcher et al., 2005; Sorokin et al., 2012; El Houari et al., 2017).
Understanding the similarities and differences between MSR and MSD carries geological importance. The antiquity of sulfate reduction has been dated back to the Archean using the MSR sulfur isotopic signature (Shen et al., 2001; Bontognali et al., 2012). On the other hand, the antiquity of sulfur disproportionation is harder to pinpoint. Chemical and isotopic signatures suggest the rise to ecological significance of MSD to be as late as the Mesoproterozoic (Johnston et al., 2005) or as early as the Archean (Philippot et al., 2007), and molecular clock work to refine the timing of emergence of MSD is lacking. For thermodynamic reasons, the ecological niche occupied by MSD requires low extracellular free sulfide concentrations. This is often accomplished through sulfide oxidation, sulfide scavenging with a metal, or polysulfide formation in alkaline conditions (Finster, 2008; Poser et al., 2013). For the most part, these niches became more pervasive after the Great Oxygenation Event (Canfield, 2001). This would then suggest that MSD rose to ecological significance later than MSR (Canfield and Teske, 1996). All in all, the shared nature of the MSR and MSD metabolic pathways coupled with full genome sequencing efforts have led to the inference that MSR and MSD are old and share a complex evolutionary history that is difficult to untangle.
The knowledge gap here resides with our understanding of MSD, as MSR has been far more thoroughly studied (Fry et al., 1986; LeGall and Fauque, 1988; Habicht and Canfield, 1998; Canfield, 2001; Shen et al., 2001; Habicht et al., 2002; Zane et al., 2010; Keller and Wall, 2011; Pereira et al., 2011; Leavitt et al., 2013; Parey et al., 2013; Wing and Halevy, 2014; Fike et al., 2015; Bradley et al., 2016; Bertran et al., 2018). That is, the true diversity and ecological distribution of sulfur disproportionation is still unknown owing to the lack of a unique enzymatic and genetic marker. Recently, increased efforts, technological advancements and sampling in metagenomics have expanded the ecological distribution and significance of MSR in modern sediments (Anantharaman et al., 2018; Vigneron et al., 2018;. However, and as noted above, efforts for MSD are lagging due largely to the absence of established marker genes to distinguish the capacity of MSD from MSR based on genome data alone (Anantharaman et al., 2018). It is essential to know the fraction of bacteria and archaea capable of MSD; this information will help determine the fluxes of material through the modern and ancient sedimentary sulfur cycling and refine the ecological niche occupied by sulfur disproprotionators.
An ideal case study for investigating the evolutionary relationship between MSR and MSD exists in the bacterial order Desulfobulbales. The Desulfobulbales are members of the Desulfobacterota (formerly Deltaproteobacteria) phylum (Figure 2) and include diverse and environmentally widespread members that play a central role in sulfur biogeochemical cycling in both modern and paleo-sediments (Fike et al., 2015; Figure 1). The Desulfobulbales were first described in 1980 when Widdel and colleagues described Desulfobulbus, the type genus of the order (Widdel, 1980; Kuever et al., 2005), but now consist of at least three family-level clades spanning at least ten genera (Figure 3). Members of the Desulfobulbales order have been described by a wide a range of morphological and chemotaxonomic properties (Rosenberg et al., 2014), and while they have been isolated from various sources – freshwater, marine environments, brackish water, and haloalkaline environments – most are mesophilic bacteria and all isolates are strictly anaerobic (Kuever, 2014). The Desulfobulbales order also includes the recently discovered filamentous “cable bacteria” (Kjeldsen et al., 2019), which have been shown to link redox processes across sediment layers separated by distances over 1 cm via long-distance electron transport (Müller et al., 2016) and may even be capable of sulfur disproportionation under some conditions (Müller et al., 2020). However, cable bacteria have so far resisted isolation in pure culture, preventing detailed physiological characterization (Pfeffer et al., 2012; Schauer et al., 2014; Bjerg et al., 2016; Kjeldsen et al., 2019). As a result, the capacity of cable bacteria to disproportionate (or not) has not been resolved in isolation from other metabolic pathways. Since the capacity for MSR and MSD cannot currently be distinguished from genome content alone (e.g., Anantharaman et al., 2018), we have chosen to omit cable bacteria and other organisms lacking pure culture characterization as their genome would potentially bias our analysis.
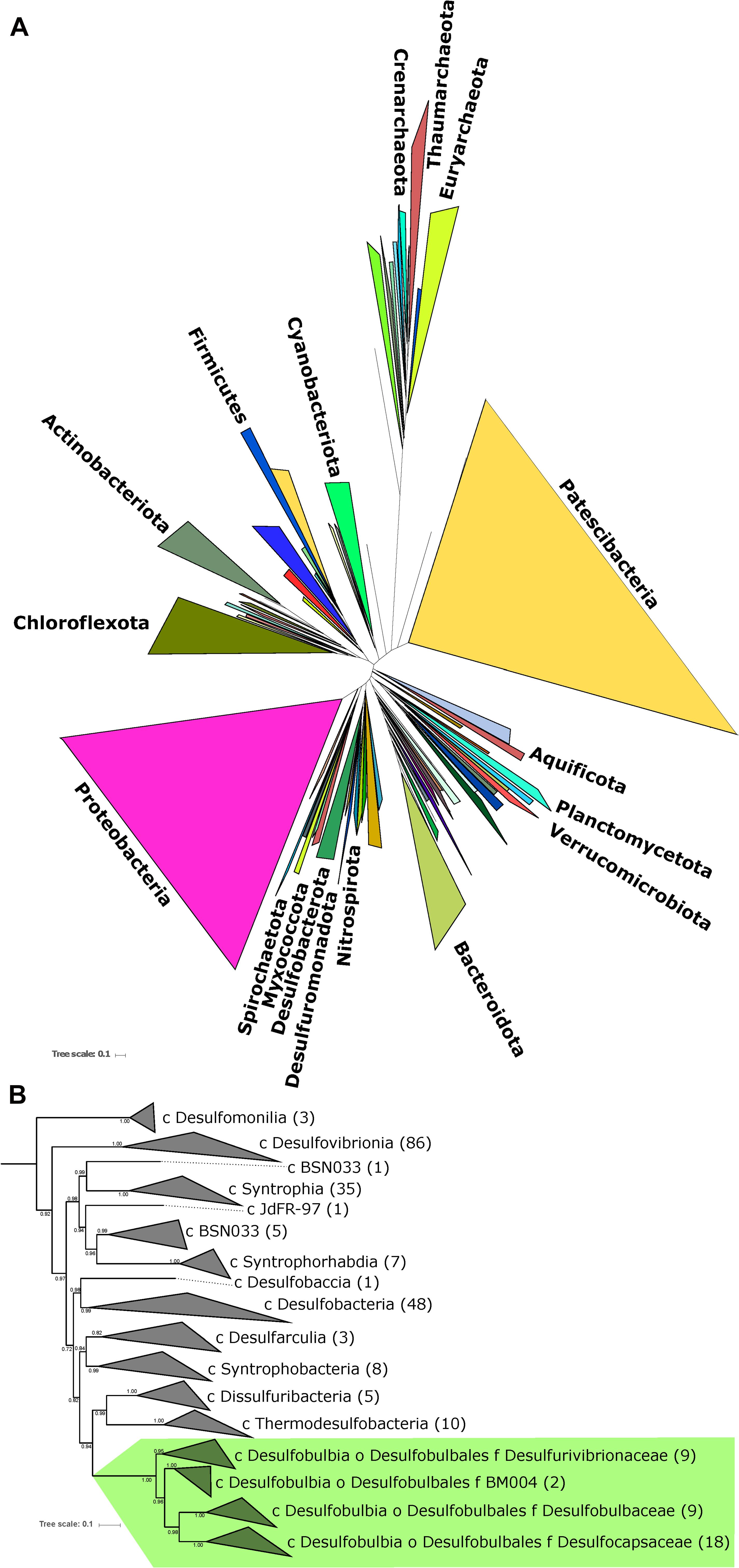
Figure 2. (A) Tree of Life built with concatenated ribosomal proteins following Hug et al. (2016) collapsed at the phylum level as classified by GTDB-Tk showing the relationship of Desulfobacterota relative to Proteobacteria and other major bacterial groups. (B) Concatenated ribosomal protein phylogeny of the Desulfobacterota binned at the family (Desulfobulbales) or class (all other lineages) levels, labeled with taxonomic assignments from GTDB-Tk, showing the placement of and relationships within the Desulfobulbales. The number of genomes from each clade used in the construction of the tree noted in parentheses after taxonomy label. Nodes are labeled with TBE support values.
