- 1Department of Biological and Agricultural Engineering, Texas A&M University, College Station, TX, United States
- 2Department of Mechanical Engineering, Iowa State University, Ames, IA, United States
- 3Department of Agricultural Sciences, Clemson University, Clemson, SC, United States
- 4Department of Soil and Crop Sciences, Texas A&M University, College Station, TX, United States
High demand for food and water encourages the exploration of new water reuse programs, including treated municipal wastewater usage. However, these sources could contain high contaminant levels posing risks to public health. The objective of this study was to grow and irrigate a leafy green (romaine lettuce) with treated wastewater from a municipal wastewater treatment plant to track Escherichia coli and antibiotic-resistant microorganisms through cultivation and post-harvest storage to assess their fate and prevalence. Contamination levels found in the foliage, leachate, and soil were directly (p < 0.05) related to E. coli concentrations in the irrigation water. Wastewater concentrations from 177 to 423 CFU ml−1 resulted in 15–25% retention in the foliage. Leachate and soil presented means of 231 and 116% retention, respectively. E. coli accumulation on the foliage was observed (p < 0.05) and increased by over 400% during 14-day storage (4°C). From randomly selected E. coli colonies, in all four biomass types, 81 and 34% showed resistance to ampicillin and cephalothin, respectively. Reclaimed wastewater usage for leafy greens cultivation could pose potential health risks, especially considering the bacteria found have a high probability of being antibiotic resistance. Successful reuse of wastewater in agriculture will depend on appropriate mitigation and management strategies to guarantee an inexpensive, efficient, and safe water supply.
Introduction
As world population and demand for food increase, safe water for agricultural use has become increasingly scarce. The water footprint of humanity is estimated at 9,087 km3 year−1, of which agriculture accounts for 92% (Hoekstra and Mekonnen, 2012). In some areas, surface water is not readily available, and other options, such as drilling a well, are not cost-effective. Non-traditional water supplies, such as treated municipal wastewater for irrigation, have the potential to meet increasing water demands and conserve current potable supplies; however, wastewaters often have microbial and chemical contaminants that may affect public health and/or environmental quality. Wastewater treatment (WWT) strategies and advanced irrigation systems may limit exposure of crops, animals, humans, and groundwater to contaminants. Several irrigation practices, such as drip, flood, and subsurface irrigation techniques used with treated wastewater, have been reported to mitigate the risk of contamination (Solomon et al., 2002; Pavione et al., 2013). However, due to the morphology of certain plants, such as lettuce or spinach, commercial-scale production requires canopy (or spray) irrigation. This irrigation process involves water coming into direct contact with the edible foliage, which poses a higher risk of contamination (Robinson, 2002).
A review by De Keuckelaere et al. (2015) reports that there are few site-specific data points available for risk assessment related to use of water and food safety of fresh produce. Specific parameters lacking hard data include rate of pathogen transfer from irrigation water to crops, and pathogen fate, transport, and survival in or on food crops. Furthermore, precise information regarding fecal coliforms, pathogens, and antibiotic-resistant bacteria (ARB) accumulation during and after harvest and their potential effect on future crops along with risks posed to human health are scarce. Therefore, in order to create adequate risk management practices and guidelines for wastewater irrigation in agriculture, site-specific studies of bacteria motility and accumulation are critical, as it is becoming an increasingly popular alternative.
Nowadays, consumption of fresh produce is on the rise, due to its associated health and nutritional benefits. At the same time, fresh produce is one of the leading causes of foodborne illnesses (Rai and Tripathi, 2007) with 377 outbreaks reported by the United States Centers for Disease Control and Prevention (CDC) from 2004 to 2012 (Callejon et al., 2015). Foodborne illnesses can emerge from poor water quality used during fresh produce production. For instance, a multistate outbreak of E. coli O157:H7 infections linked to romaine lettuce that infected 210 people from 36 states and caused five deaths indicated that the source of E. coli O157:H7 was likely from the canal water (Yuma growing region) used to irrigate the romaine lettuce (CDC, 2018). Moreover, the overuse of antibiotics can be directly related to the occurrence and propagation of ARB, which have been increasing rapidly over the past decades (Edberg et al., 2000; WHO, 2006; Bitton, 2010). The ARB issue has been identified by many global public health entities including the World Health Organization and CDC as a critical concern (Bitsch et al., 2014).
Several studies have examined the effects and risks of using wastewater effluents to irrigate fresh produce such as lettuce, spinach, rocket, and tomato (Assadian et al., 2005; Ribera and McCorkle, 2012; De Keuckelaere et al., 2015). Throughout these studies, multiple factors were tested to observe their effects on the prevalence of fecal indicator bacteria (FIB), which were used to estimate the levels of harmful pathogens for risk assessment (Mena and Pillai, 2008; Alam et al., 2014). Additionally, these previous studies reported on the levels of crop contamination; however, these studies did not show how the entire system of foliage, soil, and leachate is affected over time when using wastewater irrigation.
Reclaimed wastewater in agriculture has the potential to provide alternative irrigation and nutrient sources in water-scarce regions and consequently promote water conservation. However, to develop mitigation plans to protect public health, site-specific evaluation of bacteria movement and persistence is needed. In this study, lettuce was irrigated with secondary treated wastewater to track the fate and prevalence of E. coli and ARB throughout the entire system (foliage, soil, and leachate) during cultivation and post-harvest storage.
Materials and Methods
Escherichia coli Monitoring in Fresh Produce Materials
Wastewater
Wastewater was obtained weekly from the Texas A&M WWT Plant, College Station, TX, United States. The wastewater was collected after solids removal and secondary clarification processes. Three liters were collected using a beaker affixed to a pole and placed into sterile plastic jugs for transport to the laboratory. A sample (10 ml) of the wastewater was reserved for further analysis (described below).
Leafy Greens
Twelve young 15-cm romaine lettuce plants (Lactuca sativa var. longifolia, Bonnie Plants, Union Springs, AL, United States) were purchased from a local nursery. The plants were placed into 20-cm diameter plastic pots and filled with EcoScraps moisture retaining potting soil (EcoScraps Co., South Jordan, UT), leaving a 2-cm lip to the top. The potting soil was sterilized in an autoclave for 90 min at 121°C and analyzed by the Texas A&M Department of Soil and Crop Sciences Laboratory (College Station, TX) generating the following results: pH: 7.2, nitrate: 0 ppm, phosphorus: 95 ppm, potassium: 441 ppm, moisture content: 4.44%, soil composition with sand: 91.2%, clay: 2.6%, silt: 6.1%, and total solids: 55.63%. A suggested supplement of nitrogen was applied in the amount of 0.68 g cm−2.
Lettuce plants were transplanted and grown using sterile reverse osmosis (RO) water for 14 days prior to the irrigation experiment. Each row of six plants was grown under two 2-Light T12 fluorescent shop lights (Lithonia Lighting, Conyers, GA) containing four 1.22 m 40-watt fluorescent tube light bulbs (General Electric, Fairfield, CT). The bulbs provided 2,900 lumens each and consisted of two 6,500 K and two 3,000 K color temperature bulbs to resemble natural daylight. The lighting fixtures were plugged into a wall outlet timer that allowed 14 h of continuous light located 15 cm above the plants. Temperature (23 ± 2 C) and relative humidity (55 ± 4%) were kept constant throughout the experiment.
Inoculation
Once a week, for 3 weeks, approximately 15 ml of wastewater was applied directly to each plant’s foliage using a 150-ml sterile spray bottle (Apothecary Products, Inc., Minneapolis, MN) with the nozzle on mist position, thoroughly covering each side of all leaves from a 15-cm distance. Then, 150 ml of the wastewater was poured into each plant’s pot, completely soaking the soil following good agricultural practice recommendations. These irrigation methods were applied to mimic sprinkler and furrow irrigation, which are typically used for leafy greens cultivation (Masabni et al., 2009). Plants were also supplemented with 50 ml of RO water each day for the rest of the week to prevent drying out and wilting. The experimental design is summarized in Figure 1. All procedures were performed inside a biosafety cabinet.
