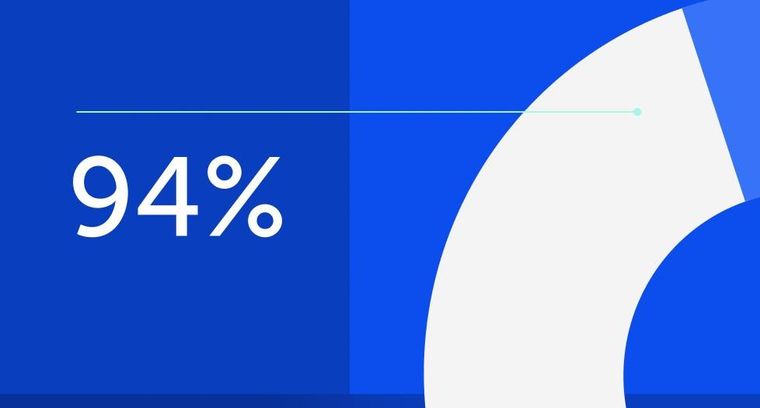
94% of researchers rate our articles as excellent or good
Learn more about the work of our research integrity team to safeguard the quality of each article we publish.
Find out more
ORIGINAL RESEARCH article
Front. Microbiol., 15 June 2021
Sec. Food Microbiology
Volume 12 - 2021 | https://doi.org/10.3389/fmicb.2021.662785
This article is part of the Research TopicInterspecies Interactions Within Fermented Food Systems and Their Impact on Process Efficiency and Product QualityView all 11 articles
The antagonistic activities of native Debaryomyces hansenii strains isolated from Danish cheese brines were evaluated against contaminating molds in the dairy industry. Determination of chromosome polymorphism by use of pulsed-field gel electrophoresis (PFGE) revealed a huge genetic heterogeneity among the D. hansenii strains, which was reflected in intra-species variation at the phenotypic level. 11 D. hansenii strains were tested for their ability to inhibit germination and growth of contaminating molds, frequently occurring at Danish dairies, i.e., Cladosporium inversicolor, Cladosporium sinuosum, Fusarium avenaceum, Mucor racemosus, and Penicillium roqueforti. Especially the germination of C. inversicolor and P. roqueforti was significantly inhibited by cell-free supernatants of all D. hansenii strains. The underlying factors behind the inhibitory effects of the D. hansenii cell-free supernatants were investigated. Based on dynamic headspace sampling followed by gas chromatography-mass spectrometry (DHS-GC-MS), 71 volatile compounds (VOCs) produced by the D. hansenii strains were identified, including 6 acids, 22 alcohols, 15 aldehydes, 3 benzene derivatives, 8 esters, 3 heterocyclic compounds, 12 ketones, and 2 phenols. Among the 71 identified VOCs, inhibition of germination of C. inversicolor correlated strongly with three VOCs, i.e., 3-methylbutanoic acid, 2-pentanone as well as acetic acid. For P. roqueforti, two VOCs correlated with inhibition of germination, i.e., acetone and 2-phenylethanol, of which the latter also correlated strongly with inhibition of mycelium growth. Low half-maximal inhibitory concentrations (IC50) were especially observed for 3-methylbutanoic acid, i.e., 6.32–9.53 × 10–5 and 2.00–2.67 × 10–4 mol/L for C. inversicolor and P. roqueforti, respectively. For 2-phenylethanol, a well-known quorum sensing molecule, the IC50 was 1.99–7.49 × 10–3 and 1.73–3.45 × 10–3 mol/L for C. inversicolor and P. roqueforti, respectively. For acetic acid, the IC50 was 1.35–2.47 × 10–3 and 1.19–2.80 × 10–3 mol/L for C. inversicolor and P. roqueforti, respectively. Finally, relative weak inhibition was observed for 2-pentanone and acetone. The current study shows that native strains of D. hansenii isolated from Danish brines have antagonistic effects against specific contaminating molds and points to the development of D. hansenii strains as bioprotective cultures, targeting cheese brines and cheese surfaces.
Mold contamination is a major problem occurring in food processing, which not only leads to severe economic losses and food waste but also may influence food safety (Garnier et al., 2017). In the dairy industry, mold contamination can appear throughout the entire cheese production as cheeses come into contact with processing equipment and air. Mold growth is especially seen during cheese ripening, storage, and distribution which results in a reduction of cheese quality including visible and invisible defects such as mold colonization, off-flavors, and potential risk of mycotoxin formation (Kure and Skaar, 2019). As a consequence, antifungal compounds and potential biocontrol agents have received increasing interest to prevent the growth of contaminating molds on cheeses (Irlinger and Mounier, 2009).
Yeasts are considered relevant for biocontrol applications, due to their simple cultivation requirements and limited biosafety concerns (Freimoser et al., 2019). A number of yeast species have been proven to have antagonistic activities against molds, though mostly applied in the control of post-harvest diseases of fruits (Bencheqroun et al., 2007; Liu et al., 2013; Grzegorczyk et al., 2017; Czarnecka et al., 2019). Within food production, antagonistic yeasts have been reported on, e.g., meat and dairy products (Liu and Tsao, 2010; Andrade et al., 2014) but their application is lacking behind. A number of antifungal volatile organic compounds (VOCs) produced by biocontrol yeasts have been associated with fungal inhibition, i.e., several alcohols (2-phenylethanol, ethanol, 2-methyl-1-butanol and 3-methyl-1-butanol, 2-methyl-1-propanol, and isoamyl alcohol) (Fialho et al., 2010; Ando et al., 2012; Núñez et al., 2015; Contarino et al., 2019) and esters (ethyl acetate, isoamyl acetate, phenylethyl acetate, isobutyl acetate, 2-phenyl ethyl acetate, and ethyl propionate) (Masoud et al., 2005; Fialho et al., 2010; Choińska et al., 2020). Although several studies state the production of VOCs involved in the antifungal activities of biocontrol yeasts, only a few of the aforementioned single VOCs have been investigated in depth. Also other antifungal actions of antagonistic yeasts have been reported including the competition for space and nutrients between yeasts and molds (Andrade et al., 2014; Medina-Córdova et al., 2016) as well as killer toxins playing a role in the defense system of yeasts against molds (Santos and Marquina, 2004; Grzegorczyk et al., 2017).
A number of yeasts have been detected and isolated from dairy products and dairy environment (Fröhlich-Wyder et al., 2019). Among these, D. hansenii has qualified presumption of safety (QPS) status by the European Food Safety Authority (EFSA) (Koutsoumanis et al., 2020) making it suitable as a biocontrol yeast in dairy products. D. hansenii is a halophilic yeasts being dominating among yeast species associated with most cheese varieties (Gori et al., 2012). Previously, we found that D. hansenii is by far the most predominant yeast species isolated from Danbo cheese brines reaching ≥ 3.5 log10 CFU/mL (Haastrup et al., 2018). Moreover, D. hansenii is a highly heterogeneous species showing phenotypic differences at the strain level. Variations among strains include differences in the ability to assimilate and ferment different carbon sources, secretion of dissimilar lipases and proteases, and diverse preferable growth conditions (Petersen and Jespersen, 2004). Consequently, strain variations in antagonistic potential might be expected. Antifungal activities of D. hansenii against contaminating molds are reported for several foods including dry-cured meat products (Andrade et al., 2014; Núñez et al., 2015), fruits (Hernández-Montiel et al., 2010), and dairy products (Van Den Tempel and Jakobsen, 2000; Liu and Tsao, 2009; Lessard et al., 2012). In dairy products, D. hansenii is reported to reduce the growth of Penicillium camemberti (Lessard et al., 2012). Further, D. hansenii strains obtained from blue mold cheeses are able to weakly inhibit P. roqueforti under aerobic conditions (Van Den Tempel and Jakobsen, 2000). Up to now, several factors have been found to influence the antifungal efficiency of D. hansenii, including water activity (aw), temperature, nutrient availability (Andrade et al., 2014; Núñez et al., 2015), and the concentration of molds (Liu and Tsao, 2009). Further, the production of metabolites varies among D. hansenii strains (Núñez et al., 2015; Grzegorczyk et al., 2017; Hernandez-Montiel et al., 2018). In previous studies, D. hansenii strains have been shown to exhibit varying antifungal actions (Van Den Tempel and Jakobsen, 2000; Medina-Córdova et al., 2018), however, further in-depth studies are required to explore these actions. We have previously determined the distinctive growth characteristics and NaCl tolerance of D. hansenii strains isolated from Danish cheese brines (Zhang et al., 2020), however, their potential abilities to inhibit mold contaminants from dairy environments have not, as yet, been explored.