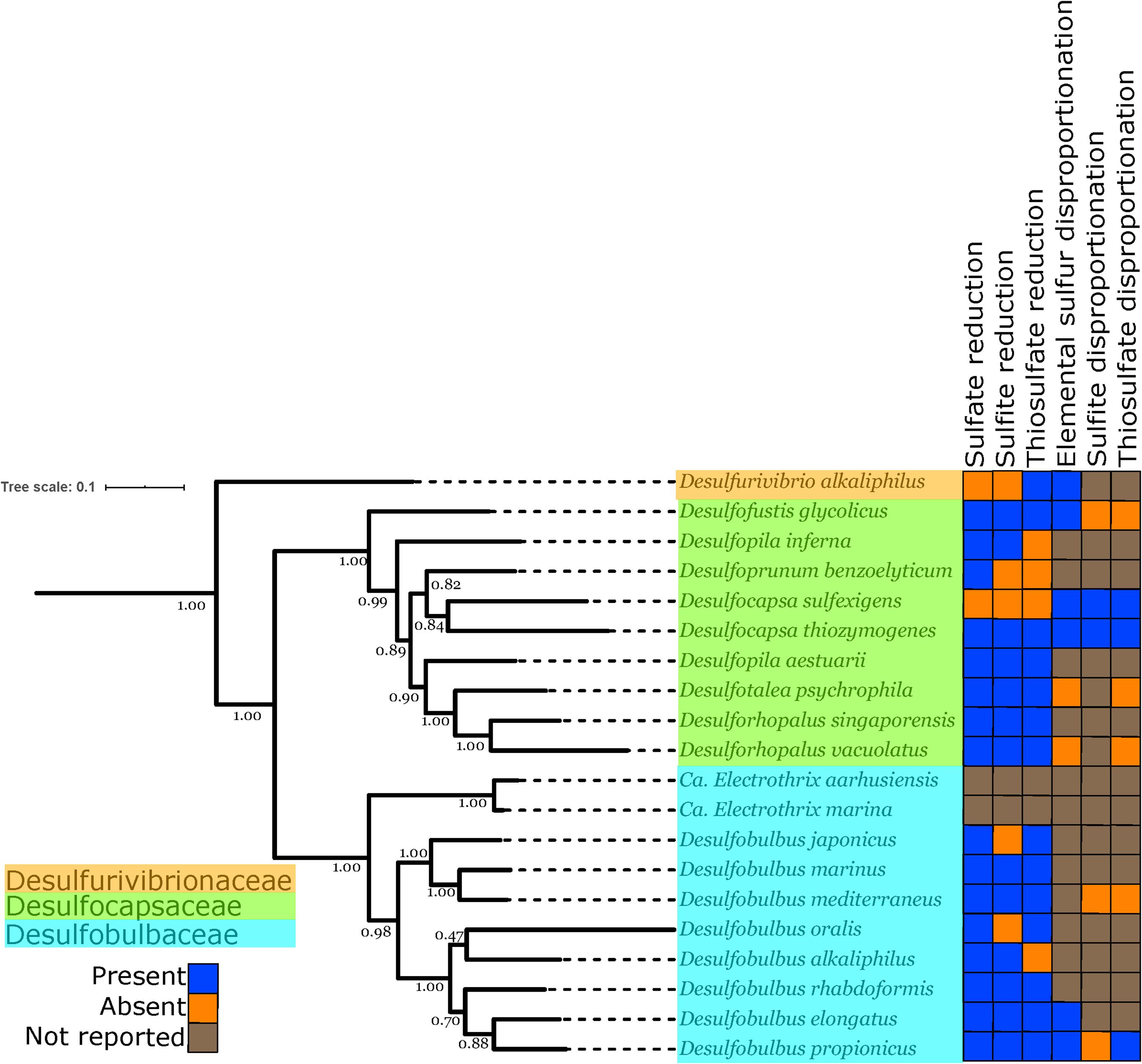
Figure 3. Phylogenetic tree showing the genomes of isolated and well-characterized members of the Desulfobulbales, including the families Desulfobulbaceae, Desulfocapsaceae, and Desulfurivibrionaceae. Nodes are labeled with TBE support values. Species names are highlighted with colors corresponding to the taxonomic family to which they are assigned. On the right, the characterized capacity for performing sulfur metabolisms is indicated.
Here, we first provide a revised genome-based taxonomy of the Desulfobulbales. We do this by presenting draft genomes of previously unsequenced isolates belonging to the Desulfobulbales, and couple this newly expanded genomic sampling with comparative genomic and phylogenetic analyses. We then plot metabolic information on the resulting phylogenetic trees to provide insight into the relationships between strains previously characterized as sulfate reducers or sulfur disproportionators. Ultimately, these will provide a refined assessment of the major evolutionary transitions between the lineages of sulfate reduction and sulfur disproportionation.
Materials and Methods
Genome Sequencing and Analysis
Our analyses focused on Desulfobulbales strains that have both been well characterized in pure culture and for which high-quality genome sequences are available. We therefore omitted organisms known only from metagenome-, environmental-, or enrichment-based analyses, including the cable bacteria. Preexisting genome sequences of Desulfobulbales were downloaded from the NCBI WGS and Genbank databases. In order to thoroughly sample the genomic diversity of well-characterized Desulfobulbales isolates, we also performed genome sequencing on six species of Desulfobulbales that are available in pure culture but for which genome sequencing had not previously been performed. These included: Desulforhopalus vacuolatus (Isaksen and Teske, 1996), Desulfobulbus marinus (Widdel and Pfennig, 1982; Kuever et al., 2015), Desulfoprunum benzoelyticum (Junghare and Schink, 2015), Desulfopila inferna (Gittel et al., 2010), Desulfobulbus rhabdoformis (Lien et al., 1998), and Desulfobulbus alkaliphilus (Sorokin et al., 2012).
Purified genomic DNA was acquired for each strain from the DSMZ and submitted to MicrobesNG for sequencing. DNA extraction was performed with a JetFlex genomic DNA purification kit from Genomed. DNA libraries were prepared using Nextera XT library prep kits using a Hamilton Microlab Star automated liquid handling system. Sequencing was performed with an Illumina HiSeq using a 250 base pair paired-end protocol. Reads were adapter trimmed with Trimmomatic 0.30 (Bolger et al., 2014). De novo assembly was performed with SPAdes version 3.7 (Bankevich et al., 2012). Genomes were annotated and analyzed using RAST v2.0 (Aziz et al., 2008). Completeness and contamination/redundancy of genomes was estimated with CheckM v1.0.12 (Parks et al., 2015). The likelihood for presence or absence of metabolic pathways was determined using MetaPOAP v1.0 (Ward et al., 2018). Taxonomic assignments were verified with GTDB-Tk v0.3.2 (Parks et al., 2018). Hydrogenase proteins were classified with HydDB (Søndergaard et al., 2016).
Phylogenetic Analyses
Genomes were downloaded from the NCBI Genbank and WGS databases. The dataset used for comparative genomics analyses consisted of all complete or high quality (following current standards, Bowers et al., 2017) genomes of isolated members of the Desulfobulbales. Phylogenetic analyses incorporated all genomes of isolates as well as metagenome-assembled genomes of members of the Desulfobacterota (Deltaproteobacteria) available on the NCBI Genbank and WGS databases as of August 2019 together with genomes of sulfur disproportionators and closely related outgroups. Protein sequences used in analyses (see below) were identified locally with the tblastn function of BLAST+ v2.6.0 (Camacho et al., 2009), aligned with MUSCLE v3.8.31 (Edgar, 2004), and manually curated in Jalview v2.10.5 (Waterhouse et al., 2009). Positive BLAST hits were considered to be full length (e.g., > 90% the shortest reference sequence from an isolate genome) with e-values better than 1e-20. Phylogenetic trees were calculated using RAxML v8.2.12 (Stamatakis, 2014) on the Cipres science gateway (Miller et al., 2010). Transfer bootstrap support values were calculated by BOOSTER (Lemoine et al., 2018). Trees were visualized with the Interactive Tree of Life viewer (Letunic and Bork, 2016). Taxonomic assignment was confirmed with GTDB-Tk v0.3.2 (Parks et al., 2018; Chaumeil et al., 2019). Amino Acid Identity of genomes was determined following methods from Rodriguez-R and Konstantinidis (2014). The shared evolutionary histories of the sulfate reduction and sulfur disproportionation lineages was inferred by comparison of topology between organismal and metabolic protein phylogenies, with congruent topologies reflecting shared vertical inheritance and incongruent topologies suggesting independent evolutionary history of metabolic genes (i.e., horizontal gene transfer) (Doolittle, 1986). Protein sequence annotation was done by GhostKOALA using default settings (Kanehisa et al., 2016) and amino acid sequences translated by Prodigal (Hyatt et al., 2010). Visualization of the presence or absence of complete or partial metabolic pathways was done using KEGG-decoder (Graham et al., 2018) after manual formatting of GhostKOALA output (Figure 4).
