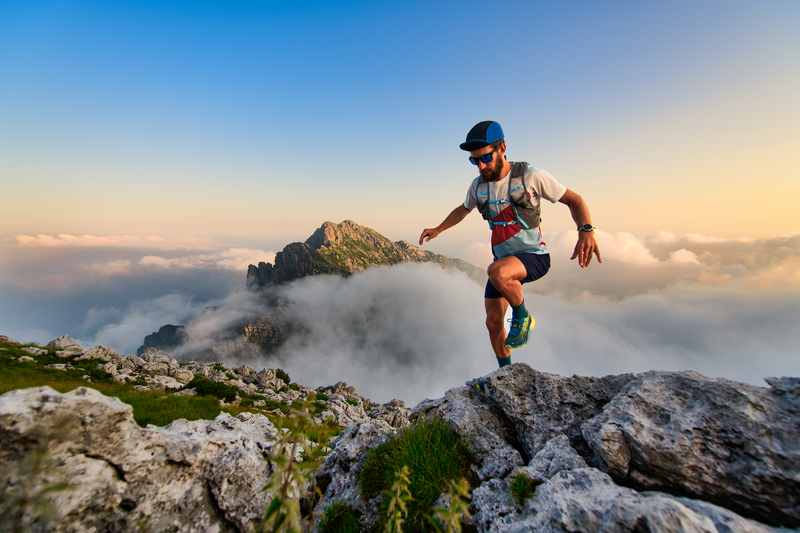
95% of researchers rate our articles as excellent or good
Learn more about the work of our research integrity team to safeguard the quality of each article we publish.
Find out more
ORIGINAL RESEARCH article
Front. Microbiol. , 17 August 2021
Sec. Antimicrobials, Resistance and Chemotherapy
Volume 12 - 2021 | https://doi.org/10.3389/fmicb.2021.659198
This article is part of the Research Topic Antimicrobial Peptides: Molecular Design, Structure Function Relationship and Biosynthesis Optimization View all 22 articles
Plantaricin BM-1, a class IIa bacteriocin produced by Lactobacillus plantarum BM-1, shows obvious antibacterial activity against Escherichia coli. However, the mechanism underlying the action of class IIa bacteriocins against gram-negative bacteria remains to be explored. The purpose of this study was to investigate the role of YbfA, a DUF2517 domain-containing protein, in the response of Escherichia coli K12 to plantaricin BM-1. The growth curve experiment and MIC experiment showed that the sensitivity of E. coli to plantaricin BM-1 was decreased by a ybfA null mutation. Electron microscopy showed that the ybfA null mutation reduced the surface rupture and contraction caused by plantaricin BM-1, and mitigated the effect of plantaricin BM-1 on the morphology of the E. coli cell membrane. Proteomics analysis showed that 323 proteins were differentially expressed in E. coli lacking the ybfA gene (P < 0.05); 118 proteins were downregulated, and 205 proteins were upregulated. The metabolic pathways containing the upregulated proteins mainly included outer membrane proteins, integral components of the plasma membrane, regulation of cell motility, and regulation of locomotion. The metabolic pathways involving the downregulated proteins mainly included outer membrane protein glycine betaine transport, amino-acid betaine transport, and transmembrane signaling receptor activity. The results of the proteomics analysis showed that the protein expression of the BasS/BasR two-component system was significantly increased (P < 0.05). Moreover, the expression levels of downstream proteins regulated by this two-component system were also significantly increased, including DgkA, FliC, and MlaE, which are involved in cell membrane structure and function, and RT-qPCR also confirmed this result. The growth curve showed that the sensitivity of E. coli to plantaricin BM-1 was significantly increased due to deletion of the BasS/BasR two-component system. Thus, deletion of ybfA in E. coli can increase the expression of the BasS/BasR two-component system and positively regulate the structure and function of the cell membrane to reduce the sensitivity to plantaricin BM-1. This will help to explore the mechanism of action of class IIa bacteriocins against gram-negative bacteria.
Bacteriocins are secreted hydrophobic antimicrobial peptides, are 20–60 amino acids in length, and are synthesized by bacterial ribosomes. These peptides have been shown to inhibit both gram-negative and gram-positive food pathogens (Tagg et al., 1995). Bacteriocins produced by lactic acid bacteria (LAB) are regarded as potential natural preservatives because of their high bacteriostasis and low toxicity (Tagg et al., 1995). These bacteriocins can not only be used in food processing and storage but also are expected to be an alternative to antibiotics due to their antiseptic and antibacterial properties (Cotter et al., 2013; Liu et al., 2017).
Bacteriocins can be divided into classes I–IV according to their chemical structure, molecular mass, and stability (Klaenhammer, 1993). Class II bacteriocins are unmodified peptides that are resistant to high temperatures, with or without a leading strand (except for disulfide bond modifications). Class IIa bacteriocins are small molecular weight, thermally stable peptides with strong antibacterial activity against Listeria (Klaenhammer, 1993), and class IIa bacteriocins from LAB are the most abundant and extensively studied bacteriocins. Mature class IIa bacteriocins contain 37–48 amino acids, and the peptide chain is roughly divided into two regions: a positively charged, highly conserved hydrophilic N-terminus and a poorly conserved amphiphilic or hydrophobic C-terminus (Nes et al., 2007). In the highly conserved N-terminal region, there is a “streptococcin box,” which has a YGNGV/L consensus sequence, and two cysteines form a disulfide bond (Nes et al., 2007). At present, there are two model mechanism for the action of class IIa bacteriocins against gram-positive bacteria; the first one is the “barrel-stave” model, in which the hydrophobic part of the bacteriocin interacts with the hydrophobic core of the cell membrane, resulting in the formation of holes in the surface of the cell membrane (Liu et al., 2013a,b), and the second is the “carpet” model, in which the bacteriocin isn't inserted into the hydrophobic core of the plasma membrane, causing the cell membrane to disintegrate without hole formation (Zhang et al., 2012). However, the mechanism of class IIa bacteriocins in gram-negative bacteria remains to be explored.