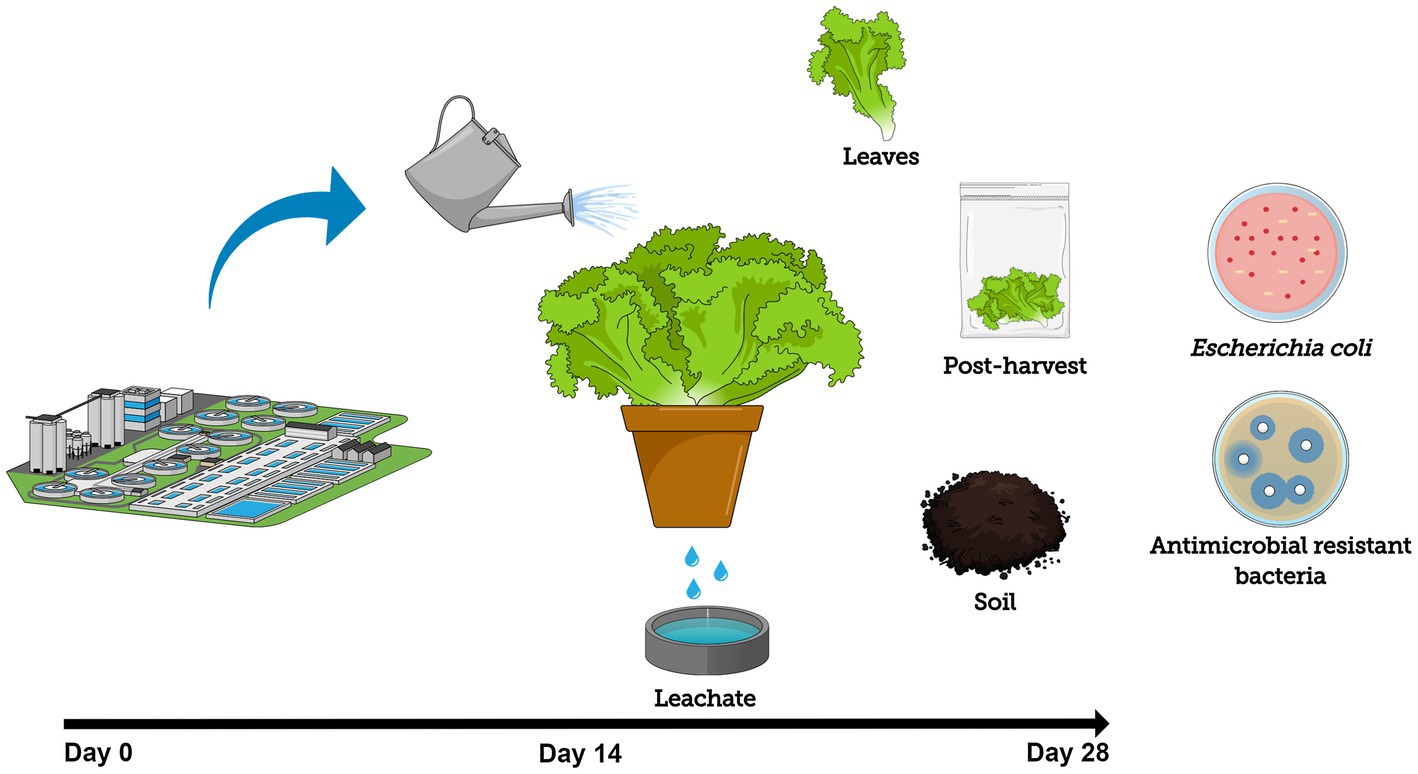
Figure 1. Experimental design schematic showing lettuce cultivation using secondary-treated wastewater as irrigation source to track the fate and prevalence of Escherichia coli and antibiotic-resistant bacteria (ARB) throughout the entire system (foliage, soil, and leachate) during cultivation (14-day period) and post-harvest storage at 4°C (14-day period).
Sample Collection and Analysis
A 10-ml sample of the wastewater was taken and placed into a sterile conical centrifuge tube (VWR International, Radnor, PA). After wastewater irrigation, 10 ml of leachate from each plant was immediately collected from the pot saucer. Foliage samples were collected 1 h after irrigation, by cutting the outermost leaves from their stems. Leaf blades were removed from the vein and cut into 2.5-cm strips. From each plant, 5 g of foliage was stored into sterile Whirl-Pak® bags (eNasco, Fort Atkinson, WI). Then, 10 ml of buffered peptone water (BPW, Becton, Dickinson and Company, Franklin Lakes, NJ, United States) was added to each foliage sample to create a 1:2 ratio of foliage to buffer suspension, and the bags were homogenized for 2 min. Post-harvest foliage samples were collected at the same time as the pre-harvest day 14 samples and stored at 4°C in sterile plastic bags (low-density polyethylene, Whirl-Pak® bags) for 7–14 days when BPW was then added and samples were processed accordingly. Dry weight for foliage samples was obtained by moisture content measurement following AOAC method 930.04 (AOAC, 1990).
Soil samples were collected 6 h after irrigation to allow adequate drainage. From each pot, a sterile 2-cm-diameter core tube was inserted 5 cm deep to collect 2 g of soil. Then, 8 ml of BPW was added to create a 1:4 ratio of soil to buffer suspension. The collected samples were then vortexed for 30 s to homogenize the contents. Subsequently, these samples were allowed to settle for 10 min to separate the soil from buffer.
Aliquots (0.1 ml) of the samples were then plated on MacConkey Agar (Hardy Diagnostics, Santa Maria, CA) using the spread plating method. Several serial dilutions of all samples were plated to ensure samples were within the limits of detection. Plates were incubated overnight at 35°C. Two plates per dilution of each sample were plated, counted, and reported in either CFU g−1 or CFU ml−1 of sample (USFDA, 1998).
Antibiotic-Resistant Bacteria
Ten E. coli colonies from each of the four materials collected (i.e., wastewater, soil, leachate, and foliage) for a total of 40 E. coli isolates were randomly selected per pre-harvest sampling time, and similarly, 10 E. coli colonies from foliage per post-harvest sampling time. Individual colonies were collected, suspended in BPW, streaked on Tryptic soy agar (TSA, Becton, Dickinson and Company, Franklin Lakes, NJ, United States), and incubated overnight at 35°C in accordance with the Kirby-Bauer method (USFDA, 1998). Next, E. coli cell suspensions were then prepared by placing two isolates into tubes containing 5 ml of Tryptic soy broth (TSB, Becton, Dickinson and Company, Franklin Lakes, NJ, United States) and incubating at 35°C for 3 h while shaking at 150 rpm in a water bath (VWR International). Tubes were checked for appropriate turbidity with 0.5 McFarland standard, which corresponds to a 108 CFU ml−1 bacterial cell count (USFDA, 1998).
Escherichia coli suspensions were then re-streaked onto Muller Hinton Agar (MHA, Becton, Dickinson and Company, Franklin Lakes, NJ, United States) plates. Then, antibiotic resistance of the colonies was determined by the Kirby-Bauer method for antibiotic susceptibility. Eight antibiotic susceptibility disks (Becton, Dickinson and Company, Franklin Lakes, NJ) of ampicillin (10 μg), cefoperazone (75 μg), cephalothin (30 μg), ciprofloxacin (5 μg), gentamicin (120 μg), imipenem (10 μg), sulfamethoxazole/trimethoprim (23.75/1.25 μg), and tetracycline (30 μg) were stamped onto each MHA plate using a BBL® Sensi-Disc® 8-place Dispenser (Becton, Dickinson and Company, Franklin Lakes, NJ, United States). The stamped MHA plates were incubated for 24 h at 35°C. Then, the zones of inhibition (ZOI) were measured to determine resistance, intermediate resistance, or susceptibility to each antibiotic, according to the Clinical and Laboratory Standards Institute standards (Bauer et al., 1966).
Data Analysis
All experiments were performed in triplicate as independent experiments, and the results are expressed as mean ± standard deviation. Differences among variables were tested using one-way ANOVA with a significance level of 5%, and significantly, different means were separated by the Tukey HSD test. All data were analyzed using JMP®Pro statistical software (SAS, Cary, NC 27513). Due to the rain event prior to day 14, large input (wastewater) variability of E. coli contamination levels in foliage, soil, and leachate was normalized by transforming CFU ml−1 to percentage of E. coli retention. Response material (i.e., soil, leachate, and foliage) E. coli concentration (CFU ml−1) was divided by wastewater E. coli concentration (CFU ml−1) to yield retention as a percentage. Beginning with pre-harvest data, statistical analysis was performed using a Tukey HSD post-hoc test to compare E. coli prevalence over time among all materials (wastewater, leachate, foliage, and soil). Then, differences in means were analyzed among materials for each sampling time. Finally, post-harvest foliage samples were analyzed to detect mean differences over storage time.
Antibiotic-resistant bacteria samples were analyzed, with focus on the three most common resistance patterns observed from ARB analysis: ampicillin (10 μg), cephalothin (30 μg), and ciprofloxacin (5 μg). In this study, intermediate resistant and resistant bacteria were combined and expressed as “resistant” to simplify the results. Resistance among samples was expressed as a percentage of each sampling population. ARB results were compared over time among all materials. Then, mean differences were analyzed among materials for each sampling time. All analyses were performed by using Tukey HSD post-hoc test to separate differences in means and Levene’s test to test for homoscedasticity.