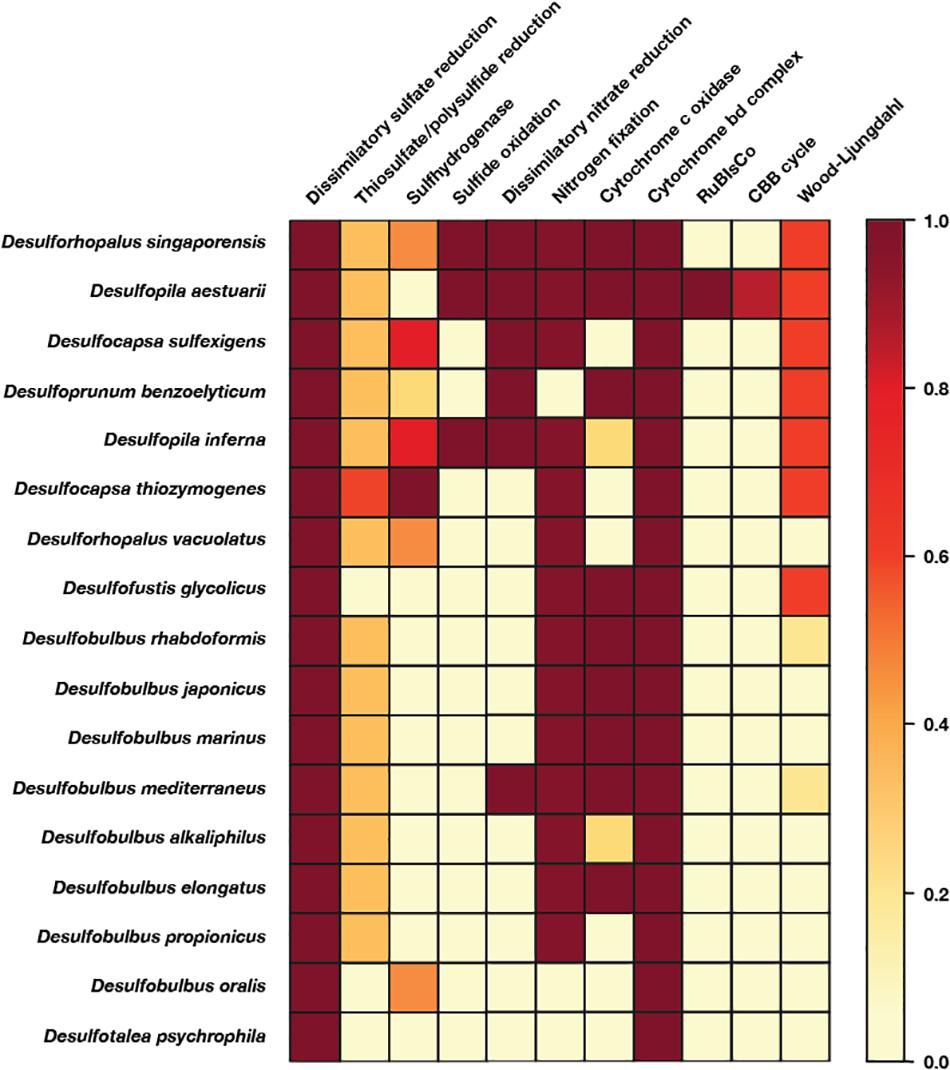
Figure 4. Heatmap of metabolic functions produced by the KEGG-decoder of the members of the Desulfobulbales sequenced here. The color gradient reflects the fractional abundance of genes associated with a pathway encoded by a particular genome. In other words, white implies no genes associated with a pathway of interest are found in the genome and thus that said pathway is not constituted. Conversely, dark red indicates all genes required to perform the pathway of interest are found and that said metabolism is fully constituted in the genome. Implications for the presence or absence of metabolic pathways of interest in each genome are discussed in the text.
Results and Discussion
Draft Genomes of Desulfobulbales Isolates
In order to improve coverage of genomic diversity of Desulfobulbales, we sequenced draft genomes from isolates of six species from the Desulfobulbaceae and Desulfocapsaceae families: Desulforhopalus vacuolatus (Isaksen and Teske, 1996), Desulfobulbus marinus (Widdel and Pfennig, 1982; Kuever et al., 2015), Desulfoprunum benzoelyticum (Junghare and Schink, 2015), Desulfopila inferna (Gittel et al., 2010), Desulfobulbus rhabdoformis (Lien et al., 1998), and Desulfobulbus alkaliphilus (Sorokin et al., 2012). All genomes qualify as high quality under current standards (Bowers et al., 2017). Genome statistics are summarized in Table 1 and presence of relevant functional genes is described below. Desulforhopalus vacuolatus, Desulfobulbus marinus, Desulfoprunum benzoelyticum, Desulfopila inferna, Desulfobulbus rhabdoformis, and Desulfobulbus alkaliphilus all encode the full enzymatic machinery shared by dissimilatory sulfate reduction and sulfur disproportionation, that is, DsrAB, DsrMKJOP, and AprAB (Keller and Wall, 2011; Pereira et al., 2011; Parey et al., 2013). While all these strains have been reported as sulfate reducing bacteria based on pure culture experiments geared to test their metabolic capacities (Widdel and Pfennig, 1982; Isaksen and Teske, 1996; Lien et al., 1998; Gittel et al., 2010; Sorokin et al., 2012; Junghare and Schink, 2015; Kuever et al., 2015) only, Desulforhopalus vacuolatus has been reported incapable of sulfur disproportionation (Isaksen and Teske, 1996). The other strains have yet to be tested for the capacity to disproportionate intermediate valence sulfur species. Further, a correlation between the length of the AprB C-terminus and the capacity to perform sulfate reduction or disproportionation has recently been suggested, where a truncated C-terminus would be indicative of sulfur disproportionation (Bertran, 2019). Desulfopila inferna encodes a full length AprB protein, whereas Desulforhopalus vacuolatus, Desulfobulbus marinus, Desulfoprunum benzoelyticum, Desulfobulbus rhabdoformis, and Desulfobulbus alkaliphilus encode a truncated C-terminal AprB domain like other sulfur disproportionators in the Desulfobulbaceae (Bertran, 2019). However, further work is needed to confirm the validity of this truncation as a distinct genetic marker for sulfur disproportionation and there is, to date, no definite feature that distinguishes sulfate reducers from sulfur disproportionators.
Despite their characterization as obligate anaerobes, Desulforhopalus vacuolatus and Desulfobulbus rhabdoformis encode the capacity for O2 reduction via a bd O2 reductase. While bd O2 reductase enzymes are sometimes coupled to aerobic respiration (e.g., in Nitrospira, Palomo et al., 2018), they can be found in obligate anaerobes (e.g., Ward et al., 2015) where they are likely associated with O2 detoxification and oxidative stress tolerance (Forte et al., 2017). Additionally, Desulfoprunum benzoelyticum, Desulfobulbus rhabdoformis and Desulfobulbus marinus also encode A-family heme copper oxidoreductases (HCOs) for O2 reduction; while these enzymes are typically coupled to aerobic respiration they can also be found in obligate anaerobes (e.g., Hemp et al., 2015; Pace et al., 2015). In anaerobic organisms, such as members of the Desulfobulbales, these proteins are likely also associated with O2 detoxification and oxidative stress tolerance (e.g., Forte et al., 2017). Closely related A-family HCO proteins are also encoded by other Desulfobulbales such as Desulfopila aestuarii, Desulfofustis glycolicus, Desulfobulbus japonicus, Desulfobulbus mediterraneus, and Desulfobulbus elongatus. These Desulfobulbales HCOs form a closely related clade in broad HCO phylogenies sampling across diverse bacteria and archaea (Supplementary Figure 1). This suggests broad vertical inheritance of HCOs from a common ancestor of the Desulfobulbales, with perhaps a small amount of intra-order horizontal gene transfer (HGT), potentially suggesting a long history of aerotolerance in the Desulfobulbales that stands in contrast to the O2 sensitivity of other orders of Desulfobacterota (e.g., Rosenberg et al., 2014). This may have served as a preadaptation to marginal redox environments in which the transition from MSR to MSD may have been favored, leading to the relatively high density of novel transitions to sulfur disproportionation in the Desulfobulbales.
Several of the Desulfobulbales genomes reported here also encode proteins involved in nitrogen redox reactions. Nitrogen fixation via a molybdenum nitrogenase is encoded by Desulforhopalus vacuolatus, Desulfopila inferna, and Desulfobulbus marinus. Desulfobulbus rhabdoformis encodes both a molybdenum nitrogenase as well as a vanadium alternative nitrogenase. Additionally, Desulfobulbus marinus encodes nitrite reduction to ammonium via NrfH. Despite being characterized as incapable of nitrate respiration (Junghare and Schink, 2015), Desulfoprunum benzoelyticum encodes a pathway for nitrate reduction to ammonia including nitrate reductase and cytochrome c552 nitrite reductase.