Two-component systems (TCSs) are one of the most common mechanisms by which bacteria perceive, react, and adapt to environmental changes (Mizuno, 1997). A typical TCSs is composed of a sensor kinase (histidine kinase, HK) located on the inner membrane and a cytoplasmic response regulator (RR) (Mizuno, 1997; Beier and Gross, 2006; Merighi et al., 2009; Gotoh et al., 2010). In most systems, the HK perceives environmental stimuli and autophosphorylates on a conserved histidine residue. Then, the phosphoryl group is transferred to the conserved aspartic acid residue on its homologous RR (Gotoh et al., 2010). The BasS/BasR two-component system is a typical TCS that functions as an iron-zinc induced transcription regulator which directly regulates a group of genes related to metal responsive membrane structure modification and membrane function regulation in E. coli K12 (Yu, 2019). In addition, the BasS/BasR two-component system can also upregulate the expression of genes related to biofilm formation in E. coli (Yu, 2019).
Lactobacillus plantarum BM-1 was isolated from a traditional fermented meat product. It produces a new type IIa bacteriocin, plantaricin BM-1, which has significant inhibitory activity against some foodborne bacteria, including E. coli (Zhang et al., 2013). In our previous study, we found that the expression of YbfA, a protein containing the DUF2517 domain and expressed by a 207 bp gene ybfA, was obviously upregulated in E. coli treated with plantaricin BM-1 (Wang et al., 2020a,b). In this study, we mainly investigated the regulatory role of YbfA in sensitivity to plantaricin BM-1 in E. coli. According to the results, YbfA upregulated the sensitivity of E. coli K12 to plantaricin BM-1 via the BasS/BasR two-component regulatory system.
The chemical and biochemical reagents used in the experiment are shown in Table 1. The chemicals and reagents used were all analytically pure.
The strains and plasmids used in this study are listed in Table 2. Escherichia coli K12 and E. coli JW0688 were cultured in Luria-Bertani (LB) broth at 37°C with aeration at 180 rpm. Lactobacillus plantarum BM-1 was cultured in de Man, Rogosa, and Sharpe (MRS) broth at 37°C with aeration at 180 rpm.
The minimum inhibitory concentration (MIC) was determined according to Clinical and Laboratory Standards Institute (CLSI) guidelines (CLSI, 2012). Plantaricin BM-1 was purified using a two-step method. According to our previous study (Zhang et al., 2013), the method of preparing plantaricin BM-1 is briefly described as follows: L. plantarum BM-1 was cultured in sterile MRS broth at 37°C for 12 h, centrifuged at 10,000 r/min at 4°C for 10 min, and the culture medium was collected. The plantaricin BM-1 was purified through pH-mediated cell adsorption–desorption and cation- exchange chromatography on an SP Sepharose Fast Flow column. The purified plantaricin BM-1 was freeze-dried and stored at −80°C.
The concentration of purified plantaricin BM-1 was quantified using a NanoDrop ND-1000 spectrophotometer. Then, doubling dilution (39.04, 19.52, 9.74, 4.88, 2.44, 1.22, 0.61, and 0.305 mg/mL) were prepared with sterile water under sterile conditions. Adding diluents to 1–8 columns (100 μL per well) of 96-well plates in a diluted gradient, and the same amount of sterile water was added to column 9 as a blank control. Escherichia coli K12 grown to logarithmic growth phase was collected and used to prepare a bacterial suspension at a concentration of 104 CFU/mL. Then, an aliquot of the E. coli K12 suspension (100 μL) was added to each well. After mixing, the 96-well plates were incubated at 37°C for 12 h, and the optical density (OD) at 600 nm (OD600) was determined using an ELISA plate reader. The minimum concentration of plantaricin BM-1 to E. coli K12 and E. coli JW0688 at which no bacterial growth was detected (i.e., no increase in the OD600) was recorded as the MIC (Lv et al., 2014). Each experiment was repeated three times.
The ybfA complemented mutant in E. coli JW0688 was constructed using the lambda Red homologous recombination method (Juhas and Ajioka, 2016). Briefly, the pKD46 plasmid was transformed into competent E. coli JW0688 prepared with cold 0.1 mol/mL CaCl2, and expression of the homologous recombinase in pKD46 was induced by adding L-arabinose (0.5 mg/mL in LB broth) at 30°C. Competent cells of E. coli JW0688 containing the pKD46 plasmid were prepared. The ybfA gene fragment was amplified from E. coli K12 using the primers ybfA-F (5′-3′): AAGGGGGAGAAAAGTATGGAACTCTACAGA and ybfA-R (5′-3′): AAGTTTTGAGTCGTTTCAATAAAAATCACCA (homology underlined), and then transfected into the prepared E. coli JW0688 competent cells. Next, 450 μL of LB broth was added, and the cells were incubated at 37°C for 2 h. Then, the cells were diluted with physiological saline, spread on LB agar, and incubated at 37°C for 12 h. A single colony was selected, and the recombinant was verified using the ybfA-F/R primers. The bacterial that containing ybfA gene was complemented successfully. And the verified complemented E. coli strain was named ReJW0688.