This paper aims to evaluate the antifungal activities of D. hansenii strains isolated from Danish cheese brines against different contaminating molds. For this purpose, the effects of different D. hansenii strains were examined for their inhibitory capacity on germination and growth of contaminating molds including determination of the half-maximal inhibitory concentration (IC50) of single VOCs.
All D. hansenii strains used in this study were previously isolated and identified by Haastrup et al. (2018) from three different Danish dairies; D. hansenii strains KU-9, KU-10, KU-11, and KU-12 were isolated from dairy A (vat1); KU-27, KU-28, KU-29, and KU-30 were isolated from dairy A (vat2); KU-72 was isolated from dairy B; KU-78 and KU-80 were isolated from dairy C (vat1 and vat2, respectively). C. inversicolor, C. sinuosum, and F. avenaceum were obtained from a Danish dairy and their identification was verified by Deutsche Sammlung von Mikroorganismen (DSM, Germany). M. racemosus DSM5266 (isolated from cheese) and P. roqueforti DSM1079 (isolated from cheese) were obtained from DSM. All molds were capable of growing on cheese agar, prepared according to Sørensen et al. (2011) at 25°C for 7 days (Supplementary Figure 1). Of these, C. inversicolor, C. sinuosum, F. avenaceum, and P. roqueforti reproduce asexually by the formation of conidia, while M. racemosus reproduce sexually by the formation of spores. In the following, spores will be used when referring to these reproductive cells of the five molds.
Yeast strains were propagated at 25°C in Malt Yeast Glucose Peptone broth added 4% (w/v) NaCl (MYGP added 4% (w/v) NaCl; per liter, 10 g D (+)-glucose monohydrate (Merck, Darmstadt, Germany), 5 g bactopeptone (BD, Detroit, MI, United States), 3 g yeast extract (BD), 3 g malt extract (BD), 40 g NaCl (Merck) with pH adjusted to 5.3 ± 0.1) or MYGP agar added 4% (w/v) NaCl (adding 20 g bacto agar (BD) to MYGP broth added 4% (w/v) NaCl). Mold species were routinely grown at 25°C in Malt Extract medium (MEB; per liter; 20 g malt extract (BD), 10 g D(+)-glucose monohydrate (Merck), 5 g bactopeptone (BD) with pH adjusted to 5.3 ± 0.1) or on malt extract agar (MEA, adding 20 g bacto agar (BD) to MEB broth components).
Molds were cultured on MEA at 25°C for 7-14 days. Spores were suspended in saline peptone (SPO) solution (per liter; 5 g NaCl (Merck), 0.3 g Na2HPO4⋅2H2O (Merck), 1 g bactopeptone (BD) with pH adjusted to 5.3 ± 0.1) containing 0.01% Tween 80 (Sigma-Aldrich, St. Louis, United States) followed by filtering through six layers of sterilized gauze to remove hyphal fragments. Spore concentrations were estimated by bright field microscopy (Olympus BX40, Japan) using a Neubauer counting chamber and adjusted to a final concentration of 104 spores/mL using SPO solution.
The DNA preparation of yeast strains and the running condition of pulsed-field gel electrophoresis (PFGE) were performed according to Petersen and Jespersen (2004). The gels were visualized with UV transillumination and photographed (alphaeasefc software, Alpha-InnoTec GmbH, Germany). Estimation of the band size was analyzed using the LabImage 1D, ver.7.1.3 software (Kapelan Bio-Imaging, Germany). The cluster analysis was carried out using BioNumerics version 7.1 (Applied Maths, Kortrijk, Belgium). The similarities between profiles of bands were determined using the fraction of shared bands (Dice coefficient), and the cluster analysis was calculated by the unweighted pair group method using arithmetic average linkage (UPGMA method).
All D. hansenii strains were grown in MYGP broth added 4% (w/v) NaCl overnight. OD600nm of the overnight cultures were measured using a spectrophotometer (UV 1800, Shimadzu, Japan) followed by dilution with fresh media at initial concentration of 0.01 ± 0.005 (OD600nm). The cell cultures were grown in MYGP broth added 4% (w/v) NaCl for 72 h at 25°C. Measurements of pH were carried out using an electrode (In Lab 426, Mettler-Toledo, Glostrup, Denmark) connected to a pH meter (1120, Mettler-Toledo) and plate counting method on MYGP agar added 4% (w/v) NaCl was used to monitor the growth of D. hansenii at the initial and end time point.
Cell-free supernatants were made for each D. hansenii strain in the stationary growth phase (cultured in MYGP broth added 4% (w/v) NaCl for 72 h at 25°C) by centrifugation (3000 × g, 10 min) followed by filtering the supernatants using 0.22 μm pore size filter (Frisenette ApS, Knebel, Denmark).
Aliquots of 100 μL of double-strength MEB containing 104 spores/mL plus 100 μL of the cell-free supernatant collected from each D. hansenii strains or 100 μL of Yeast Peptone (per liter, 5 g bactopeptone, 3.0 g yeast extract, 40 g NaCl, pH 5.3) as control were loaded into wells (96 microtiter plates, Corning, New York, America). The antifungal activities of cell-free supernatants against molds were determined by measuring the growth curve using oCelloScopeTM Unisensor (Philips BioCell A/S, Denmark) at 25°C for 48 h. The oCelloScope detection system (objective, 4×) was described in detail by Fredborg et al. (2013). The image distance was 4.90 μm and the illumination exposure time was 2 ms. Time-lapse scanning microscopy through a fluid sample was conducted thereby generating a series of 6 images in each well, every 2 h. Growth curves were generated automatically by using the segmentation and extraction of surface areas (SESA) algorithm in UniExplorer (Philips BioCell A/S, Denmark).
Germination ratios of spores were analyzed by ImageJ (v1.51g-v1.51n; Fiji package). A spore with a germination tube longer than the spore itself was considered as a germinated spore. For each image, 90-120 spores were counted and relative germination ratios were calculated as follows:
where g1 is the number of germinated spores added cell-free supernatant, t1 is the total number of spores added cell-free supernatant, g0 is the number of germinated spores without cell-free supernatant, t0 is the total number of spores without cell-free supernatant. The time points for counting germination ratio were chosen according to the method from Trinci (1971).
Growth rate (μ) values were analyzed using the DMfit software available on the Combase website1 based on the aforementioned growth curve values and the model proposed by Baranyi and Roberts (1994). Relative mycelium growth rate was calculated as follows:
where μ1 is the growth rate added cell-free supernatant, μ0 is the growth rate without yeast cell-free supernatant. The μ values fit with R2 > 0.9 were considered valid data.
Debaryomyces hansenii cell-free supernatants were prepared as described above. VOCs of supernatants were collected in a dynamic headspace sampling (DHS) system. Each sample contained 20 mL supernatant sample plus 1 mL 4-methyl-1-pentanol (5 ppm) as the internal standard. The gas flask equipped with a purge head was equilibrated at 37°C in a water bath with magnetic stirring (200 rpm), and then purged with nitrogen (100 mL/min, 20 min). VOCs were collected by Tenax-TA traps (250 mg, mesh size 60/80, Buchem BV, Apeldoorn, Netherlands). Then the traps were continually purged with nitrogen (100 mL/min, 10 min) to remove excess water. The dynamic headspace collection was carried out in duplicates for all samples. VOCs were analyzed using gas chromatography-mass spectrometry (GC–MS) as described by Liu et al. (2015) and were identified based on the commercial database (Wiley275.L, HP product no. G1035A). The identified compounds were confirmed by comparing with retention indices (RI) of authentic reference compounds or the average of retention indices reported in the literature. All identified VOCs were semi-quantified as peak areas in the total ion chromatogram (TIC).