Members of the Desulfobulbales utilize various electron donors for growth, typically including simple alcohols, organic acids, and other small organic compounds which are typically incompletely oxidized (producing CO2 and acetate) (Kuever, 2014). Among notable exceptions to this trend in the Desulfobulbales strains discussed here, Desulfoprunum benzoelyticum is known to completely degrade benzoate to CO2 (Junghare and Schink, 2015). Benzoate degradation is known to be performed by a pathway consisting first of benzoate-CoA ligase and downstream enzymes including benzoyl-CoA reductase and benzoyl-CoA 2,3-epoxidase. The Desulfoprunum benzoelytcium genome recovered genes encoding for benzoate-CoA ligase but not known genes for downstream steps. Given the high completeness of the Desulfoprunum benzoelyticum genome (∼99.85%), the probability that the complete genome encodes additional benzoate degradation genes is incredibly low (<10–7) as determined by MetaPOAP (Ward et al., 2018). This suggests that Desulfoprunum benzoelyticum may utilize a novel pathway for benzoate metabolism using previously uncharacterized genes, though additional genetic and biochemical study will be necessary to validate this hypothesis.
Diversity and Taxonomy of Desulfobulbales
Classically, the family Desulfobulbaceae within the Desulfobacterales order of the Deltaproteobacteria phylum has included the genera Desulfobulbus, Desulfocapsa, Desulfofustis, Desulfopila, Desulforhopalus, Desulfotalea, and Desulfurivibrio (Kuever, 2014). However, recent attempts at more systematic and normalized taxonomies based on full genome comparisons (e.g., Rinke et al., 2020; Waite et al., 2020) provide an opportunity to reassess this classification. The Deltaproteobacteria in particular have proven excellent cases for the necessity of taxonomic reappraisal as lineages assigned to this phylum have been shown to be polyphyletic, not closely related to other groups defined as Proteobacteria, and likely to represent several phylum-level groups (e.g., Hug et al., 2016, Waite et al., 2020). In recognition of these facts, the Genome Taxonomy Database (GTDB) has divided the Deltaproteobacteria into several monophyletic phyla including Desulfobacterota, which contains the bulk of classical Deltaproteobacteria such as Desulfovibrio, Desulfobacter, and Desulfobulbus (Parks et al., 2018; Waite et al., 2020). Additionally, the GTDB proposes further subdivision of lower taxonomic levels in order to remove poly- or para-phyletic groupings and normalize taxonomic ranks (Parks et al., 2018). In the case of the Desulfobulbaceae, the GTDB has reassigned these organisms to four families (Desulfobulbaceae, Desulfocapsaceae, Desulfurivibrionaceae, and BM004) within the new order Desulfobulbales of the Desulfobacterota phylum.
Our expanded phylogeny of the Desulfobulbales is broadly consistent with the revised GTDB taxonomy (Figures 1, 3), recapitulating a monophyletic Desulfobulbales order within the Desulfobacterota as well as producing consistent family-level groupings within this order. The GTDB further suggests subdivision of the Desulfobulbus genus into at least two genera within the Desulfobulbaceae family. AAI analyses (Supplementary Table 2) shows no higher than 75% similarity in any pairwise comparison of characterized Desulfobulbales strains, consistent with each strain representing at least a unique species. Genus-level cutoffs of 55–60% largely follow taxonomic boundaries assigned based on physiology and other classical metrics. While the GTDB suggests the subdivision of Desulfobulbus into at least two genera – that is, Desulfobulbus sensu stricto which includes Desulfobulbus marinus, Desulfobulbus oralis, Desulfobulbus propionicus, and Desulfobulbus rhabdoformis, and a genus Desulfobulbus A containing Desulfobulbus japonicus and Desulfobulbus mediterraneus – this subdivision is only somewhat supported by AAI analyses. Pairwise AAI similarity between Desulfobulbus strains is only < 0.55 for Desulfobulbus oralis when compared against Desulfobulbus japonicus, Desulfobulbus mediterraneus, or Desulfobulbus marinus. Pairwise comparisons between other members of Desulfobulbus sensu stricto and Desulfobulbus A largely show AAI values in the range of 0.6–0.7, consistent with a single Desulfobulbus genus. This, together with generally poor support for the phylogenetic placement of Desulfobulbus oralis, suggests that the relatively high divergence of Desulfobulbus oralis from other members of Desulfobulbus may artificially inflate the apparent taxonomic breadth of strains classified as Desulfobulbus. It is currently unclear whether Desulfobulbus oralis shows particularly high divergence given factors relating to adaptation to it unique niche (for Desulfobulbales strains) in the human mouth, because of elevated rates of mutation or HGT, or for other reasons. In summary, our results support the reassignment of the Desulfobulbales to the new taxonomic classification proposed by the GTDB, particularly at the family level and above. We therefore use GTDB-based clade names (e.g., Desulfobacterota, Desulfobulbales) throughout.
Congruence of Organismal and Sulfur Metabolic Protein Phylogenies in the Desulfobulbales
The distribution of sulfur metabolisms in the Desulfobulbales is scattered, with the capacity for reduction and disproportionation reactions interspersed in different groups (Figure 3). The capacity for sulfur disproportionation in particular appears to be polyphyletic. As a result, it is impossible to confidently assert a simple evolutionary history for sulfur metabolisms in the Desulfobulbales. Viable scenarios for the history of sulfur metabolisms in this clade could include, for instance, (1) an ancestor capable of both sulfate reduction and sulfur disproportionation followed by loss of either metabolism in many lineages, (2) an ancestor capable of sulfate reduction but not sulfur disproportionation, followed by convergent evolution of sulfur disproportionation, with or without loss of sulfate reduction, independently and in many lineages, or (3) the presence of sulfate reduction but not sulfur disproportionation, followed by a single evolutionary origin of sulfur disproportionation and ensuing HGT to distribute this metabolism into multiple lineages. More complicated scenarios involving multiple origins, losses, and horizontal transfers of pathways are also conceivable. Distinguishing between these scenarios is challenging, particularly given the inability to distinguish between the capacity for sulfate reduction and sulfur disproportionation via genome content alone (Anantharaman et al., 2018). The capacity for sulfate reduction and sulfur disproportionation is currently determined only through culture-based characterization; however, the capacity for disproportionation metabolisms is frequently not determined or reported (Figure 3). As a result, our ability to interpret the evolutionary history of sulfur metabolisms in the Desulfobulbales is limited. However, sufficient data is available to draw some conclusions about overall trends.
It is well established that sulfur disproportionation utilizes the same basic biochemical pathways as sulfate reduction, albeit with modifications to enzymes or regulation that allows some steps to run in reverse (Finster et al., 1998, 2013; Frederiksen and Finster, 2003; Finster, 2008; Thorup et al., 2017; Pellerin et al., 2019). Patterns of vertical versus horizontal transfer of components in this pathway should reflect vertical versus horizontal inheritance of the metabolisms themselves. We therefore applied methods comparing organismal to functional protein phylogenies to investigate whether HGT of sulfur metabolizing proteins was responsible for the scattered distribution of sulfur disproportionation in the Desulfobulbales. If sulfur metabolism proteins (e.g., AprA, DsrA) phylogenies differ from organismal phylogenies (as determined by concatenated ribosomal proteins or other markers), this would suggest a history of horizontal gene transfer. Instead, it appears that sulfur metabolizing proteins have been vertically inherited within the Desulfobulbales, with few, if any, instances of HGT (Supplementary Figures 2, 4, 5). Rather, this supports scenarios of multiple instances of convergent evolution of sulfur disproportionation or, alternatively, the capacity for both sulfur disproportionation and sulfate reduction in the last common ancestor of the Desulfobulbales followed by many instances of loss of one pathway. The absence of intra-order HGT of sulfur metabolism pathways is further supported by the scattered but consistent localization of sulfur metabolisms genes across Desulfobulbales genomes, preventing straightforward HGT of a single operon or cluster of genes, but broadly retaining position of particular genes in the genome between members of the Desulfobulbales (e.g., colocalization of aprAB with the anaerobic respiratory complex qmoABC).
While the antiquity of sulfur disproportionation is not entirely clear, the simplest explanation for the distribution of sulfate reduction is that this metabolism was present in the last common ancestor of the Desulfobulbales and was secondarily lost in a few lineages (e.g., Desulfocapsa sulfexigens). This scenario is particularly compelling given the broad distribution of sulfate reduction and the relatively sparse distribution of sulfur disproportionation in the Desulfobacterota (e.g., Anantharaman et al., 2018). Whether sulfur disproportionation arose multiple times in different Desulfobulbales lineages or originated once in the stem group of this clade, it appears to represent convergent evolution with disproportionators in other lineages of Desulfobacterota and other phyla.