Plantaricin BM-1 was purified as described by (Zhang et al., 2013). Wild-type E. coli K12, E. coli JW0688, and E. coli ReJW0688, at an initial concentration of 2.0 log10 CFU/mL, were cultured in LB broth with or without plantaricin BM-1 (3 × MIC) at 37°C for 14 h. The OD600 was measured every 2 h, and the mean values of triplicate experiments were plotted. All experiments were repeated three times.
Escherichia coli K12 and E. coli JW0688 strains (2.0 log10 CFU/mL) were cultured in LB broth with or without plantaricin BM-1 (3 × MIC) at 37°C for 4 h. The bacterial cells were collected by centrifugation (8,000 rpm, 10 min, 4°C), washed three times with 0.1 mol/L PBS, and then prepared for EM analysis (Wang et al., 2020a,b).
Scanning EM (SEM): The washed cells were fixed with 2.5% glutaraldehyde at 25°C for 2 h and then washed with PBS (0.1 mol/L) three times. The cells were dehydrated with a graded series of ethanol (50, 70, 80, 90, and 100%, three times). The dehydrated samples were treated with 100% tert-butyl alcohol three times and dried for 2 h in a freeze dryer. The sample was placed on the sample table and coated with a 10 nm gold film using an ion sputter coater (Cao et al., 2018). The specimens were imaged using an SEM (SU8010; Hitachi, Japan).
Transmission EM (TEM): Cells were washed with PBS (0.1 mol/L) three times, fixed with 2.5% glutaraldehyde for 2.5 h, and washed with PBS (0.1 mol/L) three times. The dehydration steps were the same as those described for SEM. After dehydration, the sample was washed with acetone three times, soaked in a mixture of acetone and embedding agent (3:1) for 2 h, and then placed on an embedding plate containing pure embedding agent. The embedding plates were polymerized at 40 and 60°C for 48 h. The sample was processed to a trapezoid shape with a surface area of less than 0.2 × 0.2 mm. The embedded material was ultrathin sectioned to a thickness of 50–90 nm. Finally, the ultra-thin sections were stained with uranium and lead dyes and washed for 5 min (Yi et al., 2018). Imaging and analysis of samples were performed using a TEM (7800; Hitachi, Japan).
Escherichia coli K12 and E. coli JW0688 (2.0 log10 CFU/mL) were cultured in LB broth at 37°C for 12 h. After centrifugation, the collected cells were resuspended in lysate buffer (8 M urea, 1% SDS, protease inhibitor). The samples were ground three times and then dissolved on ice for 30 min. Finally, the treated samples were centrifuged at 10,000 rpm for 30 min at 4°C to isolate the total protein. The total protein concentration was measured using the Pierce bicinchoninic acid (BCA) assay (Thermo Fisher, USA).
A 10 μL protein sample was mixed with 90 μL of lysate buffer and then treated with 10 mM TECP (Thermo Scientific, USA) and 40 mM iodoacetamide (Thermo Scientific, USA) at room temperature for 40 min. The sample was mixed with chilled acetone at a volume ratio of 6:1 (sample:acetone), and incubated at −20°C for 4 h to precipitate protein. The samples were centrifuged at 10,000 rpm for 20 min. The precipitated proteins were completely dissolved in 100 μL of 100 mm TEAB and digested with trypsin at a ratio of 1:50. The peptides (100 μg) were mixed with an appropriate amount of TMT reagent for labeling (Thermo Fisher, USA) and incubated at room temperature for 2 h. Following this, hydroxylamine was added, and the sample was incubated at room temperature for 15 min, and then lyophilized in a vacuum freeze dryer for liquid chromatography with tandem mass spectrometry (LC-MS/MS) analysis. The analysis was conducted using a reversed-phase liquid chromatography system (Thermo Scientific Vanquish Flex; Thermo Scientific, USA) equipped with a reverse phase C18 column (ACQUITY UPLC BEH C18 Column, 1.7 μm, 2.1 × 150 mm; Waters, USA) and high pH liquid phase separation TMT labeling peptide. Peptide elution was monitored at 214 nm, and after 5 min, the eluted peptide fractions were collected once per min. A total of 10 fractions were pooled and lyophilized. The dried fractions were identified and quantified on a Q Exactive MS (Thermo Scientific, USA) using a C18 column (75 μm × 25 cm; Thermo Scientific, USA). The parameters for identification were as previously published (Wu et al., 2018).
The data were processed using Proteome DiscovererTM Software 2.2 (Thermo Fisher Scientific, USA). A protein search was conducted using the UniProt-E. coli (strain K12) [83333]-4353s-20190412 database, with a false discovery rate (FDR) ≤0.01. Proteins with at least one unique peptide were used for protein quantification. Differential proteins with fold changes >1.2 (upregulated) or <0.83 (downregulated) were selected. A P value less than 0.05 was considered significant. Gene ontology (GO) and KEGG pathway analysis of the proteomics results were performed using BLAST2GO and KOBAS.
Wild-type E. coli K12 and mutant E. coli JW0688 at an initial concentration of 2.0 log10 CFU/mL, were cultured in LB broth at 37°C for 14 h. Total bacterial RNA was extracted for RT-qPCR. The RT-qPCR reaction conditions were 50°C for 15 min, 95°C for 30 s, 95°C for 10 s, 60°C for 30 s, 40 cycles, using primers as shown in Table 3. Using wild-type E. coli K12 as internal reference factor, the relative gene expression in mutant E. coli JW0688 was calculated by 2−ΔΔCT method.