Results and Discussion
Fresh Produce Materials and E. coli Monitoring
The concentrations of E. coli present in the irrigation water varied and were recorded in log CFU ml−1 as 2.3 ± 0.0, 2.6 ± 0.2, and 5.1 ± 0.1 log CFU ml−1 for days 0, 7, and 14, respectively. There was a large rain event of 32.8 mm (Weather Underground, 2016) in the Bryan/College Station (Texas) area on November 6, 2016, 1 day prior to the collection of the day 14 sample. It was also observed that the WWT plant was not operating at full effectiveness because of a failure in the aeration system, which provides oxygen to microorganisms in the solids removal tank. The WWT plant reported an E. coli concentration of 2.5 ± 0.6 log CFU 100 ml−1 in UV-treated effluent on day 14 and an average of 2.6 ± 2.0 log CFU 100 ml−1 in the 4 days following the rain event and system failure. These are significantly higher concentrations than the average for the rest of the month which was 1.0 ± 0.7 log CFU 100 ml−1, which consequently affected the results by introducing very large concentrations of E. coli that were significantly different (p < 0.05) than the previous two irrigations. The EPA standard for final effluent discharge is a geometric mean of 2.1 log CFU 100 ml−1 (USEPA, 2017).
For irrigation water, including alternative water sources, the E. coli concentration must not exceed 126 CFU 100 ml−1 (geometric mean or 2.10 log CFU 100 ml−1) without triggering a responsive action (FDA, 2017; EC, 2020; EPA, 2021). This study was carried out with an initial E. coli concentration that exceeded regulatory requirements in order to track the fate and prevalence of E. coli and ARB throughout the entire system (foliage, soil, and leachate) during cultivation and post-harvest storage, and also to demonstrate the worst-case scenario in the event of high E. coli levels in irrigation water. Recent reviews have addressed the technological challenges of implementing these federal guidelines for the specific case of alternative water sources (e.g., treated wastewater and brackish water; Markland et al., 2017; Rock et al., 2019) that would benefit with data-informed decision support tools being actively used to monitor microbial contamination (McLamore et al., 2019; Giacobassi et al., 2021).
A recent study conducted by Solaiman et al. (2020) assessed the prevalence of bacteria indicating water quality, fecal contamination and crop contamination risk (E. coli, total coliforms, Enterococcus, and Aeromonas) over a 26-month longitudinal study in the mid-Atlantic region of the United States. For all water types, higher E. coli counts (p < 0.05) were observed in the vegetable crop growing (May-October) than non-growing (November-April) season. Additionally, this study found that bacterial counts in reclaimed water generally met microbial standards by federal guidelines or needed minimal mitigation (Solaiman et al., 2020). Another recent work studied the prevalence of Shigatoxigenic E. coli (STEC) and atypical enteropathogenic E. coli (aEPEC) in untreated surface water and reclaimed water in the mid-Atlantic United States (Haymaker et al., 2019). These pathogenic strains were selected since they have been responsible for several outbreaks of infections associated with leafy greens consumption recently. The study found that 2.35% (12/510) of water samples contained STEC isolates, while 9.0% (46/510) contained aEPEC isolate. The authors pose that STEC isolates at reclaimed water sites may have been introduced after WWTs (Haymaker et al., 2019), which reinforces the need to monitor irrigation water quality to minimize the risk of foodborne illnesses associated with leafy greens.
Initially, soil and leachate displayed higher concentrations of E. coli than foliage until day 14 when foliage surpassed the soil concentrations (Figures 2A–C). Overall, each material showed an increase in E. coli concentration from the previous sampling time except for soil on day 7 (Figure 3B). Foliage consistently increased the concentration throughout cultivation and post-harvest storage (Figure 3A). Meanwhile, leachate samples had the largest concentrations at each sampling time (Figure 3C). Foliage displayed a positive trend in retention with 16, 31, and 43% on days 0, 7, and 14, respectively (Figure 3D). This shows that there was accumulation of E. coli on the foliage throughout the cultivation process. The bacteria were able to survive and persist on foliage for more than 1 week. Similar results were observed by Alam et al. (2014), which studied cessation of irrigation prior to harvest, and how the elapsed time affected E. coli concentration. In a recent study by Allard et al. (2019), fecal indicators, pathogenic bacteria, and total bacterial communities were tracked from a creek water irrigation source used to irrigate fresh produce via drip irrigation to assess the impact of irrigation events on soil and produce microbiota. The study reported that total coliforms in soil were significantly increased immediately and 3 days post-irrigation compared to pre-irrigation, and E. coli level in soil increased after irrigation; however, the difference was not significant, and bacterial die-off was not observed neither in soil nor on produce (Allard et al., 2019).
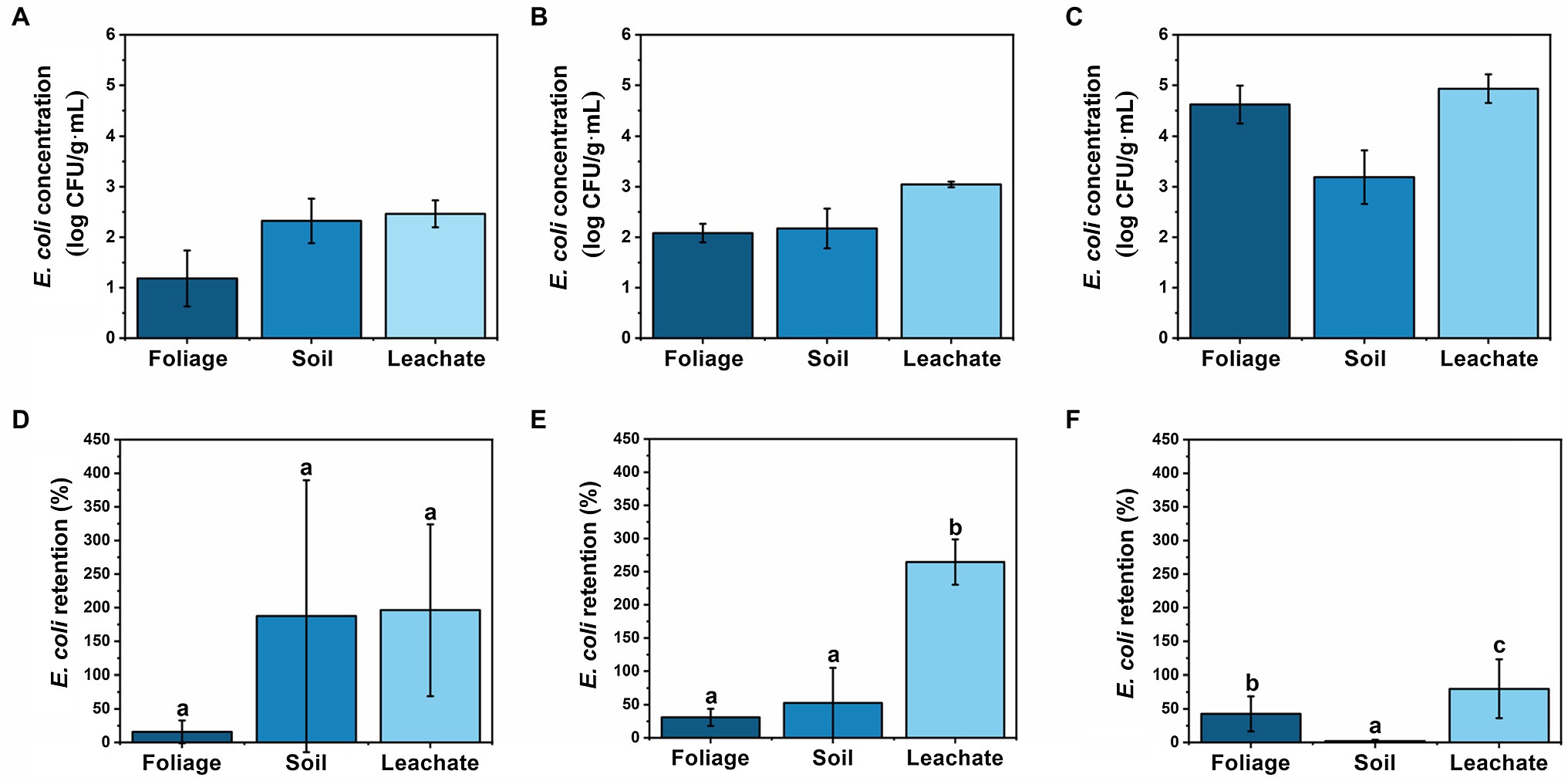
Figure 2. Comparison of E. coli concentration at (A) day 0, (B) day 7, and (C) day 14 in log CFU g−1 or log CFU ml−1 (wet weight basis) and retention on (D) day 0, (E) day 7, and (F) day 14 in % for foliage, soil, and leachate samples, respectively. Retention was calculated by dividing E. coli sample concentration (CFU ml−1 or CFU g−1) by weekly E. coli irrigation water concentration (CFU ml−1). Moisture content of foliage = 91.3% and soil = 4.44%. Sample sizes: wastewater = 10 ml, leachate = 10 ml, foliage = 5 g, and soil = 2 g. Error bars denote standard deviation for arithmetic mean (n = 6 for day 0 foliage and n = 12 for all others). Different letters indicate statistical difference using Tukey-Kramer HSD, α = 0.05.