Conclusion
The distribution and evolutionary history of MSR and MSD in the Desulfobacterota, and in microbes in general, appears to be a complex palimpsest of vertical inheritance, occasional HGT, and extensive convergent evolution. The expanded genomic diversity of the Desulfobulbales order presented here provides additional context for investigating transitions between MSR and MSD but is unable to resolve a simple evolutionary history for this process. While it has long been apparent that sulfur disproportionation is derived from sulfate reduction, there still exists no unambiguous molecular markers to distinguish the capacity for these metabolisms from genomic data alone, nor is it clear what ecological or evolutionary processes underlie the innovation of sulfur disproportionation with or without the concurrent loss of sulfate reduction. However, the expanded genomic diversity presented here for well-characterized isolates, coupled with comparative phylogenetic approaches, can provide significant insight into the history of the Desulfobulbales. In this group, it is clear that the ancestral phenotype is of sulfate reduction, with multiple, convergent transitions to sulfur disproportionation either with or without the concurrent loss of sulfate reduction. This is in line with earlier work that supported the derivation of MSD from MSR (Canfield and Teske, 1996; Habicht and Canfield, 1998; Shen et al., 2001; Johnston et al., 2005; Philippot et al., 2007; Fike et al., 2015). By demonstrating the vertical inheritance of sulfur metabolic genes in the Desulfobulbales, we can rule out a major role for HGT in the distribution of MSD across the diversity of this clade. While the precise biochemical mechanisms and ecological triggers for the transition from MSR to MSD in this clade are still unknown, the propensity for the Desulfobulbales to invent and reinvent MSD may be related to a genomic background that includes pre-adaptations to marginal redox environments (e.g., presence of pathways for O2 detoxification) as well as alleles that allow more ready reversibility of key enzymes (e.g., the truncated AprB tail; Bertran, 2019). Further determination of markers for MSD in the Desulfobulbales and other organisms will require more thorough characterization and reporting of the capacity for disproportionation in sulfate reducing strains to reduce the burden of missing data (e.g., Figure 3) and to better allow thorough comparative genomics to identify genetic differences between disproportionator and non disproportionator lineages.
The apparent phenotypic plasticity between MSR and MSD over relatively short evolutionary timescales (i.e., species- or genus-level variability, versus evolution over family or higher longer timescales as is typically seen in other metabolic traits like phototrophy and carbon fixation, e.g., Shih et al., 2017; Ward et al., 2021) has significant implications for our understanding of the roles of these metabolisms in Earth history. If sulfate reducing microbes can readily and independently evolve the capacity for disproportionation, this suggests that this process may occur frequently in diverse lineages over geologic time. As a result, it is likely that sulfur disproportionating microbes have been present for as much of Earth history as there have been viable environmental niches for this metabolism to operate within — but, importantly, these likely have consisted of different, unrelated lineages at different times in Earth history. It is therefore reasonable to assume the activity of MSD in shaping sulfur isotopes and other sedimentary records from periods of Earth’s past, but it may not be possible to assume taxonomic affinity or other traits of the organisms responsible.
While expanded genomic sampling of the Desulfobulbales can improve our current understanding of the taxonomic and phylogenetic relationships in this clade, it is insufficient to fully untangle trends and processes in the evolutionary relationships between MSR and MSD. A major barrier to our understanding of these processes is our inability to distinguish the capacity for these metabolisms from genome content alone. Isolation and extensive physiological characterization of the sulfur metabolism capacity for Desulfobulbales strains continues to be essential; this includes the successful isolation of novel organisms in this clade (e.g., the enigmatic cable bacteria) but also the thorough testing and reporting of the sulfur disproportionation capacity for existing isolates (i.e., filling in the extensive “Not Reported” entries in Table 1 and Figure 3). Alternatively, identifying robust and consistent genomic markers to distinguish MSR from MSD may allow more accurate screening of the metabolic capacity of microorganisms from genome content alone in the absence of characterized isolates. Such markers have not yet been identified but are a target of active investigation (e.g., Umezawa et al., 2020). Finally, purely phylogenetic approaches to understanding the evolution of sulfur cycling in the Desulfobulbales provide an understanding of the timing of these processes only in relative evolutionary time. Tying this understanding to absolute, geologic time will require the application of additional approaches such as molecular clock analyses or calibrations using sediment geochemical and stable isotope records.
Data Availability Statement
The datasets presented in this study can be found in online repositories. The names of the repository/repositories and accession number(s) can be found below: https://www.ncbi.nlm.nih.gov/genbank/, PRJNA579145.
Author Contributions
LW and EB collected data, performed analyses, wrote the manuscript, and created figures. All authors conceived the study and edited the manuscript.
Funding
EB and DJ acknowledge support from NASA Exobiology (NNX15AP58G). This work was supported by a grant from the Simons Foundation (Grant number 653687 to LW). Genomic DNA was acquired from the Deutsche Sammlung vonMikroorganismen und Zellkulturen (DSMZ). Genome sequencing was provided by MicrobesNG (http://www.microbesng.uk) which is supported by the BBSRC (grant number BB/L024209/1).
Conflict of Interest
The authors declare that the research was conducted in the absence of any commercial or financial relationships that could be construed as a potential conflict of interest.
Supplementary Material
The Supplementary Material for this article can be found online at: https://www.frontiersin.org/articles/10.3389/fmicb.2021.666052/full#supplementary-material
Supplementary Figure 1 | Phylogeny of Heme-Copper Oxidoreductase (HCO) proteins from members of the Desulfobacterota. Leaves are labeled with the family of HCO ( A-, B-, or C-family) GTDB taxonomic assignments and WGS or Genbank IDs, nodes are labeled with TBE support value.
Supplementary Figure 2 | Phylogeny of concatenated DsrA, DsrB, DsrC, AprA, and AprB proteins from members of the Desulfobacterota. Leaves are labeled with GTDB taxonomic assignments and WGS or Genbank IDs, nodes are labeled with TBE support value.
Supplementary Figure 3 | Phylogeny of bd oxidase proteins from members of the Desulfobacterota. Leaves are labeled with GTDB taxonomic assignments and WGS or Genbank IDs, nodes are labeled with TBE support value.
Supplementary Figure 4 | Phylogeny of DsrA proteins from members of the Desulfobacterota. Leaves are labeled with GTDB taxonomic assignments and WGS or Genbank IDs, nodes are labeled with TBE support value.
Supplementary Figure 5 | Phylogeny of AprA proteins from members of the Desulfobacterota. Leaves are labeled with GTDB taxonomic assignments and WGS or Genbank IDs, nodes are labeled with TBE support value.
Supplementary Table 1 | Summary of capacity to perform microbial sulfate reduction (type of electron acceptor used indicated) and/or microbial sulfur disproportionation (type of disproportionation performed indicated) as described in peer-reviewed reports. NR, not reported; NA, not applicable; (1) Only “personal communication” found then, by default, indicated as Not Reported; (2) Reported in the reference given but no supporting study found.
Supplementary Table 2 | AAI matrix of Desulfobulbales.
References
Anantharaman, K., Hausmann, B., Jungbluth, S. P., Kantor, R. S., Lavy, A., Warren, L. A., et al. (2018). Expanded diversity of microbial groups that shape the dissimilatory sulfur cycle. The ISME Journal 12, 1715–1728. doi: 10.1038/s41396-018-0078-0
Aziz, R. K., Bartels, D., Best, A. A., DeJongh, M., Disz, T., Edwards, R. A., et al. (2008). The RAST Server: rapid annotations using subsystems technology. BMC genomics 9:75. doi: 10.1186/1471-2164-9-75
Bankevich, A., Nurk, S., Antipov, D., Gurevich, A. A., Dvorkin, M., Kulikov, A. S., et al. (2012). SPAdes: A New Genome Assembly Algorithm and Its Applications to Single-Cell Sequencing. Journal of Computational Biology 19, 455–477. doi: 10.1089/cmb.2012.0021
Berner, R., and Canfield, D. (1989). A new model for atmospheric oxygen over Phanerozoic time. American Journal Of Science 289, 333–361.
Berner, R. A., and Raiswell, R. (1984). C/S method for distinguishing freshwater from marine sedimentary rocks. Geology 12, 365–368. doi: 10.1130/0091-7613198412<365:CMFDFF<2.0.CO;2
Bertran, E. (2019). Cellular and intracellular insights into microbial sulfate reduction and sulfur disproportionation. Ph.D. dissertation. Cambridge, MA: Harvard University.