The MIC of E. coli JW4073 (basS null mutant) and E. coli JW4074 (basR null mutant) to plantaricin BM-1were determinded by using the same method in Determination of minimal inhibitory concentration (MIC).
Three E. coli strains, E. coli JW4073 (basS null mutant), E. coli JW4074 (basR null mutant), and E. coli K12 (at an initial concentration of 2.0 log10 CFU/mL) were treated with 3 × MIC plantaricin BM-1 and cultured in LB broth at 37°C for 24 h. The OD600 was measured every 2 h, and the average of the three growth curves was plotted. All experiments were repeated three times.
After culturing of the bacterial strains with various concentrations of plantaricin BM-1 at 37°C for 12 h, the OD600 of each bacterial suspension was determined, and the concentration at which the OD did not increase, indicating that the bacteria were not growing, was recorded as the MIC. The MIC of plantaricin BM-1 in E. coli K12 and E. coli JW0688 were 1.22 and 8.54 mg/mL respectively.
The 207 bp ybfA gene fragment was amplified from the E. coli K12 genome by PCR using the primer pair ybfA-F/R, which was then used to replace the kanamycin resistance gene in E. coli JW0688 via Red homologous recombination. Genomic DNA was extracted from E. coli JW0688 and the complemented strain E. coli ReJW0688, and PCR was performed on the two extracted genomes using primers ybfA-F/R to confirm proper construction. A 207 bp product was amplified from the complemented strain E. coli ReJW0688, but no amplified band was detected in E. coli JW0688 (Figure 1). Sequencing showed that the amplified gene fragment from E. coli ReJW0688 had the same sequence as the ybfA gene fragment in E. coli K12, which proved that E. coli ReJW0688 was successfully constructed.
Figure 1. Verification of E. coli ReJW0688 construction. PCR products were detected using 2% agarose electrophoresis. Lane M is the 2000 bp DNA Marker, Lanes 1 and 2 contain the PCR product from E. coli ReJW0688, and lanes 3 and 4 contain the PCR products from mutant E. coli JW0688.
The effects of plantaricin BM-1 on the growth of E. coli K12, E.coli JW0688, and E. coli ReJW0688 were assessed by generating standard growth curves (Figure 2). In the absence of plantaricin BM-1, all three strains showed similar exponential growth. The OD600 values of E. coli K12, E. coli JW0688, and E. coli ReJW0688 at 14 h were 0.585, 0.454, and 0.608, respectively. At this time, the growth of E. coli JW0688 was slightly lower than that of wild-type E. coli K12, which might be caused by some changes in growth metabolism and carbon and nitrogen source absorption after ybfA gene mutation was removed. In the presence of plantaricin BM-1, wild-type E. coli K12 grew slowly over the 14 h period, and the OD600 at 14 h was 0.030, indicating that wild-type E. coli K12 is sensitive to plantaricin BM-1. In comparison, E. coli JW0688 grew slowly from 0 to 6 h, and then grew rapidly after 6 h, reaching exponential phase. The OD600 at 14 h was 0.306, which was significantly (P < 0.05) higher than that of wild-type E. coli K12. Our results indicated that the ybfA null mutation reduced the sensitivity of E. coli K12 to plantaricin BM-1. In addition, the growth curve of the complemented strain (E. coli ReJW0688) in the presence of plantaricin BM-1 was similar to that of wild-type E. coli K12. The growth of E. coli ReJW0688 was slow, and the OD600 at 14 h was 0.035, which indicated that the sensitivity of the complemented strain was increased to wild-type levels, further indicating that YbfA regulates the sensitivity of E. coli to plantaricin BM-1.
Figure 2. Effect of plantaricin BM-1 on the growth of wild type E. coli K12, mutant E. coli JW0688, and E. coli ReJW0688. ■, ▴ and ♦ represent the OD600 values of wild type E. coli K12, mutant E. coli JW0688, and E. coli ReJW0688 in the absence of bacteriocin, respectively. •, ▴ and ◂ represent the OD600 values of wild type E. coli K12, mutant E. coli JW0688, and E. coli ReJW0688 in the presence of plantaricin BM-1 (3× MIC), respectively.
Morphological changes in E. coli K12 and E. coli JW0688 with and without plantaricin BM-1 treatment were observed by SEM (Figure 3) and TEM (Figure 4). SEM showed that, in the absence of plantaricin BM-1, the bacterial morphology of E. coli K12 and E. coli JW0688 was not significantly different. The cells were short, rod-shaped, and smooth in appearance, and the edges were intact (Figures 3A,B). In the presence of plantaricin BM-1, the morphology of E. coli JW0688 was not obviously different than the morphology without plantaricin BM-1; the cell surface showed a slight fold contraction, the overall edge was intact, and there was no obvious rupture (Figure 3C). However, the morphology of E. coli K12 significantly changed in the presence of plantaricin BM-1; the cell surface was incomplete, the edges were uneven, and the surface of the cell showed obvious pitting and rupture (Figure 3D).
Figure 3. SEM of E. coli K12 and E. coli JW0688 with and without plantaricin BM-1. (A,B) show wild type E. coli K12 and E. coli JW0688 without plantaricin BM-1, respectively; (C,D) show wild type E. coli JW0688 and E. coli K12 with plantaricin BM-1(magnification: 20,000×).
Figure 4. TEM of E. coli K12 and E. coli JW0688 with and without plantaricin BM-1. (A,B) show wild type E. coli K12 and E. coli JW0688 without plantaricin BM-1, and (C,D) show E. coli JW0688 and E. coli K12 with plantaricin BM-1.