Volatile compounds were selected based on correlation between the relative peak area of each compound produced by D. hansenii and the inhibition data (relative germination ratio and relative mycelium growth rate obtained from the experiments described in the section “Effect of D. hansenii Cell-Free Supernatants on the Growth of Molds”) through Spearman correlation analysis. Those with significant strong correlations (P < 0.05) were selected for the subsequent experiments and purchased from Sigma-Aldrich. The interpretation of the rank correlation was according to the rules in Akoglu (2018).
Aliquots of 200 μL of MEB containing 104 spores/mL supplemented with acetic acid, 3-methylbutanoic acid, acetone, 2-pentanone, and 2-phenylethanol (Sigma-Aldrich, St. Louis, MO, United States) to the final concentrations 100, 10–1, 10–2, 10–3, 10–4, 10–5, and 10–6 mol/L were loaded into 96 well plates. Aliquots of 200 μL of MEB containing 104 spores/mL were included as controls. The antifungal activities of single identified VOCs were determined by measuring the growth curve using oCelloScopeTM Unisensor at 25°C for 48 h. Relative germination ratios and relative mycelium growth rates were calculated as described above. In addition, IC50 of single targeted VOCs for each mold was calculated. Long-term impacts of the five single targeted VOCs on the formation of mycelial pellicles were evaluated by prolonged incubation of the 96-well microtiter plates at 25°C for 7 days with the concentrations of the five single targeted VOCs as listed above. By visual inspection inhibition of mycelial pellicle formation was scored.
Standard curves based on matrix-spiked samples were performed for two acids (acetic acid and 3-methylbutanoic acid), one alcohol (2-phenylethanol), and two ketones (acetone, and 2-pentanone). The peak area of the quantifier ion was used for estimating the concentration of each aforementioned VOCs produced by D. hansenii strains.
Data were expressed as mean ± standard deviation and compared using one-way ANOVA analyzed by SPSS 17.0. Comparison of means was performed using Duncan’s multiple range test and the statistical significance was applied at the level P < 0.05. IC50 of single VOCs was analyzed by GraphPad Prism 5, while the Spearman correlation analysis was analyzed by R software (“Hmisc” package).
The chromosomal profiles of the 11 D. hansenii strains isolated from brines at three different Danish dairies are shown in Figure 1 and bands sizes are reported in Supplementary Table 1. Among the strains, genetic diversity was evident. The numbers of chromosomal bands varied between five and seven with sizes from 0.98 to 3.14 Mb. A cluster analysis based on the chromosomal profiles divided the 11 D. hansenii strains into four clusters at a similarity level of 60% (Figure 1B). Nine of the D. hansenii strains had unique profiles, while the two strains in cluster III (KU-11 and KU-28) isolated from two different vats at dairy A (A1 and A2) had identical profiles. D. hansenii strains (KU-9, KU-10, KU-11, KU-12, KU-27, KU-28, KU-29, and KU-30) isolated from the two vats at dairy A (A1 and A2) were divided into three different clusters (cluster II, III, and IV). Further, strains from dairy C were in cluster I (KU-78 and KU-80), clearly separated from the remaining dairies. On the contrary, no clear separation could be observed between dairy A and B as they clustered together in cluster II (KU-9, KU-12, KU-30, and KU-72), though at low similarity.
Figure 1. (A) Chromosome polymorphism of D. hansenii strains isolated from Danish cheese brines. Pulsed-field gel electrophoresis (PFGE) was carried out at a 200 switch interval at 150 V for 24 h followed by a 700 s switch interval at 100 V for 48 h. Markers: H, Hansenula wingei; S, Saccharomyces cerevisiae. (B) Dice/UPMGA clustering dendrogram of D. hansenii strains.
Growth and changes in pH of D. hansenii strains grown in MYGP added 4% (w/v) NaCl for 72 h at 25°C are shown in Table 1. Examples of growth curves of D. hansenii strains are shown in Supplementary Figure 2. The initial inocula of the 11 D. hansenii strains were 0.7 ± 0.2 × 104 CFU/mL and reached 4.2 ± 1.7 × 107 CFU/mL after 72 h of growth. Even though all of the 11 D. hansenii strains grew to similar levels there were huge variations in their acidification abilities. After 72 h growth, acidification of MYGP from pH 5.3 to a minimum of 4.3 was observed for six D. hansenii strains (KU-9, KU-11, KU-12, KU-28, KU-30, and KU-72). Contrary, deacidification of MYGP from pH 5.3 to a maximum of 6.1 was observed for four D. hansenii strains (KU-10, KU-27, KU-29, and KU-78), whereas one strain (KU-80) did not significantly change the pH during the 72 h growth. Hence, compared to the cluster analysis, the genetic diversity and acidification/deacidification abilities were partly correlated. The acidifying strains were in clusters II and III, while cluster IV comprised three of the four deacidifying strains. Finally, cluster I contained one deacidifying strain (KU-78) and one strain that did not change pH (KU-80), however, rather high genetic differences between the two strains were detected at 62%.
Table 1. Growth of D. hansenii strains and change in pH of MYGP broth added 4% (w/v) NaCl after 72h inoculation at 25°C.
Effects on germination ratios and mycelium growth rates of the five molds when exposed to D. hansenii cell-free supernatants collected from stationary phase cultures are shown in Table 2. For the germination ratios, the highest significant (P < 0.05) inhibition, among the five molds, was observed for C. inversicolor, with germination ratios below 5% when exposed to cell-free supernatants of all D. hansenii strains. For P. roqueforti, cell-free supernatants of D. hansenii KU-9 and KU-11 showed significant strong inhibition on the germination ratio to 9.5% and 6.8%, respectively, compared to cell-free supernatants of the other D. hansenii strains (13.7-33.8%). Slight inhibition by D. hansenii cell-free supernatants was observed on the germination ratio of C. sinuosum (82.9%). Contrary, no significant (P > 0.05) inhibitory effect on the germination ratios of F. avenaceum and M. racemosus was observed for any of the D. hansenii cell-free supernatants.
Table 2. Germination ratios (%) and mycelium growth rates (μ/h) of molds in presence of D. hansenii cell-free supernatants as compared to the control.
For mycelium growth rates, corresponding to exponential growth phase of molds, cell-free supernatants of all D. hansenii strains induced inhibition of C. inversicolor (69.3%) while slight inhibition of P. roqueforti (83.0%) and M. racemosus (86.8%) was observed. Mycelium growth rates of C. sinuosum and F. avenaceum were not affected by any of the D. hansenii cell-free supernatants.
The limited effect of cell-free supernatants collected from stationary phase cultures of D. hansenii strains were additionally confirmed by the growth curves of the molds (Figure 2). Hence, for C. inversicolor and P. roqueforti, the time point of reaching stationary phase was delayed by the D. hansenii cell-free supernatants, while no inhibitory effect was observed for C. sinuosum, F. avenaceum, and M. racemosus. Moreover, at the end of incubation (i.e., 48 h), the D. hansenii cell-free supernatants did not lead to reduced mycelium growth of any of the five tested molds. Overall, these results showed that the D. hansenii cell-free supernatants predominantly influenced the germination phase in a mold species dependent manner, rather than in a D. hansenii strain dependent manner.
Figure 2. Effects of cell-free supernatants from D. hansenii strains KU-9, KU-10, KU-11, KU-12, KU-27, KU-28, KU-29, KU-30, KU-72, KU-78 and KU-80 on the growth of five mold species measured as Normalized SESA units using oCelloScopeTM. (A) Cladosporium sinuosum, (B) Fusarium avenaceum, (C) Mucor racemosus, (D) Cladosporium inversicolor, (E) Penicillium roqueforti. Each spot represents the mean values from three independent experiments, and the error bars represent the standard deviation. (F) Examples of oCelloScopeTM images of growth measurements of C. inversicolor (blue) and P. roqueforti (red) in yeast peptone media (control) or in the presence of cell-free supernatants of D. hansenii strain KU-27 at 24 h. Scale bar: 300 μm.