Bertran, E., Leavitt, W. D., Pellerin, A., Zane, G. M., Wall, J. D., Halevy, I., et al. (2018). Deconstructing the dissimilatory sulfate reduction pathway: Isotope fractionation of a mutant unable of growth on sulfate. Frontiers in Microbiology 9:3110. doi: 10.3389/fmicb.2018.03110
Bolger, A. M., Lohse, M., and Usadel, B. (2014). Trimmomatic: a flexible trimmer for Illumina sequence data. Bioinformatics 30, 2114–2120. doi: 10.1093/bioinformatics/btu170
Bontognali, T. R., Sessions, A. L., Allwood, A. C., Fischer, W. W., Grotzinger, J. P., Summons, R. E., et al. (2012). Sulfur isotopes of organic matter preserved in 3.45-billion-year-old stromatolites reveal microbial metabolism. Proceedings of the National Academy of Sciences 109, 15146–15151. doi: 10.1073/pnas.1207491109
Böttcher, M. E., Thamdrup, B., Gehre, M., and Theune, A. (2005). 34S/32S and 18O/16O Fractionation During Sulfur Disproportionation by Desulfobulbus propionicus. Geomicrobiology Journal 22, 219–226. doi: 10.1080/01490450590947751
Bowers, R. M., Kyrpides, N. C., Stepanauskas, R., Harmon-Smith, M., Doud, D., et al. (2017). Minimum information about a single amplified genome (MISAG) and a metagenome-assembled genome (MIMAG) of bacteria and archaea. Nature biotechnology 35, 725–731. doi: 10.1038/nbt.3893
Bradley, A. S., Leavitt, W. D., Schmidt, M., Knoll, A. H., Girguis, P. R., and Johnston, D. T. (2016). Patterns of sulfur isotope fractionation during microbial sulfate reduction. Geobiology 14, 91–101. doi: 10.1111/gbi.12149
Camacho, C., Coulouris, G., Avagyan, V., Ma, N., Papadopoulos, J., Bealer, K., et al. (2009). BLAST+: architecture and applications. Bmc Bioinformatics 10:421. doi: 10.1186/1471-2105-10-421
Canfield, D. (2001). Isotope fractionation by natural populations of sulfate-reducing bacteria. Geochimica et Cosmochimica Acta 65, 1117–1124. doi: 10.1016/S0016-7037(00)00584-6
Canfield, D. E., and Teske, A. (1996). Late Proterozoic rise in atmospheric oxygen concentration inferred from phylogenetic and sulphur-isotope studies. Nature 382, 6587. doi: 10.1038/382127a0
Canfield, D. E., and Thamdrup, B. (1994). The production of 34S-depleted sulfide during bacterial disproportionation of elemental sulfur. Science 266, 1973–1975. doi: 10.1126/science.11540246
Canfield, D. E., Thamdrup, B., and Fleischer, S. (1998). Isotope fractionation and sulfur metabolism by pure and enrichment cultures of elemental sulfur disproportionating bacteria. Limnology and Oceanography 43, 253–264. doi: 10.4319/lo.1998.43.2.0253
Chaumeil, P. A., Mussig, A. J., Hugenholtz, P., and Parks, D. H. (2019). GTDB-Tk: a toolkit to classify genomes with the Genome Taxonomy Database. Bioinformatics 36, 1925–1927. doi: 10.1093/bioinformatics/btz848
Cross, K. L., Chirania, P., Xiong, W., Beall, C. J., Elkins, J. G., Giannone, R. J., et al. (2018). Insights into the Evolution of Host Association through the Isolation and Characterization of a Novel Human Periodontal Pathobiont, Desulfobulbus oralis. mBio 9, e2061–e2017. doi: 10.1128/mBio.02061-17 ∗∗e02061-17,
Doolittle, R. F. (1986). Of Urfs And Orfs: Primer On How To Analyze Derived Amino Acid Sequences. Mill Valley, CA: University Science Books.
Edgar, R. C. (2004). MUSCLE: multiple sequence alignment with high accuracy and high throughput. Nucleic Acids Research 32, 1792–1797. doi: 10.1093/nar/gkh340
El Houari, A., Ranchou-Peyruse, M., Ranchou-Peyruse, A., Dakdaki, A., Guignard, M., Idouhammou, L., et al. (2017). Desulfobulbus oligotrophicus sp. nov., a sulfate-reducing and propionate-oxidizing bacterium isolated from a municipal anaerobic sewage sludge digester. International journal of systematic and evolutionary microbiology 67, 275–281. doi: 10.1099/ijsem.0.001615
Fike, D., Bradley, A., and Rose, C. (2015). Rethinking the ancient sulfur cycle. Annual Review of Earth and Planetary Sciences 43, 593–622. doi: 10.1146/annurev-earth-060313-054802
Finster, K. (2008). Microbiological disproportionation of inorganic sulfur compounds. Journal of Sulfur Chemistry 29, 281–292. doi: 10.1080/17415990802105770
Finster, K., Liesack, W., and Thamdrup, B. (1998). Elemental Sulfur and Thiosulfate Disproportionation by Desulfocapsa sulfoexigens sp. nov., a New Anaerobic Bacterium Isolated from Marine Surface Sediment. Applied and Environmental Microbiology 64, 119. doi: 10.1128/AEM.64.1.119-125.1998
Finster, K. W., Kjeldsen, K. U., Kube, M., Reinhardt, R., Mussmann, M., Amann, R., et al. (2013). Complete genome sequence of Desulfocapsa sulfexigens, a marine deltaproteobacterium specialized in disproportionating inorganic sulfur compounds. Standards in genomic sciences 8, 58–68. doi: 10.4056/sigs.3777412
Frederiksen, T.-M., and Finster, K. (2003). Sulfite-oxido-reductase is involved in the oxidation of sulfite in Desulfocapsa sulfoexigens during disproportionation of thiosulfate and elemental sulfur. Biodegradation 14, 189–198. doi: 10.1023/A:1024255830925
Fry, B., Cox, J., Gest, H., and Hayes, J. M. (1986). Discrimination between 34S and 32S during bacterial metabolism of inorganic sulfur compounds. J Bacteriol 165, 328–330. doi: 10.1128/jb.165.1.328-330.1986
Fuseler, K., and Cypionka, H. (1995). Elemental sulfur as an intermediate of sulfide oxidation with oxygen by Desulfobulbus propionicus. Archives of Microbiology 164, 104–109. doi: 10.1007/BF02525315
Gittel, A., Seidel, M., Kuever, J., Galushko, A. S., Cypionka, H., and Konneke, M. (2010). Desulfopila inferna sp. nov., a sulfate-reducing bacterium isolated from the subsurface of a tidal sand-flat. International Journal of Systematic and Evolutionary Microbiology 60, 1626–1630. doi: 10.1099/ijs.0.015644-0
Graham, E. D., Heidelberg, J. F., and Tully, B. J. (2018). Potential for primary productivity in a globally-distributed bacterial phototroph. ISME Journal 350, 1861–1866. doi: 10.1038/s41396-018-0091-3
Habicht, K. S., and Canfield, D. E. (1998). Sulfur isotope fractionation during bacterial reduction and disproportionation of thiosulfate and sulfite. Geochimica et Cosmochimica Acta 62, 2585–2595. doi: 10.1016/S0016-7037(98)00167-7
Habicht, K. S., Gade, M., Thamdrup, B., Berg, P., and Canfield, D. E. (2002). Calibration of sulfate levels in the Archean ocean. Science 298, 5602. doi: 10.1126/science.1078265
Hemp, J., Ward, L. M., Pace, L. A., and Fischer, W. W. (2015). Draft genome sequence of Levilinea saccharolytica KIBI-1, a member of the Chloroflexi class Anaerolineae. Genome announcements 3, e1357–e1315. doi: 10.1128/genomeA.01357-15 ∗∗e01357-15,
Hug, L. A., Baker, B. J., Anantharaman, K., Brown, C. T., Probst, A. J., Castelle, C. J., et al. (2016). A new view of the tree of life Nature. Microbiology 1:16048. doi: 10.1038/nmicrobiol.2016.48
Hyatt, D., Chen, G. L., LoCascio, P. F., Land, M. L., Larimer, F. W., and Hauser, L. J. (2010). Prodigal: prokaryotic gene recognition and translation initiation site identification. BMC bioinformatics 11:119. doi: 10.1186/1471-2105-11-119
Isaksen, M. F., and Teske, A. (1996). Desulforhopalus vacuolatus gen. nov., sp. nov., a new moderately psychrophilic sulfate-reducing bacterium with gas vacuoles isolated from a temperate estuary. Archives of Microbiology 166, 160–168. doi: 10.1007/s002030050371
Janssen, P. H., Schuhmann, A., Bak, F., and Liesack, W. (1996). Disproportionation of inorganic sulfur compounds by the sulfate-reducing bacterium Desulfocapsa thiozymogenes gen. nov., sp. nov. Archives of Microbiology 166, 184–192. doi: 10.1007/s002030050374
Johnston, D. T., Poulton, S. W., Fralick, P. W., Wing, B. A., Canfield, D. E., and Farquhar, J. (2005). Evolution of the oceanic sulfur cycle at the end of the Paleoproterozoic. Geochimica et Cosmochimica Acta 70, 5723–5739. doi: 10.1016/j.gca.2006.08.001
Jørgensen, B. (1982). Mineralization of organic matter in the seabed–the role of sulphate reduction. Nature 296, 643-645. doi: 10.1038/296643a0
Junghare, M., and Schink, B. (2015). Desulfoprunum benzoelyticum gen. nov., sp. nov., a Gram-stain-negative, benzoate-degrading, sulfate-reducing bacterium isolated from a wastewater treatment plant. International journal of systematic and evolutionary microbiology 65(Pt 1), 77–84. doi: 10.1099/ijs.0.066761-0
Kanehisa, M., Sato, Y., and Morishima, K. (2016). BlastKOALA and GhostKOALA: KEGG tools for functional characterization of genome and metagenome sequences. Journal of Molecular Biology 428, 726–731. doi: 10.1016/j.jmb.2015.11.006
Keller, K. L., and Wall, J. D. (2011). Genetics and molecular biology of the electron flow for sulfate respiration in Desulfovibrio. Frontiers in Microbiology 2:135. doi: 10.3389/fmicb.2011.00135
Kjeldsen, K. U., Schreiber, L., Thorup, C. A., Boesen, T., Bjerg, J. T., Yang, T., et al. (2019). On the evolution and physiology of cable bacteria. Proceedings of the National Academy of Sciences 116, 19116–19125. doi: 10.1073/pnas.1903514116
Knoblauch, C., Sahm, K., and Jorgensen, B. (1999). Psychrophilic sulfate-reducing bacteria isolated from permanently cold Arctic marine sediments: description of Desulfofrigus oceanense gen.nov., sp. nov., Desulfofrigus fragile sp. nov., Desulfofaba gelida gen. nov., sp. nov., Desulfotalea psychrophila gen. nov., sp. nov. and Desulfotalea arctica sp. Nov. International Journal of Systematic Bacteriology 49, 1631–1643. doi: 10.1099/00207713-49-4-1631
Kramer, M., and Cypionka, H. (1989). Sulfate formation via ATP sulfurylase in thiosulfate- and sulfite-disproportionating bacteria. Archives of Microbiology 151, 232–237. doi: 10.1007/BF00413135
Kuever, J. (2014). “The Family Desulfobulbaceae,” in The Prokaryotes, eds E. Rosenberg, E. F. DeLong, S. Lory, E. Stackebrandt, and F. Thompson (Berlin: Springer).