TEM was used to observe structural details within the bacterial cells (Figure 4). In the absence of plantaricin BM-1, the bacterial morphology of the E. coli K12 and E. coli JW0688 strains was not significantly different, and both bacteria showed uniform internal structures and smooth and complete appearance (Figures 4A,B). However, in the presence of plantaricin BM-1, the cytoplasm of E. coli JW0688 showed a slight contraction (Figure 4C). In contrast, the cytoplasm of E. coli K12 had contracted significantly in the presence of plantaricin BM-1, the cell surface had ruptured, the contents leaked out, and the outer membrane was separated from the inner membrane. Thus, the cell morphology was not maintained (Figure 4D).
TMT labeling-based proteomics was applied to characterize the effect of plantaricin BM-1 on E. coli. A total of 2,780 proteins were identified, and 323 proteins were differentially expressed in E. coli JW0688 using a threshold of 1.2-fold (P < 0.05, Figure 5). Among the 323 differentially expressed proteins, 284 proteins were labeled as cell components (CC), and the content related to membrane proteins was 56.04%. Of these 323 differentially expressed proteins, 118 were downregulated and 205 were upregulated in E. coli JW0688 when compared with E. coli K12.
Figure 5. Changes in the E. coli K12 proteome after ybfA deletion. Volcano plots of 2780 identified proteins are shown. The colors indicate the fold changes. Red represents proteins with the fold changes greater than 1.20, and green represents proteins with the fold changes <0.83 (P < 0.05).
Among the downregulated proteins, many proteins related to biological pathways (BP) were significantly enriched, including localization (GO: 0051179), cellular component organization or biogenesis (GO: 0071840), biological regulation (GO: 0065007) and regulation of biological process (GO: 0050789). In terms of molecular function (MF), binding (GO: 0005488), catalytic activity (GO: 0003824) and transporter activity (GO: 0005215) were significantly enriched (Table 4). Among the downregulated differential proteins, the HTH-type transcriptional regulator HdfR (P0A8R9), which negatively regulates the transcription of the main flagellar operon, was downregulated 2.27 fold, and the expression of quorum-sensing molecule AI-2 in the receptor protein transcriptional regulator LsrR (P76141) was reduced 1.35 fold.
Among the upregulated proteins, contains 117 membrane proteins (GO:0016020),these included 16 outer membrane proteins (GO: 0019867) and 15 cell outer membrane proteins (GO: 0009279); membrane part (GO: 0044425), cell part (GO: 0044464), and macromolecular complex (GO: 0032991) were significantly enriched in the cellular component. In addition, among the upregulated proteins, a variety of proteins related to biological pathways were significantly upregulated, including cellular process (GO: GO:0009987), metabolic process (GO: 0008152), single-organism process (GO:0044699), and response to stimulus (GO: 0050896) (Table 5). In biological pathways, we found that the expression of the BasS/BasR two-component system was significantly upregulated in E. coli JW0688.
As show in Table 6, compared with wild-type E. coli, the expressions of BasR (P30843) and BasS (P30844) were respectively 2.24 and 2.26 times higher in E. coli JW0688. Proteins that are targets of BasR include MlaE (P64606), PutF (P07117), DgkA (P0ABN1), PotF (P31133), and others that also increased to a certain extent (Ogasawara et al., 2012). In addition, RT-qPCR results (Figure 6) also showed that the transcriptional levels of basS, basR, mlaE, and dgkA of E. coli JW0688 were all increased significantly (p < 0.05). These results also supported proteomic results, indicated that the expression of BasS/BasR TCS and its downstream regulatory genes in E. coli increased by ybfA gene mutation.
Figure 6. Expression levels of BasS/BasR two-component system and its downstream regulatory genes in E. coli JW0688. *Means significant result.
The MIC of E. coli JW4073 and E. coli JW4074 to plantaricin BM-1 were 0.46 and 0.61 mg/mL, all of which significantly decreased compared with that for wild type E. coli and Figure 7 shows that the growth curves of E. coli K12, E. coli JW4073, and E. coli JW4074 with and without the presence of plantaricin BM-1. During the experiment time, the three strains cultured without plantaricin BM-1 showed a typical growth trend. However, in the first 14 h, the three strains without the presence of plantaricin BM-1 showed no obvious growth. Therefore, growth was inhibited in the first 14 h. Beginning on the 16th hour, the growth of all three strains increased significantly. At 24 h, the OD600 of E. coli K12 was 0.550, while the OD600 of E. coli JW4073 and E. coli JW4074 were 0.274 and 0.301, respectively, (p < 0.05). Based on the OD600 values of E. coli K12, E. coli JW4073, and E. coli JW4074 at 24 h, compared with E. coli K12, E. coli JW4073 and E. coli JW4074 were more sensitive to plantaricin BM-1, that is, plantaricin BM-1 had a more obvious inhibitory effect on the E. coli strains that did not express the BasS/BasR two-component system.
Figure 7. Effect of plantaricin BM-1 on the growth of wild type E. coli K12, E. coli JW4073, and E. coli JW4074. ■, • and ▴ represent the OD600 of wild type E. coli K12, E. coli JW4073, and E. coli JW4074 in the presence of plantaricin BM-1 (3× MIC), respectively. ◂, ▾ and ♦ represent the OD600 of wild type E. coli K12, E. coli JW4073, and E. coli JW4074 without the presence of plantaricin BM-1, respectively.