To elucidate the mechanisms of action involved in the antagonistic activities, the VOCs produced by stationary phase cultures of the 11 D. hansenii strains were determined. In total, 71 metabolites were detected by GC-MS and identified, i.e., 6 acids, 22 alcohols, 15 aldehydes, 3 benzene derivatives, 8 esters, 3 heterocyclic compounds, 12 ketones, and 2 phenols (Supplementary Table 2 lists all detected VOCs). The relative contents of each metabolite group are shown in Table 3. Variations in VOC contents were seen among the D. hansenii strains. Especially acids, alcohols, benzene derivatives, esters, ketones, and phenols were among the produced VOCs. Significantly decreased content of aldehydes was detected for all D. hansenii strains, except KU-80, while all D. hansenii strains had decreased content of heterocyclic compounds. Moreover, significant contents of acids and ketones were produced by D. hansenii strains (KU-12 and KU-30). While some D. hansenii strains produced significant contents of benzene derivatives (KU-9, KU-11, and KU-27), esters (KU-78, KU-9, KU-10, KU-29, and KU-27), and phenols (KU-11). Hence, strain-dependent VOCs profiles were obtained from the D. hansenii strains, however, no correlation between VOC profiles and the genetic clusters from the PFGE could be observed.
To better understand the correlation between the VOCs produced by the D. hansenii strains and their antifungal behaviors, Spearman’s rank correlation analysis was applied. The results of the correlation and coefficient analysis are shown in Table 4 and Supplementary Figure 3. Among the 71 VOCs, acetic acid, 3-methylbutanoic acid, and 2-pentanone exhibited a strong negative correlation with germination ratios for C. inversicolor. For P. roqueforti, 2-phenylethanol had a strong negative correlation with both germination ratio and mycelium growth rate, while acetone had a strong negative correlation with germination ratio. Thus, these five VOCs were chosen as targeted single VOCs in the following experiments.
Table 4. Spearman’s correlation coefficient analysis between germination ratio (%)/mycelium growth rate (μ/h) and the VOCs detected in the cell-free supernatants of D. hansenii strains.
The inhibitory effects of targeted single VOCs were examined successively at different growth stages of C. inversicolor and P. roqueforti, i.e., on germination ratios and mycelium growth rates within 48 h (Figure 3 and Supplementary Figure 4). Based on germination ratios and mycelium growth rates in Figure 3, half-maximal inhibitory concentrations (IC50) were calculated for each growth stage, respectively (Table 5).
Figure 3. Inhibitory effects of targeted single VOCs on germination ratios (%) of C. inversicolor (A) and P. roqueforti (C) and mycelium growth rates (μ/h) of C. inversicolor (B) and P. roqueforti (D) at different concentrations (100, 10–1, 10–2, 10–3, 10–4, 10–5, and 10–6 mol/L). Each spot represents the mean value from three independent experiments, and the error bars represent the standard deviations.
Table 5. IC50 of different VOCs for inhibiting germination and mycelium growth of P. roqueforti and C. inversicolor.
For the germination ratios, gradually increasing concentration of 3-methylbutanoic acid, 2-phenylethanol, acetic acid, 2-pentanone, and acetone, respectively, were needed to obtain IC50 for C. inversicolor and P. roqueforti. For 3-methylbutanoic acid, IC50 were obtained at 9.53 × 10–5 and 2.00 × 10–4 mol/L for C. inversicolor and P. roqueforti, respectively. For 2-phenylethanol, IC50 were 1.99 × 10–3 and 1.73 × 10–3 mol/L for C. inversicolor and P. roqueforti, respectively. For acetic acid, IC50 were 2.47 × 10–3 and 2.80 × 10–3 mol/L for C. inversicolor and P. roqueforti, respectively. 2-pentanone had an inhibitory effect on germination ratios of C. inversicolor and P. roqueforti with an IC50 of 1.06 × 10–2 and 2.60 × 10–2 mol/L, respectively. Finally, acetone exhibited almost no inhibitory effect on germination ratio of C. inversicolor within the experimental period (48 h) but weakly inhibited germination ratio of P. roqueforti at IC50 of 9.21 × 10–2 mol/L.
For mycelium growth rates, gradually increasing concentrations of 3-methylbutanoic acid, acetic acid, 2-phenylethanol, 2-pentanone, and acetone, respectively, were needed to obtain IC50 for C. inversicolor and P. roqueforti. For 3-methylbutanoic acid, IC50 were 6.32 × 10–5 and 2.67 × 10–4 mol/L for C. inversicolor and P. roqueforti, respectively. For acetic acid, IC50 were 1.35 × 10–3 and 1.19 × 10–3 mol/L for C. inversicolor and P. roqueforti, respectively. For 2-phenylethanol, IC50 were 7.49 × 10–3 and 3.45 × 10–3 mol/L for C. inversicolor and P. roqueforti, respectively. Additionally, 2-pentanone showed a weak inhibitory effect on mycelium growth rate of C. inversicolor and P. roqueforti, whereas acetone exhibited no inhibitory effect on mycelium growth rates of the two tested molds.
Finally, for confirming the IC50 results as well as for evaluating long-term effects of exposure to different concentrations of the targeted VOCs, complete inhibition of mycelial pellicle formation was subsequently scored by visual inspections after 7 days incubation (Table 6). As expected, after long-term exposure to the targeted VOCs, inhibition of mycelial pellicle formation confirmed the IC50 results for both C. inversicolor and P. roqueforti. Hence, the highest inhibition was obtained for 3-methylbutanoic acid for both C. inversicolor and P. roqueforti, for which the concentrations were 10–3 and 10–2 mol/L, respectively. The concentrations of 2-phenylethanol and acetic acid for preventing the formation of mycelial pellicles were 10–2 and 10–1 mol/L for C. inversicolor and P. roqueforti, respectively. Furthermore, the concentration of 2-pentanone was 1 mol/L for C. inversicolor, while no inhibition on mycelial pellicle formation was observed for P. roqueforti after 7 days. Finally, acetone did not inhibit mycelial pellicle formation of either of the two molds after 7 days.
Table 6. Inhibitory effects of five VOCs on the formation of mycelial pellicles of molds after 7 days.
Among the targeted VOCs, estimated concentrations produced by the D. hansenii strains were calculated, when grown under the experimental conditions. The D. hansenii strains were found to produce estimated concentrations of 2-phenylethanol at 10–3 mol/L (data not shown), which was at the same concentration level as the detected IC50 for both C. inversicolor and P. roqueforti. The strains were additionally found to produce an estimated concentration of 10–6 mol/L for 3-methylbutanoic acid and 2-pentanone, and 10–4 mol/L for acetic acid and acetone (data not shown), which were all lower than the detected IC50 for both C. invercolor and P. roqueforti for the respective VOCs.
In this study, we evaluated D. hansenii strains isolated from Danish cheese brines for their antifungal activities against dairy contaminating molds. Our PFGE result confirmed the genetic heterogeneity of D. hansenii as previously reported (Petersen et al., 2002; Petersen and Jespersen, 2004). In Petersen et al. (2002), chromosome polymorphism among D. hansenii strains could be linked to different pH and NaCl tolerances. The variations in acidification or deacidification capabilities among the D. hansenii strains, found in the present study, could to some extend be linked to the genetic variance observed. The pH differences of the broth media (MYGP added with 4% (w/v) NaCl) might be related to D. hansenii producing or assimilating organic acids, proteolytic activity, production of ammonia, cell lysis, as well as several other factors (Gustafsson, 1979; Gancedo and Serrano, 1989; Freitas et al., 1999; Flores et al., 2004; Gori et al., 2007, 2012). Contrary, the VOCs profiles produced by the D. hansenii strains, in the present study, could not be linked to the specific chromosomal clusters as observed by PFGE, even though strain dependent differences in VOC production were observed. Strain-dependent VOC profiles have previously been observed among D. hansenii strains differing in M13 minisatellites, isolated from traditional ewe’s and goat’s cheese (Padilla et al., 2014b).