Kuever, J., Rainey, F. A., and Widdel, F. (2005). “Genus I Desulfobulbus,” in Bergey’s manual of systematic bacteriology (The Proteobacteria), part C (The Alpha-, Beta-, Delta-, and Epsilonproteobacteria), 2nd Edn, Vol. 2, eds D. J. Brenner, N. R. Krieg, J. T. Staley, and G. M. Garrity (New York, NY: Springer), 988–992.
Kuever, J., Rainey, F. A., and Widdel, F. (2015). Desulfobulbus. Berg. Manu. Systemat. Archaea Bact. 1–6.
Leavitt, W. D., Halevy, I., Bradley, A. S., and Johnston, D. T. (2013). Sulfur isotopes record Phanerozoic redox shift. Proceedings of the National Academy of Sciences 110, 11244–11249. doi: 10.1073/pnas.1218874110
LeGall, J., and Fauque, G. (1988). “Dissimilatory reduction of sulfur compounds,” in Biology of Anaerobic Microorganisms, ed. A. J. B. Zehnder (New York, NY: Wiley), 587–639.
Lemoine, F., Entfellner, J. B. D., Wilkinson, E., Correia, D., Felipe, M. D., Oliveira, T., et al. (2018). Renewing Felsenstein’s phylogenetic bootstrap in the era of big data. Nature 556, 452. doi: 10.1038/s41586-018-0043-0
Letunic, I., and Bork, P. (2016). Interactive tree of life (iTOL) v3: an online tool for the display and annotation of phylogenetic and other trees. Nucleic Acids Research 44, W242–W245. doi: 10.1093/nar/gkw290
Lie, T. J., Clawson, M. L., Godchaux, W., and Leadbetter, E. R. (1999). Sulfidogenesis from 2-Aminoethanesulfonate (Taurine) Fermentation by a Morphologically Unusual Sulfate-Reducing Bacterium, Desulforhopalus singaporensis sp. nov. Applied and Environmental Microbiology 65, 3328. doi: 10.1128/AEM.65.8.3328-3334.1999
Lien, T., Madsen, M., Steen, I. H., and Gjerdevik, K. (1998). Desulfobulbus rhabdoformis sp. nov., a sulfate reducer from a water–oil separation system. International Journal of Systematic Bacteriology 48, 469–474. doi: 10.1099/00207713-48-2-469
Lovley, D. R., and Phillips, E. J. P. (1994). Novel processes for anaerobic sulfate production from elemental sulfur by sulfate-reducing bacteria. Applied and Environmental Microbiology 60, 2394–2399.
Miller, M. A., Pfeiffer, W., and Schwartz, T. (2010). “Creating the CIPRES Science Gateway for inference of large phylogenetic trees,” in Proceedings of the Gateway Computing Environments Workshop (GCE), 14 Nov. 2010, (New Orleans, LA), 1–8.
Müller, H., Bosch, J., Griebler, C., Damgaard, L. R., Nielsen, L. P., Lueders, T., et al. (2016). Long-distance electron transfer by cable bacteria in aquifer sediments. The ISME Journal 10, 2010–2019. doi: 10.1038/ismej.2015.250
Müller, H., Marozava, S., Probst, A. J., and Meckenstock, R. U. (2020). Groundwater cable bacteria conserve energy by sulfur disproportionation. The ISME Journal 14, 623–634. doi: 10.1038/s41396-019-0554-1
Pace, L. A., Hemp, J., Ward, L. M., and Fischer, W. W. (2015). Draft genome of Thermanaerothrix daxensis GNS-1, a thermophilic facultative anaerobe from the Chloroflexi class Anaerolineae. Genome announcements 3, e1354–e1315. doi: 10.1128/genomeA.01354-15 ∗∗e01354-15,
Parey, K., Fritz, G., Ermler, U., and Kroneck, P. M. H. (2013). Conserving energy with sulfate around 100oC-structure and mechanism of key metal enzymes in hyperthermophilic archaeoglobus fulgidus. Metallomics 5, 302–317. doi: 10.1039/c2mt20225e
Parks, D. H., Chuvochina, M., Waite, D. W., Rinke, C., Skarshewski, A., Chaumeil, P. A., et al. (2018). A standardized bacterial taxonomy based on genome phylogeny substantially revises the tree of life. Nature biotechnology 36, 996–1004. doi: 10.1038/nbt.4229
Parks, D. H., Imelfort, M., Skennerton, C. T., Hugenholtz, P., and Tyson, G. W. (2015). CheckM: assessing the quality of microbial genomes recovered from isolates, single cells, and metagenomes. Genome research 25, 1043–1055. doi: 10.1101/gr.186072.114
Pellerin, A., Antler, G., Holm, S. A., Findlay, A. J., Crockford, P. W., Turchyn, A. V., et al. (2019). Large sulfur isotope fractionation by bacterial sulfide oxidation. SCIENCE ADVANCES 5, EAAW1480. doi: 10.1126/sciadv.aaw1480
Pereira, I. A. C., Ramos, A. R., Grein, F., Marques, M. C., Marques da Silva, S., and Venceslau, S. S. (2011). A comparative genomic analysis of energy metabolism in sulfate reducing bacteria and archaea. Frontiers of Microbiology 2:69. doi: 10.3389/fmicb.2011.00069
Pfeffer, C., Larsen, S., Song, J., Dong, M., Besenbacher, F., Meyer, R. L., et al. (2012). Filamentous bacteria transport electrons over centimetre distances. Nature 491, 218–221. doi: 10.1038/nature11586
Philippot, P., Van Zuilen, M., Lepot, K., Thomazo, C., Farquhar, J., and Van Kranendonk, M. J. (2007). Early archaean microorganisms preferred elemental sulfur, not sulfate. Science 317, 5844. doi: 10.1126/science.1145861
Palomo, A., Pedersen, A. G., Fowler, S. J., Dechesne, A., Sicheritz-Pontén, T., and Smets, B. F. (2018). Comparative genomics sheds light on niche differentiation and the evolutionary history of comammox Nitrospira. ISME J 12, 1779–1793. doi: 10.1038/s41396-018-0083-3
Poser, A., Lohmayer, R., Vogt, C., Knoeller, K., Planer-Friedrich, B., Sorokin, D., et al. (2013). Disproportionation of elemental sulfur by haloalkaliphilic bacteria from soda lakes. Extremophiles 17, 1003–1012. doi: 10.1007/s00792-013-0582-0
Rinke, C., Chuvochina, M., Mussig, A. J., Chaumeil, P. A., Waite, D. W., Whitman, W. B., et al. (2020). A rank-normalized archaeal taxonomy based on genome phylogeny resolves widespread incomplete and uneven classifications. bioRxiv [Preprint] doi: 10.1101/2020.03.01.972265
Rodriguez-R, L. M., and Konstantinidis, K. T. (2014). Bypassing cultivation to identify bacterial species. Microbe 9, 111–118.