IIa bacteriocins are small peptides that significantly inhibit the activity of the gram-positive bacterium Listeria monocytogenes (Hu et al., 2012). The antibacterial mechanism of IIa bacteriocins in gram-positive bacteria involves the mannose permeable enzyme , which is located on the bacterial cell membrane, as the receptor. is part of the phosphotransferase system, which is involved the phosphorylation and transport of sugars in some bacteria (Stoll and Goebel, 2010). In L. monocytogenes, is composed of three subunits, IIA and IIB, which comprise the hydrophilic phosphotransferase domain located in the cytoplasm that is involved in phosphorylation and IIC and IID, which are hydrophilic phosphoryl transferase domains located on the membrane that are involved in mannitose transport (Kjos et al., 2011). In E. coli, the mannose phosphotransferase system has been shown not to be a bacteriocin receptor (Kjos et al., 2009), and E. coli can maintain the ultrastructure of cells by accelerating peptidoglycan synthesis and regulating the expression of membrane proteins to resist the damage of bacteriocins (Wang et al., 2020a,b).
The cell membrane of E. coli is the primary barrier by which defend against external harmful substances. Bacteriocins affect bacteria through adsorption and insertion into the cell membrane, resulting in increased membrane permeability, holes, leakage of cell contents, and finally collapse and death (Huang et al., 2010; Cui and Guo, 2018). The barrier effect of the cell membrane is important for resistance to antimicrobial peptides and can protect bacteria from external antimicrobial substances (Liu et al., 2013a,b). Thus, the ability of bacteria to maintain and modify their cell membranes is critical to resistance (Chen and Groisman, 2013).
In this study, an E. coli strain with an ybfA mutation (E. coli JW0688) was used. Studies have shown that YbfA is associated with sensitivity to radiation (Neil et al., 2016) and is a carrier gene of type 2 integrants (Wang et al., 2019). YbfA is also a predicted inner membrane protein. In our growth curve experiment, E. coli JW0688 showed reduced sensitivity to plantaricin BM-1, proteomic analysis and RT-qPCR showed that the expression of the BasS/BasR two-component system and its downstream genes were increased in the ybfA deletion strain. Previous studies have shown that the E. coli BasS/BasR two-component system can regulate the composition of outer membrane lipopolysaccharide or the expression of modification genes through targets, thereby controlling the formation and function of the membrane (Ogasawara et al., 2012) and enhancing membrane permeability and altering the resistance to antimicrobial peptides (Lee et al., 2005). The BasS/BasR two-component system is an iron-zinc induction transcription regulator that is mainly involved in the reaction to divalent metal ions (Fe, Zn) that while essential are also toxic to E. coli (Ogasawara et al., 2012). The regulatory mechanism is similar to that of the PmrA/PmrB two-component system of Salmonella. BasS protein, which is responsible for receiving environmental signals, is stimulated by external signals. The HATPase domain on BasS provides energy for the hisKA domain, phosphorylates the histidine in this domain, and sends a signal to BasR activating it. The BasR protein further regulates other target genes to respond to external stimuli (Gunn, 2008; Yu et al., 2015). Target genes that are activated by BasR usually contain a fixed BasR Box binding sequence consisting of five nucleosides. Studies have shown that some proteins in E. coli contain this binding sequence and have been proven to be BasR targets. These proteins are related to membrane structure and function (Ogasawara et al., 2012). The proteomics experiment showed that DgkA (P0ABN1), MlaE (P64606), PutF (P07117), and PotF (P31133) are downstream proteins regulated by BasR, and their expression levels were significantly changed. These proteins are mainly responsible for regulating cell membrane function and increasing the rigidity of the cell outer membrane (Ogasawara et al., 2012). The increased expression of these proteins indicates that excessive expression of the BasS/BasR two-component system in E. coli K12 can stabilize the expression of E. coli membrane proteins, maintain the function of the cell membrane, and promote the stability of the outer membrane (Ogasawara et al., 2012).
In summary, the ybfA gene in E. coli K12 regulates the expression of the BasS/BasR two-component system: when the ybfA gene is knocked down, the expression of BasS/BasR two-component system in E. coli K12 is increased, which directly affects the expression of proteins regulated by this two-component system. In this study, we found that the expression of the BasS/BasR two-component system was upregulated by ybfA gene deletion, which promoted cell membrane modifications in E. coli, which protected the bacteria and reduce the damage caused by the bacteriocin. In conclusion, YbfA regulates plantaricin BM-1 sensitivity in E. coli via the BasS/BasR two-component regulatory system.
The datasets presented in this study can be found in online repositories. The names of the repository/repositories and accession number(s) can be found below: iProX, IPX0002721000.
XC: conceived and designed the experiments, performed the experiments, and analyzed the data. YL: analyzed the data. JJ, HL, and YH: contributed materials. HZ: conceptualization and methodology. YX: conceived and designed the experiments. All authors contributed to the article and approved the submitted version.
The authors declare that the research was conducted in the absence of any commercial or financial relationships that could be construed as a potential conflict of interest.
All claims expressed in this article are solely those of the authors and do not necessarily represent those of their affiliated organizations, or those of the publisher, the editors and the reviewers. Any product that may be evaluated in this article, or claim that may be made by its manufacturer, is not guaranteed or endorsed by the publisher.
We thank the Research project of Beijing Municipal Commission of Education, this work was supported by the Research project of Beijing Municipal Commission of Education (KM201810020016).