Among the five contaminating molds, P. roqueforti is, besides being a contaminant on surface ripened cheeses, a well known starter culture used in the production of blue-veined cheeses. Mucor spp. are well known on a variety of mold-ripened cheeses, especially from raw milk hard cheeses such as Saint Nectaire and Tomme de Savoie (Fox et al., 2004), and M. racemosus has especially been isolated from French cheeses (Hermet et al., 2015). Further, F. avenaceum has predominantly been reported as a mycotoxin producing pathogen on cereals, especially pronounced under cool and wet or humid conditions (Uhlig et al., 2007), however, it has also been isolated as a contaminating mold on Italian cheese (Montagna et al., 2004). Likewise, F. avenaceum was isolated at Danish dairies and identified to the species level in the present study. Finally, Cladosporium spp. have been isolated from traditional Italian cheese (De Santi et al., 2010). Originally isolated at Danish dairies, C. inversicolor and C. sinuosum were identified to species level, for the purpose of this study. However, C. inversicolor and C. sinuosum are so far mostly reported from plant materials (Bensch et al., 2015). Altogether, this indicates a huge species diversity among contaminating molds in dairy environments, far beyond what is usually investigated.
In the present study, the D. hansenii cell-free supernatants inhibited spore (conidia) germination and had only minor inhibitory effect on mold growth, indicating a fungistatic effect, which might be associated with the morphology of the spores or conidia of the five molds. Interestingly, the two Cladosporium spp., i.e., C. inversicolor and C. sinuosum responded very different to the D. hansenii cell-free supernatants. Germination and mycelium growth of C. inversicolor were significantly inhibited by cell-free supernatants of the D. hansenii strains, whereas we only observed a slight inhibition of germination for C. sinuosum and no effect on mycelium growth. The Cladosporium genus represents one of the larges genera of dematiaceous hyphomycetes and comprises several complexes, which are differentiated morphologically based on especially conidia morphology. C. inversicolor is in the Cladosporium cladosporioides complex and contains numerous conidia in long chains of up to eight conidia with walls typically being unthickened. C. sinuosum is in the Cladosporium herbarum complex and contains solitary conidia or short chains of up to four conidia which appear to be thick walled due to surface ornamentation (Bensch et al., 2015). These differences might explain some of the variance in the susceptibility observed between the two Cladosporium species.
Among the 71 VOCs produced by D. hansenii, five targeted VOCs (acetic acid, 3-methylbutanoic acid, acetone, 2-phenylethanol, and 2-pentanone) had a strong association with inhibition of germination and/or mycelium growth of C. inversicolor and P. roqueforti and were selected for detailed analyses. Accordingly, D. hansenii has been shown to produce acetic acid, acetone, 3-methylbutanoic acid, 2-pentanone, and 2-phenylethanol in cheese-like medium (Padilla et al., 2014a). Moreover, D. hansenii increased the amount of 2-pentanone in feta cheese (Bintsis and Robinson, 2004). In our study, 3-methylbutanoic acid had the strongest inhibitory effect and the lowest IC50 against germination and mycelium growth of C. inversicolor and P. roqueforti at 10–5 and 10–4mol/L, respectively. The sensory threshold for 3-methylbutanoic acid is reported to 120-700 ppb (1.17-6.85 × 10–6 mol/L) and at higher concentrations, this branched-chain fatty acid has been described to contribute with unpleasant odors as floor cloth, feet, and sweaty flavors in surface ripened cheeses from France (Lecanu et al., 2002). The estimated concentration of 3-methylbutanoic acid (10–6 mol/L) detected in the present study was therefore within the sensory thresholds but lower than the IC50 values mentioned above. Even so, the potential use of D. hansenii strains producing 3-methylbutanoic acid as a biocontrol agent is still interesting. Higher levels of 3-methylbutanoic acid might potentially be obtained under different processing conditions, as reported by Durá et al. (2004) where NaCl and lactate increased the levels of 3-methylbutanoic acid produced by D. hansenii, and detailed analyses still need to be carried out in cheese under relevant maturation conditions.
The VOC 2-phenylethanol is reported as a common inhibitory compound produced by several biocontrol yeasts including Debaryomyces nepalensis, Wickerhamomyces anomalus, Metschnikowia pulcherrima, Saccharomyces cerevisiae, and Pichia manshurica (formerly, Pichia galeiformis) (Zhou et al., 2018; Contarino et al., 2019; Chen et al., 2020). In this study, we found a strong inhibitory effect of 2-phenylethanol on germination and mycelium growth of C. inversicolor and P. roqueforti at 10–3 mol/L. Accordingly, we have previously found that 2-phenylethanol completely impaired conidia germination, hyphal membrane integrity, and growth of another two food spoilage molds, i.e., Penicillium expansum and Penicillium nordicum at 15 × 10–3 mol/L (Huang et al., 2020). The concentration of 2-phenylethanol produced by D. hansenii strains in the present study was approximately 10–3 mol/L and therefore comparable with the IC50 (10–3 mol/L level), thus highlighting the potential application of the D. hansenii strains for biological production of 2-phenylethanol as an antifungal agent. Moreover, 2-phenylethanol is a well-known quorum sensing molecule produced by D. hansenii, and is found to upregulate traits involved in adhesion and sliding motility of D. hansenii (Gori et al., 2011), increasing the colonization probability on cheese surfaces (Mortensen et al., 2005; Gori et al., 2011), which in turn could contribute to the quality of the final product.
Acetic acid is a common antimicrobial compound and its antifungal activity is well investigated (Cabo et al., 2002; Fernandez et al., 2017; Guimarães et al., 2018; Sadiq et al., 2019). In this study, the IC50 for acetic acid was found at 10–3 mol/L against C. inversicolor and P. roqueforti. Quattrini et al. (2019) reported the minimum inhibitory concentration (MIC) of acetic acid for P. roqueforti growth to 2.5 ± 0.6 × 10–2 mol/L after 5 days, which is somewhat lower than the concentration of acetic acid for preventing mycelial pellicle formation of P. roqueforti (approximately 10–1 mol/L) after 7 days, in the present study. In Quattrini et al. (2019), MIC was determined after 5 days in conditions mimicking sourdough using modified MRS, while in the present study inhibition was scored after 7 days in MEB, a medium for optimal mold growth. These differences could explain the different concentrations determined.
In soft cheeses, 2-pentanone has been detected in Camembert, Vacherin, Limburger, Trappist, Gorgonzola and blue cheese above the odor threshold of 0.5-61 ppm contributing fruity, acetone, sweet, and ethereal odor (Sablé and Cottenceau, 1999). In the current study, 2-pentanone showed a weak inhibitory effect on the germination and mycelium growth of C. inversicolor and P. roqueforti. Further, the estimated concentration of 2-pentanone produced by D. hansenii in this study, was lower than the detected IC50 but within the ranges of odor threshold reported in previous studies (Sablé and Cottenceau, 1999).
The results of the present study indicate that the antifungal activity of D. hansenii is complex and likely results from a synergistic and or additive effect of several inhibitory VOCs, rather than the effect of single compounds. Similarly, growth inhibition of postharvest pathogenic molds in packaged fresh fruit by the proven biocontrol yeasts W. anomalus BS91 and M. pulcherrima MPR3 has been suggested to arise from a synergistic effect of VOCs and CO2 (Contarino et al., 2019). Moreover, inhibition of Fusarium spp. growth in the presence of cell-free supernatants of Lactiplantibacillus plantarum (formerly, Lactobacillus plantarum) has been shown not to arise alone from the lactic acid being produced by the lactic acid bacteria (Laitila et al., 2002). Further, additional mechanisms, not investigated in the current study, might also add to the combined antifungal activity of D. hansenii, including non-volatile compounds like killer toxins (Banjara et al., 2016), and competition for nutrients and space (Spadaro and Droby, 2016). As nutrients and space are generally limited in cheese processing (Irlinger and Mounier, 2009), this could be especially relevant. Interestingly, D. hansenii isolated from dry-cured meat products has been reported to inhibit toxigenic penicillia in co-culture assays on solid media, which could not be fully reproduced by cell-free supernatants or mouth-to-mouth assays. This indicates that efficient mold inhibition relied on additive or synergistic effects of the yeast inhibition factors such as competition for nutrients and space as well as production of soluble compounds or VOCs (Núñez et al., 2015). Similarly, P. manshurica has been shown to colonize and amplify quickly in citrus wounds and further to produce eight VOCs, including acetic acid and 2-phenylethanol during growth, which inhibited growth of Penicillium digitatum, causing citrus green mold (Chen et al., 2020).