Rosenberg, E., DeLong, E. L., Lory, S., Stackebrandt, E., and Thompson, F. (2014). The Prokaryotes – Deltaproteobacteria and Epsilonproteobacteria. Berlin: Springer-Verlag, doi: 10.1007/978-3-642-39044-9_267
Samain, E., Dubourguier, H. C., and Albagnac, G. (1984). Isolation and characterisation of Desulfobulbus elongatus sp. nov. from a mesophilic industrial digester. Systematic and Applied Microbiology 5, 391–401. doi: 10.1016/S0723-2020(84)80040-5
Sass, A., Rutter, H., Cypionka, H., and Sass, H. (2002). Desulfobulbus mediterraneus sp. nov., a sulfate-reducing bacterium growing on mono- and disaccharides. Archives of Microbiology 177, 468–474. doi: 10.1007/s00203-002-0415-5
Schauer, R., Risgaard-Petersen, N., Kjeldsen, K. U., Tataru Bjerg, J. J., Jørgensen, B. B., Schramm, A., et al. (2014). Succession of cable bacteria and electric currents in marine sediment. The ISME Journal 8, 1314–1322. doi: 10.1038/ismej.2013.239
Shen, Y., Buick, R., and Canfield, D. E. (2001). Isotopic evidence for microbial sulphate reduction in the early Archaean era. Nature 410, 6824. doi: 10.1038/35065071
Shih, P. M., Ward, L. M., and Fischer, W. W. (2017). Evolution of the 3-hydroxypropionate bicycle and recent transfer of anoxygenic photosynthesis into the Chloroflexi. Proceedings of the National Academy of Sciences 114, 10749–10754. doi: 10.1073/pnas.1710798114
Søndergaard, D., Pedersen, C. N., and Greening, C. (2016). HydDB: a web tool for hydrogenase classification and analysis. Scientific reports 6, 34212. doi: 10.1038/srep34212
Sorokin, D. Y., Tourova, T. P., Panteleeva, A. N., and Muyzer, G. (2012). Desulfonatronobacter acidivorans gen. nov., sp. nov. and Desulfobulbus alkaliphilus sp. nov. haloalkaliphilic heterotrophic sulfate-reducing bacteria from soda lakes. International Journal of Systematic and Evolutionary Microbiology 62, 2107–2113. doi: 10.1099/ijs.0.029777-0
Stamatakis, A. (2014). RAxML version 8: a tool for phylogenetic analysis and post-analysis of large phylogenies. Bioinformatics 30, 1312–1313. doi: 10.1093/bioinformatics/btu033
Suzuki, D., Ueki, A., Amaishi, A., and Ueki, K. (2007a). Desulfobulbus japonicus sp. nov., a novel Gram-negative, propionate-oxidizing, sulfate-reducing bacterium isolated from an estuarine sediment in Japan. International Journal of Systematic and Evolutionary Microbiology 57, 849–855. doi: 10.1099/ijs.0.64855-0
Suzuki, D., Ueki, A., Amaishi, A., and Ueki, K. (2007b). Desulfopila aestuarii gen. nov., sp. nov., a Gram-negative, rod-like,sulfate-reducing bacterium isolated from an estuarine sediment in Japan. International Journal of Systematic and Evolutionary Microbiology 57, 520–526. doi: 10.1099/ijs.0.64600-0
Thamdrup, B., Finster, K., Hansen, J. W., and Bak, F. (1993). Bacterial disproportionation of elemental sulfur coupled to chemical reduction of iron or manganese. Applied and Environmental Microbiology 59, 101–108.
Thorup, C., Schramm, A., Findlay, A. J., Finster, K. W., and Schreiber, L. (2017). Disguised as a Sulfate Reducer: Growth of the Deltaproteobacterium Desulfurivibrio alkaliphilus by Sulfide Oxidation with Nitrate. mBio 8, e671–e617. doi: 10.1128/mBio.00671-17
Umezawa, K., Kojima, H., Kato, Y., and Fukui, M. (2020). Disproportionation of inorganic sulfur compounds by a novel autotrophic bacterium belonging to Nitrospirota. Systematic and Applied Microbiology 43, 126110. doi: 10.1016/j.syapm.2020.126110
Vigneron, A., Cruaud, P., Alsop, E., de Rezende, J. R., Head, I. M., and Tsesmetzis, N. (2018). Beyond the tip of the iceberg; a new view of the diversity of sulfite- and sulfate-reducing microorganisms. ISME J 12, 2096–2099. doi: 10.1038/s41396-018-0155-4
Waite, D. W., Chuvochina, M., Pelikan, C., Parks, D. H., Yilmaz, P., Wagner, M., et al. (2020). Proposal to reclassify the proteobacterial classes Deltaproteobacteria and Oligoflexia, and the phylum Thermodesulfobacteria into four phyla reflecting major functional capabilities. International Journal of Systematic and Evolutionary Microbiology 70, 5972–6016. doi: 10.1099/ijsem.0.004213
Ward, L. M., Hemp, J., Pace, L. A., and Fischer, W. W. (2015). Draft genome sequence of Leptolinea tardivitalis YMTK-2, a mesophilic anaerobe from the Chloroflexi class Anaerolineae. Genome announcements 3, e1356–e1315. doi: 10.1128/genomeA.01356-15 ∗∗e01356-15,
Ward, L. M., Lingappa, U. F., Grotzinger, J. P., and Fischer, W. W. (2020). Microbial mats in the Turks and Caicos Islands reveal diversity and evolution of phototrophy in the Chloroflexota order Aggregatilineales. Environmental Microbiome 15, 9. doi: 10.1186/s40793-020-00357-8
Ward, L. M., and Shih, P. M. (2019). The evolution and productivity of carbon fixation pathways in response to changes in oxygen concentration over geological time. Free Radical Biology and Medicine 140, 188–199. doi: 10.1016/j.freeradbiomed.2019.01.049
Ward, L. M., Shih, P. M., and Fischer, W. W. (2018). MetaPOAP: presence or absence of metabolic pathways in metagenome-assembled genomes. Bioinformatics 34, 4284–4286. doi: 10.1093/bioinformatics/bty510
Ward, L. M. and Shih, P. M. (2021). Granick revisited: synthesizing evolutionary and ecological evidence for the late origin of bacteriochlorophyll via ghost lineages and horizontal gene transfer PloS one 16:e0239248. doi: 10.1371/journal.pone.0239248
Waterhouse, A. M., Procter, J. B., Martin, D. M. A., Clamp, M., and Barton, G. J. (2009). Jalview Version 2-a multiple sequence alignment editor and analysis workbench. Bioinformatics 25, 1189–1191. doi: 10.1093/bioinformatics/btp033
Widdel, F. (1980). Anaerober Abbau von Fettsauren und Benzoesaure durch neu isolierte Arten sulfat-reduzierender Bakterien. Dissertation. Lindhorst/Schaumburg-Lippe, Gottingen: Georg-August-Universitat zu Gottingen, 7–149.
Widdel, F., and Pfennig, N. (1982). Studies on dissimilatory sulfate-reducing bacteria that decompose fatty acids. II. Incomplete oxidation of propionate by Desulfobulbus propionicus gen. nov. sp. Nov. Arch Microbiol 131, 360–365. doi: 10.1007/BF00411187
Wing, B. A., and Halevy, I. (2014). Intracellular metabolite levels shape sulfur isotope fractionation during microbial sulfate respiration. Proceedings of the National Academy of Sciences 111, 18116–18125. doi: 10.1073/pnas.1407502111
Keywords: comparative genomics, sulfur, convergent evolution, dissimilatory sulfur metabolism, Desulfobulbaceae
Citation: Ward LM, Bertran E and Johnston DT (2021) Expanded Genomic Sampling Refines Current Understanding of the Distribution and Evolution of Sulfur Metabolisms in the Desulfobulbales. Front. Microbiol. 12:666052. doi: 10.3389/fmicb.2021.666052
Received: 09 February 2021; Accepted: 09 April 2021;
Published: 19 May 2021.
Edited by:
Denis Grouzdev, Federal Center Research Fundamentals of Biotechnology (RAS), RussiaReviewed by:
Kai Waldemar Finster, Aarhus University, DenmarkFilipa L. Sousa, University of Vienna, Austria
Copyright © 2021 Ward, Bertran and Johnston. This is an open-access article distributed under the terms of the Creative Commons Attribution License (CC BY). The use, distribution or reproduction in other forums is permitted, provided the original author(s) and the copyright owner(s) are credited and that the original publication in this journal is cited, in accordance with accepted academic practice. No use, distribution or reproduction is permitted which does not comply with these terms.
*Correspondence: Lewis M. Ward, bGV3aXNfd2FyZEBmYXMuaGFydmFyZC5lZHU=; Emma Bertran, ZWJlcnRyYW5AcHJpbmNldG9uLmVkdQ==
†These authors have contributed equally this work