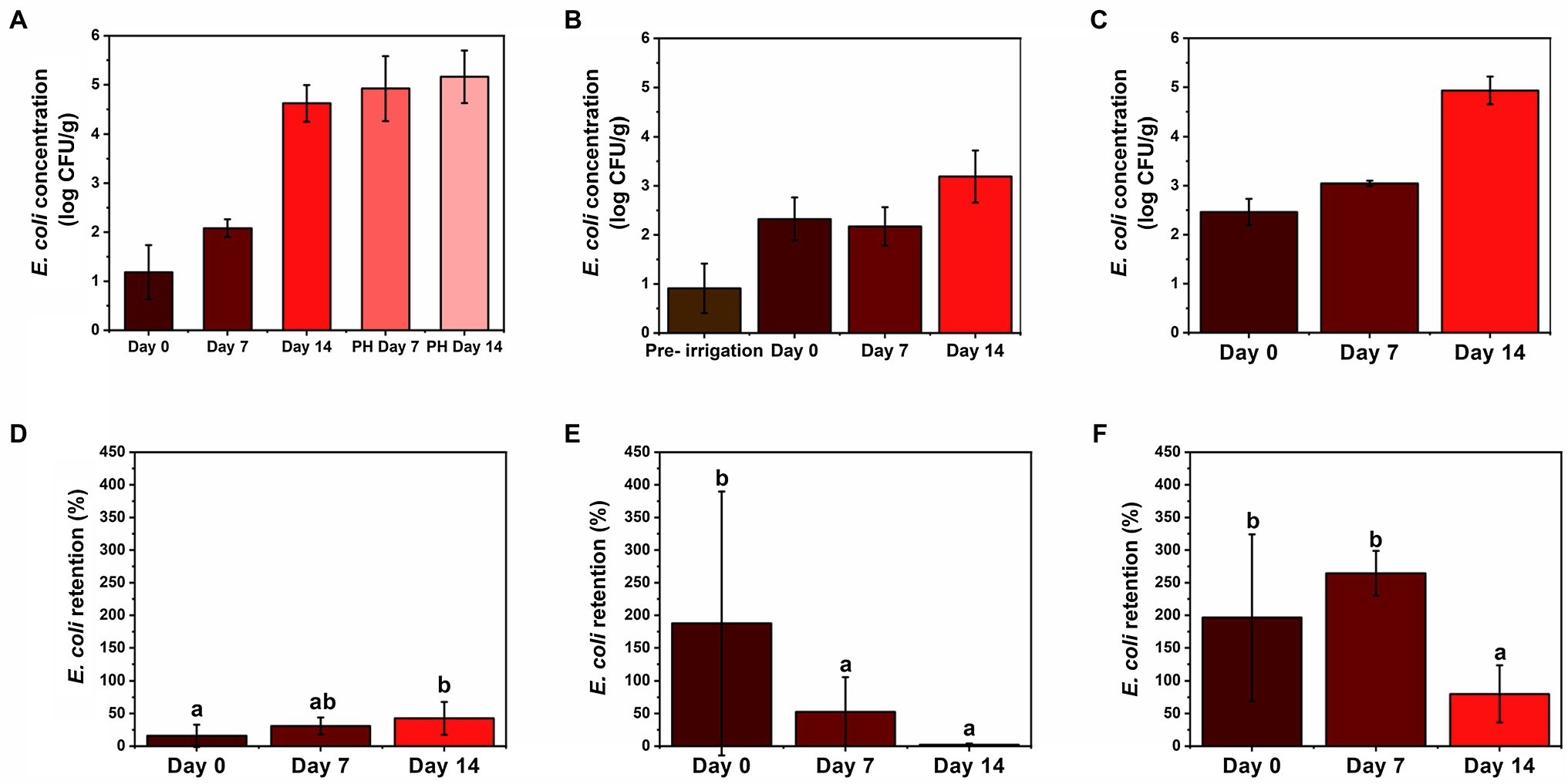
Figure 3. Comparison of E. coli concentration on (A) foliage, (B) soil, and (C) leachate in log CFU g−1 or log CFU ml−1 (wet weight basis) and retention on (D) foliage, (E) soil, and (F) leachate in % over time (days 0, 7, and 14 and post-harvest (PH) days 7 and 14), respectively. Retention was calculated by dividing E. coli sample concentration (CFU ml−1 or CFU g−1) by weekly E. coli irrigation water concentration (CFU ml−1). Moisture content of foliage = 91.3% and soil = 4.44%. Sample sizes: wastewater = 10 ml, leachate = 10 ml, foliage = 5 g, and soil = 2 g. Error bars denote standard deviation for arithmetic mean (n = 6 for day 0 foliage and n = 12 for all others). Different letters indicate statistical difference using Tukey-Kramer HSD, α = 0.05.
Soil and leachate retention rates were often higher than 100% (Figures 3E,F) which shows that the soil was not completely sterile prior to the first irrigation of wastewater on day 0. Possibly, the soil in the lettuce transplants was contaminated with E. coli, which propagated during the first 2 weeks of sterile irrigation prior to day 0. Prior to any wastewater application, there was no detectable E. coli on the foliage, and the autoclaved soil had 13 ± 10 CFU g−1 of E. coli, presenting a 25-fold increase after first irrigation. These findings are similar to Orlofsky et al. (2016), which studied the correlation of FIB and pathogens found on fresh crops irrigated with different types of water, including potable, secondary-treated wastewater (TWW), and tertiary TWW, and found E. coli in soil, which had only been irrigated with potable water. Conversely, in the present study, soil concentrations were relatively consistent but displayed a negative trend in retention with 188, 53, and 2% on days 0, 7, and 14, respectively (Figures 3B,E). This result suggests a maximum contamination load in the soil and that the excess of E. coli will stay in the irrigation water to become leachate. Such results are particularly important for low-growing crops, since they have a closer contact with the ground, increasing the infection risk coming from the contaminated soil (Pavione et al., 2013). Allard et al. (2019) showed that when using drip irrigation to cultivate kale and radish from creek water as irrigation source, even though target pathogens (Salmonella enterica and Listeria monocytogenes) were detected in irrigation water, they were not likely transferred to the field via drip irrigation (i.e., only one post-irrigation kale sample was positive for S. enterica). However, this study reported that elevated total coliforms and E. coli levels in surface water irrigation influenced bacterial communities in soil and on produce (Allard et al., 2019).
Leachate exhibited the largest retention rates among response materials during the cultivation process (Figures 2D–F). The leachate collected the existing E. coli in the soil in addition to the E. coli introduced by the irrigation water, yielding a retention rate greater than 100% for days 0 and 7 (Figure 3F). According to Dwivedi et al. (2016), the saturated water content of the soil is an important parameter in subsurface E. coli transport, and in this study, sterile supplemental water was provided during cultivation to avoid drying out and wilting of the lettuce. Similar to soil, day 14 leachate retention was affected by large input concentration and was significantly less than the previous two sampling times, dropping to 80% (Figure 3F), even though accumulation increased over time (Figure 3C). Our results show that contaminated water can penetrate through 15 cm of soil, but further investigation is needed to determine E. coli’s fate as water percolates down to groundwater reservoirs. According to Stall et al. (2014), depth of soil has a positive effect on reducing E. coli concentrations in leachate.