In conclusion, genetic differences of D. hansenii together with diverse acidifying or deacidifying capability were confirmed. Cell-free supernatants of D. hansenii strains exhibited a strong inhibitory effect on C. inversicolor and P. roqueforti. Using DHS-GC-MS, 71 VOCs produced by D. hansenii strains were identified. Among the VOCs, strong correlation between inhibition of germination of C. inversicolor and P. roqueforti were obtained for five VOCs (acetic acid, acetone, 3-methylbutanoic acid, 2-pentanone, and 2-phenylethanol), while 2-phenylethanol also correlated strongly with inhibition of mycelium growth of P. roqueforti. 3-methylbutanoic acid showed the strongest inhibitory effects on germination and mycelium growth of the two mold species, i.e., IC50 of 10–5 and 10–4 mol/L, respectively. 2-phenylethanol and acetic acid also exhibited strong inhibitory effects on germination and mycelium growth at IC50 of 10–3 mol/L. Relative weak inhibitory effects of 2-pentanone and acetone were obtained in this study. The results from the current study point to an additive and possibly synergistic effect of the antifungal compounds produced by D. hansenii, which needs to be further studied. From an overall perspective, it can be concluded that some native D. hansenii strains in the dairy manufacturing environment have a so far neglected role in the natural preservation of cheeses and are eligible for biocontrol of contaminating molds in cheese production. The results additionally point to the coming use of halophilic D. hansenii strains as biocontrol agents to be added as bioprotective cultures in the dairy industry, e.g., as an additive to cheese brines.
The original contributions presented in the study are included in the article/Supplementary Material, further inquiries can be directed to the corresponding author/s.
CH, LZ, PJ, NA, and LJ conceived and designed the study. MP contributed to the GC-MS analyses. CH performed the experiments and drafted the manuscript. PJ, NA, and LJ revised the manuscript. All authors contributed to the article and approved the submitted version.
This work was conducted as a part of the Danish InBrine project. The authors are grateful to the Danish Dairy Board (MFF) for funding of the research. Additional funding was obtained by the Chinese Scholarship Council (CSC201706350030 and CSC201706350054).
The authors declare that the research was conducted in the absence of any commercial or financial relationships that could be construed as a potential conflict of interest.
The Supplementary Material for this article can be found online at: https://www.frontiersin.org/articles/10.3389/fmicb.2021.662785/full#supplementary-material
Akoglu, H. (2018). User’s guide to correlation coefficients. Turkish J. Emerg. Med. 18, 91–93. doi: 10.1016/j.tjem.2018.08.001
Ando, H., Hatanaka, K., Ohata, I., Yamashita-Kitaguchi, Y., Kurata, A., and Kishimoto, N. (2012). Antifungal activities of volatile substances generated by yeast isolated from Iranian commercial cheese. Food Control 26, 472–478. doi: 10.1016/j.foodcont.2012.02.017
Andrade, M. J., Thorsen, L., Rodríguez, A., Córdoba, J. J., and Jespersen, L. (2014). Inhibition of ochratoxigenic moulds by Debaryomyces hansenii strains for biopreservation of dry-cured meat products. Int. J. Food Microbiol. 170, 70–77. doi: 10.1016/j.ijfoodmicro.2013.11.004
Banjara, N., Nickerson, K. W., Suhr, M. J., and Hallen-Adams, H. E. (2016). Killer toxin from several food-derived Debaryomyces hansenii strains effective against pathogenic Candida yeasts. Int. J. Food Microbiol. 222, 23–29. doi: 10.1016/j.ijfoodmicro.2016.01.016
Baranyi, J., and Roberts, T. A. (1994). A dynamic approach to predicting bacterial growth in food. Int. J. Food Microbiol. 23, 277–294. doi: 10.1016/0168-1605(94)90157-0
Bencheqroun, S. K., Bajji, M., Massart, S., Labhilili, M., Jaafari, S. E., and Jijakli, M. H. (2007). In vitro and in situ study of postharvest apple blue mold biocontrol by Aureobasidium pullulans: evidence for the involvement of competition for nutrients. Postharvest Biol. Technol. 46, 128–135. doi: 10.1016/j.postharvbio.2007.05.005
Bensch, K., Groenewald, J. Z., Braun, U., Dijksterhuis, J., de Jesús Yáñez-Morales, M., and Crous, P. W. (2015). Common but different: the expanding realm of Cladosporium. Stud. Mycol. 82, 23–74. doi: 10.1016/j.simyco.2015.10.001
Bintsis, T., and Robinson, R. K. (2004). A study of the effects of adjunct cultures on the aroma compounds of Feta-type cheese. Food Chem. 88, 435–441. doi: 10.1016/j.foodchem.2004.01.057
Cabo, M. L., Braber, A. F., and Koenraad, P. M. F. J. (2002). Apparent antifungal activity of several lactic acid bacteria against Penicillium discolor is due to acetic acid in the medium. J. Food Prot. 65, 1309–1316. doi: 10.4315/0362-028X-65.8.1309
Chen, O., Yi, L., Deng, L., Ruan, C., and Zeng, K. (2020). Screening antagonistic yeasts against citrus green mold and the possible biocontrol mechanisms of Pichia galeiformis (BAF03). J. Sci. Food Agric. 100, 3812–3821. doi: 10.1002/jsfa.10407
Choińska, R., Piasecka-Jóźwiak, K., Chabłowska, B., Dumka, J., and Łukaszewicz, A. (2020). Biocontrol ability and volatile organic compounds production as a putative mode of action of yeast strains isolated from organic grapes and rye grains. Antonie van Leeuwenhoek Int. J. Gen. Mol. Microbiol. 113, 1135–1146. doi: 10.1007/s10482-020-01420-7
Contarino, R., Brighina, S., Fallico, B., Cirvilleri, G., Parafati, L., and Restuccia, C. (2019). Volatile organic compounds (VOCs) produced by biocontrol yeasts. Food Microbiol. 82, 70–74. doi: 10.1016/j.fm.2019.01.008
Czarnecka, M., Żarowska, B., Połomska, X., Restuccia, C., and Cirvilleri, G. (2019). Role of biocontrol yeasts Debaryomyces hansenii and Wickerhamomyces anomalus in plants’ defence mechanisms against Monilinia fructicola in apple fruits. Food Microbiol. 83, 1–8. doi: 10.1016/j.fm.2019.04.004
De Santi, M., Sisti, M., Barbieri, E., Piccoli, G., Brandi, G., and Stocchi, V. (2010). A combined morphologic and molecular approach for characterizing fungal microflora from a traditional Italian cheese (Fossa cheese). Int. Dairy J. 20, 465–471. doi: 10.1016/j.idairyj.2010.02.004
Durá, M. A., Flores, M., and Toldrá, F. (2004). Effect of growth phase and dry-cured sausage processing conditions on Debaryomyces spp. generation of volatile compounds from branched-chain amino acids. Food Chem. 86, 391–399. doi: 10.1016/j.foodchem.2003.09.014
Fernandez, B., Vimont, A., Desfossés-Foucault, É, Daga, M., Arora, G., and Fliss, I. (2017). Antifungal activity of lactic and propionic acid bacteria and their potential as protective culture in cottage cheese. Food Control 78, 350–356. doi: 10.1016/j.foodcont.2017.03.007
Fialho, M. B., Toffano, L., Pedroso, M. P., Augusto, F., and Pascholati, S. F. (2010). Volatile organic compounds produced by Saccharomyces cerevisiae inhibit the in vitro development of Guignardia citricarpa, the causal agent of citrus black spot. World J. Microbiol. Biotechnol. 26, 925–932. doi: 10.1007/s11274-009-0255-4
Flores, M., Durá, M. A., Marco, A., and Toldrá, F. (2004). Effect of Debaryomyces spp. on aroma formation and sensory quality of dry-fermented sausages. Meat Sci. 68, 439–446. doi: 10.1016/j.meatsci.2003.04.001
Fox, P. F., McSweeney, P. L., Cogan, T. M., and Guinee, T. P. (2004). “General aspects,” in Cheese: Chemistry, Physics and Microbiology, Vol. 1, eds P. F. Fox, P. L. McSweeney, T. M. Cogan, and T. P. Guinee (London: Elsevier), 1–18.