Beier, D., and Gross, R. (2006). Regulation of bacterial virulence by two-component systems. Curr. Opin. Microbiol. 9, 143–152. doi: 10.1016/j.mib.2006.01.005
Cao, S., Zhao, F. K., Du, R. P., Xiao, H. Z., Han, Y., and Zhou, Z. J. (2018). The mode of action of bacteriocin CHQS, a high antibacterial activity bacteriocin produced by Enterococcus faecalis TG2. Food Control 96, 470–478. doi: 10.1016/j.foodcont.2018.09.028
Chen, H. D., and Groisman, E. A. (2013). The Biology of the PmrA/PmrB Two-Component system: the major regulator of lipopolysaccharide modifications. Annu. Rev. Microbiol. 67, 83–112. doi: 10.1146/annurev-micro-092412-155751
CLSI (2012). Methods for Dilution Antimicrobial Susceptibility Tests for Bacteria That Grow Aerobically: Approved Standards. Pittsburgh, PA: Clinical and Laboratory Standards Institute.
Cotter, P. D., Ross, R. P., and Hill, C. (2013). Bacteriocins-a viable alternative to antibiotics. Nat. Rev. Microbiol. 11, 95–105. doi: 10.1038/nrmicro2937
Cui, L., and Guo, W. G. (2018). Antibacterial substances produced by lactic acid bacteria and their mechanism. J. Food Safety Qual. 9, 2578–2584.
Gotoh, Y., Eguchi, T., Watanabe, S., Okamoto, S., Doi, A., and Utsumi, R. (2010). Two-component signal transduction as potential drug targets in pathogenic bacteria. Curr. Opin. Microbiol. 13, 232–239. doi: 10.1016/j.mib.2010.01.008
Gunn, J. S. (2008). The Salmonella PmrAB regulon: Lipopolysaccharide modifications, antimicrobial peptide resistance and more. Trends Microbiol. 16, 264–290. doi: 10.1016/j.tim.2008.03.007
Hu, X. Y., Wang, A. R., Huang, J. Z., Teng, D., and Wang, J. H. (2012). Bacteriocin from Lactic Acid Bacteria. China Feed 20, 9–12. doi: 10.15906/j.cnki.cn11-2975/s.2012.20.004
Huang, X. Q., Gao, X. P., Zhao, G. M., Li, M. Y., Liu, Y. X., Zhang, Q. H., et al. (2010). Review of bacteriostasis mechanisms of antimicrobial peptides. J. Biol. 27, 62–66.
Juhas, M., and Ajioka, J. W. (2016). Lambda Red recombinase-mediated integration of the high molecular weight DNA into the Escherichia coli chromosome. Microb. Cell Fact. 15:172. doi: 10.1186/s12934-016-0571-y
Kjos, M., Nes, I. F., and Diep, D. B. (2009). Class II one-peptide bacteriocins target a phylogenetically defined subgroup of mannose phosphotransferase systems on sensitive cells. Microbiology 155, 2949–2961. doi: 10.1099/mic.0.030015-0
Kjos, M., Nes, I. F., and Diep, D. B. (2011). Mechanisms of resistance to bacteriocins targeting the mannose phosphotransferase system. Appl. Environ. Microbiol. 77, 3335–3342. doi: 10.1128/AEM.02602-10
Klaenhammer, T. R. (1993). Genetics of bacteriocins produced by lactic acid bacteria. FEMS Microbiol. Rev. 12, 39–85. doi: 10.1016/0168-6445(93)90057-G
Lee, L. J., Barrett, J. A., and Poole, R. K. (2005). Genome-Wide Transcriptional Response of Chemostat-Cultured Escherichia coli to Zinc. J. Bacteriol. 187, 1124–1134. doi: 10.1128/JB.187.3.1124-1134.2005
Liu, L. W., Zhang, L. W., Yi, H. X., and Shi, J. (2013a). Research progress on the structure-activity relationship of bacteriocins of IIa lactic acid bacteria. J. Food Indust. Technol. 34, 369–373. doi: 10.13386/j.issn1002-0306.2013.21.033
Liu, W., Ji, S. L., and Song, Y. Z. (2013b). Mechanisms of antimicrobial peptide resistance. Chinese Bull. Life Sci. 25, 1008–1014. doi: 10.13376/j.cbls/2013.10.004
Liu, W. T, Wang, W., Yi, H. X., Zhang, J. M., Han, X., Zhang, L. W., and He, S. H. (2017). Anti-biofilmand antimicrobial activity of bacteriocin lac-b23 from lactic acid bacteria against pseudomonas fluorescens. J. Food Sci. 38, 1–7. doi: 10.7506/spkx1002-6630-201724001
Lv, X., Yi, L. H., Dang, J., Dang, Y., and Liu, B. F. (2014). Purification of novel bacteriocin produced by Lactobacillus coryniformis MXJ 32 for inhibiting bacterial foodborne pathogens including antibiotic-resistant microorganisms. Food Control 46, 264–271. doi: 10.1016/j.foodcont.2014.05.028
Merighi, M., Septer, A. N., Carroll, P. A., Bhatiya, A., Porwollik, F., McClelland, M., et al., (2009). Genome-wide analysis of the PreA/PreB (QseB/QseC) regulon of Salmonella enterica serovar Typhimurium. BMC Microbiol. 9:42. doi: 10.1186/1471-2180-9-42
Mizuno, T. (1997). Compilation of all genes encoding twocomponent phosphotransfer signal transducers in the genome of Escherichia coli. DNA Res. 4, 161–168. doi: 10.1093/dnares/4.2.161
Neil, J. S., Nicholas, P. G., and Deborah, A. H. (2016). Screen for genes involved in radiation survival of Escherichia coli and construction of a reference database. Mutation Res. 793, 1–14. doi: 10.1016/j.mrfmmm.2016.10.001