Escherichia coli concentration in foliage increased during post-harvest storage at 4°C (Figure 3A). Similar results were reported by Lopez-Velasco et al. (2010), which studied the effect of post-harvest storage temperatures (4 and 10°C) and times (5, 10, and 15 days) on E. coli-contaminated spinach. Even though this is not the ideal temperature for E. coli growth (35 ± 2°C), stress response mechanisms can trigger the expression of genes, such as RpoS which is believed to be directly related to the synthesis of internal trehalose in the bacterial cell resulting in the increase of cold resistance (Battesti et al., 2011). E. coli counts increased from 4.6 ± 0.4 log CFU g−1 on the harvest day to 4.9 ± 0.7 log CFU g−1 after 7 days of refrigerated storage. After 14 days of storage, 5.2 ± 0.5 log CFU g−1 was observed, a 200% increase from day 7 of post-harvest storage. Days 7 and 14 were significantly different (p < 0.05) than day 0, but not significantly different from each other (p > 0.05, Figure 3A). These results support the importance of fresh produce being free of any pathogenic microbial contamination during cultivation and processing, as E. coli left on the surface can quickly propagate at recommended storage temperature (4°C) and pose health risks to consumers if no disinfection treatments are applied prior consumption (Lopez-Velasco et al., 2010). For post-harvest, there is a requirement of no detectable generic E. coli in 100 ml of water used in direct contact with produce or on food contact surfaces (FDA, 2017). Additionally, the United States Food and Drug Administration Food Safety Modernization Act established standards in a Produce Safety Rule specific to pre-harvest agricultural water that will come in direct contact with edible portions of fresh produce crops during cultivation including mitigation measure of allowing up to 4 days elapse between irrigation and harvest to allow for bacterial die-off (Havelaar et al., 2017; FDA, 2020, 2021a). These requirements in combination with the “hold and test” policy adopted in 2012 by the United States Department of Agriculture (USDA) significantly reduce the risk of consumer exposure to unsafe products via food recalls (USDA-FSIS, 2013). Notably, to date, there have been no reported foodborne illnesses resulting from the use of reclaimed water (tertiary treated) in irrigation practices in the United States (EPA, 2021; FDA, 2021b).
Antibiotic-Resistant Bacteria
A total of 140 E. coli isolates across all sampling times and materials were tested for antibiotic resistance against eight antibiotics. Ampicillin had the highest recorded resistance among isolates at 81% (n = 114), followed by cephalothin at 34% (n = 47; Figure 4). Silva et al. (2006) reported that WWT plants have generally been ineffective at removing certain strains of resistant bacteria, specifically Enterococcus isolates resistant to the antibiotics ciprofloxacin, erythromycin, and tetracycline, and that the prevalence of ciprofloxacin resistance increased throughout the treatment process. Recently, Chopyk et al. (2020) characterized the taxonomic and functional variations in microbial communities of untreated surface and reclaimed water used in irrigation applications in the mid-Atlantic region of the United States. Among their findings, antimicrobial resistance genes to commonly used antibiotics (aminoglycosides, sulfonamides, rifamycins, macrolides, cephalosporins, fluoroquinolones, and tetracyclines) were found with the highest diversity and abundance in samples from a reclamation facility and a wastewater-impacted freshwater creek (Chopyk et al., 2020). Additionally, the authors reported that bacterial community characteristics varied depending on the date sampled and the specific site (Chopyk et al., 2020), which corroborates with this study findings. Gentamicin and imipenem displayed the lowest rate of resistance, with 1% (n = 1) and 0%, respectively. Several antibiotics including ampicillin (n = 13), cefoperazone (n = 11), cephalothin (n = 14), and ciprofloxacin (n = 13) displayed larger intermediate rates of resistance, ranging from 8 to 10% of all isolates. These findings are important because there is a high probability that these organisms will adapt to their environment and become more resistant, as suggested by Lagacé-Wiens et al. (2013). For this reason, isolates displaying intermediate resistance were categorized as resistant for the remainder of analysis, similar to Laird (2016).
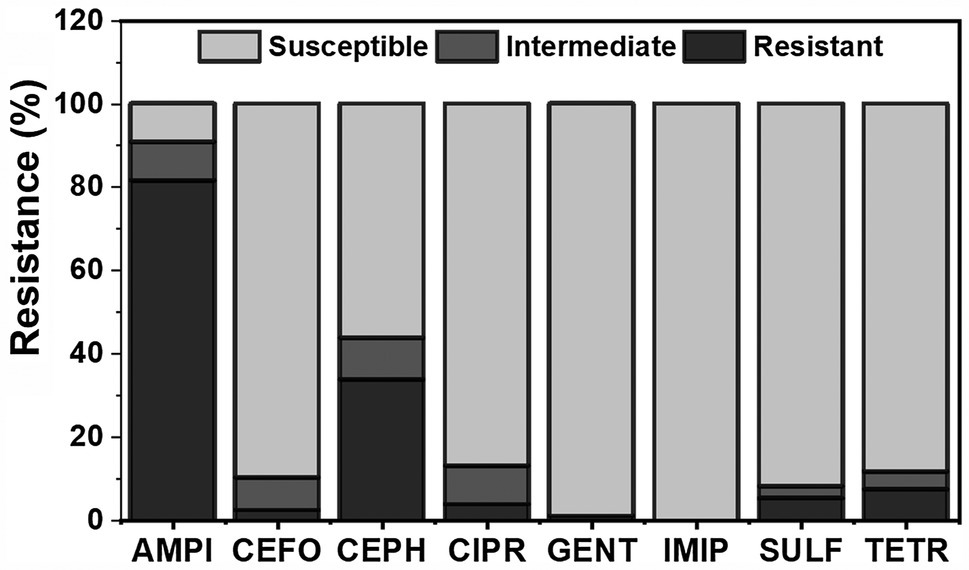
Figure 4. Distribution of antibiotic resistance (resistant, intermediate, and susceptible) among all materials (wastewater, foliage, leachate, and soil) for eight different antibiotics tested (AMPI, ampicillin; CEFO, cefoperazone; CEPH, cephalothin; CIPR, ciprofloxacin; GENT, gentamicin; IMIP, imipenem; SULF, sulfamethoxazole; and TETR, tetracycline) throughout romaine lettuce production (days 0, 7, and 14, post-harvest day 7, and post-harvest day 14). Total E. coli isolates were n = 140.
Three antibiotics with the highest combined prevalence of resistance and intermediate resistance were selected to further investigate their fate and transport throughout fresh produce production (Figure 5). These antibiotics were ampicillin, cephalothin, and ciprofloxacin with 90% (n = 127), 44% (n = 61), and 13% (n = 18) rate of resistance in all isolates, respectively. For an E. coli isolate to be categorized as resistant or intermediate resistant, the bacterial lawn on the Kirby-Bauer plate had to show little to no ZOI around a given antibiotic disc (Bauer et al., 1966). As shown in Figure 5A, ampicillin had the highest overall resistance prevalence in foliage. There was no distinct trend or significant differences in antibiotic resistance over the duration of the experiment. Of the 20 post-harvest foliage samples, 17 samples (85%) were resistant to ampicillin. Conversely, only 5% (n = 1) of post-harvest foliage isolates were resistant to cephalothin and 0% to ciprofloxacin. In the United States, ampicillin and ciprofloxacin are two of the top five antibiotics prescribed to adults (Shapiro et al., 2014). These antibiotics have been found in WWTPs in varying concentrations and treatment plant designs (Batt et al., 2007) due to their frequent use in the past and today’s society, which suggests that treatment plants may be contributing to the prevalence of ARB found downstream.