Fredborg, M., Andersen, K. R., Jørgensen, E., Droce, A., Olesen, T., Jensen, B. B., et al. (2013). Real-time optical antimicrobial susceptibility testing. J. Clin. Microbiol. 51, 2047–2053. doi: 10.1128/JCM.00440-13
Freimoser, F. M., Rueda-Mejia, M. P., Tilocca, B., and Migheli, Q. (2019). Biocontrol yeasts: mechanisms and applications. World J. Microbiol. Biotechnol. 35, 1–19. doi: 10.1007/s11274-019-2728-4
Freitas, A. C., Pintado, A. E., Pintado, M. E., and Malcata, F. X. (1999). Organic acids produced by lactobacilli, enterococci and yeasts isolated from Picante cheese. Eur. Food Res. Technol. 209, 434–438. doi: 10.1007/s002170050522
Fröhlich-Wyder, M. T., Arias-Roth, E., and Jakob, E. (2019). Cheese yeasts. Yeast 36, 129–141. doi: 10.1002/yea.3368
Gancedo, C., and Serrano, R. (1989). “Energy-yielding metabolism,” in The Yeasts, eds J. S. Rose and A. H. Harrison (London&New York, NY: Academic Press), 205–259.
Garnier, L., Valence, F., and Mounier, J. (2017). Diversity and control of spoilage fungi in dairy products: an update. Microorganisms 5:42. doi: 10.3390/microorganisms5030042
Gori, K., Knudsen, P. B., Nielsen, K. F., Arneborg, N., and Jespersen, L. (2011). Alcohol-based quorum sensing plays a role in adhesion and sliding motility of the yeast Debaryomyces hansenii. FEMS Yeast Res. 11, 643–652. doi: 10.1111/j.1567-1364.2011.00755.x
Gori, K., Mortensen, H. D., Arneborg, N., and Jespersen, L. (2007). Ammonia production and its possible role as a mediator of communication for Debaryomyces hansenii and other cheese-relevant yeast species. J. Dairy Sci. 90, 5032–5041. doi: 10.3168/jds.2006-750
Gori, K., Sørensen, L. M., Petersen, M. A., Jespersen, L., and Arneborg, N. (2012). Debaryomyces hansenii strains differ in their production of flavor compounds in a cheese-surface model. Microbiologyopen 1, 161–168. doi: 10.1002/mbo3.11
Grzegorczyk, M., Żarowska, B., Restuccia, C., and Cirvilleri, G. (2017). Postharvest biocontrol ability of killer yeasts against Monilinia fructigena and Monilinia fructicola on stone fruit. Food Microbiol. 61, 93–101. doi: 10.1016/j.fm.2016.09.005
Guimarães, A., Venancio, A., and Abrunhosa, L. (2018). Antifungal effect of organic acids from lactic acid bacteria on Penicillium nordicum. Food Addit. Contam. Part A Chem. Anal. Control. Expo. Risk Assess. 35, 1803–1818. doi: 10.1080/19440049.2018.1500718
Gustafsson, L. (1979). The ATP pool in relation to the production of glycerol and heat during growth of the halotolerant yeast Debaryomyces hansenii. Arch. Microbiol. 120, 15–23. doi: 10.1007/BF00413266
Haastrup, M. K., Johansen, P., Malskær, A. H., Castro-Mejía, J. L., Kot, W., Krych, L., et al. (2018). Cheese brines from Danish dairies reveal a complex microbiota comprising several halotolerant bacteria and yeasts. Int. J. Food Microbiol. 285, 173–187. doi: 10.1016/j.ijfoodmicro.2018.08.015
Hermet, A., Méheust, D., Mounier, J., Barbier, G., and Jany, J. L. (2015). Molecular systematics in the genus Mucor with special regards to species encountered in cheese. Fungal Biol. 119:857. doi: 10.1016/j.funbio.2015.06.001
Hernandez-Montiel, L. G., Gutierrez-Perez, E. D., Murillo-Amador, B., Vero, S., Chiquito-Contreras, R. G., and Rincon-Enriquez, G. (2018). Mechanisms employed by Debaryomyces hansenii in biological control of anthracnose disease on papaya fruit. Postharvest Biol. Technol. 139, 31–37. doi: 10.1016/j.postharvbio.2018.01.015
Hernández-Montiel, L. G., Ochoa, J. L., Troyo-Diéguez, E., and Larralde-Corona, C. P. (2010). Biocontrol of postharvest blue mold (Penicillium italicum Wehmer) on Mexican lime by marine and citrus Debaryomyces hansenii isolates. Postharvest Biol. Technol. 56, 181–187. doi: 10.1016/j.postharvbio.2009.12.010
Huang, C., Qian, Y., Viana, T., Siegumfeldt, H., Arneborg, N., Larsen, N., et al. (2020). The quorum-sensing molecule 2-phenylethanol impaired conidial germination, hyphal membrane integrity and growth of Penicillium expansum and Penicillium nordicum. J. Appl. Microbiol. 129, 278–286. doi: 10.1111/jam.14621
Irlinger, F., and Mounier, J. (2009). Microbial interactions in cheese: implications for cheese quality and safety. Curr. Opin. Biotechnol. 20, 142–148. doi: 10.1016/j.copbio.2009.02.016
Koutsoumanis, K., Allende, A., Alvarez-Ordóñez, A., Bolton, D., Bover-Cid, S., Chemaly, M., et al. (2020). Update of the list of QPS-recommended biological agents intentionally added to food or feed as notified to EFSA 12: suitability of taxonomic units notified to EFSA until March 2020. EFSA J. 18:e06174. doi: 10.2903/j.efsa.2020.6174
Kure, C. F., and Skaar, I. (2019). The fungal problem in cheese industry. Curr. Opin. Food Sci. 29, 14–19. doi: 10.1016/j.cofs.2019.07.003
Laitila, A., Alakomi, H. L., Raaska, L., Mattila-Sandholm, T., and Haikara, A. (2002). Antifungal activities of two Lactobacillus plantarum strains against Fusarium moulds in vitro and in malting of barley. J. Appl. Microbiol. 93, 566–576. doi: 10.1046/j.1365-2672.2002.01731.x
Lecanu, L., Ducruet, V., Jouquand, C., Gratadoux, J. J., and Feigenbaum, A. (2002). Optimization of headspace solid-phase microextraction (SPME) for the odor analysis of surface-ripened cheese. J. Agric. Food Chem. 50, 3810–3817. doi: 10.1021/jf0117107
Lessard, M. H., Bélanger, G., St-Gelais, D., and Labrie, S. (2012). The composition of camembert cheese-ripening cultures modulates both mycelial growth and appearance. Appl. Environ. Microbiol. 78, 1813–1819. doi: 10.1128/AEM.06645-11
Liu, J., Sui, Y., Wisniewski, M., Droby, S., and Liu, Y. (2013). Review: utilization of antagonistic yeasts to manage postharvest fungal diseases of fruit. Int. J. Food Microbiol. 167, 153–160. doi: 10.1016/j.ijfoodmicro.2013.09.004
Liu, J., Toldam-Andersen, T. B., Petersen, M. A., Zhang, S., Arneborg, N., and Bredie, W. L. P. (2015). Instrumental and sensory characterisation of Solaris white wines in Denmark. Food Chem. 166, 133–142. doi: 10.1016/j.foodchem.2014.05.148
Liu, S. Q., and Tsao, M. (2009). Biocontrol of dairy moulds by antagonistic dairy yeast Debaryomyces hansenii in yoghurt and cheese at elevated temperatures. Food Control 20, 852–855. doi: 10.1016/j.foodcont.2008.10.006
Liu, S. Q., and Tsao, M. (2010). Biocontrol of spoilage yeasts and moulds by Williopsis saturnus var. saturnus in yoghurt. Nutr. Food Sci. 40, 166–175. doi: 10.1108/00346651011029192
Masoud, W., Poll, L., and Jakobsen, M. (2005). Influence of volatile compounds produced by yeasts predominant during processing of Coffea arabica in East Africa on growth and ochratoxin A (OTA) production by Aspergillus ochraceus. Yeast 22, 1133–1142. doi: 10.1002/yea.1304