Nes, I. F., Yoon, S. S., and Diep, D. B. (2007). Ribosomally synthesiszed antimicrobial peptides (Bacteriocins) in lactic acid bacteria. Food Sci. Biotechnol. 16, 675–690. doi: 10.1016/j.foodqual.2007.04.004
Ogasawara, H., Shinohara, S., Yamamoto, K., and Ishihama, A. (2012). Novel regulation targets of the metal-response BasS–BasR two-component system of Escherichia coli. Microbiology. 158, 1482–1492. doi: 10.1099/mic.0.057745-0
Stoll, R., and Goebel, W. (2010). The major PEP-phosphotransferase systems (PTSs) for glucose, mannose and cellobiose of Listeria monocytogenes, and their significance for extra- and intracellular growth. Microbiology 156, 1069–1083. doi: 10.1099/mic.0.034934-0
Tagg, J. R., Jack, R. W., and Ray, B. (1995). Bacteriocins of Gram-positive bacteria. Microbiology 59, 171–200. doi: 10.1128/MMBR.59.2.171-200.1995
Tomoya, B., Takeshi, A., Miki, H., Takai, Y., Okumura, Y., and Baba, M. (2006). Construction of Escherichia coli K-12 in-frame, single-gene knockout mutants: the Keio collection. Mol. Syst. Biol. 2:2006.0008. doi: 10.1038/msb4100050
Wang, H., Xie, Y. H., Zhang, H. W., Jin, J. H., and Zhang, H. X. (2020a). Quantitative proteomic analysis reveals the influence of plantaricin BM-1 on metabolic pathways and peptidoglycan synthesis in Escherichia coli K12. PLoS ONE 15:e0231975. doi: 10.1371/journal.pone.0231975
Wang, H., Xie, Y. H., Zhang, H. W., Zhang, H. X., and Jin, J. H. (2020b). Outer Membrane Channel Protein TolC Regulates Escherichia coli K12 Sensitivity to Plantaricin BM-1 via the CpxR/CpxA two-component regulatory system. Probiotics Antimicrob. Proteins 13, 238–248. doi: 10.1007/s12602-020-09671-6
Wang, Y. N., Liu, X. R., and Liu, Y. S. (2019). Research progress of E. coli integrants. J. Chin. J. Vet. Sci. 39:1858–1863. doi: 10.16303/j.cnki.1005-4545.2019.09.32
Wu, Z., Wang, G., Wang, W., Pan, D., Peng, L., and Lian, L. (2018). Proteomics analysis of the adhesion activity of Lactobacillus acidophilus ATCC 4356 upon growth in an intestine-like pH environment. Proteomics 18:e1700308. doi: 10.1002/pmic.201700308
Yi, L. H., Li, X., Luo, L. L., Lu, Y. Y., Yan, H., Qiao, Z., et al., (2018). A novel bacteriocin BMP11 and its antibacterial mechanism on cell envelope of, Listeria monocytogenes and Cronobacter sakazakii. Food Control 91, 160–169. doi: 10.1016/j.foodcont.2018.03.038
Yu, L. M. D. R. (2019). The regulatory mechanism of BasSR, McbR and AI-2 on the pathogenicity and antibiotic resistance in avian pathogenic Escherichia coli (PhD thesis), Anhui Agricultural University
Yu, Z. L., Qin, W. R., Lin, J. X., Fang, S., and Qiu, J. (2015). Antibacterial mechanisms of polymyxin and bacterial resistance. Biomed Res. Int. 2015:679109. doi: 10.1155/2015/679109
Zhang, H. X., Liu, L., Hao, Y. L., Zhong, S. Q., Liu, H., Han, T., et al., (2013). Isolation and partial characterization of a bacteriocin produced by Lactobacillus plantarum BM-1 isolated from a traditionally fermented Chinese meat product. Microbiol. Immunol. 57, 746–755. doi: 10.1111/1348-0421.12091
Keywords: bacteriocins, proteome, YbfA, BasS/BasR two-component, biofilm
Citation: Chen X, Liu Y, Jin J, Liu H, Hao Y, Zhang H and Xie Y (2021) YbfA Regulates the Sensitivity of Escherichia coli K12 to Plantaricin BM-1 via the BasS/BasR Two-Component Regulatory System. Front. Microbiol. 12:659198. doi: 10.3389/fmicb.2021.659198
Received: 27 January 2021; Accepted: 23 July 2021;
Published: 17 August 2021.
Edited by:
Octavio Luiz Franco, Catholic University of Brasilia (UCB), BrazilReviewed by:
Apon Zaenal Mustopa, Indonesia Institute of Sciences (LIPI), IndonesiaCopyright © 2021 Chen, Liu, Jin, Liu, Hao, Zhang and Xie. This is an open-access article distributed under the terms of the Creative Commons Attribution License (CC BY). The use, distribution or reproduction in other forums is permitted, provided the original author(s) and the copyright owner(s) are credited and that the original publication in this journal is cited, in accordance with accepted academic practice. No use, distribution or reproduction is permitted which does not comply with these terms.
*Correspondence: Yuanhong Xie, eGlleXVhbmhAMTYzLmNvbQ==; Hongxing Zhang, aHh6aGFuZzUxQDE2My5jb20=
Disclaimer: All claims expressed in this article are solely those of the authors and do not necessarily represent those of their affiliated organizations, or those of the publisher, the editors and the reviewers. Any product that may be evaluated in this article or claim that may be made by its manufacturer is not guaranteed or endorsed by the publisher.
Research integrity at Frontiers
Learn more about the work of our research integrity team to safeguard the quality of each article we publish.