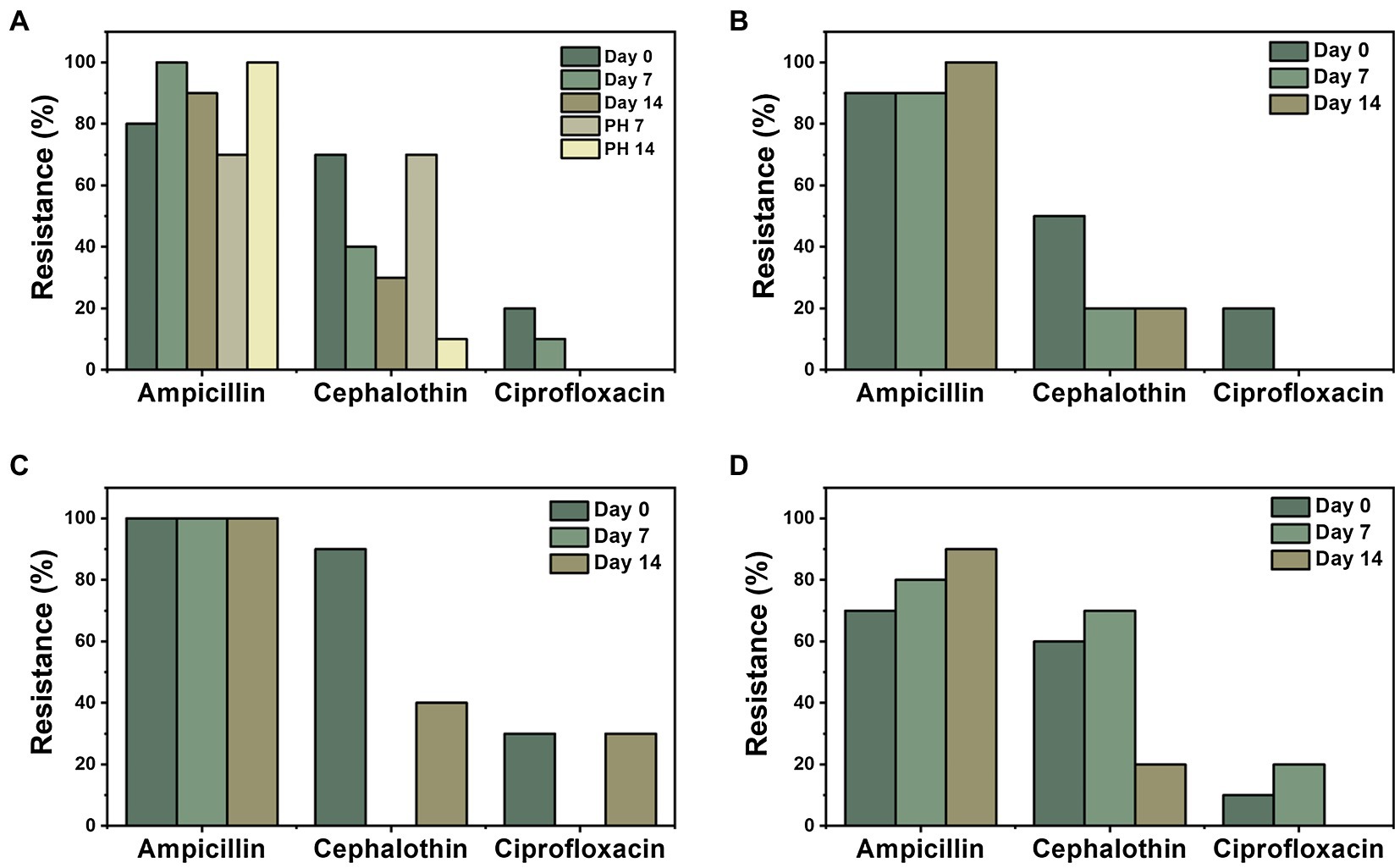
Figure 5. Distribution of ARB over time (days 0, 7, and 14 and post-harvest (PH) days 7 and 14) for three antibiotics that displayed the highest prevalence of resistance (ampicillin, cephalothin, and ciprofloxacin) among response materials: (A) foliage, (B) leachate, (C) soil, and (D) wastewater source.
Day 0 sampling time displayed the largest resistance in isolates from ampicillin (n = 34), followed by cephalothin (n = 27) and ciprofloxacin (n = 8), for all four materials tested (Figure 6). Furthermore, ampicillin was the most prevalent isolate resistance among each material throughout sampling times (n = 127). Ciprofloxacin showed the least resistance (13%) of the three selected antibiotics, for all materials for each sampling day, except for day 7 for soil samples, where both cephalothin and ciprofloxacin showed 0% isolate resistance. For overall ampicillin resistance, soil and wastewater were significantly different from each other (p = 0.049); however, no significant differences were observed from foliage nor leachate. There were no other significant differences among sample materials for cephalothin and ciprofloxacin.
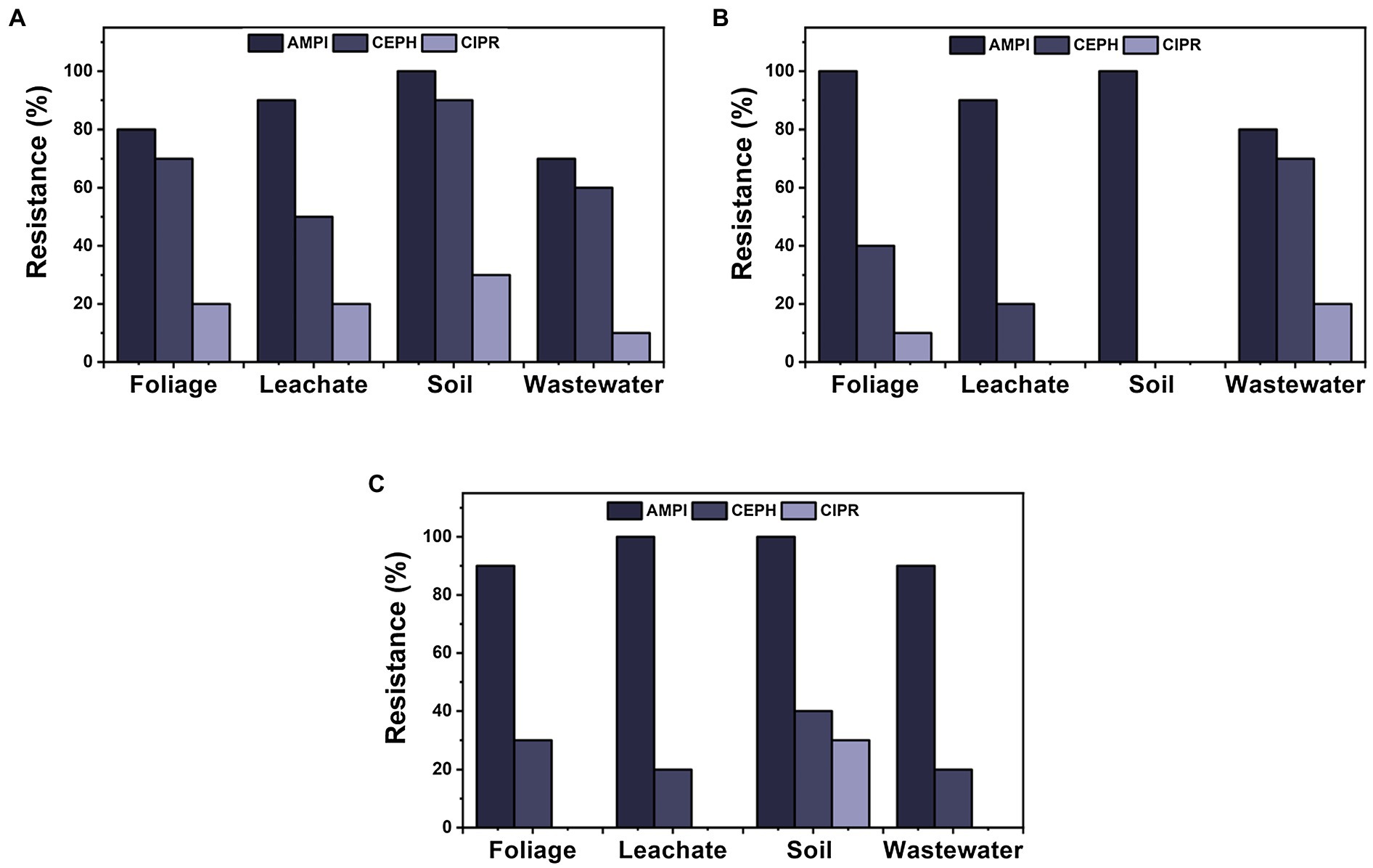
Figure 6. Comparison of ARB for three antibiotics that displayed the highest prevalence of resistance (AMPI, ampicillin; CEPH, cephalothin; and CIPR, ciprofloxacin) among materials over sampling times during cultivation: (A) day 0, (B) day 7, and (C) day 14.
Tracking ARB in leachate and soil in addition to foliage is equally important since these bacteria can make their way back into water sources like rivers and creeks, via runoff and leaching. Furthermore, moist soil provides an optimal environment for resistant bacteria to propagate and pass along resistant genes (Orlofsky et al., 2016). Consequently, water systems are a key vehicle for these bacteria containing antibiotic resistant traits to propagate, multiply, and transfer their resistant genes (Pei et al., 2006). Extensive research on the fate and transport of ARB in water sources resulting from livestock production has been carried out (Addison, 1984; Humphrey et al., 2005). A recent study by Malayil et al. (2020) described the metabolically active bacteria diversity and abundance from reclaimed water and agricultural ponds used as alternative irrigation water sources from the mid-Atlantic United States region. The study observed that antimicrobial resistance and virulence gene profiles appeared to be more diverse and abundant in relic (inactive) DNA than in viable cells (metabolically active) in the tested water types with Actinobacteria, Flavobacterium spp., Pseudomonas spp., and Aeromonas spp. being the most abundant and metabolic-active microorganisms (Malayil et al., 2020). Our study presented some baseline information on the prevalence of viable ARB during fresh produce production irrigated with treated municipal wastewater. Further studies are needed to identify potential mitigation and intervention points in the farm-to-fork continuum when treated wastewater effluents for irrigation of fresh produce.