Medina-Córdova, N., López-Aguilar, R., Ascencio, F., Castellanos, T., Campa-Córdova, A. I., and Angulo, C. (2016). Biocontrol activity of the marine yeast Debaryomyces hansenii against phytopathogenic fungi and its ability to inhibit mycotoxins production in maize grain (Zea mays L.). Biol. Control 97, 70–79. doi: 10.1016/j.biocontrol.2016.03.006
Medina-Córdova, N., Rosales-Mendoza, S., Hernández-Montiel, L. G., and Angulo, C. (2018). The potential use of Debaryomyces hansenii for the biological control of pathogenic fungi in food. Biol. Control 121, 216–222. doi: 10.1016/j.biocontrol.2018.03.002
Montagna, M. T., Santacroce, M. P., Spilotros, G., Napoli, C., Minervini, F., Papa, A., et al. (2004). Investigation of fungal contamination in sheep and goat cheeses in southern Italy. Mycopathologia 158, 245–249. doi: 10.1023/B:MYCO.0000041897.17673.2c
Mortensen, H. D., Gori, K., Jespersen, L., and Arneborg, N. (2005). Debaryomyces hansenii strains with different cell sizes and surface physicochemical properties adhere differently to a solid agarose surface. FEMS Microbiol. Lett. 249, 165–170. doi: 10.1016/j.femsle.2005.06.009
Núñez, F., Lara, M. S., Peromingo, B., Delgado, J., Sánchez-Montero, L., and Andrade, M. J. (2015). Selection and evaluation of Debaryomyces hansenii isolates as potential bioprotective agents against toxigenic penicillia in dry-fermented sausages. Food Microbiol. 46, 114–120. doi: 10.1016/j.fm.2014.07.019
Padilla, B., Belloch, C., López-Díez, J. J., Flores, M., and Manzanares, P. (2014a). Potential impact of dairy yeasts on the typical flavour of traditional ewes’ and goats’ cheeses. Int. Dairy J. 35, 122–129. doi: 10.1016/j.idairyj.2013.11.002
Padilla, B., Manzanares, P., and Belloch, C. (2014b). Yeast species and genetic heterogeneity within Debaryomyces hansenii along the ripening process of traditional ewes’ and goats’ cheeses. Food Microbiol. 38, 160–166. doi: 10.1016/j.fm.2013.09.002
Petersen, K. M., and Jespersen, L. (2004). Genetic diversity of the species Debaryomyces hansenii and the use of chromosome polymorphism for typing of strains isolated from surface-ripened cheeses. J. Appl. Microbiol. 97, 205–213. doi: 10.1111/j.1365-2672.2004.02293.x
Petersen, K. M., Westall, S., and Jespersen, L. (2002). Microbial succession of Debaryomyces hansenii strains during the production of Danish surfaced-ripened cheeses. J. Dairy Sci. 85, 478–486. doi: 10.3168/jds.S0022-0302(02)74098-8
Quattrini, M., Liang, N., Fortina, M. G., Xiang, S., Curtis, J. M., and Gänzle, M. (2019). Exploiting synergies of sourdough and antifungal organic acids to delay fungal spoilage of bread. Int. J. Food Microbiol. 302, 8–14. doi: 10.1016/j.ijfoodmicro.2018.09.007
Sablé, S., and Cottenceau, G. (1999). Current knowledge of soft cheeses flavor and related compounds. J. Agric. Food Chem. 47, 4825–4836. doi: 10.1021/jf990414f
Sadiq, F. A., Yan, B., Tian, F., Zhao, J., Zhang, H., and Chen, W. (2019). Lactic acid bacteria as antifungal and anti-mycotoxigenic agents: a comprehensive review. Compr. Rev. Food Sci. Food Saf. 18, 1403–1436. doi: 10.1111/1541-4337.12481
Santos, A., and Marquina, D. (2004). Killer toxin of Pichia membranifaciens and its possible use as a biocontrol agent against grey mould disease of grapevine. Microbiology 150, 2527–2534. doi: 10.1099/mic.0.27071-0
Sørensen, L. M., Gori, K., Petersen, M. A., Jespersen, L., and Arneborg, N. (2011). Flavour compound production by Yarrowia lipolytica, Saccharomyces cerevisiae and Debaryomyces hansenii in a cheese-surface model. Int. Dairy J. 21, 970–978. doi: 10.1016/j.idairyj.2011.06.005
Spadaro, D., and Droby, S. (2016). Development of biocontrol products for postharvest diseases of fruit: The importance of elucidating the mechanisms of action of yeast antagonists. Trends Food Sci. Technol. 47, 39–49. doi: 10.1016/j.tifs.2015.11.003
Trinci, A. P. J. (1971). Exponential growth of the germ tubes of fungal spores. J. Gen. Microbiol. 67, 345–348. doi: 10.1099/00221287-67-3-345
Uhlig, S., Jestoi, M., and Parikka, P. (2007). Fusarium avenaceum - The North European situation. Int. J. Food Microbiol. 119, 17–24. doi: 10.1016/j.ijfoodmicro.2007.07.021
Van Den Tempel, T., and Jakobsen, M. (2000). The technological characteristics of Debaryomyces hansenii and Yarrowia lipolytica and their potential as starter cultures for production of Danablu. Int. Dairy J. 10, 263–270. doi: 10.1016/S0958-6946(00)00053-4
Zhang, L., Huang, C., Malskær, A. H., Jespersen, L., Arneborg, N., and Johansen, P. G. (2020). The effects of NaCl and temperature on growth and survival of yeast strains isolated from Danish cheese brines. Curr. Microbiol. 77, 3377–3384. doi: 10.1007/s00284-020-02185-y
Keywords: Debaryomyces hansenii, antagonistic activities, biocontrol, contaminating molds, cheese brine
Citation: Huang C, Zhang L, Johansen PG, Petersen MA, Arneborg N and Jespersen L (2021) Debaryomyces hansenii Strains Isolated From Danish Cheese Brines Act as Biocontrol Agents to Inhibit Germination and Growth of Contaminating Molds. Front. Microbiol. 12:662785. doi: 10.3389/fmicb.2021.662785
Received: 01 February 2021; Accepted: 20 May 2021;
Published: 15 June 2021.
Edited by:
Jian Zhao, University of New South Wales, AustraliaReviewed by:
Chao-An Long, Huazhong Agricultural University, ChinaCopyright © 2021 Huang, Zhang, Johansen, Petersen, Arneborg and Jespersen. This is an open-access article distributed under the terms of the Creative Commons Attribution License (CC BY). The use, distribution or reproduction in other forums is permitted, provided the original author(s) and the copyright owner(s) are credited and that the original publication in this journal is cited, in accordance with accepted academic practice. No use, distribution or reproduction is permitted which does not comply with these terms.
*Correspondence: Lene Jespersen, bGpAZm9vZC5rdS5kaw==
Disclaimer: All claims expressed in this article are solely those of the authors and do not necessarily represent those of their affiliated organizations, or those of the publisher, the editors and the reviewers. Any product that may be evaluated in this article or claim that may be made by its manufacturer is not guaranteed or endorsed by the publisher.
Research integrity at Frontiers
Learn more about the work of our research integrity team to safeguard the quality of each article we publish.