This study has shown the existence of a direct relationship between the bacterial contamination of irrigation water and the contamination levels of subsequent biomass such as foliage, soil, and leachate. Contaminated soil and leachate can generate health risks for future generations of crops, especially those with low growing foliage that have direct contact with the ground. There are potential public health risks from using non-disinfected wastewater effluent to irrigate crops. The results show that leafy greens irrigated with treated wastewater effluents could pose health risks to humans, especially considering the bacteria found have a high probability of being resistant to one or more antibiotic. Overall, the reuse of wastewater as irrigation source for crops attracts enormous interest, mainly in water scarce regions, and its successful application will depend on management strategies to guarantee an inexpensive, efficient, and safe water supply.
Data Availability Statement
The original contributions presented in the study are included in the article/supplementary material; further inquiries can be directed to the corresponding author.
Author Contributions
Conceptualization and funding acquisition: TG, RK, EM, and CG. Methodology: HS, CP, TG, RK, and CG. Formal analysis: HS, CP, TG, EM, RK, and CG. Resources: TG, RK, and CG. Data curation, original draft preparation, and visualization: HS, CP, and CG. Review and editing: CP, RK, and CG. Supervision: CG. All authors contributed to the article and approved the submitted version.
Funding
This project was supported by Agriculture and Food Research Initiative (AFRI) Competitive Grant no. 2018-67016-27578 awarded as a Center of Excellence from the USDA National Institute of Food and Agriculture and by the Biological and Agricultural Engineering (BAEN) Department. CP would like to acknowledge the Minas Gerais Research Foundation (FAPEMIG) fellowship (grant no. BDS-00467-15).
Conflict of Interest
The authors declare that the research was conducted in the absence of any commercial or financial relationships that could be construed as a potential conflict of interest.
Acknowledgments
The authors thank Terry Smith from Texas A&M Wastewater Treatment Plant. We also thank Prof. Ronald Lacey for his guidance on the experimental design, and Kevin Chacon and John Abuhamad at BAEN Department for their assistance with the experiments. An abstract of this paper was previously published at the 2017 annual Institute of Food Technologists international conference, Las Vegas, NA, United States.
References
Addison, J. B. (1984). Antibiotics in sediments and run-off waters from feedlots. Residue Rev. 92, 1–28. doi: 10.1007/978-1-4612-5266-5_1
Alam, M., Ahlström, C., Burleigh, S., Olsson, C., Ahrné, S., El-Mogy, M., et al. (2014). Prevalence of Escherichia coli O157:H7 on spinach and rocket as affected by inoculum and time to harvest. Sci. Hort. 165, 235–241. doi: 10.1016/j.scienta.2013.10.043
Allard, S. M., Callahan, M. T., Bui, A., Ferelli, A. M. C., Chopyk, J., Chattopadhyay, S., et al. (2019). Creek to table: tracking fecal indicator bacteria, bacterial pathogens, and total bacterial communities from irrigation water to kale and radish crops. Sci. Total Environ. 666, 461–471. doi: 10.1016/j.scitotenv.2019.02.179
AOAC (1990). Moisture in Plants. Association of Official Analytical Collaboration Official Method 930:4.
Assadian, N. W., Di Giovanni, G. D., Enciso, J., Iglesias, J., and Lindemann, W. (2005). The transport of waterborne solutes and bacteriophage in soil subirrigated with a wastewater blend. Agric. Ecosyst. Environ. 111, 279–291. doi: 10.1016/j.agee.2005.05.010
Batt, A. L., Kim, S., and Aga, D. S. (2007). Comparison of the occurrence of antibiotics in four full-scale wastewater treatment plants with varying designs and operations. Chemosphere 68, 428–435. doi: 10.1016/j.chemosphere.2007.01.008
Battesti, A., Majdalani, N., and Gottesman, S. (2011). The RpoS-mediated general stress response in Escherichia coli. Annu. Rev. Microbiol. 65, 189–213. doi: 10.1146/annurev-micro-090110-102946
Bauer, A. W., Kirby, W. M., Sherris, J. C., and Turck, M. (1966). Antibiotic susceptibility testing by a standardized single disk method. Am. J. Clin. Pathol. 45, 493–496. doi: 10.1093/ajcp/45.4_ts.493
Bitsch, V., Koković, N., and Rombach, M. (2014). Risk communication and market effects during foodborne illnesses: a comparative case study of bacterial outbreaks in the US and in Germany. Int. Food Agribus. Man. 17, 97–114. doi: 10.22004/ag.econ.183451
Bitton, G. (2010). Wastewater Microbiology: Bitton/Wastewater Microbiology 4E. Hoboken: Wiley-Blackwell.
Callejon, R. M., Rodriguez-Naranjo, M. I., Ubeda, C., Hornedo-Ortega, R., Garcia-Parrilla, M. C., and Troncoso, A. M. (2015). Reported foodborne outbreaks due to fresh produce in the United States and European Union: trends and causes. Foodborne Pathog. Dis. 12, 32–38. doi: 10.1089/fpd.2014.1821
CDC (2018). Multistate outbreak of E.coli O157:H7 infections linked to Romaine lettuce (final update). Available at: https://www.cdc.gov/ecoli/2018/o157h7-04-18/index.html (Accessed October 25, 2018).
Chopyk, J., Nasko, D. J., Allard, S., Bui, A., Treangen, T., Pop, M., et al. (2020). Comparative metagenomic analysis of microbial taxonomic and functional variations in untreated surface and reclaimed waters used in irrigation applications. Water Res. 169:115250. doi: 10.1016/j.watres.2019.115250
De Keuckelaere, A., Jacxsens, L., Amoah, P., Medema, G., McClure, P., Jaykus, L.-A., et al. (2015). Zero risk does not exist: lessons learned from microbial risk assessment related to use of water and safety of fresh produce. Compr. Rev. Food Sci. Food Saf. 14, 387–410. doi: 10.1111/1541-4337.12140
Dwivedi, D., Mohanty, B. P., and Lesikar, B. J. (2016). Impact of the linked surface water-soil water-groundwater system on transport of E. coli in the subsurface. Water Air Soil Pollut. 227, 1–16. doi: 10.1007/s11270-016-3053-2
EC (2020). European Commision. Water reuse. Regulation on minimim requirements for water reuse enters into force. Available at: https://ec.europa.eu/environment/water/reuse.htm (Accessed March 20, 2021).
Edberg, S. C., Rice, E. W., Karlin, R. J., and Allen, M. J. (2000). Escherichia coli: the best biological drinking water indicator for public health protection. J. Appl. Microbiol. 88, 106S–116S. doi: 10.1111/j.1365-2672.2000.tb05338.x
EPA (2021). Water Reuse and Recycling. United States Environmental Protection Agency. Available at: https://www.epa.gov/waterreuse (Accessed April 02, 2021).
FDA (2017). Food ad Drug Administration. Standards for the growing, harvesting, packing, and holding of produce for human consuption: what you need to know about FDA Regulation: Guidance for industry small entity compliance guide. Available at: https://www.fda.gov/media/107298/download (Accessed March 20, 2021).
FDA (2020). Food and Drug Administration. Standard for the growing, harvesting, packing, and holding of produce for human consuption. Department of Health and Human Services. Available at: https://www.accessdata.fda.gov/scripts/cdrh/cfdocs/cfCFR/CFRSearch.cfm?CFRPart=112 (Accessed March 22, 2021).
FDA (2021a). Food and Drug Administration. FSMA Final Rule on Produce Safety. Standards for the growing, harvesting, paking, and holding of produce for human consumption. Available at: https://www.fda.gov/food/food-safety-modernization-act-fsma/fsma-final-rule-produce-safety (Accessed March 25, 2021).
FDA (2021b). Outbreak of foodborne illness. U.S. Food and Drug Administration. Available at: https://www.fda.gov/food/recalls-outbreaks-emergencies/outbreaks-foodborne-illness (Accessed April 02, 2021).
Giacobassi, C. A., Oliveira, D. A., Pola, C. C., Xiang, D., Tang, Y., Datta, S. P. A., et al. (2021). Sense–Analyze–Respond–Actuate (SARA) paradigm: proof of concept system spanning nanoscale and macroscale actuation for detection of Escherichia coli in aqueous media. Actuators 10:2. doi: 10.3390/act10010002
Havelaar, A. H., Vazquez, K. M., Topalcengiz, Z., Muñoz-Carpena, R., and Danyluk, M. D. (2017). Evaluating the U.S. food safety modernization act produce safety rule standard for microbial quality of agricultural water for growing produce. J. Food Prot. 80, 1832–1841. doi: 10.4315/0362-028X.JFP-17-122
Haymaker, J., Sharma, M., Parveen, S., Hashem, F., May, E. B., Handy, E. T., et al. (2019). Prevalence of Shiga-toxigenic and atypical enteropathogenic Escherichia coli in untreated surface water and reclaimed water in the Mid-Atlantic U.S. Environ. Res. 172, 630–636. doi: 10.1016/j.envres.2019.02.019
Hoekstra, A. Y., and Mekonnen, M. M. (2012). The water footprint of humanity. Proc. Natl. Acad. Sci. U. S. A. 109, 3232–3237. doi: 10.1073/pnas.1109936109
Humphrey, T. J., Jorgensen, F., Frost, J. A., Wadda, H., Dominque, G., Elviss, N. C., et al. (2005). Prevalence and subtypes of ciprofloxacin-resistant Campylobacter spp. in commercial poultry flocks before, during, and after treatment with fluoroquinolones. Antimicrob. Agents Chemother. 49, 690–698. doi: 10.1128/AAC.49.2.690-698.2005
Lagacé-Wiens, P. R. S., Adam, H. J., Low, D. E., Blondeau, J. M., Baxter, M. R., Denisuik, A. J., et al. (2013). Trends in antibiotic resistance over time among pathogens from Canadian hospitals: results of the CANWARD study 2007–11. J. Antimicrob. Chemother. 68, i23–i29. doi: 10.1093/jac/dkt023
Laird, D. (2016). Characterization of antibiotic resistance profiles of surface water bacteria in an urbanizing watershed. master’s thesis. Texas A&M University.
Lopez-Velasco, G., Davis, M., Boyer, R. R., Williams, R. C., and Ponder, M. A. (2010). Alterations of the phylloepiphytic bacterial community associated with interactions of Escherichia coli O157:H7 during storage of packaged spinach at refrigeration temperatures. Food Microbiol. 27, 476–486. doi: 10.1016/j.fm.2009.12.010
Malayil, L., Ramachandran, P., Chattopadhyay, S., Cagle, R., Hittle, L., Ottesen, A., et al. (2020). Metabolically-active bacteria in reclaimed water and ponds revealed using bromodeoxyuridine DNA labeling coupled with 16S rRNA and shotgun sequencing. Water Res. 184:116185. doi: 10.1016/j.watres.2020.116185
Markland, S. M., Ingram, D., Kniel, K. E., and Sharma, M. (2017). Water for agriculture: the convergence of sustainability and safety. Microbiol. Spectrum 5, 143–157. doi: 10.1128/microbiolspec.PFS-0014-2016
Masabni, J., Dainello, F., and Cotner, S. (2009). The Texas vegetable growers handbook. Texas, USA: Texas A&M University, college station.
McLamore, E. S., Palit Austin Datta, S., Morgan, V., Cavallaro, N., Kiker, G., Jenkins, D. M., et al. (2019). SNAPS: Sensor Analytics Point Solutions for detection and decision support systems. Sensors 19:4935. doi: 10.3390/s19224935
Mena, K. D., and Pillai, S. D. (2008). An approach for developing quantitative risk-based microbial standards for fresh produce. J. Water Health 6, 359–364. doi: 10.2166/wh.2008.047
Orlofsky, E., Nirit, B., Mollie, S., Ahuva, V., Maya, B., Arti, K., et al. (2016). Comparable levels of microbial contamination in soil and on tomato crops after drip irrigation with treated wastewater or potable water. Agric. Ecosyst. Environ. 215, 140–150. doi: 10.1016/j.agee.2015.08.008
Pavione, D. M., Bastos, R. K., and Bevilacqua, P. D. (2013). Quantitative microbial risk assessment applied to irrigation of salad crops with waste stabilization pond effluent. Water Sci. Technol. 67, 1208–1215. doi: 10.2166/wst.2013.674
Pei, R., Kim, S.-C., Carlson, K. H., and Pruden, A. (2006). Effect of river landscape on the sediment concentrations of antibiotics and corresponding antibiotic resistance genes (ARG). Water Res. 40, 2427–2435. doi: 10.1016/j.watres.2006.04.017
Rai, P. K., and Tripathi, B. D. (2007). Microbial contamination in vegetables due to irrigation with partially treated municipal wastewater in a tropical city. Int. J. Environ. Health Res. 17, 389–395. doi: 10.1080/09603120701628743
Ribera, L. A., and McCorkle, D. (2012). Economic impact estimate of irrigation water shortages on the Lower Rio Grande Valley agriculture. Available at: http://agecoext.tamu.edu/files/2013/08/EconImpactIrrigWaterShortLRGV.pdf (Accessed July 15, 2015).
Robinson, J. (2002). Alternative approaches to estimate the impact of irrigation water shortages on Rio Grande Valley agriculture. Texas Water Resources Institute. Available at: http://twri.tamu.edu/reports/2002/2002-015/sr2002-015.pdf (Accessed August 20, 2016).
Rock, C. M., Brassill, N., Dery, J. L., Carr, D., McLain, J. E., Bright, K. R., et al. (2019). Review of water quality criteria for water reuse and risk-based implications for irrigated produce under the FDA Food Safety Modernization Act, produce safety rule. Environ. Res. 172, 616–629. doi: 10.1016/j.envres.2018.12.050
Shapiro, D. J., Hicks, L. A., Pavia, A. T., and Hersh, A. L. (2014). Antibiotic prescribing for adults in ambulatory care in the USA, 2007–09. J. Antimicrob. Chemother. 69, 234–240. doi: 10.1093/jac/dkt301
Silva, J., Castillo, G., Callejas, L., Lopez, H., and Olmos, J. (2006). Frequency of transferable multiple antibiotic resistance amongst coliform bacteria isolated from a treated sewage effluent in Antofagasta, Chile. Electron. J. Biotechnol. 9, 533–540. doi: 10.2225/vol9-issue5-fulltext-7
Solaiman, S., Allard, S. M., Callahan, M. T., Jiang, C., Handy, E., East, C., et al. (2020). Longitudinal assessment of the dynamics of Escherichia coli, total coliforms, Enterococcus spp., and Aeromonas spp. in alternative irrigation water sources: a CONSERVE study. Appl. Environ. Microbiol. 86:e00342-20. doi: 10.1128/AEM.00342-20
Solomon, E. B., Potenski, C. J., and Matthews, K. R. (2002). Effect of irrigation method on transmission to and persistence of Escherichia coli O157:H7 on lettuce. J. Food Prot. 65, 673–676. doi: 10.4315/0362-028X-65.4.673
Stall, C., Amoozegar, A., Lindbo, D., Graves, A., and Rashash, D. (2014). Transport of E. coli in a sandy soil as impacted by depth to water table. J. Environ. Health 76, 92–100.
USDA-FSIS (2013). United Stats Department of Agriculture Food Safety and Inspection Services. FSIS compliance guideline for controlling meat and poultry products pending FSIS test results. Available at: https://www.fsis.usda.gov/guidelines/2013-0003 (Accessed March 25, 2021).
USEPA (2017). U.S. Environmental Protection Agency. Title 40. Protection of Environment. Part 131.Water quality standards. Accessed on 07/19/2017. U.S. Government Publishing Office. Available at: http://www.ecfr.gov/cgi-bin/retrieveECFR?gp=&SID=e47d88969d2c1ee17f098f238adf00a9&mc=true&r=PART&n=pt40.22.131 (Accessed July 19, 2017).
USFDA (1998). U.S. Food and Drug Administration. Bacteriological Analytical Manual. 8th Edn. USFDA (USA: College Station, TX).
Weather Underground (2016). "Easterwood, College Station, TX". Available at: https://www.wunderground.com/history/airport/KCLL/2016/11/6/DailyHistory.html?req_city=College+Station&req_state=TX&req_statename=&reqdb.zip=77840&reqdb.magic=1&reqdb.wmo=99999 (Accessed July 11, 2016).
Keywords: wastewater, produce irrigation, water reuse, fecal coliforms prevalence, antibiotic-resistant bacteria, food safety
Citation: Summerlin HN, Pola CC, McLamore ES, Gentry T, Karthikeyan R and Gomes CL (2021) Prevalence of Escherichia coli and Antibiotic-Resistant Bacteria During Fresh Produce Production (Romaine Lettuce) Using Municipal Wastewater Effluents. Front. Microbiol. 12:660047. doi: 10.3389/fmicb.2021.660047
Edited by:
Lisa M. Durso, United States Department of Agriculture, United StatesReviewed by:
Leena Malayil, University of Maryland, United StatesXu Li, University of Nebraska-Lincoln, United States
Copyright © 2021 Summerlin, Pola, McLamore, Gentry, Karthikeyan and Gomes. This is an open-access article distributed under the terms of the Creative Commons Attribution License (CC BY). The use, distribution or reproduction in other forums is permitted, provided the original author(s) and the copyright owner(s) are credited and that the original publication in this journal is cited, in accordance with accepted academic practice. No use, distribution or reproduction is permitted which does not comply with these terms.
*Correspondence: Carmen L. Gomes, Y2FybWVuQGlhc3RhdGUuZWR1